- Space and Telecommunications Sector, Department of Electrical and Computer Engineering, Demokritos University of Thrace, Xanthi, Greece
The ultra low frequency (ULF) electromagnetic (EM) wave activity usually recorded on Earth’s ground has been found to depend on various types of space weather. In addition ULF waves observed before an earthquake have been hypothesized to be a result of geotectonic processes. In this study we elaborate for the first time the origin of sub-ULF (<1 msec) magnetic field waves before an earthquake (Chi-Chi/Taiwan, 20.9.1999) by comparing simultaneously obtained measurements in the interplanetary space (ACE satellite) and on the Earth’s ground (Taiwan). The most striking result of our data analysis, during a period of 7 weeks, is that the detection of four groups of sub-ULF waves in Taiwan coincide in time with the quasi-periodic detection of two solar wind streams by the satellite ACE with approximately the solar rotation period (∼28 days). The high speed solar wind streams (HSSs) in the interplanetary space were accompanied by sub-ULF Alfvén wave activity, quasi-periodic southward IMF and solar wind density perturbations, which are known as triggering agents of magnetic storm activity. The four HSSs were followed by long lasting decreases in the magnetic field in Taiwan. The whole data set examined in this study strongly suggest that the subULF magnetic field waves observed in Taiwan before the Chi-Chi 1999 earthquake is a normal consequence of the incident of HSSs to the magnetosphere. We provide some observational evidence that the sub-ULF electromagnetic radiation on the Earth was most probably a partner to (not a result of) geotectonic processes preparing the Taiwan 1999 earthquake.
Introduction
The Origin of Terrestrial Ultra Low Frequency Electromagnetic Waves and the Taiwan Chi-Chi 7.7Mw 1999 Earthquake
Ultra low frequency (ULF) waves observed by terrestrial observatories is a matter of scientific research of great interest. ULF waves provide significant information about geomagnetic processes and earthquake (EQ) preparation processes and they have significant influence on biological organisms (Reichmanis et al., 1979; Pilipenko, 1990; Poole, 1993; Kivelson and Russell, 1995; Poole et al., 1993; McPherron, 2005; Pulinets and Boyarchuk, 2004; Grimalsky et al., 2010; Anagnostopoulos. 2015, 2016). Despite the extensive research done so far, there exist many open questions about their generating mechanisms. For instance, sub-ULF (<1 msec) waves have been reported before several earthquakes. However, low geomagnetic storm activity has been considered as a criterion of their terrestrial origin, without any other direct evidence. Therefore the question is: is it possible that sub-ULF (<1 msec) wave activity and moderate or low geomagnetic storm activity are related with solar activity and subsequent space weather? The answer to this question is positive from the side of space science. However, there is not, as far as we know, any study which directly compares space weather and terrestrial observations to address the above question of ULF activity before a particular earthquake. For this reason, in this case study, the subULF wave activity before the great Taiwan 1999 earthquake is reexamined in order to elaborate its origin.
The Taiwan 7.7 Mw earthquake (EQ) was one of the most catastrophic earthquake of the 20th century in Taiwan (Ma et al., 1999). The EQ struck the small town Chi-Chi at 1:47 local time (17:47 UT), 21 September (20 September), 1999. The earthquake epicenter was located at geographic latitude 23.850N and longitude 120.780E, with a depth of 8.0 km. In this earthquake, approximately 2500 lives were lost, about 11,305 people injured, more than 100,000 people made homeless, thousands of buildings were destroyed and NT$300 billion worth of damage was done. One month after the disastrous Chi-Chi earthquake (October 22, 199) another great EQ (Mw 6.4) occurred in Chia-yi, in southern Taiwan (Chang and Wang, 2002; Huang et al., 2006).
Several investigators have reported various types of precursory electromagnetic (EM) phenomena, which occurred before the Chi-Chi 1999 earthquake. Almost all of the relative studies suggested that the precursory electromagnetic signals were the results of terrestrial processes. Since most researchers accept the hypothesis that seismic activity is a phenomenon related with a physical process in the interior of Earth, no systematic research has been made on the space weather and its possible effects on the Chi-Chi pre-EQ EM signals as well. The presence of a “weak” geomagnetic activity before the Chi-Chi EQ was hypothesized as an evidence of non space effects in Taiwan before the great 1999 EQ (Yen et al., 2004), according to the generally accepted geocentric paradigm.
Today the situation is different in understanding the importance of space weather on the planet Earth. Ιn this respect, several studies have recently provided overwhelming evidence that the quiet Sun producing “weak” storms is a significant agent provoking terrestrial seismicity (Anagnostopoulos et al., 2021 and references therein). However, despite the great achievements in space weather, the scientific literature lacks any studies examining the possible space origin of any pre-EQ electromagnetic (EM) precursory signals based on a direct comparison of simultaneously obtained space and terrestrial measurements.
The Sun is the principal source of energy in our Solar System and the agent of many physical processes taking place in Earth’s magnetosphere, ionosphere, atmosphere, lithosphere and biosphere (Odintsov et al., 2007; Gray et al., 2010; Vencloviene et al., 2012; Anagnostopoulos and Papandreou, 2012; Anagnostopoulos et al., 2015; Patsourakos et al., 2016; Kumar, A., and Kumar, S., 2018; Firoz, 2019; Tsurutani et al., 2020). Moreover, nowadays our knowledge about the Sun-Earth electromagnetic relationships allows a new insight into important physical and biological phenomena of great social interest (Anagnostopoulos et al., 2021; Zenchenko and Breus, 2021).
The solar wind-magnetospheric interaction is one of the most important issues in space research. In particular, space science has provided significant information on the role of solar wind turbulence on the energy and momentum transfer from the Sun to the Earth’s environment (Dungey, 1961; Borovsky and Valdivia 2018). Moreover, in recent years, rich information has been accumulated concerning the impact of high speed solar wind streams (HSSs) on the status of the geomagnetosphere, and, in particular, on the trapped radiation belts (Kataoka, R. and Miyoshi, Y. 2006; Borovsky and Denton, 2006; Potapov, 2013, Baker et al., 2018; Richardson, 2018). Furthermore, HSSs periods were found to be related with enhanced seismicity occurring during “quiet” geomagnetic periods (Anagnostopoulos et al., 2021 and references therein).
This paper is devoted to elaborating on the space processes preceding a great earthquake. To this end, we investigate, for the first time, simultaneously obtained space and terrestrial observations for a specific earthquake, in order to extract information on the space weather – seismicity relationship additional to that received from our previous statistical study (Anagnostopoulos et al., 2021). We address the question of whether the ULF waves (<1 msec) recorded by the magnetic field observatories in Taiwan some weeks before the Chi-Chi EQ could be the result of space weather, during a quiet Sun.
Theoretical and Observational Framework
Ultra Low Frequency Electromagnetic Waves in Space and on the Ground
ULF waves in the magnetosphere and on Earth, known as Pc or Pi pulsations (McPherron, 2005), are one of the most known phenomena in space science. There is a wide variety of ULF types, which are classified according to their form and frequency band.
ULF waves of different frequencies and polarizations observed on the ground may originate from different regions of the magnetosphere, the distant space or the Sun’s environment. Ultra-low frequency waves are detected on the ground as geomagnetic pulsations, ranging in frequency from some Hz to less than 1 mHz and numerous theories have been proposed to explain their origin (Pilipenko, 1990; McPherron, 2005; Pulinets and Boyarchuk, 2004; Grimalsky et al., 2010)
On the other hand, there have been many reports of ULF waves observed before an earthquake, which have been considered as originating from tectonic processes. Many other electromagnetic EM field changes have also been reported before earthquakes on the ground, in the atmosphere and in a wide range of frequency of the EM spectrum as sub-ULF, ULF/ELF, VLF, IR (Hayakawa and Fujinawa, 1994; Molchanov and Hayakawa, 1998; Ouzounov and Freund, 2004; Hattori, 2004; Liu et al., 2004; Balasis and Mandea, 2007; Hayakawa et al., 2010; Uyeda et al., 2009, Pulinets and Ouzounov, 2011; Athanasiou et al., 2011, 2014; Freund et al., 2012; ZhangShen et al., 2012; Tramutoli et al., 2015, De Santis et al., 2015, 2017; Ouzounov et al., 2018; Han, 2020). Furthermore, some characteristic charged particle variations have been reported in the ionosphere and the inner radiation belt before great EQs, as, for instance, variations in TEC and in radiation belt electron precipitation as well as ionospheric plasma perturbations (Lui et al., 2000, 2001; Pulinets and Boyarchuk, 2004; Akhoondzadeh et al., 2010; Pulinets and Ouzounov, 2011; Sidiropoulos et al., 2011; Anagnostopoulos et al., 2012). Broad band VLF wave activity is related with electron precipitation from the inner radiation belts. Extensive summary of pre-earthquake phenomena have been reported by Ouzounov et al. (2018) and Pulinets and Ouzounov, 2018.
Enhanced ULF EM activity has been considered to be a significant earthquake precursory signal (Hattori, 2004; Hayakawa et al., 2007; Zhang et al., 2012; Athanasiou et al., 2014). In general, the detection of various electromagnetic perturbations before fault ruptures has been proposed as a useful way to monitor activities of Earth’s crust.
An increasing number of investigators have used electromagnetic methods in the last two decades in order to achieve earthquake prediction. Furthermore, satellite observations have been extensively used in earthquake prediction research. The first space mission dedicated to the detection of pre-EQ EM emissions was the French satellite DEMETER (Parrot et al., 2006; Sauvaud et al., 2006), during the years 2004–2010. DEMETER mission greatly aided the scientific community in improving its methodologies on earthquake prediction research. Similarly, a second space mission, the Chinese satellite CSES (Shen et al., 2018) is currently in orbit around the Earth and provides us with current data.
In the scientific community the ultra low frequency electromagnetic radiation has been considered to be an important earthquake precursor, because of its deeper skin depths (Hattori and Han, 2018). ULF magnetic field activity has been recognized as a precursor to well-known and broadly discussed earthquakes, such as in Loma Prieta, Guam, Sumatra 2004, and Haiti 2010 (Fraser-Smith et al., 1994; Hayakawa et al., 1996; Athanasiou et al., 2011).
A short discussion about the origin of ULF wave activity before an EQ was made in the case of the Loma Prieta earthquake. Campbell (2009) compared magnetic field records from Fresno, Bolder and Tucson with magnetic field observations from Stanford University (Fraser-Smith et al., 1994) near Loma Prieta, and he found that a similar trend for the ULF wave activity characterized all these locations. Campbell concluded that the ULF waves recorded before the Loma Prieta earthquake was not a local signal originated from local preparation processes.
Thus, we think that we should address the following questions: 1) Are all types of pre-EQ ULF magnetic field waves the result of tectonic stresses? 2) Is it possible for some types of preearthquake ULF wave activity to originate from tectonic processes and some other types from some space phenomena? 3) Is there any observational evidence supporting the space origin of the subULF electromagnetic radiation observed before the Chi-Chi 1999 earthquake?
Space Weather and Solar Wind Ultra Low Frequency Waves
There is increasing evidence that supports the concept of two supplementary sources contributing to the generation of earthquakes: tectonic stresses and space weather phemomena. There are several studies, which have provided significant evidence that seismicity is related with solar cycle (SC) phase (Simpson, 1967; Gousheva et al., 2003; Bakhmutov et al., 2007; Odintsov et al., 2007; Khain and Khalilov, 2008; Straser and Cataldi, 2015) and geomagnetic activity (Sobolev et al., 2001; Duma and Ruzhin, 2003; Bakhmutov et al., 2007; Urata et al., 2018). Love and Thomas (2013) disputed the solar-terrestrial interaction in triggering great earthquakes, but they tested an incorrect hypothesis (active Sun affects seismicity) and wrong time scale (1 year) of averaged sunspot number (Anagnostopoulos et al., 2021).
The long-term (1964–2013) relationship between the occurrence of earthquakes with magnitudes M ≥ 5 was investigated by Gulyaeva (2014); it was found that the global number of earthquakes tends to grow towards the solar cycle minimum.
Anagnostopoulos et al. (2021) performed various statistical studies based on solar, space and seismological data between 1900 and 2017 and we also found strong evidence that both the great earthquakes as well as high global seismic energy release occur during the rising and the decay phase of SC as well as at specific times of the maximum solar cycle phase with temporal (# months) weak solar activity. In general, the analysis of monthly averaged solar sunspot number (SSN) confirms a systematic and significant negative correlation of the seismic activity with the SSN (Gulyeva, 2014; Rekapali, 2014; Anagnostopoulos et al., 2021).
During periods with small SSNs, coronal hole (CH) driven-recurrent HSSs and corοtating interaction regions (CIRs) rotate in space with the solar rotation period of ∼27 days. This periodicity is clear in various kinds of observations in the interplanetary space, the terrestrial magnetosphere and the ionosphere. For instance, solar wind speed and auroral electron power exhibit solar rotational periodicities (Emery et al., 2009). Since the ∼27-days period recurrent solar wind structures influences Earth via EM interactions, the ∼27 days-periodicity might be found in seismological data. Indeed, the power spectrum analysis of 4-h averaged global energy release values demonstrated a ∼27-days periodicity during the decay phase of SC22 and SC23 and at the maximum of SC23 (Anagnostopoulos et al., 2021). This result is consistent with the concept that HSSs, which emanate from the coronal hole of the quiet Sun, provoke seismic activity on the planet Earth.
Since we found strong evidence that the impact of CH-driven HSSs/CIRs on the Earth’s magnetosphere trigger earthquakes, the next question is how the magnetosphere mediates the energy transfer of the solar wind plasma to the ionosphere and the lithosphere. Anagnostopoulos et al. (2021) put forward the hypothesis that solar wind magnetohydrodynamic (MHD) waves, which are transformed into electromagnetic waves in the ionosphere, may mediate space weather with seismicity. Indeed, Alfvèn waves are present in the environment of HSSs and CIRs (McPherron, 2005; Richarson, 2018).
Alfvèn waves often reach the Earth’s magnetosphere in a quasi-periodic southward direction, which is a condition for triggering magnetospheric storms (Dungey, 1961). HSSs and CIRs, which guide the Alfvèn waves, cause weak or moderate, but long lasting (several weeks to some months) geomagnetic storms, which are characterized by almost continuous UV Auroras often covering the entire dayside and nightside auroral zones (Tsurutani and Gongalez, 1987). Under such space conditions, ionospheric electric fields and thermospheric winds from the high latitudes can affect the middle and the low latitude ionosphere (Abdu et al., 2006). At these times, magnetospheric and ionospheric EM variations produce electromagnetic effects on the ground (Marhavilas, 2007).
Since solar wind sub-ULF Alfvèn waves are transformed into sub-ULF electromagnetic waves reaching Earth’s surface, they could probably mediate space weather with seismic activity. Furthermore, HSSs themselves are highly related to sub-ULF electromagnetic waves observed at Earth (Kim et al., 2002; McPherron, 2005).
Geomagnetic Ultra Low Frequency Waves Before the Taiwan Chi-Chi 1999 Earthquake
The first reason why we choose the Taiwan 1999 earthquake as a case study is the fact of CIRinduced extreme geomagnetic activity in late 1999 (Borovsky and Denton, 2006). A second reason is that sub-ULF waves were observed in Taiwan before the Chi-Chi 1999 EQ and they have been discussed in the scientific literature extensively. Geomagnetic sub-ULF fluctuations recorded by several stations in Taiwan before the 1999 Chi-Chi EQ have been reported and discussed among others by (Lin. 2013), Liu et al. (2000, 2004), Ohta et al. (2001), Akinaga et al. (2001); (Shin, 2004), Yen et al. (2004), Freund and Pilorz (2012), Tsai et al. (2018).
Yen et al. (2004) hypothesized that the geomagnetic sub-ULF fluctuations were connected to the process of accumulation and releases of crustal stress, and the subsequent severe surface ruptures. They noted that the mechanisms producing the geomagnetic fluctuations are not clearly understood.
Freund and Pilorz (2012), Tsai et al. (2018) suggested that the sub-ULF geomagnetic fluctuations before the Chi-Chi EQ, were the result of a large current generated by accumulation and release of crustal stress.
Pulinets and Boyarchuk (2004) noted that the near Chi-Chi earthquake magnetic anomalies were probably connected with effectiveness of solar events, while Anagnostopoulos et al. (2021) reported that HSSs/CIRs transferred Alfvèn waves in the magnetosphere before the Chi-Chi 1999 EQ.
Given that there exist several reports on the existence of ULF EM waves before the Chi-Chi earthquake, in this study we investigate the hypothesis that the ULF geomagnetic fluctuations in certain frequency bands may be originated from solar wind. To this end we reasonably address the question whether sub-ULF geomagnetic anomalies are not the result of the microseismicity observed for ∼7 seven weeks before the Chi-Chi earthquake, but an agent of micro-earthquakes.
Geomagnetic Ultra Low Frequency Waves of Space Origin
ULF waves of space origin are observed on Earth, in particular at low frequencies around 1 mHz (Barnes, 1983). Some of these waves originate at the Sun and are both carried by, and propagate through the solar wind.
The location of the regions on the Earth where ULF waves are observed depends on the solar wind dynamic pressure, the interplanetary magnetic field, and the conductivity of the Earth underneath the observer (Kivelson and Russell, 1995; McPherron, 2005). Changes in orientation of the interplanetary magnetic field (IMF) or Alfvén waves in HSSs/CIRs have dramatic effects on the type of waves seen on the Earth.
Besides the ULF waves transferred by the solar wind, waves are also locally produced upstream from the Earth’s bow shock, at the bow shock and the magnetopause and they are sources of ULF waves penetrating into the magnetosphere (McPherron, 2005). Another type of ULF waves is produced by oscillatory dynamic pressure fluctuations in the solar wind (Kepko et al., 2002).
Μany observations obtained simultaneously by satellites in space and on the ground have shown that solar wind perturbations deeply penetrate into the magnetosphere. Furthermore, it was found that the lower-latitude data on the nightside are important in monitoring the external source variations (Kim et al., 2002; Villante and Tiberi, 2016). Τhe ULF signal seen at the ground is an electromagnetic wave radiating from electric currents induced in the ionosphere (and not the magnetohydromagnetic waves themselves).
Russell et al. (1992) reported that the best correlation between ground level changes and the change in the solar wind dynamic pressure occurs at geomagnetic latitudes from 150 to 300.
Sarafopoulos (2005) demonstrated that sometimes monochromatic or quasi-periodic geomagnetic pulsations were exo-magnetospherically excited by a wave-source with a periodicity from ∼3 to ∼10 min and that the magnetosphere in these cases can respond to solar wind ULF waves in such small time scales as 2.7 min.
In the next section we compare space and terrestrial observations in order to identify the origin of the sub-ULF (<1 mHz) magnetic field waves observed before the catastrophic Taiwan 1999 earthquake.
Results
In this section we compare space and terrestrial observations in order to test two hypotheses on the origin of the sub-ULF magnetic field waves observed before the occurrence of the Chi-Chi earthquake (21/20 September 1999), that is a space versus a geotectonic model of the magnetic field wave generation. To this end, we compare observations from the Advanced Composition Explorer (ACE) spacecraft (http://www.srl.caltech.edu/ACE/ace_mission.html), which is in orbit around the L1 Sun-Earth libration point, magnetic field observations in Taiwan, along with geomagnetic indexes (http://wdc.kugi.kyoto-u.ac.jp/ae_provisional/199809/index_19980913.html)
The ACE spacecraft was launched in 1997 and thereafter has been remained in a halo orbit about the L1 point.
The ACE instruments used in this study are the Electron, Proton, and Alpha Monitor (EPAM) (Gold et al., 1998), the Magnetometer Instrument (MAG) (Smith et al., 1998), and the Solar Wind Electron, Proton, and Alpha Monitor (SWEPAM) (McComas et al., 1998).
EPAM was constructed to investigate energetic ions and electrons, and it is composed of five telescope apertures of three different types; the Composition Aperture (CA), two low energy magnetically shielded telescopes (LEMS30 and LEMS120) and two low energy foil shielded telescopes (LEFS60 and LEFS150). The telescopes use the spin of the spacecraft to sweep the full sky. Solid-state detectors are used to measure the energy and composition of the incoming particles. ACE/EPAM data consist of electrons and ions in the energy range from ∼50 keV to 5 MeV (Gold et al., 1998; Marvavilas et al., 2015).
The NASA Advanced Composition Explorer (ACE) ACE spacecraft is used, in a routine basis, in investigating space weather (https://www.swpc.noaa.gov/products/ace-real-time-solar-wind). The ACE satellite enables United States National Oceanic and Atmospheric Administration (NOAA) Space Weather Prediction Center (SWPC) to give advance warning of geomagnetic storms and recognize their types.
Test 1. A Space Origin of Magnetic Activity Before the Taiwan 1999 Earthquake Is Not Impossible
Geomagnetic Conditions Before the Chi-Chi Earthquake
The first question we examine relating to the origin of the sub-ULF (<1 mHz) magnetic field activity observed before the Taiwan 1999 earthquake (Akinaga et al., 2001; Yen et al., 2004; Freund and Pilorz, 2012) is whether there exists direct observational evidence that the sub-ULF magnetic field waves were produced by some tectonic process in the Earth’s lithosphere.
Figure 1 shows the total geomagnetic intensity at the station LP, between mid-August and November 1999 (adapted from Yen et al., 2004). Yen et al. (2004) noted that a magnetic storm was observed ∼2 days after the Chi-Chi earthquake and another one about 1 month later, at almost the time of occurrence of the Chia-Yi earthquake. These authors claimed that no other space-induced magnetic anomaly was observed except for these two storms and they concluded that the total geomagnetic intensity at the reference station LP did not show a disturbance at the times of the ChiChi earthquake and the Chia-Yi earthquake or times previous to the two EQs. However, this argumentation presupposes the unproven hypothesis that, beyond a terrestrial source, a space agent of the magnetic field wave activity could be a CME-induced short-lasting strong geomagnetic storm. Based on the absence of such a storm before the Chi-Chi and Chia-Yi EQs, a terrestrial origin is inferred.
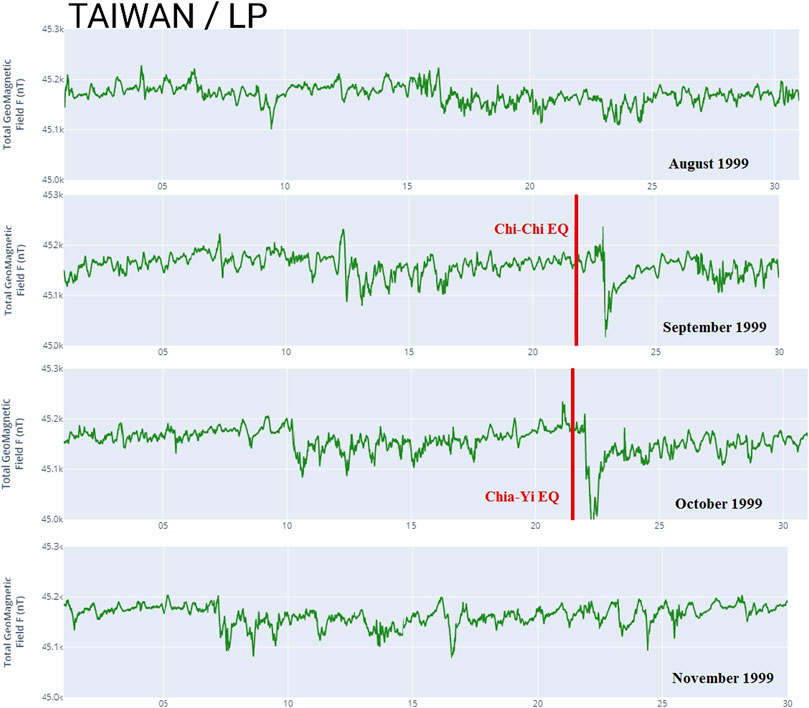
FIGURE 1. Geomagnetic total intensity data recorded at the reference station (LP) from August to November 1999 adapted from Yen et al. (2004).
The above argumentation follows the generally accepted hypothesis that only a short time great magnetic field variation might be an alternative physical process related with a future earthquake. However, it is well known from space science literature that there are two main types of geomagnetic storms (Borovsky and Denton, 2006), which are followed by a similar magnetic field pattern on the ground: 1) a short time great magnetic field variation and 2) a weak or moderate long lasting but long lasting magnetic field depression.
Actually, in Figure 1 we identify both types of imprints of geomagnetic storms. The two intense short lasting events (green arrows), which are obviously induced by Coronal Mass Ejections (CMEs) and three quasi-periodic (orange horizontal lines in Figure 1) long lasting moderate magnetic field depressions, which originate from a ∼27-days recurrent HSS/CIR. Since, it is generally accepted that “in order to detect changes in the geomagnetic field associated with tectonic stress, it is necessary to eliminate the changes originating from external sources in the observed data”, a space influence cannot be eliminated on the magnetic field data shown in Figure 1 before both the Chi-Chi and Chia-Yi earthquakes.
In the next subsection we describe in some more detail the basic features of the two types of geomagnetic storms, since this information is crucial for the present study as well as the general research of the pre-EQ electromagnetic phenomena.
Coronal Mass Ejections and ∼27-days Recurrent High Speed Solar Wind Streams Triggering Geomagnetic Storms
The most dramatic effect of the Sun in the interplanetary space is of course the Coronal Mass Ejections (CME). CMEs are large explosions of plasma and magnetic field from the Sun’s corona, which are released into the solar wind and they often follow solar flares (Tsurutani et al., 2020). CMEs most often occur during solar maxima. On the other hand, the coronal holes are the least active regions of the Sun. They have magnetic fields opening freely into the heliosphere and they are associated with rapidly expanding open magnetic fields and the acceleration of the high-speed solar wind. They are the source of HSSs and CIRs, which are most often observed during the decay and the rising phase of the solar cycle (Richardson, 2018; Badruddin et al., 2018). During the year 1999 the solar activity was in the rising phase of solar cycle and in late 1999 (including July and August) ∼27-days recurrent CIRs were noted as triggering long lasting storms (Borovsky and Denton, 2006).
However, despite the fact that dramatic solar flares and large CMEs are known to be associated with strong geomagnetic storms (Patsourakos et al., 2016 and references therein), the importance of the CH-driven HSSs in triggering geomagnetic storms is also significant (AGU collection of papers in Geophysical Monograph #167 edited by Alfvén, 1950; Kivelson and Russell, 1995; Tsurutani et al., 2006; Abdu et al., 2006; Kavanagh and Denton, 2007; Cranmer, 2009; Richardson and Cane, 2011; Tsurutani et al., 2011; Richardson and Cane, 2012a; Richardson and Cane, 2012b; Gerontidou et al., 2018; Richardson, 2018; Watari, 2018, and references therein).
HSSs dominate in the declining phase of the solar cycle, and in general during times of low SSNs, when polar CHs extend towards lower solar latitudes. Therefore, the flow of plasma from the solar corona is non-uniform in both time and space (Phillips et al., 1995). The fast solar wind emanating from a large coronal hole catches up with upstream slow solar wind and a compressive region is formed at the interface of the two streams. These structures reappear with the ∼27-days rotation period of the Sun. When these CH-associated streams are long lasting, they lead to the formation of corotating interaction regions (CIRs) in the interplanetary space and when the CIRs are well developed, they are bound by fast forward (FS) and fast reverse (RS) shocks. It is interesting to note that HSSs and CIRs can drive important physical processes over longer periods than the more transient CMEs can (Tsurutani et al., 2006; Borovsky and Denton, 2006; Kavanagh and Denton, 2007).
The CME-driven storm is a strong, but short lived phenomenon, which usually lasts from a few hours to a few days (Tsurutani et al., 2006). The HSSs/CIRs cause weaker storms than the CMEs. However, the “weak” HSS-induced storms last for long times; the recovery phases of CIR-induced magnetic storms can last for a few up to 27 days. Furthermore, since during a quiet Sun, CH-driven HSSs corotate with the Sun’s 27-days period, one or more HSSs, can affect semi-continuously the Earth’s magnetosphere for several months. The important point to note is that during such long time periods, HSSs/CIRs can transfer much more energy in the magnetosphere than a single CME. During HSS-induced storms, the Dst value normally ranges between −25 nT and −75 nT and typically does not reach intensities of −100 nT.
Long-duration high-speed-stream-driven geomagnetic storms also lead to the strongest electron-radiation-belt radiation hazards, to the strongest plasma sheet spacecraft-charging hazards, and to the related spacecraft anomalies (Wrenn et al., 2002; Wrenn, 2009).
The semi-continuous arrival of HSS structures cause almost continuous UV Auroras, which often cover the entire dayside and nightside auroral zones for days to many weeks (Tsurutani and Gongalez, 1987). The Aurora phenomenon is known as High Intensity Long Duration Continuous Auroral Electrojet Activity (HILDCAAs).
Periodicities of ∼14/27–28 days in Taiwan Geomagnetic Data in Late 1999
Imprints of the two types of storms, CIR-induced storm and CME-induced storm, on the geomagnetic field observed in Taiwan are evident in Figure 1, as we mentioned above. In particular, from Figure 1 we see the imprints of a long-lasting storm between days 12–20.9.1999 before the ChiChi (September 20, 1999) EQ, and a short-lasting CME-induced storm after the EQ, on days 2223.9.1999. We also see a similar pattern in the magnetic field data before the Chia-Yi EQ.
The geomagnetic field data in Taiwan, which show a moderate, but long lasting magnetic field decrease with sub-ULF wave activity, between days 12–20 in September 1999 and ∼27-days recurrent similar magnetic field patterns in October and November (Figure 1), during a period of ∼27 days recurrent storms (Borovsky and Denton, 2006), suggest that we cannot reject the hypothesis that an external (space) agent might be the source of the sub-ULF waves between days 12–20.9.1999. On the contrary, since HSSs/CIRs trigger long-lasting weak to moderate magnetic storms, which produce sub-ULF EM waves at Earth, we infer that the presence of sub-ULF waves before the Chi-Chi EQ is consistent with a space source.
Since we cannot eliminate an external origin for the sub-ULF magnetic field waves before the Chi-Chi and Chia-Yi earthquakes, the question is: is there any direct observational evidence that they were the result of geotectonic processes? Freund and Pilorz (2012) compared the sub-ULF (<1 mHz) magnetic field waves with seismological data before the Chi-Chi earthquake, in order to directly test such a possibility. To this end, they compared the intensity of the ULF fluctuations by using LY/HL observatory 12-h running average data with the excess seismic energy output from August 1 to September 21,1999 (their Figure 19).
Their study suggested a dominant periodicity at ∼14 days in the ULF magnetic activity. The periodicity was attributed to strong influence from the Earth’s tides. However, if the ∼14 days ULF periodicity was due to some gravitational effects of the Sun or the moon, then a clear argumentation on the correlation of the ULF ∼14-days periodicity with the moon phase or the Sun-Earth relationship should be demonstrated. Instead, the authors noted that “Because the gravitational forces are relatively weak, the seemingly tight coupling between the Earth tides and both, ULF emission and regional seismicity, comes as a surprise». Therefore, we think that until a correlation between the ULF ∼14-days periodicity and direct gravitational features can be shown, the attribution of the ULF ∼14-days periodicity to gravitational forces cannot be accepted as a reliable explanation of the data.
A second argument by the same authors for a geotectonic source of the magnetic anomalies was based on the correlation between the curves of the ULF activity and the pre-EQ energy output excess between August 1-September 20, 1999. It was suggested that there exists a correlation and causal link between Earth tides, the frequency distribution of earthquakes, and the emission of ULF signals. However, in such a case, if the seismicity was the cause of the ULF fluctuations, the seismic energy release increase should have preceded the increase of the ULF activity.
However, the opposite pattern is obvious in Figure 19 of the paper by Freund and Pilorz. The pre-EQ excess seismic energy output curve E is shifted relative to the ULF line in such a way that both the onset and the maximum of the ULF activity reach earlier than the excess seismic energy E in all the four long lasting events analyzed (except for the onset of the last event, where the increases of the two parameters occurred almost simultaneously). Thus we cannot reject the hypothesis that space weather triggered the ULF EM waves at the LY station/Taiwan (red). Moreover, the time delay between ULF activity and seismic energy E are consistent with an external source of the ULF activity.
Test 2. The Taiwan Magnetic Activity Is Correlated to Geomagnetic Storm Activity
Here we check the hypothesis that the anomalous magnetic field activity in Taiwan and the geomagnetic storm may have a common space agent. For this reason we investigate the possible relation of the Taiwan magnetic field decrease associated with the sub-ULF magnetic activity between 12 and 21 September 1999 with the Earth’s geomagnetic storm activity as provided by the global Dst index (Borovsky and Shprits, 2017), [http://wdc.kugi.kyotou.ac.jp/dst_final/199909/index.html].
For this reason, In Figure 2 we compare the local B-field measurements by the LP/Taiwan observatory (Panel A) with the global geomagnetic index Dst (Borovsky and Shprits, 2017; http://wdc.kugi.kyoto-u.ac.jp/dst_final/199909/index.html) (panel B) during the whole of September.
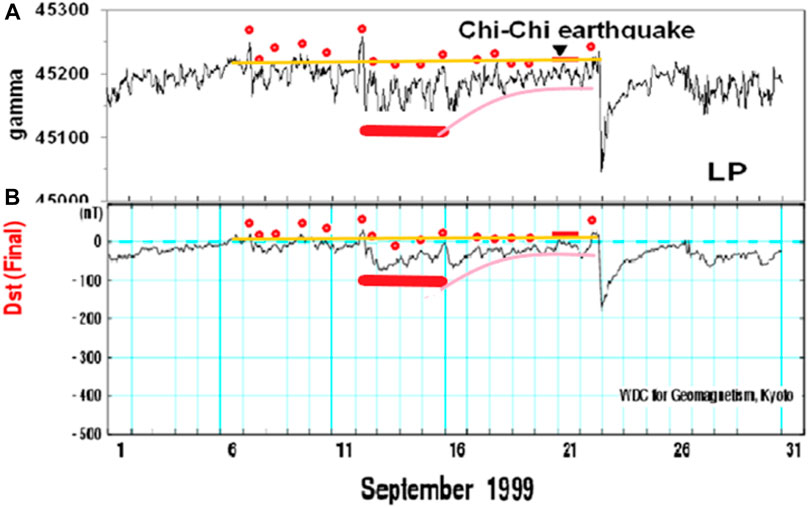
FIGURE 2. Local magnetic field B at LP observatory, Taiwan (top panel) along with the Global Dst index of the geomagnetic storm activity in September 1999, including the time of the catastrophic Chi-Chi, 1999 earthquake. It is obvious that the two profiles are similar (A, B) during almost all September, and enhanced fluctuation occurs almost simultaneously in (B) and Dst values, during a general decrease in both profiles, before the time of the Chi-Chi earthquake (black arrow in (A). The pre-earthquake magnetic profile in Taiwan obviously reflects the geomagnetic storm activity as indicated by the index Dst (see in the text).
The Dst index shows the strength of the Earth’s ring current, which flows toroidally around the Earth, centered at the equatorial plane and at altitudes of-10,000–60,000 km. Changes in the ring current are responsible for global decreases in the Earth’s surface magnetic field and reveals the occurrence of a geomagnetic storm (Daglis et al., 1999).
The Dst index also reacts to the partial ring current (Liemohn et al., 2001), the cross-tail current (Ohtani et al., 2001), and the Chapman-Ferraro dayside-magnetopause current (Burton et al., 1975) and is primarily a measure of plasma pressure in the bipolar magnetosphere (Dessler and Parker, 1959; Greenspan and Hamilton, 2000). Dst is also often used to define the duration of a storm, and to distinguish between quiet and disturbed geomagnetic conditions (Borovsky and Shprits, 2017). A Dst categorization that is often used to categorize storm intensity is (I) a weak storm (−30 nT > Dst > −50 nT), (II) a moderate storm (−50 nT > Dst > −100 nT), (III) a strong storm (−100 nT > Dst > −200 nT), (IV) a severe storm has (−200 nT > Dst > −350 nT), and a (V) great storm (Dst < 350 nT) (Loewe and Prolss, 1997). Dst is derived from a network of geomagnetic field observatories around the globe and its changes occur every time when Bz component of IMF B turns negative. The energy transfer is closely related to the Bz component of the IMF through a large-scale reconnection process, on the dayside, when a negative IMF meets the northward magnetic field of Earth’s and a fraction of the available solar wind energy is able to penetrate our magnetosphere (Dungey, 1961).
Figure 2B reveals two different patterns of geomagnetic storms (Borovsky and Denton, 2006): 1) a long lasting CIR-induced Type II (moderate) storm, between 12 and 20.9.1999 and 2) a short time Type III CME-induced storm, on days 22–24.9.1999.
A comparison of Panels a and b suggests that the values in both panels follow a similar pattern during the whole of September 1999: 1) an increase between days 1–6, 2) a disturbance between days 7–11, 3) an abrupt decrease on day 12, 4) a general decrease along with a fluctuating profile between d. 12–16, 5) a recovery between d. 16–20, 6) an intense decrease on day 22, 7) a recovery phase until d. 26, and 8) a general small decrease between days 27–31. The impressive similarity of the local magnetic field in Taiwan with the index Dst, which reflects the global variation of magnetic field suggests that: 9) the magnetic field profile in Taiwan reflects not local physical processes but global variations in the Earth’s magnetic field, and (b) the long lasting weak magnetic field decrease (days 12–20.9.1999) and the abrupt intense decrease (22–25.9.1999) in Taiwan reveals a CIR-induced storm and a CME-induced storm, respectively. In particular, we infer that the striking similarity of the profiles of two profiles strongly suggests a dependence of the local magnetic field before the Chi-Chi 1999 earthquake (days 12–20.9.1999) on space physical processes.
In order to further check the nature of the magnetic field variations in September 1999, we also check the presence of geomagnetic storm activity at high latitudes by examining the auroral electrojet indeces AU, Al, AE and AO. The Auroral Electrojet indexes monitor the magnetic signature of the eastward and westward auroral electrojets in the Northern hemisphere and they have been usefully employed both qualitatively and quantitatively as a correlative index in studies of substorm morphology. To calculate the AE index, magnetograms of the H components of 12 observatories have been uniformly distributed over the longitude in the northern hemisphere at auroral or subauroral latitudes between 60° and 70° (Shadrina, 2017).
In Figure 3 the AU, Al, AE and AO indexes are shown on a day near the beginning of the long lasting magnetic field disturbance in Taiwan (13.9.1999), and on a day at the recovery phase of the field. It is evident that the auroral electrojet indexes AU, Al, AE and AO show a strong storm activity on day 13 (http://wdc.kugi.kyoto-u.ac.jp/ae_provisional/199809/index_19980913.html and a weak activity on day 20.9.1999 (http://wdc.kugi.kyoto-u.ac.jp/ae_provisional/199809/index_19980910.html), in agreement with the Dst variations seen in Figure 2B. We infer that the local magnetic variations in Taiwan between days 12–20.9.1999 reflect the progress of a geomagnetic storm recorded at both the low and the high latitudes. The comparison of Figure 3B to Figure 2B and Figure 3 confirm a space and not a local (terrestrial) physical process being the cause of the Taiwan magnetic field variations (including sub-ULF activity) for about 10 days before Chi-Chi EQ.
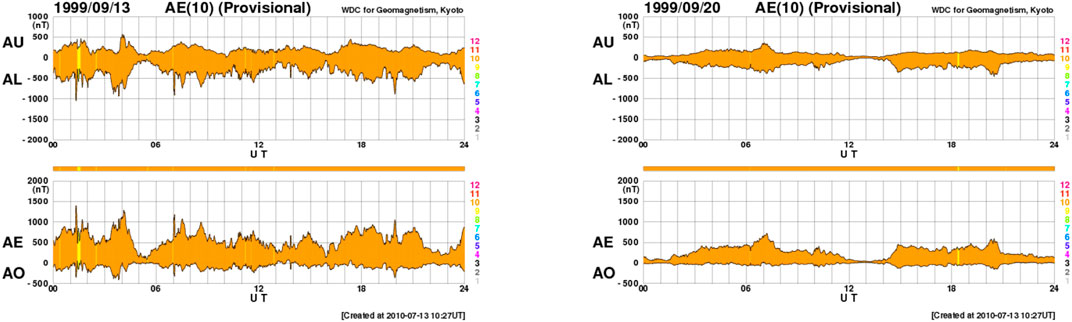
FIGURE 3. Auroral Electrojet indexes on 13.9.1999, the day after the storm onset and during a day at its recovering phase of the long lasting storm occurred before the Chi-Chi earthquake.
Test 3. The Magnetic Variations in Taiwan Is Controlled by Space Weather Conditions
We mentioned earlier that the flow of plasma from the solar corona is non-uniform in both time and space (Phillips et al., 1995), and a compressive region is often formed at the interface of the fast stream with the upstream slow stream. When these structures last for long times they form corotating interaction regions (CIRs) in the interplanetary space and when the CIRs are well developed, they are bound by a fast forward (FS) and fast reverse shock (RS).
The most typical variations of plasma parameters of a CIR are: 1) an increase in the solar wind plasma density N and the interplanetary magnetic field B, due to the ‘pile-up’ of material at the leading edge of the fast wind, 2) a long lasting high speed solar wind and 3) the presence of large amplitude low frequency (Alfvén) waves, in particular within the CIR, between the FS and the RS. When the CIR incident on the Earth’s magnetosphere trigger long lasting weak or moderate magnetic storms (Tsurutani et al., 2006; Borovsky and Denton, 2006; Kavanagh and Denton, 2007; Richarson, 2018) and various other important phenomena in the Earth’s environment. We investigate now the possible incident of a CIR as possible agent of the long duration time moderate geomagnetic storm (Figure 2B and Figure 3) and the subsequent magnetic field imprint in Taiwan (Figure 1), between September 1220, 1999.
In order to apply Test 3, that is the possible relation of the geomagnetic variations between 1220.9.1999 with the arrival of a CIR, we analyze space observations from the Advanced Composition Explorer (ACE) spacecraft, which is in orbit around the L1 Sun-Earth libration point, at a distance of ∼220 RE from Earth (RE: the Earth’s radius). The ACE is a satellite, which is in general used in space weather studies in order to predict or explain space induced variations in the environment of Earth, as, for instance, geomagnetic storms, radiation belt electron variation status etc. (Stone et al., 1998).
Figure 4 shows magnetic field data in spherical coordinates (B, θ φ) in the three top panels, A, B and C, from the Magnetometer Instrument (MAG) (Smith et al., 1998) and solar wind density, temperature and speed in panels D, E and F from the Solar Wind Electron, Proton, and Alpha Monitor (SWEPAM), for the time interval September 8–22, 1999.
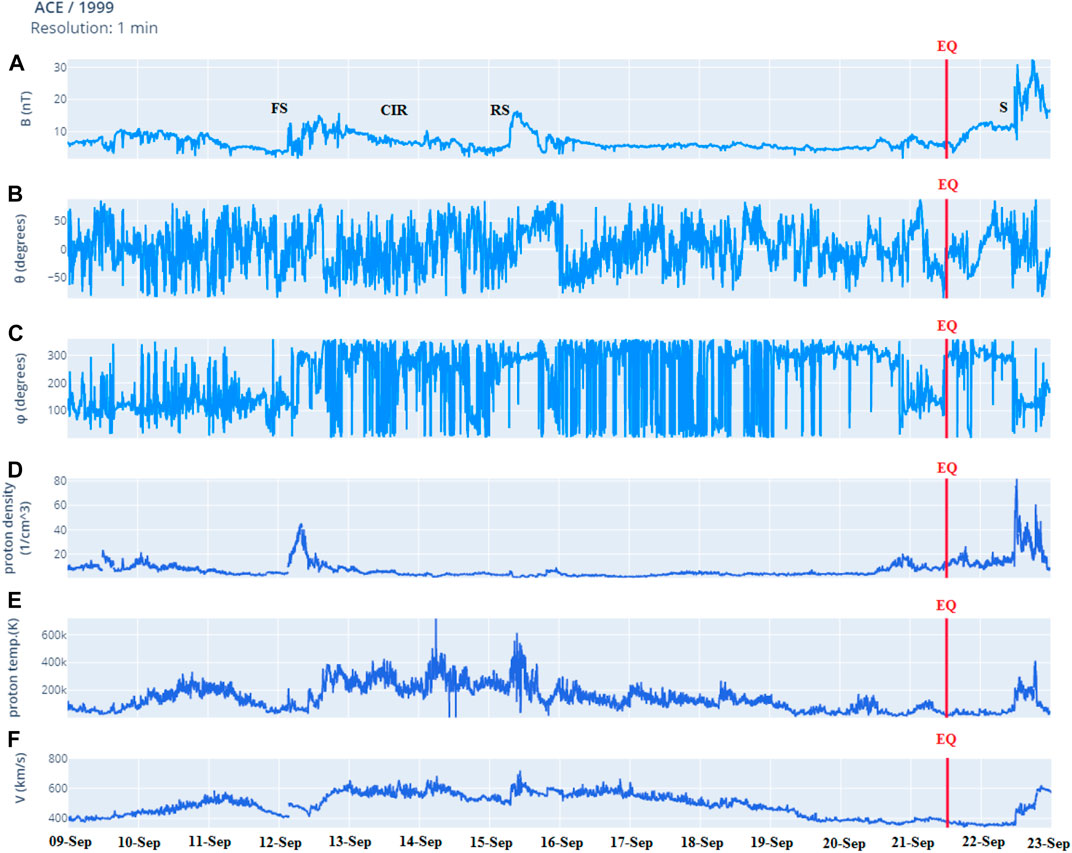
FIGURE 4. Magnetic field data (B, θ φ) in spherical coordinates three top panels; (A-C), Brms, and proton plasma density, temperature and speed three bottom panels; (D-F) as recorded by the ACE spacecraft, for the time interval September 9–22, 1999. The red normal line indicates the time of Taiwan earthquake occurrence, at the end of a corotating interaction region (CIR), which is accompanied by rich presence of ULF Alfvèn waves.
In this figure we can see characteristic features of a CIR structure bounded by a MHD FS on day 12 of September and a RS on day 15.9.1999. For instance, we can notice an increase in magnetic field magnitude B (panel A), in solar wind speed Vp (panel F) in solar wind density Np (panel D), and proton density which indicate the arrival of the FS, on day 12th of September. Furthermore, we see a long lasting increase of the solar wind speed Vp, which starts with the arrival of the CIR, on September 12, 1999 and drops to pre-CIR values on September 20, 1999, along with intense northsouth sub-ULF magnetic field direction fluctuations (values; panel B).
By comparing Figures 1, 2, 4 we infer that both the long lasting geomagnetic storm and the magnetic field decrease in Taiwan started on day 12.9.1999 at almost the same time as the arrival of the CIR in the near Earth interplanetary space (ACE). Furthermore, the time interval of the most intense magnetic field fluctuations recorded in Taiwan coincides with the time interval of the CIR at ACE; the intense magnetic field fluctuations started on day, 12.9.1999, the day of FS and lasted until 15 September 1999, the day of RS (red bar in Figure 1A).
The ACE observations shown in Figure 4 obviously confirm the hypothesis that the longlasting geomagnetic variations between 12 and 20.9.1999 in Taiwan and in the magnetosphere are related with the arrival of a CIR, which evidently was the cause of the magnetic field variations (Kavanagh and Denton, 2007; Badruddin et al., 2018; Richardson, 2018) observed in Taiwan between 1220.9.1999. Test 3 definitely confirms that a CIR was the cause of the long lasting geomagnetic storm (Figures 2, 3) and of the related magnetic field anomalies in Taiwan (Figure 1) observed before the Chi-Chi earthquake.
Test 4. Magnetic Sub-Ultra Low Frequency Waves in Taiwan Are Related With Magnetohydrodynamic Wave Activity
Since we found strong observational evidence that the long lasting magnetic field decrease before the Chi-Chi EQ (12–20.9.1999) was a signature of the CIR incidence on the Earth’s magnetosphere, we now further focus on the generation process of the pre-EQ sub-ULF magnetic field waves themselves.
In the previous (sub)sections we provided significant evidence that not only the pre-EQ magnetic field decreases, but also its general profile, including the sub-ULF magnetic field wave activity, is consistent with an external (space) cause. Now we investigate the exact generating mechanism of the sub-ULF magnetic field fluctuations between 12 and 20.9.1999.
It has been found that solar wind speed, solar wind ULF waves, the negative component Bz of the IMF and solar wind density perturbations are correlated with ULF magnetic pulsations on the ground (Bentley et al., 2018; Hynönen et al., 2020). Although solar wind speed has been considered as having the largest effect on the ground ULF power compared to the other parameters (solar wind ULF waves, the negative IMF component Bz and solar wind density perturbations), we examine here the (possible) contribution of the solar wind ULF Alfvén waves to the ground sub-ULF fluctuations before the Taiwan 1999 EQ. For this reason we compare here, for the first time, simultaneously obtained measurements in space, by the ACE spacecraft, and on Earth, by magnetic field observatories in Taiwan.
We have already mentioned that the solar wind is a source of MHD sub-ULF Alfvén waves, particularly at frequencies around 1 mHz (Barnes, 1983). In particular a series of studies has also confirmed that Alfvén waves are a ubiquitous feature of CIRs (Smith et al., 1995; Richardson, 2018) and that they have important implications for coupling of the interplanetary space to the magnetosphere (Tsurutani et al., 2006). Two processes transform the ULF wave energy that enters the magnetosphere from outside: the field line resonance and the cavity resonance (Southwood and Hughes, 1983). The two processes interact with each other. Standing waves in the cavities feed energy to the field line resonances. Many of the pulsations seen at the ground are caused at the ends of field lines set into motion by a complex process involving coupling of the propagating waves to resonant cavities, and these, in turn coupling to field line resonances (McPherron, 2005). Furthermore, it has been confirmed that the 2–10 mHz EM power increases with an approximate power law dependence on solar wind speed, at all local times (Hynönen et al., 2020). Finally it worths noting that the space controlled signal seen at the ground is not the MHD waves themselves, but electromagnetic waves radiating from electric currents induced in the ionosphere by the external source (McPherron, 2005).
In Figure 5 we compare magnetic field data obtained by the ACE spacecraft upstream from the Earth’s bow shock (panels A and B) and by the LNP observatory in Taiwan (panels C and D) on day 13 September 1999, between 0 and 12UT. In particular, the two upper panels display the magnetic field magnitude (panel A) and the angle in polar coordinates (panel B), as measured by the magnetometer instrument MAG onboard ACE. The two bottom panels show the magnetic field component Bz (panel C) as well as the magnitude of the total magnetic field, as measured at LNP observatory in Taiwan.
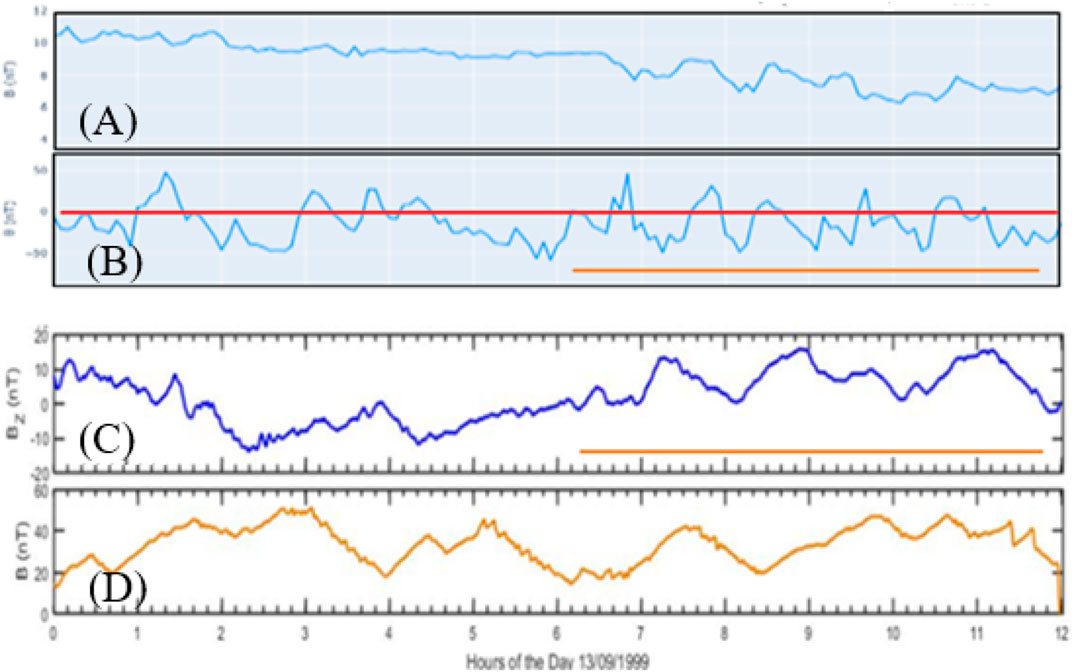
FIGURE 5. The sub-ULF B wave activity in Taiwan (C) changes after ∼7 UT following a change in the MHD Alfvén wave pattern at ACE satellite, in the interplanetary space (A, B).
We have seen that day 13 was characterized by intense geomagnetic storm activity (Figure 3), which was caused by the arrival of a CIR on day 12.9.1999 (Figures 3, 4). Figure 5 suggests that day 13, 0-12UT, was also a period of large amplitude magnetic field fluctuation at the position of the ACE spacecraft, far upstream from the Earth’s magnetosphere. From Figure 5 we also see that the magnetic field gradually decreases and it shows a variable magnetic field direction during the whole 12-h period examined.
Panel b suggests that the magnetic field turns quasi-periodically in the southward direction. The southward IMF turning is the main condition that triggers geomagnetic storms (Dungey, 1961) and, indeed, we see that the storm activity is high at the same time period (Figures 2, 3). The type of the waves are MHD Alfvén waves, as inferred from the almost stable magnetic field magnitude and the large variations of the azimuthal angle (Chen, 2016), which sometimes changes from ∼−500 to ∼+500 (panel b).
From the comparison of the curves in panels a, b, c, and d, we infer that the magnetic field at the LNO/Taiwan observatory also fluctuated. In particular, we see that the wavy pattern changes after ∼6 UT both in the interplanetary space (ACE) and on the ground (LNO/Taiwan) in the first and the second part of the time period examined. After ∼6 UT (horizontal orange lines in Figure 4) both the IMF and the terrestrial magnetic field become much more turbulent than between 0 and 6 UT; this evident when we compare the latitudinal magnetic field angle at ACE (panel B) and the northsouth magnetic field variation in Taiwan (panel C).
There is another interesting point to note in Figure 5. The increased magnetic field fluctuation at Earth after ∼6 UT is preceded by successive southward turning of IMF, which is a cause of magnetic storms and a more turbulent magnetic field on Earth. Furthermore, some variations in the IMF magnitude, after ∼07:00 UT, might contribute to geomagnetic triggering due to changes in the electromagnetic pressure on the Earth’s magnetosphere (Takahashi et al., 2012). The magnetic field fluctuation variation at both sites, in the interplanetary space and in Taiwan, shows a period of ∼1 h, that is a frequency <1 mHz (with somewhat longer period waves at Earth than at ACE).
We infer that the wave patterns in Taiwan and at ACE seen in Figure 5 show remarkable relations. Since a variety of physical factors influence the propagation of an electromagnetic signal from the position of ACE, in the interplanetary space, to the Earth’s surface, the above noted relations are remarkable and suggest that the sub-ULF activity in the solar wind made a significant contribution on the ground magnetic field waves (Fraser, 2009; Shi et al., 2020)
Test 5. The Geomagnetic Activity in Taiwan Is Correlated With a ∼28-days Corotating Interaction Region of Solar Origin
Tests 1 to 4 support the concept that the sub-ULF magnetic field wave activity observed in Taiwan before the Chi-Chi EQ (12–20.1999) is consistent with a CIR-induced long lasting geomagnetic disturbance.
We now perform one more test concerning the space origin of the sub-ULF magnetic fluctuation before the M7.2 Chi-Chi EQ. Since 1) CIRs are a provoking agent of great EQs (Anagnostopoulos et al., 2021 and references therein), 2) CIRs-induced storms appear a characteristic ∼27-days periodicity in the late 1999 (Borovsky and Denton, 2006) and 3) the CIRinduced storms are characterized by sub-ULF wave activity on the ground (McPherron, 2005; Shi et al., 2020), we examine the hypothesis that ULF wave activity in Taiwan follows the solar ∼27/14 days periodicity of the CIRs. A correlation of the ground pre-EQ sub-ULF activity with CIRs in the interplanetary space would be an additional, very strong evidence of their space origin. The data in the interplanetary space were obtained by the ACE satellite (Figure 6).
For this reason, in Figure 7, we compare physical processes in interplanetary space, in the magnetosphere and on Earth (Taiwan) during a period of 7 weeks (from August 13 to September 30, 1999). The near Chi-Chi, Taiwan (adapted from Freund and Pilorz, 2012) from August 13 to September 30, 1999.
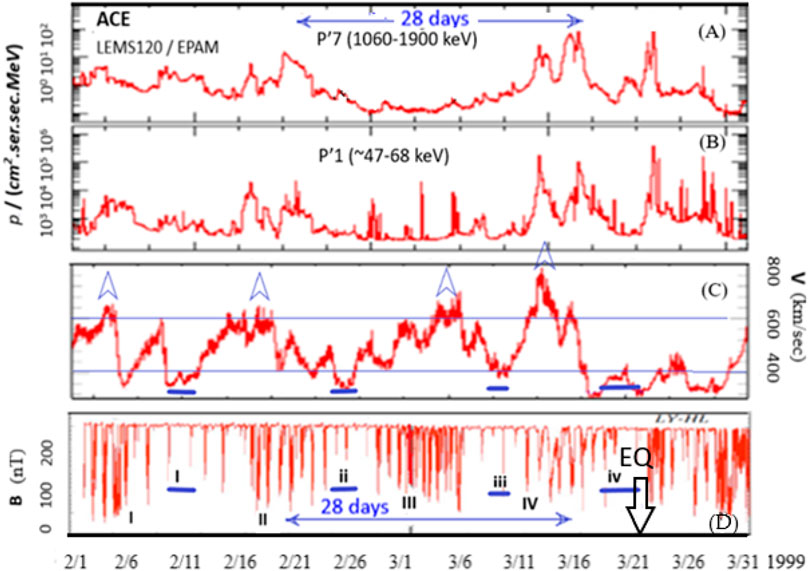
FIGURE 7. From top to bottom: proton flux of low (ACE/LEMS120/P’2) and high (ACE/LEMS120/P’7) energy protons in the interplanetary space from the solar direction (A, B), solar wind speed (A), and (D) the magnetic field fluctuations. Tests 5, 6 and 7 are based on the comparison of simultaneous measurements obtained by the ACE spacecraft in the interplanetary space and by the magnetic field observatories in Taiwan for time long enough to cover at least two successive, about 28 days separated CIRs.
In particular, in Figure 7, we display, from top to bottom, 1) interplanetary space low and high energy proton flux measurements from the EPAM instrument onboard the ACE spacecraft (panels A and B) 2) the space plasma proton speed measured by the Solar Wind Electron, Proton, and Alpha Monitor (SWEPAM) onboard the same spacecraft, (panel C), and 3) the 12-h running average difference of magnetic field values measured at the LY station minus those at the HL station in Taiwan. (adapted from Figures 15A,B of Freund and Pilorz (2012). The 12-h running average difference of magnetic field values were used to emphasize the overall envelope. The energetic proton fluxes from channels P’1 (∼47-68 kev), and P’7 (1060-1900 keV) were selected because of the general Sunward direction of the LEMS120/EPAM telescope (http://sdwww.jhuapl.edu/ACE/EPAM/idf.html), which allows investigation for particle streaming from the Sun.
When focusing on the time period before the Chi-Chi EQ (indicated by an arrow in panel E) we see a structure indicating an increased wave activity between days 12–19.9.1999 (ULF wave group marked IV), which correspond to the geomagnetic storm and the wave activity in Taiwan examined in the previous subsections (Figures 1–5; Tests 1–5). During this period the solar wind speed V (panel C) reached its highest values V = ∼800 km/s that is ∼2 times the normal value of solar wind speed.
Figure 7 also shows that, during days 12–20.9.1999, the enhanced solar wind speed values and the ULF wave group IV are also associated with enhanced proton fluxes showing two distinct peaks (panels A and B). These two flux peaks are related to the forward shock (FS) and the reverse shock (RS) of the CIR examined in Figure 4, and they reveal proton accelerating at the two edges of the CIR shocks (FS and RS). The coincidence of 1) the energetic particle flux enhancements (panels A and b), 2) the high solar wind speed structure (panel c), 3) the magnetic field profiles revealing the presence of a CIR (Figure 3) and (iv) the ULF wave group IV in Taiwan is in agreement with the CIR as a cause of the terrestrial magnetic field fluctuation at those times (Fraser, 2009; Richardson, 2018).
We examine now the hypothesis that the 27-days period corotating high solar wind speed streams/CIRs in late 1999 (Borovsky and Denton, 2006) may be related with the ULF wave activity in Taiwan. The observations in Figure 6 (panels A–E) clearly show that the ULF wave group II preceded the ULF group IV by ∼28 days, that is a time almost equal to the solar rotation period. The data in Figure 7 also suggests the presence of: 1) a high speed solar wind stream (panel F) and 2) two peaks in energetic proton flux (panels a and b) associated with a FS and RS (data not shown here), during the ULF wave group II.
The time difference between ULF magnetic field wave groups II and IV and the appearance of a CIR structure at the times of the two wave groups II and IV suggest that the ULF wave groups II and group IV are related with the same CIR.
It is obvious that the two CIRs, which are associated in time with high solar wind speeds, strong magnetic storm activity and the ULF wave groups II and IV separated by a period of ∼28 days, before the Chi-Chi EQ, strongly suggest that the two ULF magnetic field wave groups II and IV observed in Taiwan were caused by the incidence of a CIR on the Earth’s magnetosphere (Kivelson and Russell, 1995; Fraser, 2009; Richardson, 2018).
TEST 6. Solar Wind Speed and Taiwan Sub-ULF Magnetic Field Fluctuation
The sub-ULF magnetic field fluctuation (i.e. Pc 5 pulsations, in the frequency band 2–7 mHz; Jacobs et al., 1964) correlate well with solar wind speed (Engebretson et al., 1998; Kessel, 2008, Pahud et al., 2009; Hynönen et al., 2020). However, solar wind speed has been considered to have the largest effect on the ground ULF power compared to the solar wind ULF waves, the negative component Bz of the IMF and the presence of solar wind density perturbations (Bentley et al., 2018; Hynönen et al., 2020).
Based on the above space weather-terrestrial relations, we apply an additional test for the origin of the Taiwan sub-ULF wave activity before the Chi-Chi EQ (TEST 6). Since it is known that the sub-ULF wave activity is a normal result on Earth related with high solar wind speeds, we elaborate on the whole period examined in Figure 6, the possible relation of the changes of the sub-ULF wave activity before the Chi-Chi EQ with changes in the solar wind speed as observed by ACE in the interplanetary space.
In order to do that we name the four groups of the sub-ULF magnetic field fluctuation by numbering I, II, III, IV and the periods with absence or weak magnetic field activity by i, ii, iii, iv. The quiet periods i, ii, iii, iv, at times when the solar wind speed falls below the usual limit of 400 km/s, for more than 1 day, are marked by horizontal bars (panels D and E) to facilitate the solar wind speeds-Taiwan ULF EM waves comparison.
Such a comparison reveals that (A) during the four groups I, I, III and IV the solar wind speed V reaches maximum values between 600 and ∼800 km/sec (blue horizontal line) and (B) during the four periods of weak or absence of magnetic field wave activity (intervals called i, ii, iii and iv), the solar wind speed reaches its lowest values, below the usual level of 400 km/s (blue bars in panels D and E).
This correlation between the solar wind speed values and the presence/absence of the magnetic fluctuation in Taiwan, along with the previous Test 5, confirms that an external agent controls the sub-ULF magnetic fluctuations in Taiwan during 7 months before the Chi-Chi 1999 earthquake. This finding is consistent with recent statistical results (Gulyaeva, 2014; Rekapali, 2014; Anagnostopoulos et al., 2021)
Furthermore, it worths noting that the analysis of polarization (the ratio of vertical magnetic field component Z to the horizontal component G), that the polarization (Z/G) showed a significant enhancement for 2 months before the Chi-Chi earthquake. Akinaga et al. (2001) pointed out that this temporal evolution of polarization seems be associated with the Chi-Chi earthquake. These authors accepted as the generation mechanism of the sub-ULF (∼0.01) Hz emissions the microfracturing model by Molchanov and Hayakawa (1995). However, the present study suggests that the temporal evolution of the polarization (Z/G) is related with the special space weather during the last 2 months before the Chi-Chi earthquake and most probably is the result of semi-continuous magnetic storm activity. However, this result should be further tested.
Test 7. Long Time ∼14-days Periodicities in Space and in Taiwan
It is generally known that the ∼27-days solar rotation controls many physical processes in the Earth’s environment. The existence of a sole intense high speed solar wind stream emanating from the Sun causes ∼27/28-days periodicities in various physical phenomena both in the near Earth environment and on the ground. The existence of more than one solar wind stream can produce additional periodicities. For instance a second high speed solar wind stream produces a ∼13/14-days periodicity in the interplanetary space, in the Earth space and on Earth. Intense CIRs are observed as periodic (∼27/28-days, ∼13/14-days) phenomena even beyond the Earth’s orbit, in the deep space (Burlaga, 1997; Anagnostopoulos et al., 2009).
The ∼27/∼13-days periodicity of high speed solar wind streams influence the Earth’s magnetosphere in many ways. Among other phenomena, a power spectrum analysis recently revealed a ∼27/13-days periodicity in the global seismic energy output, in particular during a quiet Sun (Anagnostopoulos et al., 2021).
The ∼14 days-periodicity in sub-ULF activity is also seen in Figure 6 in the ∼14 days time separation of the four groups I, II, III and IV. This periodicity was also clear in the results of a Fourier Transform performed by Freund and Pirolz and (2012).
Since the four sub-ULF magnetic field wave groups I, II, III and IV are related with the arrival of the presence of high speed solar wind speed, we infer that the ∼14 days-periodicity evaluated by Freund and Pirolz was of a clear space origin (and it is not due to the gravitational forces exerted by the moon and the Sun).
The space origin of the ∼14 days-periodicity is also consistent with the fact that (both the onset and the maximum of) the four ULF groups I, II, III and IV earthquake reach earlier than the seismic energy E in all the four long lasting events before the Chi-Chi 1999 EQ.
The results from Tests 1–7 in a variety of observations in the interplanetary space and on the ground strongly suggest that the sub-ULF magnetic fluctuations in Taiwan during 7 months before the Chi-Chi 1999 earthquake are related with physical processes resulting from the interaction of high speed solar wind streams.
Discussion
Great earthquakes as well as global seismic energy output have recently been confirmed as depending on solar activity and the subsequent space weather. Enhanced seismicity was found to be higher during times of a quiet Sun, when the sunspot number (SN) is low (but not in the deep minimum). Such solar conditions allow high solar wind speed streams to emanate from coronal holes and form structures rotating in the interplanetary space with the ∼27-days period of solar rotation. Low SSN conditions and ∼27-days corotating interaction regions (CIRs) are mostly observed in the decay phase throughout the ∼11-years solar cycle, but also during the rising solar cycle phase as well as during short time intervals (some months) in the solar maximum phase. During such periods with small SN a ∼27-days periodicity was revealed in seismic energy release at Earth (Anagnostopoulos et al., 2021). The seismic activity is in general anti-correlated to the SSN. One of the results of our previous paper is the finding that all of the 16 giant (M ≥ 8.5) EQs between 1900 and 2017 occurred during the decay and the rising phase of the solar cycle or at times of a strong SN reduction.
The question raised from the above Solar-terrestrial relationship is which physical mechanism mediates space weather (CIRs) with the lithosphere in order to provoke great earthquakes or high global seismic energy release.
In the present case study we tested the hypothesis whether the sub-ULF (<1 mHz) magnetic field activity before a great EQ may be of a space origin, and therefore, a possible final agent of the EQ itself. The sub-ULF (<1 mHz) magnetic field activity is a well known phenomenon in the space community resulting from CIR incidence on the Earth’s magnetosphere, while it has been also accepted as an earthquake precursor resulted from lithospheric processes, as we reported in Theoretical and Observational Framework.
As a case study we selected to investigate the great (7.7 Mw) Taiwan September 20, 1999 earthquake, which has been shown to be preceded by a long time period of sub-ULF (<1 mHz) magnetic field activity. To this end, we performed seven observational tests. Furthermore, for the first time in earthquake prediction scientific literature, we compared simultaneous space observations in the interplanetary space and magnetic field waves on the ground.
The enhanced sub-ULF (<1 mHz) magnetic field wave activity in Taiwan was previously attributed to tidal loading and unloading of the Earth’s crust, due to the gravitational forces exerted by the moon and the Sun. However, it was also noted that since the gravitational forces are relatively weak, the seemingly tight coupling between the Earth tides and both ULF emission and regional seismicity, comes as a surprise (Freund and Pirolz, 2012).
The concept of gravitational tidal triggering has been known for more than 110 years (Schuster, 1897; Emter, 1997). However, several studies have reported either no correlation between the Earth’s tide and earthquake occurrence (Heaton, 1982; Vidale et al., 1998; Kennedy et al., 2004) or small positive correlations (Tanaka et al., 2002; Cochran et al., 2004) under several restrictions, as for instance, for a particular geographic region (Tolstoy et al., 2002; Kasahara, 2002), earthquake magnitude range (Wilcock, 2001; Tanaka et al., 2002; Kasahara, 2002) and increasing tidal stress levels. Métivier et al. (2009) found that small magnitude (less than magnitude 4.0) EQs are more easily triggered by tides.
Our data analysis during the period of 7 weeks before the Taiwan 1999 earthquake provides significant information concerning the origin of ∼27/28 and ∼13-days ULF wave periodicity found by Freund and Pilorz (2012). Our analysis strongly suggests that the ∼14-days periodic detection of four groups of ULF wave activity in Taiwan was the result of four almost simultaneous events of high speed solar wind streams observed by ACE in the interplanetary space. More studies are needed to further check the origin of sub-ULF (<1 mHz) magnetic field waves before other great earthquakes.
However, preliminary examination of several great earthquakes suggests that the pre-EQ sub-ULF (<1 mHz) magnetic field wave activity seems to be in general CIR-induced geomagnetic phenomena.
The data examined in the present study provide strong evidence that four groups of enhanced sub-ULF (<1 mHz) magnetic field waves in Taiwan were related in time with four groups (Figure 6; groups I, II, III, IV) of high speed solar wind streams for 7 weeks before the great September 21, 1999 E. The relation of magnetic storm activity, its imprints on the ground and the associated subULF magnetic fluctuation in Taiwan with the high speed solar wind streams and sub-ULF Alfvèn waves in the interplanetary space is consistent with space weather as the agent of ULF magnetic terrestrial fluctuation (Bentley et al., 2018; Hynönen et al., 2020) observed for 7 weeks before the Chi-Chi EQ.
Our study includes a more detailed data analysis for the fourth group of enhanced sub-ULF magnetic field activity (noted I Figure 6 as IV), which was observed between days 12–20.9.1999. At almost the same time a strong CIR produced a significant reduction in magnetic field magnitude, which was recorded by observatories in Taiwan. The Chi-Chi EQ occurred at the end of the CIR, on day 20.9.1999, whereas another earthquake in southern Taiwan (Chia-Yi) occurred after a solar rotation, at the end of the next arrival of the CIR preceding the Chi-Chi EQ.
Enhanced solar wind speed, sub-ULF Alfvèn wave activity, magnetic pressure and southward IMF have been confirmed in space science to produce sub-ULF magnetic field activity at Earth (Fraser, 2009). In the time period we examined in this study, actually both enhanced solar wind speed (up to ∼800 km/s) and intense sub-ULF Alfvèn wave activity were observed by the spacecraft ACE, almost simultaneously with the enhanced magnetic field activity in Taiwan during the four groups. Some observational evidence on day 13.9.1999, 0-12UT, during the main phase of the CIR-induced storm, suggests that when the pattern of the IMF wave activity changed, the terrestrial magnetic field wave activity changed as well. This observation suggests a principal role of the IMF wave activity at least at that time (day 13.9.1999, 0-12UT).
Our present results from the study of the precursory physical conditions before the Taiwan 7.7 Mw EQ provide evidence that the pre-EQ sub-ULF (<1 min) magnetic wave activity may indicate a potential cause and not the result of tectonic stresses. This hypothesis is consistent with the fact that MHD generators have been used to activate local earthquakes (Pulinets and Boyarchuk, 2004; Sorokin et al., 2012; Novikov et al., 2017). The impact of electrical pulses of DC current injected through emitting dipole into the earth crust on the spatial and temporal distribution of weak seismicity has been confirmed (Tarasova et al., 2000; Tarasov and Tarasova, 2002, 2004; Chelidze et al., 2006). Furthermore, modern laboratory studies of electric triggering of macro events (laboratory “earthquakes”) were carried out at simplified slider system (Chelidze et al., 2002) and spring-block model simulated the seismogenic fault (Novikov et al., 2020).
The statistical results of Anagnostopoulos et al. (2021) and those of the present case study suggest that enhanced seismicity is preceded by CIRs and CIR-induced weak or moderate, but long lasting geomagnetic disturbances. In several cases examined, including the case of the present study (Taiwan 1999) some repeated CIRs, with a period of ∼27 days, precede great EQs. We do believe that thereafter a good analysis of space weather should not be ignored in earthquake prediction research. In particular, a weak geomagnetic activity may be misleading criterion concerning the origin of EQ EM precursory phenomena. Recently, Biswas et al. (2020) presented a paper, where they tried to separate the contamination in ionospheric parameters due to geomagnetic storm and acoustics parameters.
Although, the CME-driven storm is usually a strong phenomenon, it lasts for a short time period; from a few hours to a few days (Tsurutani et al., 2006). The HSSs/CIRs cause weaker magnetic disturbances in Earth’s magnetic field than the CMEs, but during a quiet Sun high speed solar wind streams corotate with the Sun’s 27-days period. Under such conditions several CIRs can affect the Earth’s magnetosphere semi-continuously for a long period of some months. During these long time periods, CIRs can transfer much more energy into the magnetosphere than a single CME (Tsurutani et al., 2006). For this reason high seismicity is well related with the presence of CIR–induced geomagnetic events around the minimum phase of the solar cycle (Gulyaeva, 2014; Rekapali, 2014; Anagnostopoulos et al., 2021). Other external sources, as for instance CME-driven events or Earth tides, possibly make some minor contribution to the preparation process of great earthquakes, but there is not such significant observational evidence.
We note that our understanding of the CIRs–induced sub-ULF electromagnetic radiation is a partner and not just a triggering agent of great earthquakes. This understanding comes, among other reasons, from the fact that all of the 16 giant (M ≥ 8.5) EQs between 1900 and 2017 occurred during the decay, minimum and the rising phase of the solar cycle or at times of a strong reduction, that is under space weather conditions of a quiet Sun.
In concluding, our recent results suggest that the planet Earth should not be considered as an autonomous body in earthquake prediction research, but we should think about the lithosphere as a boundary between two different cosmic regions, the planet Earth and space. This fact implies that EQ prediction research can gain much from complementary space weather research.
Data Availability Statement
The original contributions presented in the study are included in the article/Supplementary Material, further inquiries can be directed to the corresponding author.
Author Contributions
The author confirms being the sole contributor of this work and has approved it for publication
Conflict of Interest
The author declares that the research was conducted in the absence of any commercial or financial relationships that could be construed as a potential conflict of interest.
Publisher’s Note
All claims expressed in this article are solely those of the authors and do not necessarily represent those of their affiliated organizations, or those of the publisher, the editors and the reviewers. Any product that may be evaluated in this article, or claim that may be made by its manufacturer, is not guaranteed or endorsed by the publisher.
Acknowledgments
The author thanks the PIs and the teams/Institutions providing the online data of the EPAM- MAG-SWEPAM onboard as well as World Data Center for Geomagnetism, Kyoto. The author also thanks his student P. Karagiorgas for his help in the construction of some figures.
References
Abdu, M. A., de Souza, J. R., Sobral, J. H. A., and Batista, I. S. (2006). Magnetic Storms Associated Disturbance Dynamo Effects in the Low and Equatorial Latitude Ionosphere, Magnetic Storms Caused by Corotating, Geophys. Res. Series 167, Recurrent Magnetic Streams. Washington, DC: AGU, 283–304. doi:10.1029/167gm22
Akhoondzadeh, M., Parrot, M., and Parrot, M. (2010). Electron and Ion Density Variations before strong Earthquakes (M>6.0) Using DEMETER and GPS Data. Nat. Hazards Earth Syst. Sci. 10, 7–18. doi:10.5194/nhess-10-7-2010
Akinaga, Y., Hayakawa, M., Liu, J. Y., Yumoto, K., and Hattori, K. (2001). A Precursory ULF Signature for the Chi-Chi Earthquake in Taiwan. Nat. Hazards Earth Syst. Sci. 1 (33–36), 33–36. doi:10.5194/nhess-1-33-2001
Alfvén, H. (1950). Cosmical Eletrodynamics (The International Series of Monographs on Physics). Clarendon Press. http://ia600703.us.archive.org/23/items/CosmicalElectrodynamics/Alfven-CosmicalElectrodynamics.pdf.
Anagnostopoulos, G. (2015). A Study of Correlation between Seismicity and Mental Health: Crete, 2008-2010. Geomatics, Nat. Hazards Risk 6, 145–175. doi:10.1080/19475705.2013.819385
Anagnostopoulos, G., Louri, I., Marhavilas, P., and Sarris, E. (2009). Jovian Periodicities (∼10 H, ∼40 Min) on Ulysses’ Distant Jupiter Encounter Observations Around the Halloween CIR Events. Adv. Space Res. 43, 573–581. doi:10.1016/j.asr.2008.09.024
Anagnostopoulos, G., Menesidou, S. A., Vassiliadis, V., and Rigas, A. (2015) Correlation between Solar Particles and Temperature in North-East USA, Proceedings, 12th Hel.A.S Conference.
Anagnostopoulos, G., and Papandreou, A. (2012). Space Conditions during a Month of a Sequence of Six M > 6.8 Earthquakes Ending with the Tsunami of 26 December 2004. Nat. Hazards Earth Syst. Sci. 12, 1551–1559. doi:10.5194/nhess-12-1551-2012
Anagnostopoulos, G., Spyroglou, I., Rigas, A., Preka-Papadema, P., Mavromichalaki, H., and Kiosses, I. (2021). The Sun as a Significant Agent Provoking Earthquakes. Eur. Phys. J. Spec. Top. 230, 287–333. doi:10.1140/epjst/e2020-000266-2,
Anagnostopoulos, G., Vassiliadis, V., Basta, M., Rigas, A., and Vgontzas, A. (2016).Study of Seismogenic ULF Radiation with Satellites and Mental Diseases. Paphos, Cyprus: Fourth International Conference on Remote Sensing and Geoinformation of Environment.
Anagnostopoulos, G., Vassiliadis, E., and Pulinets, S. (2012). Characteristics of Flux-Time Profiles, Temporal Evolution, and Spatial Distribution of Radiation-belt Electron Precipitation Bursts in the Upper Ionosphere before Great and Giant Earthquakes. Ann. Geophys. 55, 21–36. Available at: https://www.annalsofgeophysics.eu/index.php/annals/article/view/5365/5677.
Athanasiou, M. A., Anagnostopoulos, G. C., and David Machairidis, C. N. G. G. (2014). The Ultra Low Frequency Electromagnetic Radiation Observed in the Topside Ionosphere above Boundaries of Tectonic Plates. Res. Geophys. 4, 5001.
Athanasiou, M. A., Anagnostopoulos, G. C., and Iliopoulos, A. C. (2011). Enhanced ULF Radiation Observed by DEMETER Two Months Around the strong 2010 Haiti Earthquake. Nat. Hazards Earth Syst. Sci. 11, 1091–1098. doi:10.5194/nhess-11-1091-2011
Avakyan, S. V., Voronin, N. A., and Nikol’sky, G. A. (2015). Response of Atmospheric Pressure and Air Temperature to the Solar Events in October 2003. Aeron 55 (8), 1180–1185. doi:10.1134/s0016793215080034
Badruddin, F., Mustajab, F., and Derouich, M. (2018). Relative Geoeffectiveness of High-Speed Solar Wind Streams from Different Solar Sources. Adv. Space Res. 62 (4), 765–784. doi:10.1016/j.asr.2018.05.024Mustajab
Baker, D., Erickson, P. J., Fennell, P., J. F., Foster, J., J. C., Jaynes, A. N., and Verronen, A., P. T. (2018). Space Weather Effects in the Earth’s Radiation Belts. Space Sci. Rev. 214, 17. doi:10.1007/s11214-017-0452-7
Bakhmutov, V., Sedova, F., and Mozgovaya, T. (2007). Morphological Features in the Structure of Geomagnetic Variations in Relation to Earthquakes in Vrancea Publ. Inst. Geophys. Pol. Acad. Sci. 43, 931. doi:10.1134/s1069351307110031
Balasis, G., and Mandea, M. (2007). Can Electromagnetic Disturbances Related to the Recent Great Earthquakes Be Detected by Satellite Magnetometers. Tectonophysics 431, 173–195. doi:10.1016/j.tecto.2006.05.038
Barnes, A. (1983). “Hydromagnetic Waves, Turbulence, and Collisionless Processes in the Interplanetary Medium,” in Solar-Terrestrial Physics: Principles and Theoretical Foundations. Editors R. L. Carovillano, and J. M. Forbes, 155–199. doi:10.1007/978-94-009-7194-3_7
Bentley, S. N., Watt, C. E. J., Owens, M. J., and Rae, I. J. (2018). ULF Wave Activity in the Magnetosphere: Resolving Solar Wind Interdependencies to Identify Driving Mechanisms. J. Geophys. Res. Space Phys. 123 (4), 2745–2771. doi:10.1002/2017ja024740
Biswas, S., Kundu, S., Ghosh, S., Chowdhury, S., Yang, S., Hayakawa, M., et al. (2020). Contaminated Effect of Geomagnetic Storms on Pre-seismic Atmospheric and Ionospheric Anomalies during Imphal Earthquake. Open J. Earthquake Res. 9, 383–402. doi:10.4236/ojer.2020.95022
Borovsky, J. E., and Denton, M. H. (2006). Differences between CME-Driven Storms and CIR-Driven Storms. J. Geophys. Res. 111, A07S08. doi:10.1029/2005JA011447
Borovsky, J., and Valdivia, J. (2018). The Earth’s Magnetosphere: A Systems Science Overview and Assessment. Surv. Geophys. 39 (5), 817–859. doi:10.1007/s10712-018-9487-x
Borovsky, Y., and Shprits, Y. (2017). Is the Dst Index Sufficient to Define All Geospace Storms. J. Geophys. Res. Space Phys. 122 (11), 11,543–11,611. doi:10.1002/2017JA024679
Burlaga, L. F., Ness, N. F., and Belcher, J. W. (1997). Radial Evolution of Corotating Merged Interaction Regions and Flows between _14AU and _43AU. J. Geophys. Res. 102 (4661–4), 671. doi:10.1029/96ja03629
Burton, R. K., McPherron, R. L., and Russell, C. T. (1975). An Empirical Relationship between Interplanetary Conditions and Dst. J. Geophys. Res. 80 (31), 4204–4214. doi:10.1029/JA080i031p04204
Błeçki, J., Parrot, M., and Wronowski, R. (2010). Studies of the Electromagnetic Field Variations in ELF Frequency Range Registered by DEMETER over the Sichuan Region Prior to the 12 May 2008 Earthquake. Int. J. Remote Sens. 31 (13), 3615–3629. doi:10.1080/01431161003727754
Campbell, W.H. (2009). Natural Magnetic Disturbance Fields, Not Precursors, Preceding the Loma Prieta Earthquake. J. Geophy. Res. Space Phys. 114, A05307. doi:10.1029/2008JA013932
Chang, S.-H., and Wang, W.-H. (2002). Estimates of Source Parameters of Two Large Aftershocks of the 1999 Chi-Chi, Taiwan, Earthquake in the Chia-Yi Area. Terr.. Atmospheric Ocean. Sci. 17 (2), 331–343. doi:10.3319/TAO.2006.17.2.331(T)
Chelidze, T., De Rubeis, V., Matcharshvili, T., and Tosi, P. (2006). Influence of strong Electromagnetic Discharges on the Dynamic of Earthquakes Time Distribution in the Bishkek Test Area (Central Asia). Ann. Geophys. 49 (4-5), 961–975.
Chelidze, T., Varamashvili, N., Devidze, M., Chelidze, Z., Chikladze, V., and Matcharashvili, T. (2002). Laboratory Study of Electromagnetic Initiation of Slip. Ann. Geophys. 45 (5), 587–598.
ChenKuo, Y.-G. Y. T., Wu, Y-M., Chen, H.-L., Chang, C.-H., Chen, R.-Y., Lo, P.-W., et al. (2008). Seismology New Seismogenic Source and Deep Structures Revealed by the 1999 Chia-Yi Earthquake Sequence in Southwestern Taiwan. Geophys. J. Int. 172, 1049–1054. doi:10.1111/j.1365-246X.2007.03686.xGJI
Cochran, E. S., Vidale, J. E., and Tanaka, S. (2004). Earth Tides Can Trigger Shallow Thrust Fault Earthquakes. Sci. 306, 1164–1166.
Daglis, I., Thorne, R., Baumjohann, W., and Orsini, S. (1999). The Terrestrial Ring Current Origin, Formation, and Decay. Rev. Geophys. 37 (4), 407–438. doi:10.1029/1999rg900009
De Santis, A., Balasis, G., Pavón-Carrasco, F. J., Cianchini, G., and Mandea, M. (2017). Potential Earthquake Precursory Pattern from Space: The 2015 Nepal Event as Seen by Magnetic Swarm Satellites. Earth Planet. Sci. Lett. 461, 119–126. doi:10.1016/j.epsl.2016.12.037
De Santis, A., De Franceschi, G., Spogli, L., Perrone, L., Alfonsi, L., Qamili, E., et al. (2015). Geospace Perturbations Induced by the Earth: the State of the Art and Future Trends. Phys. Chem. Earth Parts A/b/c 85– 86, 17–33. doi:10.1016/j.pce.2015.05.004
Dessler, A. J., and Parker, E. N. (1959). Hydromagnetic Theory of Geomagnetic Storms. J. Geophys. Res. 64 (12), 2239–2252. doi:10.1029/JZ064i012p02239
Duma, G., and Ruzhin, Y. (2003). Diurnal Changes of Earthquake Activity and Geomagnetic SqvariationsNat. Hazards Earth Syst. Sci. 3, 171. doi:10.5194/nhess-3-171-2003
Dungey, J. W. (1961). Interplanetary Magnetic Field and Auroral Zones. Phys. Rev. Lett. 6 (2), 47–48. doi:10.1103/physrevlett.6.47
Emery, B., Richardson, I., Evans, D., and Rich, F. (2009). Solar Wind Structure Sources and Periodicities of Auroral Electron Power over Three Solar Cycles. J. Atmosph. Solar-terrest. Phys. 71, 10–11. doi:10.1016/j.jastp.2008.08.005
Emter, C. (1997). “Tidal Triggering of Earthquakes and Volcanic Events,” in Tidal Phenomena, Vol. 66 of Lecture Notes in Earth Sciences. Editors H. Wilhem, W. Zürn, and H.-G. Wenzel (Springer-Verlag), 293–310.
Engebretson, M., Glassmeier, K.-H., Stellmacher, M., Hughes, W. J., and Lühr, H. (1998). The Dependence of High-Latitude Pc5 Wave Power on Solar Wind Velocity and on the Phase of High-Speed Solar Wind Streams. J. Geophys. Res. 103 (11), 26,271–26,283.
Firoz, K. (2019). On the Relation between Flare and CME during GLE-SEP and Non-GLE-SEP Events. Ap. J., 883.
Fraser, B. (2009). ULF Waves: Exploring the Earth’s Magnetosphere. Adv. Geophys, 1–32. doi:10.1142/9789812836205_0001
Fraser-Smith, A. C., McGill, P. R., and Helliwell, R. A. (1994). Ultra-Low Frequency Magnetic Field Measurements in Southern California during the Northridge Earthquake of 17 January. Geophys. Res. Lett. 21, 2195–2198.
Freund and Pilorz (2012). Electric Currents in the Earth Crust and the Generation of Pre-earthquake ULF Signals. The Frontier of EQ Prediction Studies. Nihon-Senmontosho-Shuppan, Tokyo: M. Hayakawa, 468–508.
Freund, F. T. (2013). Earthquake Forewarning – a Multidisciplinary challenge from the Ground up to Space. Acta Geophys. 61, 775–807. doi:10.2478/s11600-013-0130-4
Freund, F. T. (2010). Toward a Unified Solid State Theory for Pre-earthquake Signals. Acta Geophysica 58 (5), 719–766. doi:10.2478/s11600-009-0066-x
Gerontidou, M., Mavromichalaki, H., and Daglis, T. (2018). High-Speed Solar Wind Streams and Geomagnetic Storms during Solar Cycle 24. Sol. Phys. 293, 131. doi:10.1007/s11207-018-1348-8
Gold, R. E., Krimigis, S. M., Hawkins, S. E., Haggerty, D. K., Lohr, D. A., Fiore, E., et al. (1998). Electron, Proton, and Alpha Monitor on the Advanced Composition Explorer Spacecraft. Space Sci. Rev. 86, 541–562. doi:10.1007/978-94-011-4762-0_19
Gousheva, M. N., Georgiva, K. Y., Kirov, B. B., and Atanssov, D. (2003). On the Relation between Solar Activity and Seismicity. Proc. Int. Conf. Recent Adv. Space Tech., 20–22. doi:10.1109/rast.2003.1303913
Gray, L., Beer, J., Geller, M., Haigh, J., Lockwood, M., Matthes, K., et al. (2010). Solar Influences on Climate. Rev. Geophys. 48, 1–53. doi:10.1029/2009RG000282
Greenspan, M. E., and Hamilton, D. C. (2000). A Test of the Dessler-Parker-Sckopke Relation during Magnetic Storms. J. Geophys. Res. 105 (A3), 5419–5430. doi:10.1029/1999JA000284
Grimalsky, V., Kotsarenko, A., Pulinets, S., and Perez-Enriquez, R. (2010). On the Modulation of Intensity of Alfven Resonances Before Earthquakes: Observations and Model. J. Atmos. Sol.-Terr. Phys. 72 (1), 1–6. doi:10.1016/j.jastp.2009.09.017
Gulyaeva, T. (2014). Association of Seismic Activity with Solar Cycle and Geomagnetic Activity, Developm. Earth Sci. 2, 14. http://www.seipub.org/des.
Han, P., Zhuang, J., Hattori, K., Chen, C-H., Febriani, F., Chen, H., et al. (2020). Assessing the Potential Earthquake Precursory Information in ULF Magnetic Data Recorded in Kanto, Japan during 2000–2010: Distance and Magnitude Dependences. Entropy 22, 859. doi:10.3390/e22080859
Hattori, K., and Han, P. (2018). “Statistical Analysis and Assessment of Ultralow Frequency Magnetic Signals in Japan as Potential Earthquake Precursors,” in Pre-Earthquake Processes. Editors D. Ouzounov, S. Pulinets, K. Hattori, and P. Taylor. doi:10.1002/9781119156949.ch13
Hattori, K. (2004). ULF Geomagnetic Changes Associated with Large Earthquakes. Terr. Atmos. Ocean. Sci. 15, 329–360. doi:10.3319/tao.2004.15.3.329(ep)
Hayakawa, M., and Fujinawa, Y. (1994). Electromagnetic Phenomena Related to Earthquake Prediction. Tokyo: Terra Sci. Pub. Co., 67–71.
Hayakawa, M., Hattori, K., and Ohta, K. (2007). Monitoring of ULF (Ultra-low-frequency) Geomagnetic Variations Associated with Earthquakes. Sensors 7, 1108–1122. doi:10.3390/s7071108
Hayakawa, M., Kasahara, Y., Nakamura, T., Hobara, Y., Rozhnoi, A., Solovieva, M., et al. (2010). A Statistical Study on the Correlation between Lower Ionospheric Perturbations as Seen by Subionospheric VLF/LF Propagation and Earthquakes. J. Geophys. Res. Atmospheres 115, A9. doi:10.1029/2009JA015143
Hayakawa, M., Kawate, R., Oleg, A., Molchanov, O., and Yumoto, K. (1996). Results of Ultra-Low-Frequency Magnetic Field Measurements During the Guam Earthquake of 8 August 1993. Geophys. Res. Lett. 23(3), 241–244. doi:10.1029/95GL02863
Huang, M., Wang, J.-H., Hwang, R.-D., and Chen, K.-C. (2006). Mechanical Properties, Slip and Nucleation of the 1999 Chia-Yi Earthquake: the Question of Static Stress Influence from the 1999 Chi-Chi Earthquake. Terr. Atmospheric Ocean. Sci. 13(3), 299–312. doi:10.3319/TAO.2002.13.3.299(CCE)
Huzaimy, J., and Yumoto, K. (2011). Possible Correlation between Solar Activity and Global Seismicity. Proceeding 2011 IEEE Int. Conf. Space Sci. Commun. (Iconspace), 138–141. doi:10.1109/IConSpace.2011.6015869
Hynönen, R., Tanskanen, E. I., and Francia, P. (2020). Solar Cycle Evolution of ULF Wave Power in Solar Wind and on Ground. J. Space Weather Space Clim. 10, 43. doi:10.1051/swsc/2020046
Jacobs, J., Kato, Y., Matsushita, S., and Troitskaya, V. (1964). Classification of Geomagnetic Micropulsations. J. Geophys. Res. Space Sci. 69, 1–181. doi:10.1029/JZ069i001p00180
Kataoka, R., and Miyoshi, Y. (2006). Flux Enhancement of Radiation belt Electrons during Geomagnetic Storms Driven by Coronal Mass Ejections and Corotating Interaction Regions. Space Weather 4, S09004. doi:10.1029/2005SW000211
Kavanagh, A., and Denton, M. (2007). High-speed Solar-Wind Streams and Geospace Interactions. Astron. Geophys. 48 (6), 6.24–6.26. doi:10.1111/j.1468-4004.2007.48624.x
Kennedy, M., Vidale, J. E., and Parker, M. G. (2004). Earthquakes and the Moon: Syzygy Predictions Fail the Test. Seismol. Res. Lett. 75, 607–612. doi:10.1785/gssrl.75.5.607
Kepko, L., SpenceSinger, H. E. H. J., and Singer, H. J. (2002). ULF Waves in the Solar Wind as Direct Drivers of Magnetospheric Pulsations. Geophys. Res. Lett. 29 (8), 39–41. doi:10.1029/2001GL014405
Kessel, R. L. (2008). Solar Wind Excitation of Pc5 Fluctuations in the Magnetosphere and on the Ground. J. Geophys. Res. 113, A04202. doi:10.1029/2007JA012255
Khain, V. E., and Kalilov, E. N. (2008). About the Possible Influence of Solar Activity upon Seismic and Volcanic Activities: Long-Term Forecast, Science without Borders. Trans. Inter. Acad. Sci. H&E., SWB 3, 217–240.
Kim, K.-H., Cattell, C. A., Lee, D.-H., Takahashi, K., Yumoto, K., Shiokawa, K., et al. (2002). Magnetospheric Responses to Sudden and Quasiperiodic Solar Wind Variations. J. Geophys. Res. Space Sci. 107, A111–A112. doi:10.1029/2002JA009342
Kivelson, G. M., and Russell, C. T. (1995). Introduction to Space Physics. Cambridge University Press.
Kumar, A., and Kumar, S. (2018). Solar Flare Effects on D-Region Ionosphere Using VLF Measurements during Low- and High-Solar Activity Phases of Solar Cycle, 24. Earth Planets Space 70, 29. doi:10.1186/s40623-018-0794-8
Liemohn, M., Kozyra, W., Thomsen, J., Roeder, M., Lu, J., Borovsky, J., et al. (2001). Dominant Role of the Asymmetric Ring Current in Producing Storm Time Dst*. J. Geophys. Res. 106 (A6), 10,883–10,904. doi:10.1029/2000JA000326
Lin, J.-W. (2013). Taiwan’ Chi-Chi Earthquake Precursor Detection Using Nonlinear Principal Component Analysis to Multi-Channel Total Electron Content Records. J. Earth Sci. 24, 244–253. doi:10.1007/s12583-013-0325-2
Liu, J., Chen, Y., Chuo, Y., and Tsai, H. (2001). Variations of Ionospheric Total Electron Content during the Chi-Chi Earthquake. Geophys. Res. Lett. 28 (7), 1383–1386. doi:10.1029/2000gl012511
Liu, J., Chen, Y., Pulinets, S., Tsai, Y., and Chuo, Y. (2000). Seismo-ionospheric Signatures Prior to M ≥ 6.0 Taiwan Earthquakes. Geophys. Res. Lett. 27 (19), 3113–3116. doi:10.1029/2000gl011395
Liu, J., Chuo, Y., Shan, S., Tsai, Y., Chen, Y., Pulinets, S., et al. (2004). Pre-Earthquake Ionospheric Anomalies Registered By Continuous Gps Tec Measurements. Ann. Geophys. 22, 1585–1593. doi:10.5194/angeo-22-1585-2004
Loewe, C. A., and Prolss, G. W. (1997). Classification of Mean Behavior of Magnetic Storms. J. Geophys. Res. 102 (A7), 14209–14214. doi:10.1029/96JA04020
Love, J.J., and Thomas, J.N. (2013). Insignificant Solar-Terrestrial Triggering of Earthquakes. Geophys. Res. Lett. 40, 1165. doi:10.1002/grl.50211
Ma, K. F., Lee, C. T., Tsai, Y. B., Shin, T. C., and Mori, J. (1999). The Chi-Chi, Taiwan Earthquake: Large Surface Displacements on an Inland Thrust Fault. EOS Trans. Am. Geophys. Union 80, 605–611. doi:10.1029/99eo00405
Marhavilas, P., Malandraki, O., and Anagnostopoulos, G. (2015). Survey of Caveats in Low-Energy Particle Measurements: Ulysses/HI-SCALE and ACE/EPAM Instruments, Planet. Space Sci. 117, 192206. doi:10.1016/j.pss.2015.06.010
Marhavilas, P. (2007). The Stormy Sun Affecting the Human Life and the Technology. EGU Newsl. 17, 13. Available at: https://cdn.egu.eu/static/e738470d/newsletter/eggs/eggs_19.pdf.
McPherron, R. (2005). Magnetic Pulsations: Their Sources and Relation to Solar Wind and Geomagnetic Activity. Surv. Geophys. 26, 545–592. doi:10.1007/s10712-005-1758-7
McComas, D., Bame, S., Barker, P., Feldman, W. C., Philips, J. L., Riley, P., et al. (1998). Solar Wind Electron Proton Alpha Monitor (SWEPAM) for the Advanced Composition Explorer. Space Sci. Rev. 86, 563–612. doi:10.1023/A:1005040232597
Métivier, L., de Viron, Ο., Conrad, C., Renault, S., Diament, M., and Patau, G. (2009). Evidence of Earthquake Triggering by the Solid Earth Tides. Earth Planet. Sci. Lett. 278, 370–375. doi:10.1016/j.epsl.2008.12.024
Molchanov, O. A., and Hayakawa, M. (1998). Subionospheric VLF Signal Perturbations Possibly Related to Earthquakes. J. Geophys. Res. 103 (17), 17489–17504. doi:10.1029/98ja00999
Molchanov, O., and Hayakawa, M. (1995). Generation of ULF Electromagnetic Emissions by Microfracturing. Geohpys. Res. Lett. 22, 3091–3094. doi:10.1029/95gl00781
Morley, S. K., Rouillard, A. P., and Freeman, M. P. (2008). Recurrent Substorm Activity during the Passage of a Corotating Interaction Region. J. Atmosph. Solar-terrest. Phys. 71, 10–11. doi:10.1016/j.jastp.2008.11.009
Novikov, V., Okunev, V., Klyuchkin, V., Liu, J., Ruzhin, Ya, Y., and Shen, X. (2017). Electrical Triggering of Earthquakes: Results of Laboratory Experiments at spring-block Models. Earthq. Sci. 30, 167–172. doi:10.1007/s11589-017-0181-8
Novikov, V., Ruzhin, Y., Sorokin, V., and Yaschenko, A. (2020). Space Weather and Earthquakes: Possible Triggering of Seismic Activity by strong Solar Flares. Anal. Gelphys. 63, 5. doi:10.4401/ag-7975
Odintsov, S. D., Ivanov-Klodonyi, G. S., and Gergieva, K. (2007). Solar Activity and Global Seismicity on Earth. Bull. Russ. Aca. Sci. Phys. 71 (4), 593–597. doi:10.3103/s1062873807040466
Ohta, K., Umeda, K., Watanabe, N., and Hayakawa, M. (2001). ULF/ELF Emissions Observed in Japan, Possibly Associated with the Chi-Chi Earthquake in Taiwan. Nat. Hazards Earth Syst. Sci. 1, 37–42. doi:10.5194/nhess-1-37-2001
Ohtani, S., Nose, M., Rostoker, G., Singer, H., Lui, A. T. Y., and Nakmura, M. (2001). Stormsubstorm Relationship: Contribution of the Tail Current to Dst. J. Geophys. Res. 106 (A10), 21. doi:10.1029/2000JA000400
Ouzounov, D., and Freund, F. (2004). Mid-infrared Emission Prior to strong Earthquakes Analyzed by Remote Sensing Data. Adv. Space Res. 33, 268–273. doi:10.1016/s0273-1177(03)00486-1
Ouzounov, D., Pulinets, S., Hattori, K., Taylor, P., and Ed’s, (2018). Pre-Earthquake Processes: A Multidisciplinary Approach to Earthquake Prediction Studies. American Geophysical UnionJohn Wiley & Sons, Inc., 385.
Ouzounov, D., Pulinets, S., Kafatos, M. C., and Taylor, P. (2018). “Thermal Radiation Anomalies Associated with Major Earthquakes,” in Pre-Earthquake Processes. Editors D. Ouzounov, S. Pulinets, K. Hattori, and P. Taylor. doi:10.1002/9781119156949.ch15
PahudI, D., RaeI, J., Mann, K. R., Murphy, R., and Amalraj, V. (2009). Ground-based Pc5 ULF Wave 1057 Power: Solar Wind Speed and MLT Dependence. J. Atmosph. Solar-Terr. Phys. 71 (10–11), 1082–1092. doi:10.1016/j.jastp.2008.12.004
Parrot, M., Benoist, D., Berthelier, J. J., Blecki, J., Chapuis, Y., Colin, , et al. (2006). The Magnetic Field experiment IMSC and its Data Processing Onboard DEMETER:scientific Objectives, Description and First Results. Planet. Space Sci. 54, 441–455. doi:10.1016/j.pss.2005.10.015
Patsourakos, S., Georgoulis, M. K., Vourlidas, A., Nindos, A., Sarris, T., Anagnostopoulos, G., et al. (2016). The Major Geoeffective 1067 Solar Eruptions of 2012 March 7: Comprehensive Sun-To-Earth Analysis. Astrophys. J. 817 (14), 114. doi:10.3847/0004-637x/817/1/14
Pilipenko, V. (1990). ULF Waves on the Ground and in Space. J. Atm. Terrst. Phys. 52 (12), 11931069–11931209.
Phillips, J. L., Bame, S. J., Barnes, A., Barraclough, B. L., Feldman, W. C., Goldstein, B. E., et al. (1995). Ulysses Solar Wind Plasma Observations from Pole to Pole. Geophys. Res. Lett. 22 (23), 3301–3304. doi:10.1029/95GL03094
Poole, C., Kavet, R., Funch, D. P., Donelan, K., Charry, J. M., and Dreyer, N. A. (1993). Depressive Symptoms and Headaches in Relation to Proximity of Residence to an Alternating-Current Transmission Line Right-Ofway. Am. J. Epidemiol. 137, 318–330. doi:10.1093/oxfordjournals.aje.a116679
Potapov, A. S. (2013). ULF Wave Activity in High-Speed Streams of the Solar Wind: Impact on the 1060 Magnetosphere. J. Geophys. Res. Space Phys. 118, 6465–6477. doi:10.1002/2013JA019119
Pulinets, S., and Ouzounov, D. (2011). Lithosphere-Atmosphere-Ionosphere Coupling (LAIC) Model—A Unified Concept for Earthquake Precursors Validation. J. Asian Earth Sci. 1073, 371–382. doi:10.1016/j.jseaes.2010.03.005
Pulinets, S., and Ouzounov, D. (2018). The Possibility of Earthquake Forecasting: Learning from Nature. Bristol, UK: Institute of Physics Books, IOP Publishing, 168.
Reichmanis, M., Perry, F. S., Marino, A. A., and Becker, R. O. (1979). Relation between Suicide and the 1078 Electromagnetic Field of Overhead Power Lines. Physiol. Chem. Phys. 11, 395–403.
Rekapali, R. (2014). Brief Communication: Correlation of Global Earthquake Rates with Temperature 1081 and sunspot Cycle, Natural Hazards and Earth Syst. Sci. doi:10.5194/nhessd-2-2851-2014
Richardson, I. G., and Cane, H. V. (2011). Geoeffectiveness (Dst and Kp) of Interplanetary Coronal Mass Ejections during 1995–2009 and Implications for Storm Forecasting. Space Weather 9, S07005. doi:10.1029/2011sw000670
Richardson, I. G., and Cane, H. V. (2012a). Solar Wind Drivers of Geomagnetic Storms during More Than Four Solar Cycles. J. Space Weather Space Clim. 2 (A01), A01–A09. doi:10.1051/swsc/2012001
Richardson, I. G., and Cane, H. V. (2012b). Near-earth Solar Wind Flows and Related Geomagnetic Activity during More Than Four Solar Cycles (1963-2011). J. Space Weather Space Clim. 2, A02. doi:10.1051/swsc/2012003
Richardson, I. G. (2018). Solar Wind Stream Interaction Regions throughout the Heliosphere. Living Rev. Sol. Phys. 15, 1. doi:10.1007/s41116-017-0011-z
Russell, C. T., Ginskey, M., Petrinec, S., and Le, G. (1992). The Effect of Solar Wind Dynamic Pressure Changes on Low and Mid-Latitude Magnetic Records. Geophys. Res. Lett. 19(12), 1227–1230. doi:10.1029/92GL0116
Sarafopoulos, D. V. (2005). Cases for Which the Earth’s Magnetosphere Does not Act as a “Low-Pass Filter”. J. Atmos. Solar Terr. Phys. 67, 1427.
Satoshi, I., Suguru, Y., and Tanaka, Y. (2016). Earthquake Potential Revealed by Tidal Influence on Earthquake Size–Frequency Statistics. Nat. Geophys. Lett. doi:10.1038/NGEO2796
Sauvaud, J., Moreau, T., Maggiolo, R., et al. (2006). High Energy Electron Detection Onboard DEMETER:the IDP Spectro-Meter, Description and First Results on the Inner belt. Planet. Space Sci. 54, 502–511. doi:10.1016/j.pss.2005.10.019
Schuster, A. (1897). On Lunar and Solar Periodicities of Earthquakes. Proc. R. Soc. Lond. 61, 455–465. doi:10.1098/rspl.1897.0060
Shadrina, L. (2017), Two Types of Geomagnetic Storms and Relationship between Dst and AE Indexes. In E3S Web of Conferences 20, 01010 Solar-Terrestrial Relations and Physics of Earthquake 1084 Precursors. doi:10.1051/e3sconf/20172001010
Shen, X., Zhang, X., and Yuan, S. (2018). The State-Of-The-Art of the China Seismo-Electromagnetic 1086 Satellite mission. Sci. China Technol. Sci. 61, 634–642. doi:10.1007/s11431-018-9242-0
Shi, Q., Shen, X.-C., Tian, A., Degeling, A., Zong, Q., Fu, S., et al. (2020). “Vortices, ULF Waves, and Aurorae, Magnetosphere Response to Solar Wind Dynamic Pressure Change,”. Editors Q. Zong, P. Escoubet, D. Sibeck, G. Le, and H. Zhang. doi:10.1002/9781119509592
Shin, T.-C. (2004).An Overview of the 1999 Chi-Chi, Taiwan, Earthquake, Bulletin of the Seismolog. Albany, CA: Society of America, 5895. doi:10.1785/0120000738
Sidiropoulos, N., Anagnostopoulos, G., and Rigas, V. (2011). Comparative Study on Earthquake and Ground Based Transmitter Induced Radiation belt Electron Precipitation at Middle Latitudes. Nat. Hazards Earth Syst. Sci. 11, 1901. doi:10.5194/nhess-11-1901-2011
Simpson, J. F. (1967). Solar Activity as Triggering Mechanism for Earthquakes. Earth Planet. Sci. Lett. 3, 417–425. doi:10.1016/0012-821x(67)90071-4
Smith, E. J., Balogh, A., Neugebauer, M., and McComas, D. (1995). Ulysses Observations of Alfvén Waves in the Southern and Northern Solar Hemispheres. Geophys. Res. Lett. 22, 3381–3384. doi:10.1029/95gl03268
Smith, C. W., L’Heureux, J., Ness, N. F., Acuña, M. H., Burlaga, L. F., and Scheifele, J. (1998). The ACE Magnetic Fields Experiment. Space Sci. Rev. 86, 613–632. doi:10.1007/978-94-011-4762-0_21
Siscoe, G., McPherron, R., and Jordanova, V. K. (2005). K., Diminished Contribution of Ram Pressure to Dst during Magnetic Storms. J. Geophys. Res. Atmospheres 110 (A12), 1–7. doi:10.1029/2005JA011120
Sobolev, G. A., Zakrzhevskaya, N. A., and Kharin, E. P. (2001). On the Relation between Seismicity and Magnetic Storms. Phys. Solid Earth, Russ. Acad. Sc. 11, 62–72.
Sorokin, V, Yaschenko, A., Ruzhin, R., and Novikov, V. (2012).Model for Solar Flare Influence to the Seismic Activity. In Proc. EMSEV-2012 Workshop. Gotemba, Japan: Abstr, 3–10.
Sorokin, V. M., Yaschenko, A. K., and Novikov, V. A. (2019). A Possible Mechanism of Stimulation of Seismic Activity by Ionizing Radiation of Solar Flares. Earthq Sci. 32, 26–34. doi:10.29382/eqs-2019-0026-3
Southwood, D., and Hughes, W ( (1983). Theory of Hydromagnetic Waves in the Magnetosphere. Space Sci. Rev. 35 (4), 301–366. doi:10.1007/bf00169231
Stone, E., Frandsen, A., Mewaldt, R., Christian, E., Margolies, D. D., Ormes, J., et al. (1998). The Advanced Composition Explorer. Space Sci. Rev. 86, 1–22. doi:10.1007/978-94-011-4762-0_1
Straser, V., and Cataldi, G. (2015). Solar Wind Ionic Variation Associated with Earthquakes Greater that Magnitude 6.0, New Conc. Glob. Tect. J. 3, 2.
Sytinskii, A. D. (1997). Influence of Interplanetary Disturbances on the Seismicity and Tmosphere of the Earth. Geomagn. Aeron. 37, 138–143.
Takahashi, K., Yumoto, K., Claudepierre, S. G., Sanchez, E. R., Troshichev, O. A., and Janzhura, A. S. (2012). Dependence of the Amplitude of Pc5-Band Magnetic Field Variations on the Solar Wind and Solar Activity. J. Geophys. Res. 117, a–n. doi:10.1029/2011JA017120
Tanaka, S., Ohtake, M., and Sato, H. (2002). Evidence for Tidal Triggering of Earthquakes as Revealed from Statistical Analysis of Global Data. J. Geophys. Res. 107 (5B10), 2211.
Tarasov, N., and Tarasova, N. (2004). Spatial-temporal Structure of Seismicity of the North Tien Shan and its Change under Effect of High Energy Electromagnetic Pulses. Ann. Geoph. 47, 199–212.
Tarasov, N., Tarasova, N., Zeigarnik, V., and Avagimov, A. (2000). The Effect of High Energy Electromagnetic Pulses on Seismicity in Central Asia and Kazakhstan. J. Volcanol. Seismol. 21, 627–639.
Tarasov, N. T., and Tarasova, N. V. (2002). The Effect of Geomagnetic Storms on the Seismicity. In Proc. of the 3rd International Workshop on Magnetic, Electric and Electromagnetic Methods in Seismology and Volcanology, 3. Moscow, Russia–6.
Tolstoy, M., Vernon, F., Orcutt, J., and Wyatt, F., (2002) Breathing of the Seafloor: Tidal Correlations of Seismicity at Axial Volcano. Geol. Soc. Am. 30(6), 503–506.
Tramutoli, V., Corrado, R., Filizzola, C., Genzano, N., Lisi, M., and Pergola, N. (2015). From Visual Comparison to Robust Satellite Techniques: 30 Years of thermal Infrared Satellite Data Analyses for the Study of Earthquake Preparation Phases. B. Geofis. Teor. Appl. 56, 167–202.
Tsai, Y-B., Liu, J-Y., Shin, T.-C., Yen, H.-Y., Chen, C-H., et al. (2018). Multidisciplinary Earthquake Precursor Studies in Taiwan, A Review and Future Prospects. Washington, DC: Pre‐Earthquake Processes: A Multidisciplinary Approach to Earthquake Prediction Studies. doi:10.1002/9781119156949
Tsurutani, B. T., Echer, E., Guarnieri, F. L., and Gonzalez, W. D. (2011). The Properties of Two Solar Wind High Speed Streams and Related Geomagnetic Activity during the Declining Phase of Solar Cycle 23. J. Atmos. Solar-Terrestrial Phys. 73, 164–177. doi:10.1016/j.jastp.2010.04.003
Tsurutani, B., Lakhina, G., and Hajra, R. (2020). The Physics of Space Weather/solar-Terrestrial Physics (STP): what We Know Now and what the Current and Future Challenges Are. Nonlinear Process. Geoph. 27 (1), 75–119. doi:10.5194/npg-27-75-2020
Tsurutani, B. T., and Gonzalez, W. D. (1987). The Cause of High-Intensity Long-Duration Continuous AE Activity (HILDCAAs): Interplanetary Alfvén Wave Trains. Planet. Space Sci. 35, 405–412. doi:10.1016/0032-0633(87)90097-3
Tsurutani, B. T., McPherron, R. L., Gonzalez, W. D., Lu, G., Gopalswamy, N., and Guarnieri, F. L. (2006). “Magnetic Storms Caused by Corotating Solar Wind Streams,” in Recurrent Magnetic Storms: Corotating Solar Wind. Editor B. Tsurutani (Washington, DC: Geophysical Monographs, AGU), 167. doi:10.1029/167GM03
Urata, N., Duma, G. G., and Freund, F. (2018). Geomagnetic Kp Index and Earthquakes. Open J. Earthq. Res. 7, 39–52. Available at: http://www.scirp.org/journal/ojer. doi:10.4236/ojer.2018.71003
Uyeda, S., Nagao, T., and Kamogawa, M. (2009) Short-Term Earthquake Prediction: Current Status of Seismo-Electromagnetics, Tectonophysics 470, 205–213.
Vencloviene, J., Babarskiene, R., Slapikas, R., and Gintare Sakalyte, G. (2012). The Association between Phenomena on the Sun, Geomagnetic Activity, Meteorological Variables, and Cardiovascular Characteristic of Patients with Myocardial Infarction. J. Biometeorol. 57 (5), 797–804. doi:10.1007/s00484-012-0609-8
Villante, U., and Tiberi, P. (2016). Occurrence and Characteristics of Nighttime ULF Waves at Low Latitude: the Results of a Comprehensive Analysis.: Nighttime ULF Waves at Low Latitude. J. Geophys. Res. Space Phys. 121, 1. doi:10.1002/2015JA022137
Watari, S. (2018). Intense Geomagnetic Storms Associated with Coronal Holes under the Weak Solar-Wind Conditions of Cycle 24. Sol. Phys. 293, 23. doi:10.1007/s11207-018-1248-y
Wilcock, S. (2001). Tidal Triggering of Microearthquakes on the Juan de Fuca Ridge. Geophys. Res. Lett. 28(20), 3999–4002. doi:10.1029/2001GL013370
Wrenn, G. L. (2009). Chronology of “Killer” Electron: Solar Cycles 22 and 23. J. Atmos. Solar - Terrestrial Phys. 71 (10-11), 1210–1218. doi:10.1016/j.jastp.2008.08.002
Wrenn, G. L., Rodgers, D. J., and Ryden, K. A. (2002). A Solar Cycle of Spacecraft Anomalies Due to Internal Charging. Ann. de Geophys. 20 (7), 953–956. doi:10.5194/angeo-20-953-2002
Yen, H.-Y., Chen, C-H., Yeh, Y.-H., Liu, J.-Y., Lin, C.-R., and Tsai, Y.-B. (2004). Geomagnetic Fluctuations during the 1999 Chi-Chi Earthquake in Taiwan Horng-Yuan Zhang. Earth Planets Space 56, 39–45.
Zenchenko, Τ., and Breus, Τ. (2021). The Combined Effect of Space and Earth Weather Factors on Human Health and Well-Being. Atmosphere 12 (3), 346. doi:10.3390/atmos12030346
Zhang, X., Shen, X., Parrot, M., Zeren, Z., Ouyang, X., Liu, J., et al. (2012). Phenomena of Electrostatic Perturbations before strong Earthquakes (2005–2010) Observed on DEMETER. Nat. Hazards Earth Syst. Sci. 12 (), 75–83. doi:10.5194/nhess-12-75-2012
Keywords: ULF waves, earthquake generation process, space weather, earthquake electromagnetic precursors, earthquake prediction, sun-earth relationships, environmental electromagnetism
Citation: Anagnostopoulos G (2021) On the Origin of ULF Magnetic Waves Before the Taiwan Chi-Chi 1999 Earthquake. Front. Earth Sci. 9:730162. doi: 10.3389/feart.2021.730162
Received: 24 June 2021; Accepted: 03 August 2021;
Published: 03 November 2021.
Edited by:
Dimitar Ouzounov, Chapman University, United StatesReviewed by:
Georgios Balasis, National Observatory of Athens, GreeceSergey Alexander Pulinets, Space Research Institute (RAS), Russia
Copyright © 2021 Anagnostopoulos. This is an open-access article distributed under the terms of the Creative Commons Attribution License (CC BY). The use, distribution or reproduction in other forums is permitted, provided the original author(s) and the copyright owner(s) are credited and that the original publication in this journal is cited, in accordance with accepted academic practice. No use, distribution or reproduction is permitted which does not comply with these terms.
*Correspondence: Georgios Anagnostopoulos, Z2FuYWdub0BlZS5kdXRoLmdy