- Department of Earth and Biological Sciences, Loma Linda University, Loma Linda, CA, United States
The Permian Coconino Sandstone of northern Arizona contains numerous small-scale, soft-sediment deformation structures (SSDSs). These novel structures may be indicators of paleoenvironment or sedimentary processes. These SSD are generally shallow and occur on the surfaces of cross-beds, in contrast to convoluted bedding up to tens of meters thick commonly observed in some other eolian sandstones. These differences in structures imply differences in the processes that formed the Coconino Sandstone, or differences in the underlying depositional conditions. These SSDSs occur in outcrops at the Grand Canyon, and farther south in quarries near the towns of Seligman and Ash Fork. Size, orientation, structure, sedimentary context, clay content, and porosity of the structures are described. The SSDSs occur as small folds and ridges on the paleo lee side of otherwise undisturbed cross-beds. Some are associated with small rotated sandstone blocks within the cross-beds. The structures are exposed on bedding plane surfaces and in cross-section on vertical quarry walls. A few SSDSs up to a meter thick also occur in the Coconino Sandstone, but the others are only up to a few cm thick, 2–10 cm wide, and 20 cm to 10 m long. Evidence is presented that liquidization (as fluidization or liquefaction) may have been involved in producing these features, implying a high water content in scattered locations at time of deformation, but this process also requires some stressor to trigger the deformation. Seismic events may provide a triggering mechanism. The Coconino Sandstone SSDSs represent unusual or previously overlooked small-scale features related to individual foreset surfaces.
Introduction
Rare examples of soft sediment deformation (SSD) have previously been reported in the Coconino Sandstone (Coconino SS), northern Arizona, United States, ranging from centimeter scale up to 4 m thick (McKee and Bigarella 1979; Whitmore et al., 2011). The current work documents numerous additional soft sediment deformation structures (SSDSs)mostly on a centimeter scale, from this formation. These structures contrast strongly with much larger SSDSs—up to tens of meters thick—that have been reported in other cross-bedded sandstones (Sanderson 1974; Bryant and Miall 2010). Such large-scale deformation examples have been interpreted to require a high water table (to liquefy the sand) or a high-moisture depositional environment (Doe and Dott 1980; Horowitz 1982; Alvarez et al., 1998; Netoff 2002; Chan et al., 2007; Rodríguez-López et al., 2008; Bryant and Miall 2010; Chan and Bruhn 2014; Bryant et al., 2016). The liquidization processes are thought to facilitate the deformation of sediment before it is fully lithified (Allen 1977).
We hypothesize that the SSDSs reported here in the Coconino SS also require liquidization, but involved smaller-scale triggers and some paleoenvironmental differences. A large global literature deals with soft-sediment deformation (SSD), which is defined as deformation in unconsolidated sediment (Mills 1983; van Loon 2009; Shanmugam 2017). Common types of soft-sediment deformation structures (SSDSs) include folds, slumps, load structures, deformed cross-bedding, fluid-escape features and many others (Owen et al., 2011; Shanmugam 2017). These occur predominantly in coarse silt to fine sand (Mills 1983), and in both eolian and water-deposited facies (Cojan and Thiry 1992; Moretti 2000; Fernandes et al., 2007; Liesa et al., 2016). Deformation structures are commonly interpreted as products of liquidization (fluidization or liquefaction) triggered by seismic shaking. However, this is often impossible or very difficult to verify, so aseismic triggers must be considered before settling on a seismic interpretation (Owen et al., 2011; Owen and Moretti 2011; Shanmugam 2017).
The middle Permian Coconino SS is fairly uniform in texture and structure throughout, and with few exceptions consists of vertically stacked, wedge planar or tabular planar cross-bed sets (McKee, 1974; Blakey and Knepp, 1989; Middleton et al., 2003). The sets of cross-beds vary from <1 m to over 20 m thick (measured from base of set). Cross-bed dips range from 13 to 27°. The grains in this strongly-cemented fine-grained sandstone are predominantly quartz (88–95%) with minor amounts of feldspar (Maithel et al., 2019, 2021). The internal structure of the cross-beds was described by Middleton et al. (2003) as mostly thin laminae (<1 cm thick) that extend a considerable distance down dip within the set. As in other sandstones, the cross-beds are thought to represent preserved dune foresets, and Maithel et al. (2021) suggested that grainflow and grainfall processes were responsible for most fine-scale sediment deposition.
The Coconino SS lacks substantial dateable material, but it has been stratigraphically defined as middle Permian (Middleton et al., 2003). The formation extends across northern Arizona (Figure 1) and is approximately 100 m thick at the Grand Canyon, thickening to the south as far as the Mogollon Rim. Near the town of Ash Fork, the lower contact is not exposed, but the unit is at least 160 m thick in that area.
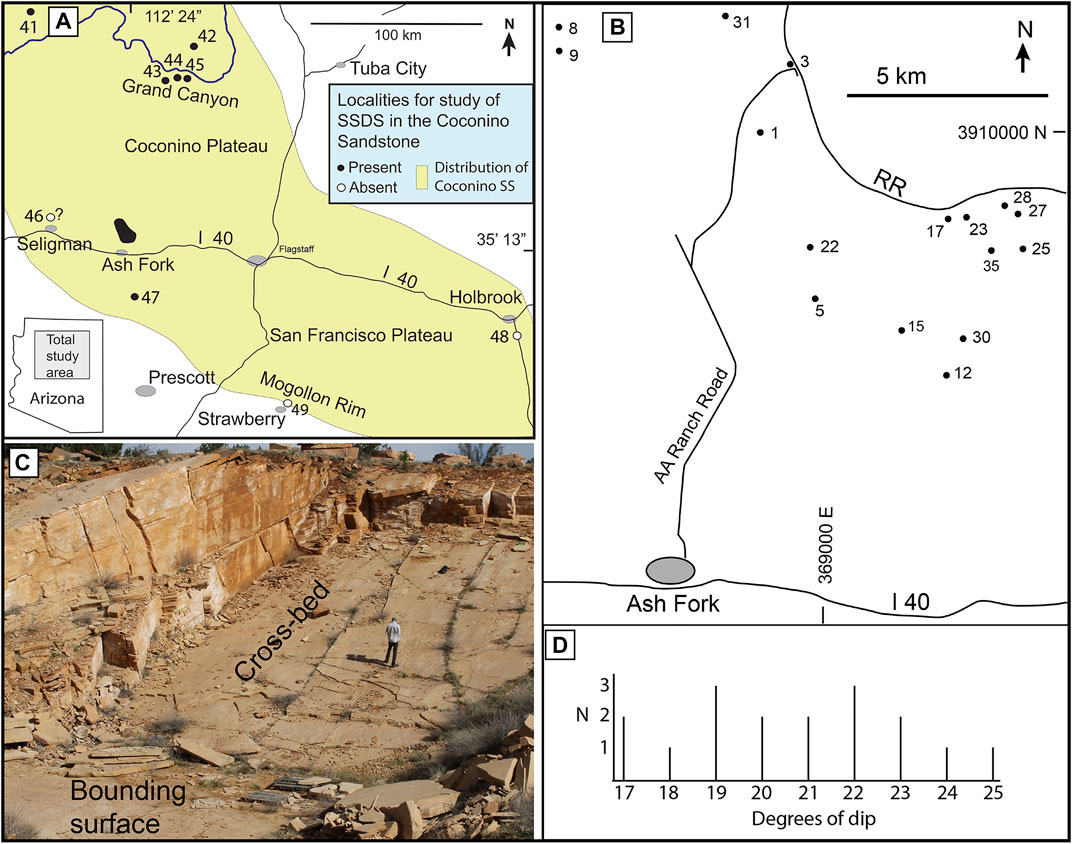
FIGURE 1. (A) Map of the study region, showing locations where we searched for SSD, and which locations had SSDSs. The Coconino Sandstone extends for short distances beyond the area shown on this map. Grey ovals represent towns. Numbered study sites: 41 – 4.5 km N of Tuweep; 42 - North Kaibab Trail; 43 - Hermit Trail; 44 - Bright Angel Trail; 45 – New Hance Trail; 46 - 4 quarries, 5 km N of Seligman; 47 - Matterhorn; 48 - 5 Mile Wash, 8 km south of Holbrook; 49 – 2 km N of Strawberry. (B) Map of quarries near Ash Fork, with numbered quarries. (C) A typical Coconino SS quarry near Ash Fork, Arizona. Height of person = 1.8m (6 ft). (D) Graph showing number (N) of quarry cross-bed surfaces with each degree of dip.
On a larger scale, the Coconino SS is part of the Colorado Plateau, which covers northern Arizona and large parts of Utah, New Mexico and western Colorado. Its tectonic setting is defined by this plateau, which has been stable and only slightly deformed, particularly during the Paleozoic. The formation is surrounded on all sides by regions with much more faulting and deformation, beginning at least in the Mesozoic (Davis and Bump 2009).
McKee (1934) was the first to propose and develop an eolian depositional model for the Coconino SS. Subsequent studies followed this work (e.g., Reiche 1938; McKee 1945; Fisher 1961; Sorauf 1962; Lundy 1973; Elcock 1993; Sumner 1999; Maithel et al., 2021), focused on stratigraphic relationships (Blakey and Middleton 1983; Blakey 1990), tetrapod trackways (Brand 1979, 1992; Brand and Tang 1991; Lockley and Hunt 1995; Brand and Kramer 1996; Millhouse 2009; Citton et al., 2012), or sedimentary properties (Maithel et al., 2019, 2021). Similarly to McKee (1934), most authors interpret the formation as an eolian dune deposit (Reiche 1938; Baars 1961; Mckee 1974; Blakey and Middleton 1983; Loope 1984; Blakey 1988; Middleton et al., 2003).
The small-scale SSD described in this paper are common in the Coconino SS, but have been almost entirely overlooked in previous studies. This research aimed to characterize these previously undocumented SSDSs, and to interpret processes and triggers involved in their formation. Understanding fine-scale processes associated with these structures is significant because it may enable refinement of larger-scale paleoenvironmental models for cross-bedded eolian sandstones.
Materials and Methods
We examined Coconino SS outcrops across northern Arizona: along the North and South Rims of the Grand Canyon, near Holbrook, at the Mogollon Rim, and in 25 flagstone quarries near the towns of Seligman (n = 4) and Ash Fork (n = 21; Figure 1). SSD was most readily studied in the flagstone quarries because of the well-exposed cross-bedding and bounding surfaces.
To determine the three dimensional distribution of the SSDSs we photographed them on cross-bedding planes and in vertical quarry walls, and documented their locations and elevation with a handheld GPS (Garmin and a CHC Model X900). The orientation of each soft-sediment structure (SSDS) was determined with a Brunton compass and recorded with photographs. To characterize the surface features and internal structure of the SSDSs we collected 53 samples from 21 quarries. From these samples, for this study and associated research, we produced 61 thin sections and 14 polished slabs for detailed study of the structures. At each quarry, we measured the strike and dip of the cross-beds with a Brunton compass (n = 21). These data allowed the determination of any correlation between SSD and cross-bed dip. Regional dip of the Coconino SS in our study area was measured at Chino Wash, 5 km north of Seligman, using an Abney level.
Quarry 25 at Ash Fork had numerous SSDSs. We measured the length of each linear SSDS (n = 25) or linear segments of a complex SSDS (separated by distinct breaks) (n = 39), and determined its directional orientation with a Brunton compass. Thicknesses and distribution of SSDSs were documented in vertical quarry walls, to evaluate the relationship between deformed and undeformed layers. Thirteen ridges and folds were described in cross-sectional view in the vertical quarry walls.
Clay mineral composition and distribution were documented to evaluate whether clays controlled the nature and occurence of SSD. Clays were described in whole rock samples (Tescan/Vega/LSH scanning electron microscope; SEM) and in thin section (Olympus BX51 petrographic microscope). Thermo energy-dispersive spectrometry (EDS) and x-ray diffraction (Bruker D8) were also used to characterize clay mineralogy.
FIJI distribution of ImageJ software was used to quantify porosity from thin sections, as a percentage of the intergranular space in a sample (Schindelin et al., 2012; Schneider et al., 2012; Collins 2018). In this study limited porosity data were used; we collected porosity data from both deformed and undeformed portions of one sample, to explore the role of porosity as an indication of liquidization.
Results
All structures described in this study were observed in sets of cross-bedded sandstone, and texture from the Seligman and Ash Fork area was uniform. Grains averaged ∼2.8–2.9 φ in size and were moderately sorted (excluding suspended fines, which were rinsed out in this study (Maithel et al., 2021). Seventeen out of the 21 Ash Fork quarries examined contain SSDSs. In these 17 quarries, cross-bed dips range from 17 to 25° (n = 17), with a mean of 20°. Most dips were toward the south. Regional dip of the Coconino SS (the upper first-order bounding surface) was measured at Seligman as 1.5° to the east. SSDSs were also observed near Tuweep and along the North Kaibab, Hermit, Bright Angel, and New Hance trails in Grand Canyon National Park, and at a quarry 23 km (15 mi) SE of Ash Fork (Figure 1A).
XRD data indicate a small percentage of kaolinite (estimated at < 1%; likely from feldspar dissolution), which was uniformly scattered throughout the thin-sectioned samples. No concentrations of kaolinite were seen in any of the samples. In contrast, concentrated clay drape laminae composed primarily of illite with a minor amount of kaolinite were observed, on the sloping surfaces of foreset beds, with an average slope of 20° (Figure 2). Figure 2B is a representative measured section on a vertical quarry surface. Thickness of sandstone layers between clay laminae ranged from 2.3 cm to over a meter. Analysis of clay was part of a separate study, and additional data on clay and its detailed distribution will be published elsewhere.
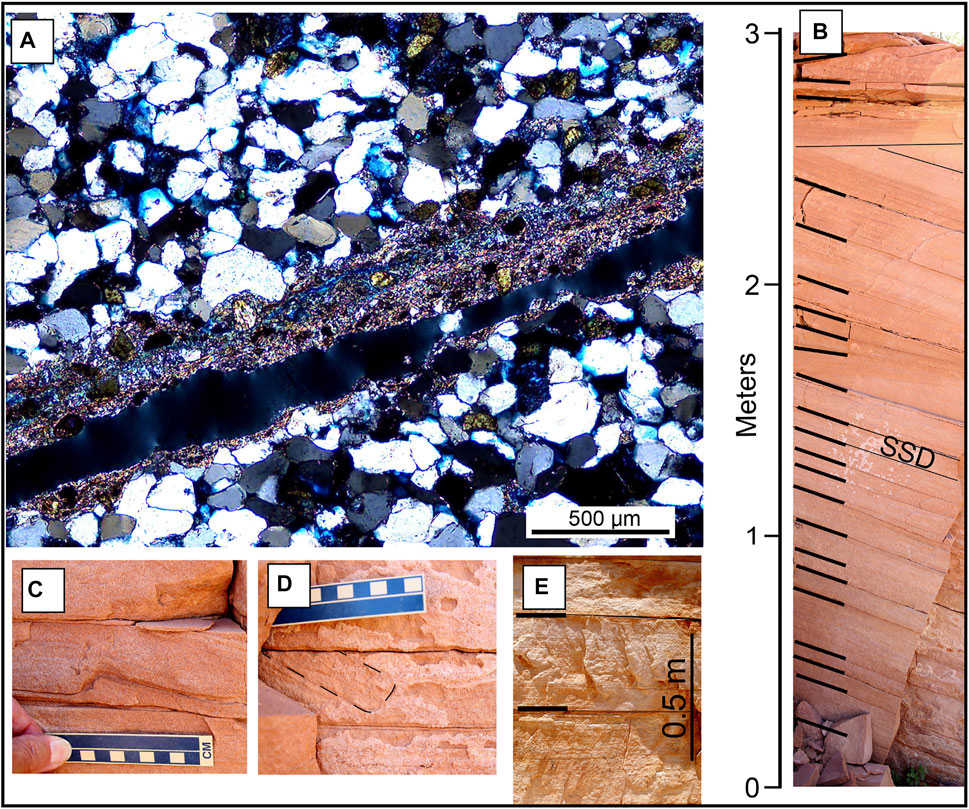
FIGURE 2. (A) Thin section showing a clay drape lamina along a dipping (∼20°dip) cross-bed surface, separating sandstone laminae below and above the clay drape. The black line shows where the bedding broke along the middle of the clay drape lamina. Up is toward the top of the photo. (B) A representative measured section on a vertical quarry surface. Thin lines outline laminae contacts; heavy lines are probable clay laminae, based on typical correlation between clay and cracks between laminae (C,D) SSDSs in the middle laminae in (B). (E) Thicker sandstone layers from another quarry. Thick lines are probable clay laminae.
We found SSD on numerous cross-bedding planes, but did not see any on the 43 bounding surfaces that we examined, with two minor exceptions described later. As defined by Kocurek (1981) these were second-order bounding surfaces.
The SSDSs fall into several distinct categories. 1) Centimeter-scale folds and ridges on cross-bedding planes. In some cases these were associated with small rotated blocks of sandstone within the cross-beds. 2) Larger monoclinal folds. These are quite different in structure, and occur on about a meter scale, rather than a centimeter scale. 3) Conical pits on the cross-bed surfaces. These are associated with other SSD features. 4) One case of convoluted bedding was found, which is different from the other structures. Evidence for liquidization in these structures was evaluated. The numbers of each type of SSDS are given in Table 1, and each will now be described individually.
Folds and Ridges
Some SSDSs consist of low-amplitude folds or sharp ridges on individual cross-beds whose surfaces are otherwise undeformed. SSDSs of this type are common in the studied quarries and in some outcrops they are especially abundant. These all occur on the surface of dipping cross-beds (bedding planes).
The folds and ridges are 2–10 cm wide and range from 20 cm to 10 m long (Figure 3).
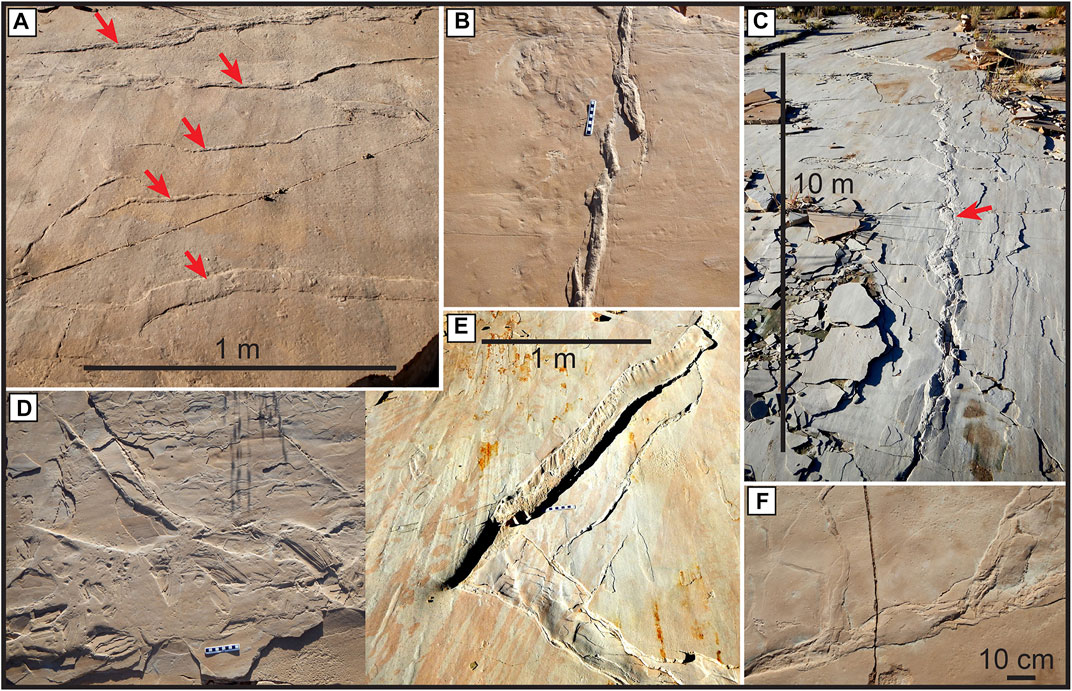
FIGURE 3. Surface view of representative SSDSs on cross-bed surfaces. (A) This structure contains five parallel folds or ridges, marked by arrows. (B) A single ridge, broken into several en echelon sections, with a featureless disturbance along the left side of the ridge. (C) A fold that is more than 10 m long, marked by an arrow. (D–F) Small sections of complex folds. The structure in (D) is wider than most, and includes several rotated blocks. Cross-beds dip toward the bottom of each photo. Scale bars in (B,D) are 10 cm long.
Figure 4 shows cut and polished sections of sandstone samples, with cross-sectional views of the types of SSDSs in Figure 3. The deformed sand forms either a sharp ridge (Figure 3B; Figures 4B,D; middle ridges in Figure 3A), or a wider, less elevated structure (lower feature in Figure 3A; Figures 3D–F). In cross sections through the ridges, laminations are tilted up sharply for a cm or more (Figure 4B; Figures 5B1,B2). Deformed laminae in the upper first few cm below the surface follow the shapes of the surface features (Figures 4, 5). Undeformed laminae were observed in the underlying beds, usually 2 to 3 cm below each SSDS, but in some cases, they first appear up to 8 cm below.
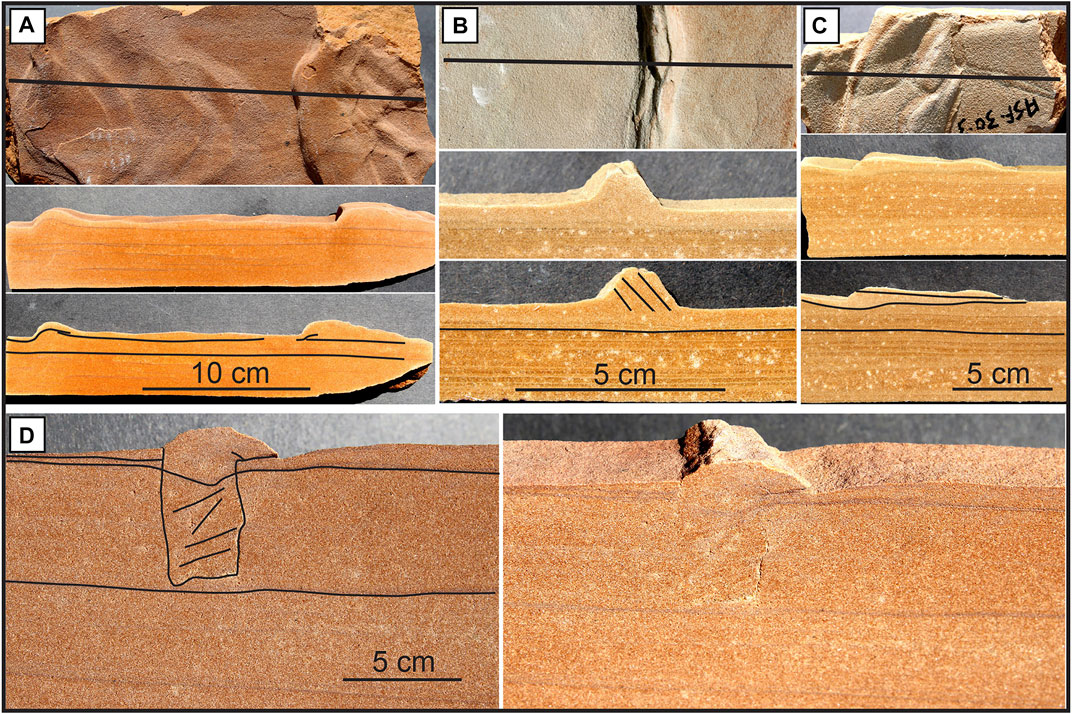
FIGURE 4. Cross-sectional views of SSDSs in sandstone samples. For each example, one photo is unmarked, and the other has line drawings to highlight internal laminations. (A–D) The upper photos are bedding plane surface views; the black lines indicate the transects along which the cross-sections were cut. (D) A section of sandstone below the ridge appears to have been shifted slightly (visible in left photo).
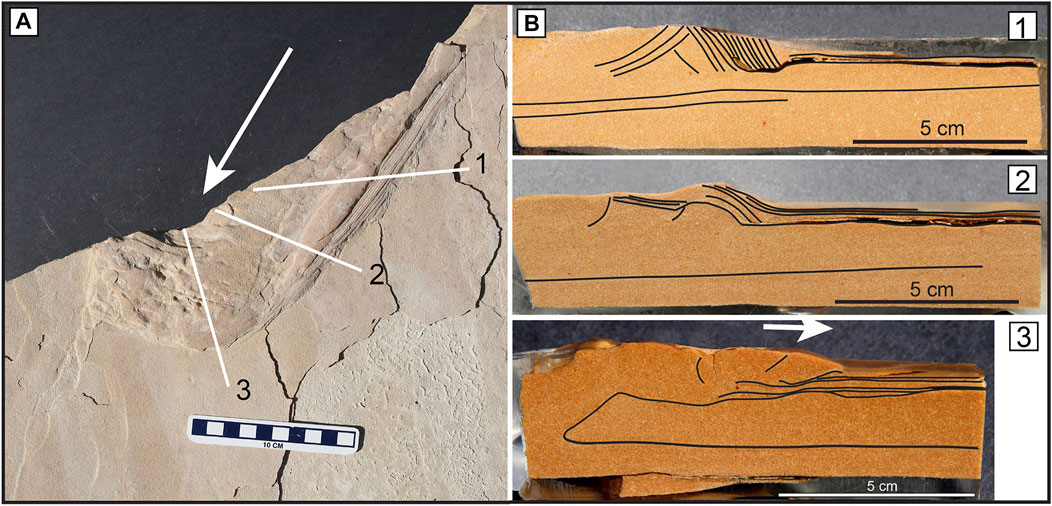
FIGURE 5. A SSDS with cross-sectional views along three transects, as shown by the white lines in the left photo. Figures B1‐B3 are transects along white lines 1‐3 in Figure 5A. Laminae have been highlighted for clarity in the vertical sections B1‐B3, and white arrows show the apparent direction of sand movement during deformation. Scale bar in the left image is 10 cm long.
A ridge occurs along the top of the slab shown in Figure 4D, and a rectangular section of sandstone below that ridge appears to have shifted slightly, tilting to one side, while only producing a slight depression of the horizontal lamination below it. This tilting of a block below the ridge was not seen in any other section.
The SSDSs in Figure 5A display another unique feature, in which sand extends over the top of the adjacent surface. Sediment appears to have moved in the direction shown by the arrows, and the subsurface structure is seen in cross section in Figure 5B; Figures 5B1,B2 show cross sections along lines 1 and 2 in Figure 5A. At the forward end of the deformation, the sand appears to have flowed over the top of the original surface (Figure 5B3). Along the side of the deformation is a ridge, containing sand laminae tilted up sharply (Figures 5B1,B2).
Most of these SSDSs are constrained to individual beds (Figures 3–5). Ridges and folds seen on vertical quarry walls were 3–8 cm thick, but some thicker examples wee documented. In all cases the layers overlying and underlying these deformed layers were undeformed. Figure 6 shows a fold about 10 cm thick that could be described as an overthrust over the adjacent sandstone layers (toward the left in the photograph). The top of the fold has been truncated by the overlying bed.
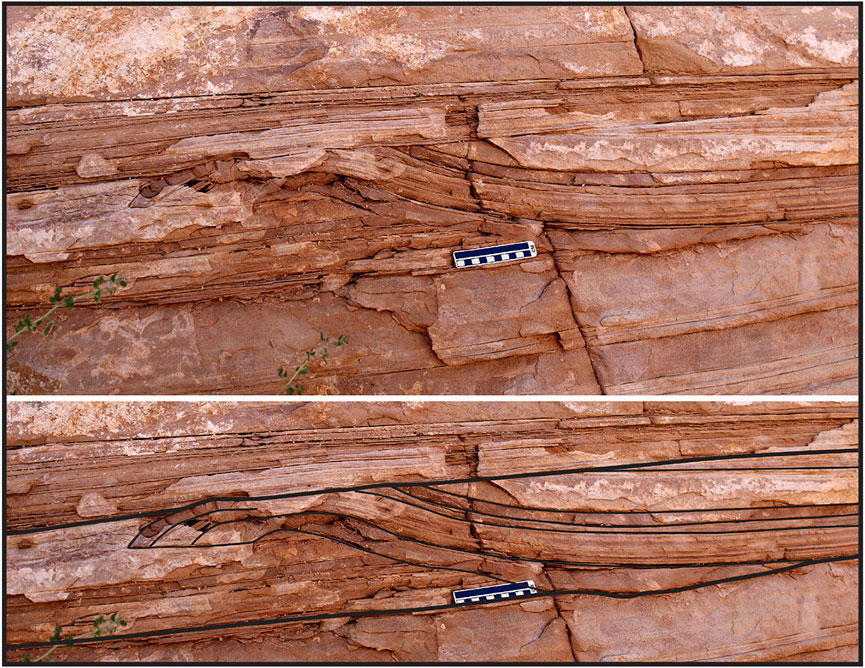
FIGURE 6. Cross-sectional view of an overthrust fold as seen in a vertical quarry wall; the upper part of this folded feature has been truncated by the overlying sandstone layer. The top photo is unmarked and the bottom photo is annotated to highlight the internal contorted laminations.
The shapes of these folds and ridges on bedding planes vary from approximately straight (Figure 3C) to complex in shape, with sharp changes in orientation at irregular intervals (Figures 3D–F, 7A).
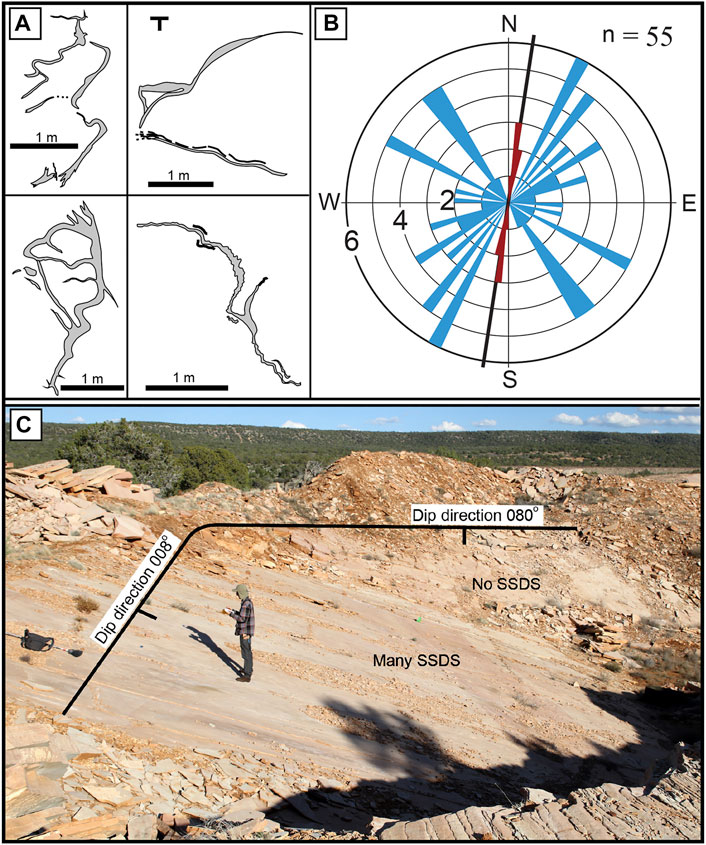
FIGURE 7. (A) Examples of complex folds on Coconino Sandstone foreset beds, drawn in plan view. The structures in these drawings are raised folds or ridges (also shown in Figure 3). Cross-beds dip 18–25° toward the bottom of each image. (B) Fold-axis orientation of deformation structures from Ash Fork quarry 25 (also pictured in Figure 8). Blue: SSD ≤2.5 m long. Red: SSD 3.5–10 m long. The black line represents the cross-bed dip direction, toward the bottom of the diagram. n = 55 simple folds or segments of 11 complex folds. (C) Sharply curved cross-bed surface at Ash Fork quarry 25. SSDSs are abundant on the left side (8° dip direction; 196 sq m of surface), but absent on the right side (80°dip direction; 35 sq m of surface). Height of person = 1.8 m (6 ft).
The unusual cross-bed surface in quarry 25 curves sharply along strike, with relatively straight “arms” (008° and 080° dip directions) and an almost perpendicular angle between the two surfaces (Figure 7C). The surface with the 008° dip direction has many SSDSs like those pictured in Figures 3, 7A, whereas the surface dipping at 080° exhibits none of these features.
At this quarry the SSDSs are sufficiently abundant to facilitate more detailed, quantitative comparisons of the folds. The orientation of straight folds or ridges, (n = 25), or segments of complex folds (n = 39) is shown in Figure 7B. Short folds or fold segments (less than 2.5 m in length) are oriented in diverse directions (Figure 7B), with very few along strike or dip, while the longer folds (3.5–10 m in length and mostly straight) are consistently oriented parallel to the dip of the cross-beds (approximately north-south; Figure 7B). In other quarries, the long folds or ridges (≥3.5 m) are also oriented approximately along the cross-bed dip direction, toward the south.
Rotated Blocks
Small, intraformational blocks of sandstone have rotated with respect to the undeformed cross-bedding surrounding them (Figure 8). Isolated examples occur, but clusters of these structures are common in some outcrops (18 clusters of these blocks were documented in various quarries). Where the upper edge of a tilted block meets the top of the bed, it is usually truncated at this level. The upper part of Figure 8D shows the typical appearance of the top surface of truncated blocks. In each case, the rotated blocks consist of fine-grained sandstone of the same texture as the surrounding bed. These blocks range from ∼3–20 cm long in cross section, and on bedding planes they measure 5–15 cm wide (Figure 8D). The rotated blocks do not usually have sharp edges, but they have a definite, recognizable outline, and they generally maintain their laminated internal structure.
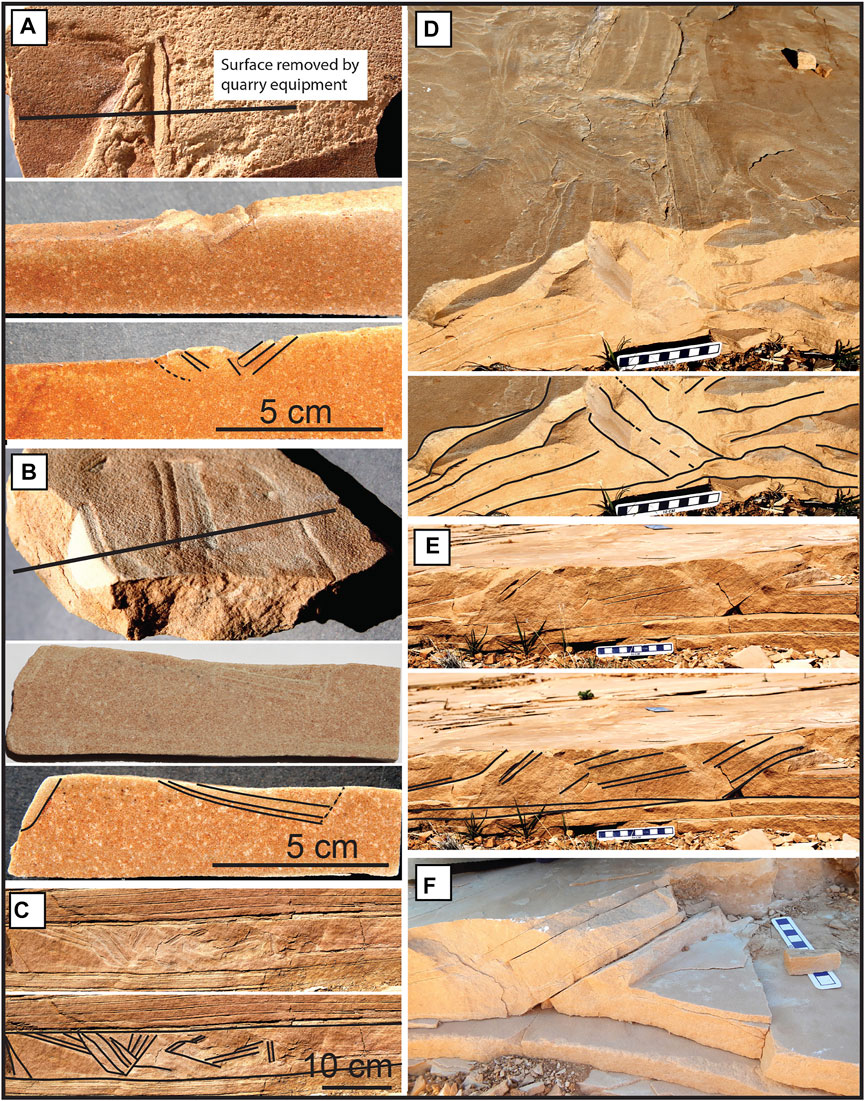
FIGURE 8. Cross-sectional views of four SSDSs, with rotated blocks. These are shown with and without lines annotating the rotated portions (A–D) Surface views of the structures, with heavy black lines in (A,B) indicating the transect orientations (D–F) Different views of a single SSDS. (D) shows the surface of the rotated blocks in (E,F), and (F) is a close-up of the right portion of (E). Scale bars in (D–F) are 10 cm long.
The rotated blocks do not tilt in a uniform vertical direction. While most blocks in Figure 8D have the same orientation, in Figures 8A–C, individual blocks tilt at different angles. Many more rotated blocks like those pictured here were observed, and they tilt at a wide range of angles, including almost vertical (perpendicular to the surrounding cross-bed; Figure 8C, in part). The orientations of rotated blocks vary widely, with most tilting upward to the north-north east, which is up dip with respect to the cross-beds (Figure 9A).
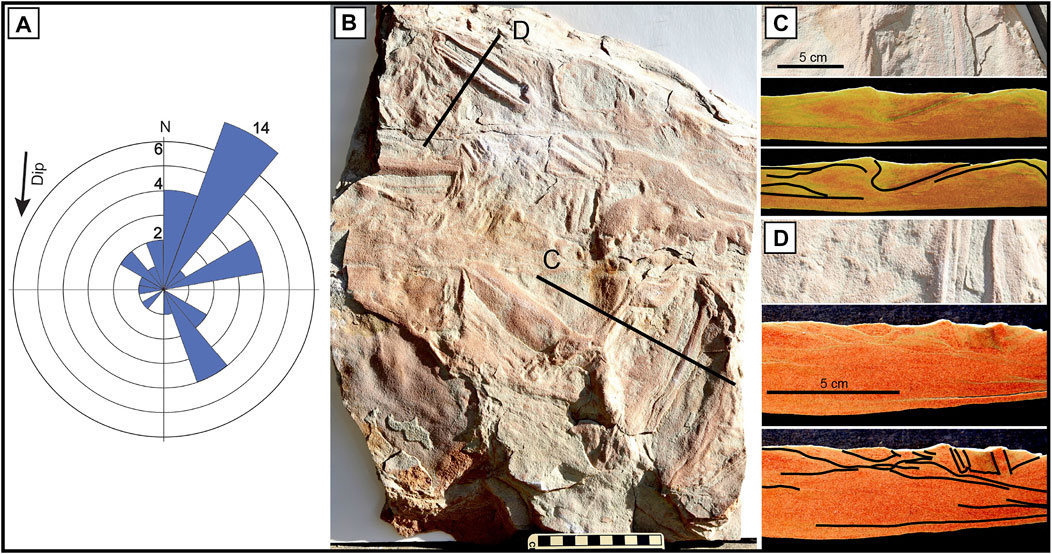
FIGURE 9. (A) is a rose diagram showing the compass directions of the upper edge of rotated blocks. Arrow indicates the average dip direction of the cross-beds. (B–D) is a sample with several rotated blocks and deformation features. Photo (B) is a surface view of the structures, with lines indicating the locations of the cross-sections in photos (C,D). This sample does not show the distinct horizontal truncation that is evident at the top surface in other samples of rotated blocks.
Figure 9 shows an additional sample with rotated blocks (Figure 9D) and other deformation features including contorted bedding (Figure 9C). In this sample, the top surfaces of the rotated blocks and other deformations were not truncated along the top of the cross-bed, but exhibit up to 0.7 cm of relief above the upper surface of the bed.
Larger Monoclinal Folds
Two larger SSDSs occur in the form of simple monoclinal folds, which differ from the previously described folds and ridges in size and form. These are meter-scale features rather than centimeter-scale, and are not just a localized deformation on an otherwise undeformed bedding surface; rather they each include a series of layers in a bed 0.5 to 1 m thick, that are folded into a monocline (Figure 10, lower part). They are unique among our findings, and are not part of a continuum of related structures. In one of these folds (Figure 10A), the deformation affects at least one vertical meter of sandstone, and it is folded 40 cm upward toward the right side of the photograph. This horizon is located stratigraphically near the top of the Coconino SS, at the top of the uppermost set of cross-beds in the section. Only 1 m of flat bedded sandstone occurs between this cross-bed set and the overlying Toroweap Formation. The axis of the fold is north west—south east, and the uplifted side is toward the north east.
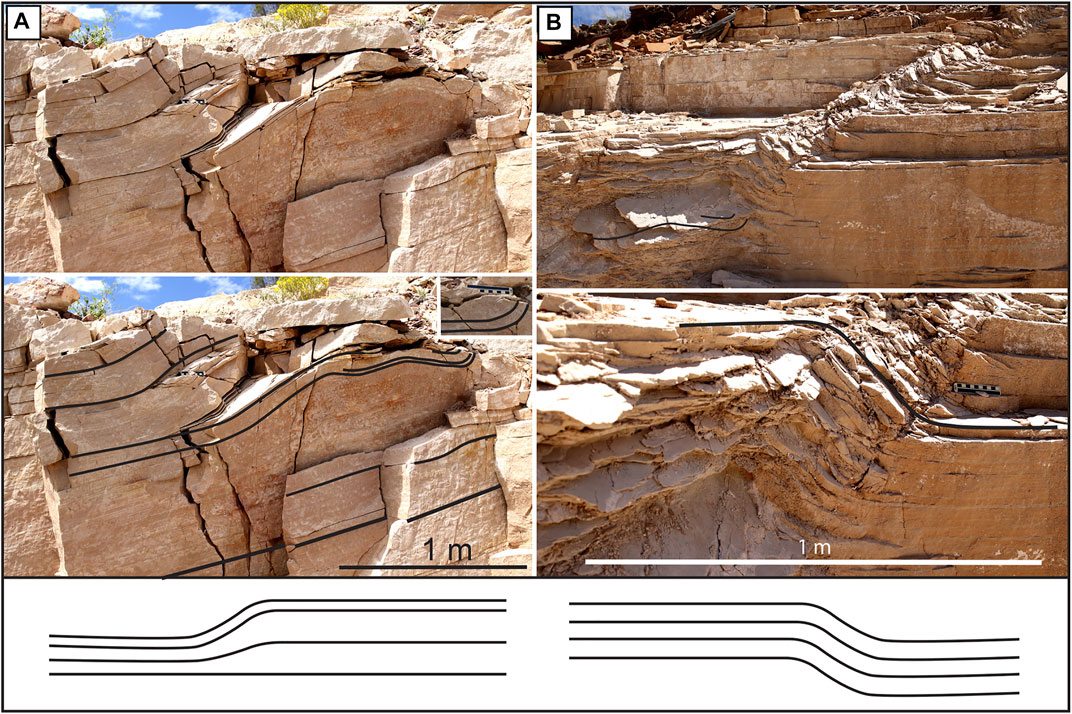
FIGURE 10. Examples of monoclinal folds in an Ash Fork quarry. (A) Deformed bedding 1 m below the contact between the Coconino Sandstone and the overlying Toroweap Formation. (B) Deformation 44 m below the Toroweap, in the same large quarry, which had multiple exposed cross-bed sets and bounding surfaces. Lower illustrations are diagrammatic cross-sections of the structures.
The second monoclinal fold (Figure 10B) is even larger and more complex. The folded unit spans a thickness of over 0.5 m, with all of these laminae exhibiting 23 cm of vertical relief, lifted up toward the left in the photo (Figure 10B, bottom photo). The fold is not limited to just one stratigraphic level, but rises upward through at least 1.2 vertical meters of successive laminae, and is spread across ∼3 m of horizontal distance (Figure 10B). The deformation likely extended much farther horizontally, but was removed by quarrying operations. This fold occurs about 44 m below the contact with the Toroweap Formation, in the same quarry as the fold shown in Figure 10A. At least part of the fold (Figure 10B) may exhibit brittle deformation, in contrast to the fold in Figure 10A, which appears to exclusively represent ductile deformation. The axis of the fold in Figure 10B is approximately east—west, and the uplift is toward the north. The fold is stratigraphically higher at the east end, sloping down to the west.
Conical Pits
Small, conical pits up to 3.5 cm in diameter were observed within 3–6 cm of some SSDSs (14 total groups of pits in various quarries) (Figure 11). They usually occur in straight rows along one side of an SSDS as seen in Figures 11C,D,F,G. Individual pits vary in width from 1 to 3.5 cm. The cross-bed shown in Figures 11F,G is 1.8 cm thick, and the pit extends vertically through at least the 1.8 cm bed (Figure 11G), and appears to continue below that.
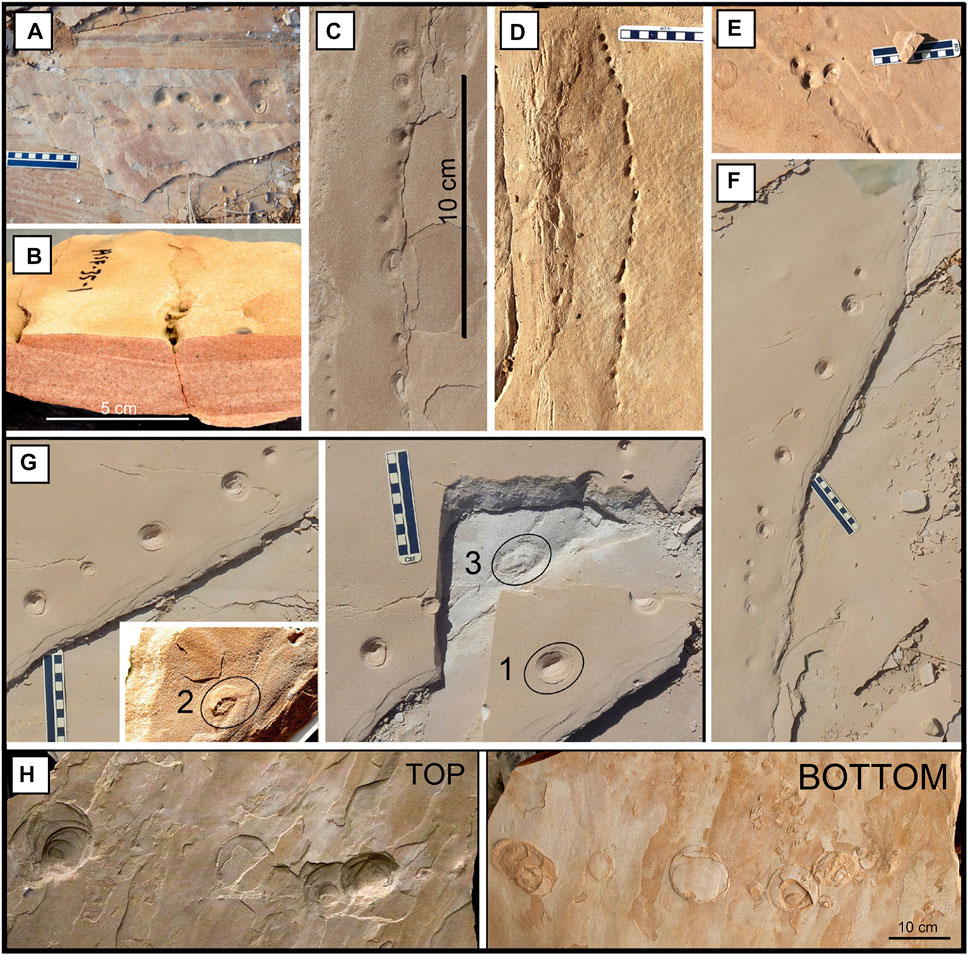
FIGURE 11. Conical pits associated with SSDSs. (A) One of two groups of pits seen on a bounding surface. All others (C–G) occur within or near SSDSs on cross-bedding planes. (B) A cross-section through a row of pits, with a localized crack leading vertically up to the pits. (F,G) These two photos are views of one group of pits, and show the successive surfaces which are part of the same structure. The plan view of one pit has been circled and annotated with a “1.” The same pit is visible on the bottom of a broken piece from that same bed, 2) and even continues into the underlying layer 3). (H) Structures similar to the above pits, but much larger. The right photograph has been reflected, horizontally, to facilitate comparison of the two sides of the slab, which is 5 cm thick. Cross-beds are dipping toward the bottom of the photos. Scale bars are in cm.
Figure 11B shows a row of pits with a localized crack underneath; the crack extends vertically through the 3.5 cm-thick sample, up to the bottom of the pits. This crack does not appear to have formed from quarrying processes, but is closely associated with the pits and was likely an original feature.
Another slab has features that are similar to the pits, but are much larger: about 10 cm in diameter (Figure 11H). This loose sample was collected from a quarry at a location called the Matterhorn, 23 km (15 mi) southeast of Ash Fork.
Convoluted Bedding
In an outcrop 4.5 km (2.8 mi) north of the Tuweep ranger station (north of the Grand Canyon), a zone of convoluted cross-bedding about 0.5 m thick occurs (Figure 12). Laminae appear intact, but are folded, and the deformation is constrained between the strata above (sharp contact) and below (gradual contact).
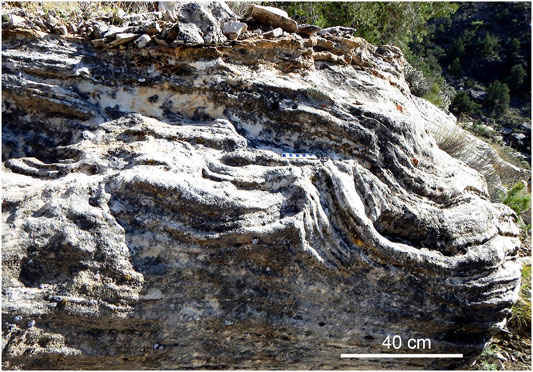
FIGURE 12. Contorted bedding observed near the Tuweep ranger station. The deformation zone here is thicker than most of the others described in this paper.
Deformed Sediment
A cross-section through one of our Coconino SS slabs (float) exhibits distinctly visible laminations for most of its length, but toward one side, the laminae appear shifted, distorted, and lifted upward (Figure 13). Porosity was measured as 8.74% in the deformed (left) side of this sample, compared to 12.85% on the right, which is a porosity difference of 32%.
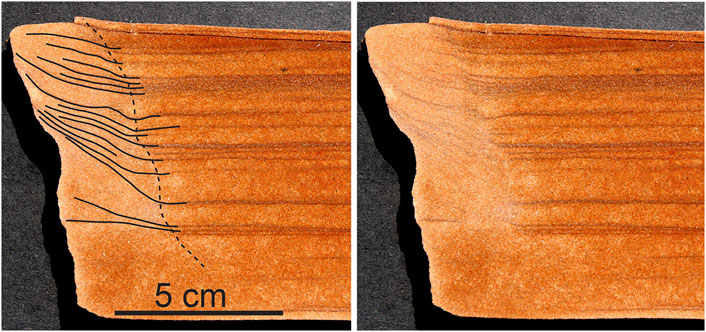
FIGURE 13. Deformed laminae on the left edge of a Coconino Sandstone float sample, with lower porosity in the deformed area. Deformed laminae are highlighted in left photo.
Geographical and Stratigraphical Distribution of SSD
SSD is common in Coconino SS outcrops at the Grand Canyon and in quarries near Ash Fork. We did not find any of these features in the southern Coconino SS exposures along the Mogollon Rim, or farther east near Holbrook. We note that good outcrops in those areas are much less abundant (especially in the Mogollon Rim area), leaving some uncertainty about whether SSD occurs there.
On a more local scale, the quarries near Ash Fork cover over 43 square kilometers (16.6 sq. miles). SSDSs are common over most of this area (Figure 1), observed in 17 of 21 quarries.
Vertical distribution of SSD within a single outcrop could only be assessed in the quarries near Ash Fork, where structures are widely distributed. The Ash Fork quarries expose sections from the upper Coconino SS contact to the lower portion of the formation. While the basal contact is not exposed in this area, at least 160 m of stratigraphic distance separates the upper and lowermost quarries. SSDSs occur at irregular intervals throughout this vertical distance, which approximates their stratigraphical position (Figure 14A).
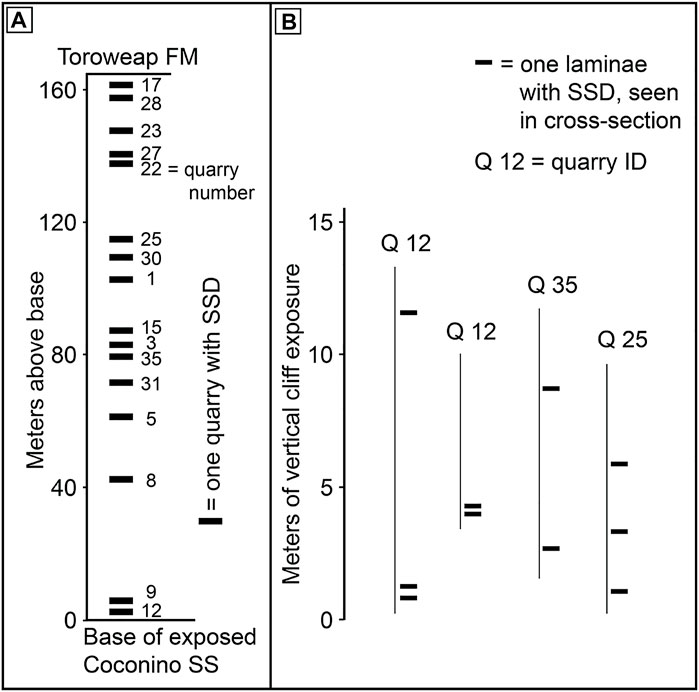
FIGURE 14. (A) Stratigraphic distribution of quarries near Ash Fork containing ridges, folds, and/or rotated blocks, based on elevation. (B) Vertical distribution of SSD zones as seen in three quarries.
Local stratigraphic distribution of SSDSs as seen in vertical walls exposed within a quarry (Figure 14B) varies widely. Vertical distance between deformed zones range from 10 cm to 12 m (as measured in the three quarries in which these measurements could be taken). However, the measurements probably underestimate the stratigraphic frequency of SSD, since the deformation usually only covers a small part of the sloping upper surface of each bedding plane. Consequently, when cross-beds are exposed in a vertical section, it is likely that many SSDSs present on some beds would not be visible in any given wall. Thus, the stratigraphic distances between beds with soft-sediment deformation structures are probably smaller than we were able to measure.
Disscussion
Most of these SSDSs, excluding the monoclinal folds and convoluted bedding, occur as shallow deformations in the otherwise extensive, undeformed cross-beds. In contrast, the majority of sandstone deformation literature describes much larger-scale structures than we observed (meters or tens of meters, versus centimeters in depth). The SSDSs we observed also only occur on the surfaces of these cross-beds, and this has implications for the associated deformation processes. To interpret these Coconino SS soft-sediment deformation structures, we will discuss potential, 1) deformation processes, and 2) triggers.
Conditions for Deformation
Conical Pits as Water Escape Structures
The conical pits appear to be the same features previously interpreted as ant lion pit traps by Elliott and Bartlett (2013), but we suggest—at least in the examples described here—that their origin was related to the soft-sediment deformation processes, since they always occur within or in close proximity to SSDSs. They resemble, on a very small scale, pits made by sand volcanoes and related fluid escape (Lowe 1975; Lowe 1976; Owen 1995, 1996; Owen and Moretti 2011). In some cases (Figure 11), we see evidence of fluid escape that extends from beneath the pits—through several cm of sandstone and up to the bedding plane where the pits are exposed (Figures 11B,G)—suggesting that these are not surface features. Evidence of liquidization or slumping along these paths is not apparent. They seem to have involved saturated sand, but with enough cohesion to maintain their integrity when fluid escape occurred. The pits in Figure 11H also look like fluid escape structures, but on a larger scale. We suggest that all of these pits, including the structures in Figure 11H, were the result of water or air escape from recently deposited sand.
Liquidization
The formation of SSD involves liquidization of sediment, as liquefaction or fluidization (Owen and Moretti 2011; Lowe 1976; Nichols 1995; Owen 1996). Rotated blocks and other SSDSs in the Coconino SS appear to have required some type of liquidization to displace or deform the sediment. The reduced porosity associated with deformation in one slab (Figure 13) provides evidence for fluidization: bedding on the left side of that sample is elevated as might occur during this process (Lowe 1976). Portions of the sample in Figures 6, 8, 9C,D also appear to be massive, structureless sandstone, which may suggest fluidization. However, this “massive” classification is not certain, since laminae are sometimes difficult to detect in the Coconino SS.
Evidence for liquidization is common in the SSD at many intervals in the Coconino SS, and the pits as probable water-escape structures indicate that a significant amount of water was present to liquefy the sand, at least at scattered intervals in time and space. If the structures were approximately synsedimentary, the sand must have contained enough water at the time of deposition to account for these processes. In most cases, the episodes of liquidization were likely quite localized, as suggested by the infrequent, scattered evidence for their presence. Experimental study would be beneficial for further characterizing the nature of these processes, and to explain why the deformation is not more pervasive.
Liquidization might be expected to occur mainly in the troughs of large bedforms, where the water table is closer to the surface. However, the SSDSs in the Coconino SS are not in troughs of large bedforms; rather they are spread across the sloping surfaces of foreset beds, with no evidence for a water table.
The Influence of Clay
The illite laminae observed between some cross-beds (Figure 1B) allowed the sandstone to split cleanly into flat slabs, revealing the SSDSs. Without this, it would have been difficult or impossible to find the SSDSs in the well-cemented Coconino SS. It is possible that the lack of cohesion along clay laminae allowed movement in cases of intrastratal SSD. In examples where SSD did not form intrastratally, the clay could have still been a factor, but the mechanism is not clear. Additional study is needed to explore these questions.
Deformation Processes
Most of the raised folds, ridges, and rotated blocks were truncated by the processes that deposited the overlying cross-beds (Figure 8), suggesting that they were likely synsedimentary. The surfaces of other SSDSs were not truncated (Figures 4, 5, 9B), but the shapes of their surface features and the persistence of their shapes for several centimeters below the surface still indicate ductile deformation. While most of the SSDSs are only a few cm deep, the monoclinal fold in Figure 10A implies that up to a meter or more of sand was unlithified at the time of deformation. The SSDSs generally do not suggest evidence for extensive cementation before they formed, but in a few cases, deformation may have been preceded by minor cementation. The small folds in Figure 8D, the larger monoclinal fold in 10B, and some of the rotated block examples are features of this type. Some of the ridges contain distinct, upward-tilted laminae (Figures 4B, 5B). These features do not generally have sharp edges, as might be expected if they represented completely brittle deformations. However, they may have involved some early lithification, since the blocks remained intact while deformation processes were occurring. The implications of this depend on how rapidly early lithification could have occurred in this sandstone. Liquidization must have been involved in producing these structures, but the sand appears to have had sufficient cohesion to maintain laminae continuity.
Three other formations contain structures that may compare to our rotated blocks, raising the question of whether they formed by similar processes. Rotated blocks in the Late Cretaceous Rio Paraná Formation in Brazil have a preferred orientation along the cross-bed, and Fernandes et al. (2007) interpreted them as landslide deformational features or collapse breccias. Shallow, brecciated zones occur in the Navajo Sandstone, with clasts derived from underlying foreset beds (Middleton and Blakey 1983). These authors interpreted the clasts as having been ripped up and transported down the foreset by the avalanching of wet sand. In the gypsum sand dunes of White Sands National Monument, New Mexico, McKee and colleagues describe small blocks that moved downslope and rotated (McKee et al., 1971). The lower parts of these blocks are tilted upward, as might be expected in a slump feature. In all three of the above examples, the blocks —which are quite different from those in the Coconino SS (Figures 8, 9B,C)—were displaced by gravity-driven processes.
Liquidization and Pore Water Pressure
The evidence for liquidization in the Coconino SS played an essential role in generating SSD (Owen et al., 2011; Owen and Moretti 2011). Another parameter associated with liquidization is the upward movement of trapped pore water producing excess pore pressure (Mills 1983; Owen 1995; Obermeier 1996). The presence of interpreted water-escape structures (pits) in association with the Coconino Sandstone SSDSs is consistent with this process model. We suggest that the pore water pressure could have provided the shear stress necessary to deform the sand, if a trigger initiated shaking or vibration of the sediment.
For the small folds and ridges (Figures 3, 4, 7A) and small-scale overthrusting (Figures 4A, 5A, 6), lateral shearing from pore pressure may have pushed the sand into these raised ridges or folds. We suggest that localized pore pressure also pushed pockets of sand upward to displace the rotated blocks (Figures 8, 9). The direction of this movement appears to have been inconsistent, even within a square meter on the bedding plane, which may suggest a lack of trigger directionality. However, the blocks were most commonly tilted up dip within the cross-beds (Figure 9A).
A paper by van Loon and Brodzikowski (1987) includes a photograph of raised ridges in Tunisia, apparently slightly lithified, which are very similar in appearance to those in the Coconino SS. Their proposed process is entirely different, however, as the horizontal Tunisia ridges are thought to have been upthrust at the contacts between salt plates in a sabkha. One possible similarity might involve internal stresses within the Coconino SS that exerted lateral forces to push up the ridges ahead of them, or to converge from two directions and push up the sediment between them.
The larger monoclinal folds likely required a stronger process of local uplift, perhaps from more significant pore-water pressure or from a direct seismic trigger. The complex fold in Figure 10B dips toward the west, and thus probably involved an uplifting force that was stronger toward the east. This folding would have occurred after sufficient burial to at least cover its upper edge, because the lateral continuity of the fold indicates that all of its laminae were present when the folding occurred. Experimental study of these processes, as done by Moretti et al. (1999), would be instructive.
It appears that some of the SSDSs involved intrastratal deformation. Our samples of raised folds and ridges (Figures 3–5), in contrast to most of the rotated blocks, do not show evidence of erosion along their upper surfaces. They were never observed in cross-sectional view except as seen in Figure 6, so it was usually not possible to evaluate their relationship to the overlying beds. However, it seems likely that exposed, unlithified structures like these ridges and folds would be at least reworked by subsequent sediment transport and deposition. Since the upper surfaces of these folds and ridges lack evidence of lithification, and yet were not eroded, they may have formed intrastratally.
The raised ridge in Figure 4D is unique among our samples. It appears that, after the raised ridge formed, pressure from above may have displaced a discrete block of sand directly below the ridge, to a depth of about 5 cm. This may indicate intrastratal deformation, and subsequent shifting from the weight of overlying sediment.
Deformation on Cross-Bedding Planes
An important characteristic of these SSDSs is that they occur on cross-bed surfaces. They were not observed as clusters buried in the sediment, but rather as long, linear or zigzag features on bedding planes (Figure 1C, 7) These folds and ridges commonly occur in groups on some cross-bed surfaces, but their distribution on layers above or below any given bed cannot usually be determined. The overlying sandstone was removed by erosion or quarrying processes, and the underlying beds are not accessible. The only way to evaluate these relationships is by studying cross-sections in vertical quarry walls, and as far as we could determine, the SSDSs are not uniformly distributed stratigraphically.
In addition to the effects of gravity, the form of these SSDSs was controlled by the sloping foreset surfaces (Figure 1B) and the extensive sloping laminae that underlie them. Some ridges extend up to 10 m along dip on a cross-bed that dips at ∼ 18° (for example Figure 3C). The Coconino SS in this area has almost no regional dip, so the 18° slope reflects depositional conditions. Because of their significant persistence and uniformity along the slope the processes and conditions that produced these ridges appear to have persisted for a substantial along-slope distance. The uniform characteristics of the original foreset surface may have controlled the movement of pore water and pressure involved in generating SSD. Clay laminae are typically quite thin, but may have influenced the movement of pore water and resulting pore pressure. This part of the deformation process, characteristic of at least some cross-bedded sandstones, requires more research to understand the dynamics of this system.
Possible Triggers for the SSD
The wide variety of SSDSs that occur in sedimentary rocks imply that multiple processes can trigger deformation. Papers on SSD commonly discuss the difficulties and uncertainty in determining deformation triggers (Rodríguez-Pascua et al., 2000; Owen et al., 2011; Owen and Moretti 2011; Shanmugam 2017). This uncertainty also applies to the Coconino SS, but some observations in this study may be helpful.
Coconino Sandstone SSDSs appear to have been triggered by an external influence, not by the processes that deposited the cross-bedding. We suggest this interpretation because SSDS orientation does not generally correlate with cross-bed dip direction.
The size of the deformations probably relates to the magnitude of the triggering force. In the Coconino SS, most of these SSDSs are only several centimeters deep, with a few extending up to a meter deep. In contrast, some SSDSs in other formations can be up to tens of meters thick, which seems to imply a difference in the magnitude of the trigger.
The SSDSs in the Coconino SS are not only shallow, but are also localized; they are usually confined to individual cross-beds. Accordingly, the triggers must have had fairly localized influence to produce these shallow features. Soft-sediment deformation depends not only on the trigger, but also on the rheological properties of the sediment (Mills 1983; Owen 1987; Nichols 1995; Moretti and Ronchi 2011), which may explain the localized distribution of the structures. Liquidization was likely involved in forming these SSDSs, but something must have triggered the liquidization and subsequent deformation. The following sections discuss possible triggers.
Gravity Slumps
It could be suggested that the folds and ridges in Figure 3 are actually slumps. Slumps or slump-like structures are abundant in the Coconino SS, but they are broad features, with their long axes usually oriented along dip (as would be expected to result from a gravity-driven process). In contrast, our SSDSs are rarely oriented along dip (Figures 3, 7B), except for the few long, narrow ridges (Figure 3C), which look very different from slumps. Many of the shorter ridges do not exhibit a preferred orientation, as they are components of larger, somewhat zigzag folds (Figures 3E, 7A,B). For this reason, gravitational slumping as a trigger is considered unlikely, at least for the elongate structures. As previously discussed, the rotated blocks also do not show evidence of being displaced by gravitational slumping.
Climatic Cycles
In the Jurassic Navajo Sandstone, some outcrops exhibit cross-beds in which shallow SSDSs—folds, faults, and slumped blocks of breccia (Loope et al., 2001)—occur, on a scale similar to many of those in the Coconino SS. These beds are relatively more resistant to weathering and repeat at regular intervals. Loope et al. (2001) attributed this deformation to wet periods in annual climatic cycles. The folds and rotated blocks in the Coconino SS do not exhibit a preferred orientation as is seen in the thrust-faulted, slumped sand masses in the Navajo Sandstone, nor are they arranged in any regular, repeating pattern that might imply climate cycles. We therefore suggest that processes which produced these structures did not result from climate-driven moisture changes.
Miscellaneous Processes
Other possible triggers for SSD described in the literature include storms, tsunamis, landslides, glacial loading, volcanic activity, cyclonic waves, and tidal currents (Jones and Omoto 2000; Owen et al., 2011; Owen and Moretti 2011; Shanmugam 2016; Shanmugam 2017). These processes are not likely to be relevant for the SSD in the Coconino SS cross-bedding, considering its continental depositional setting. Meteorite impacts are also an unlikely trigger, since no Permian meteorite strikes have been reported in northern Arizona.
Seismic Events
Seismic events provide a candidate for triggering the SSD in the Coconino SS. Possible evidence for a seismic trigger can be observed in the quarry shown in Figure 7C. In this quarry, a curved cross-bed surface has many SSDSs—like those in Figures 3, 7A—on one face and none on the other face of the same bed. It follows that the factors triggering the SSD must have affected these two surfaces quite differently. If the entire cross-bed was deposited simultaneously, this indicates a directional component to the triggering mechanism (at least for these features), and a seismic stimulus arriving from a specific direction could explain the directionality. However, if one face was deposited later (e.g., from a change in wind direction), the different times of origin of the two faces could explain the difference in SSDS occurrence.
Directionality is seen in this quarry at outcrop scale; however, the difference between these two cross-bed surfaces is the only evidence for directionality that we observed. The small-scale SSD (e.g., rotated blocks) do not exhibit consistent preferred orientation, even within an individual cross-bed, which suggests that they were probably not displaced by a unidirectional force. The individual folds and ridges also do not show a common directional orientation (Figure 7B), implying a similar lack of directionality for their trigger(s). Or even if the trigger had a directional component, it seems that the orientation of specific structures was determined more by other, local, influences.
It has been suggested that seismic triggers are more likely for SSDSs that are constrained to a specific stratigraphic interval (Rosetti and Góes 2000; Owen et al., 2011). However, this criterion cannot be applied to the Coconino SS at an outcrop or regional scale: stratigraphic correlations within cross-bedded formations are difficult, since cross-beds cannot be traced past the bounding surfaces of each set. Therefore, we cannot reliably determine the lateral extent of horizons that contain deformation structures. In our study areas, SSDSs exhibit a wide stratigraphic distribution, from low in the Coconino SS to near its upper contact.
However, other criteria may apply at the bedding scale. Some authors suggest that when a deformed bed is under- or overlain by undeformed sediment, and all beds have the same sedimentological features, this supports a seismic trigger for the deformation (Rosetti and Góes 2000; Fernandes et al., 2007; Moretti and Ronchi 2011; Owen et al., 2011). If this interpretation is correct, it could explain some of our SSDSs (e.g., folds in Figures 6, 8C, 10B). Overlying beds were not present over our other samples (e.g., folds and ridges in Figures 3, 4, 10A), but we expect that these were similar, since the Coconino SS exhibits fairly uniform sedimentological characteristics within each cross-bed set. The 13 examples of folds and ridges seen in cross-section in vertical quarry walls, including Figures 6, 8C, were always overlain and underlain by undeformed sediment. The significance of this must be qualified, since a given cross-bed could be undeformed on its exposed surface, but be deformed deeper along its length. Nevertheless, the consistency of this evidence suggests a seismic interpretation is worth considering.
Liquidization in sands can result from earthquakes of about magnitude 5 or higher (Obermeier 1996). Experimental study is needed to determine whether this applies to small SSDSs—a few cm deep—such as those in the Coconino SS. If these SSDSs were triggered by seismic events, their wide stratigraphic distribution and shallow nature may not be consistent with one or a few large earthquakes as the trigger(s). It remains possible that numerous, localized seismic events occurred, or that far-field effects from distant earthquakes played a role in deforming the sediment. In any case, an explanation is needed for how seismic events could produce such shallow, localized SSD features. Cementation is not likely to occur so rapidly as to leave only a surface layer of unlithified or semilithified sand. Further study is needed on the potential dynamics of this process.
Small-scale (cm scale to 1.5 m) faults occur in the Coconino SS, but physical evidence for seismic events, outside of the SSD, is uncommon. The source of these seismic stresses in the Coconino SS, if present, is therefore uncertain at this time. However, seismic shaking of the sediment may explain the SSD. A study of the geographic and stratigraphic distribution of these small faults in relation to the SSD could be instructive. In one case, correlation is possible: the monoclinal fold in Figure 10A is at the top of the Coconino SS, at approximately the same stratigraphic level as a fault with a vertical displacement of 1.5 m, along the Hermit Trail, Grand Canyon.
Understanding processes that could control the shallow, scattered nature of the Coconino Sandstone SSDSs remains a challenge. Since deformation is restricted to certain foreset beds among hundreds, some characteristic of the individual beds (sedimentological parameters or depositional context) may explain this distribution. Even large seismic events will vary in their effect, based on the local rheology of the sediment and other parameters (Mills 1983; Owen 1987; Martin-Chivelet et al., 2011; Moretti and Ronchi 2011; Toro and Pratt 2015). These factors might determine whether seismic events produced shallow or relatively deep features, or none, and explain why they are stratigraphically scattered. A seismic trigger for the Coconino SS is plausible, but not demonstrated. However, for the reasons discussed above, the evidence is inconsistent with the other triggers listed—gravity slumping, climatic cycles, tsunamis, landslides, glacial loading, volcanic activity, meteorites, cyclonic waves, and tidal currents—for the Coconino SS. While uncertainty remains, we think seismic processes provide the most likely candidate at present.
Paleoenvironmental Implications
The SSDSs in the Coconino SS contrast with many of those in, for example, the Navajo Sandstone, not only in size but in the location of the deformations. Both involve water and liquidization, and both may be triggered by seismic events. In the Navajo Sandstone, the deformations are interpreted to have formed below the water table, and they may be of km-scale and involve multiple sets of cross-strata (Bryant et al., 2016). In contrast, the Coconino SS SSDSs are usually cm-scale and occur on individual cross-bed surfaces. Large, water table-associated SSDSs have not been documented in the Coconino SS; rather, the deformation that we observed likely implies processes that were far more localized in time and space. More work must be done to understand the paleoenvironmental implications of water in so many localized intervals during Coconino SS deformation. The underlying Hermit Formation is thought to have been deposited in a moister environment (Blakey 2003), and provides a possible source of water.
The Navajo Sandstone is a quartz-dominated cross-bedded sandstone, as is the Coconino SS, and the grain size is fine sand in both units (Uygur and Picard 1980; Maithel et al., 2021). One prominent difference is that the Coconino SS is much more strongly cemented than the Navajo Sandstone. The latter feature is not expected to affect the formation of SSD, but increases the likelihood of identifying SSDSs in the Coconino SS. If a cross-bedded sandstone does not split into flagstone-like slabs, exposing the original foreset surfaces, it will be difficult to find small SSDSs.
This research sheds light on long-debated details of the Coconino Sandstone’s paleoenvironment. The formation is commonly interpreted as a dry, eolian environment, but the SSDSs and the clay laminae described here have not been reported before, and these structures and the conditions necessary to produce them, imply that more water was present during Coconino SS deposition than previously recognized.
Recommended Research
Cross-bedded Permian sandstones comparable to the Coconino SS occur in Scotland and in continental Europe (McKeever 1991; Maithel et al., 2015), and fluidization and SSD have been reported in some of these formations (Glennie and Hurst 2007). These units should be further studied to explore potential similarity between their documented SSD and that observed in the Coconino SS. Also the extensive North Sea core datasets could be examined for SSD of this type. We recommend that future research address the relationship between SSD and clay abundance/distribution in cross-bedded sandstones. Do these other units split into flagstone slabs, and what is the relationship between such splitting and clay? Do we see a correlation between the clay laminae and the occurrence of SSD? It would also be beneficial to document the distribution of faults in the Coconino SS and their relationship to the deformation.
Conclusion
The small-scale structures in the Coconino SS appear to represent a distinctive suite of SSD, in terms of their bedding-plane scale and their occurrence in scattered stratigraphic intervals. Liquidization, pore water pressure, and complex lateral shearing may have pushed the sand into ridges, folds, and wider elevated shapes, and rotated blocks of sediment. In some examples, deposition of the overlying cross-bed truncated the top of the deformed unit, but in others, the deformation appears to have occurred intrastratally. Most of the deformation was likely synsedimentary or happened shortly after deposition.
Even though these SSD occur over a broad region, from the Grand Canyon at least to the area of Ash Fork, Arizona (nearly 200 km), their geographic distribution was constrained; they were not found farther to the south or east. This implies that the trigger was somewhat localized.
The Coconino SS is widely thought to have been deposited in an eolian environment, but sufficient water must have been present, at least in scattered intervals, to fluidize the sediment and produce the SSD described here. While large deformations occur in other sandstones (like the Navajo Sandstone), our structures are much finer in scale and probably formed under very different conditions. Characterizing processes associated with these SSDS may refine paleoenvironmental models for Coconino SS deposition, and inform interpretations of similar structures if they are found in other sandstones.
Seismic activity provides a possible trigger for this deformation, but the presence of SSD is so far the primary documented evidence for these implied seismic events. Depositional processes and rheology of individual cross-strata may help explain why deformation was restricted to specific beds; more work should be done to explore these parameters.
Data Availability Statement
The original contributions presented in the study are included in the article/Supplementary Material, further inquiries can be directed to the corresponding author.
Author Contributions
Both authors were fully involved in data collection and analysis. Manuscript written primarily by Brand, with input by SM.
Funding
Funding provided by Loma Linda University, Department of Earth and Biological Sciences, research account of LB.
Conflict of Interest
The authors declare that the research was conducted in the absence of any commercial or financial relationships that could be construed as a potential conflict of interest.
Publisher’s Note
All claims expressed in this article are solely those of the authors and do not necessarily represent those of their affiliated organizations, or those of the publisher, the editors and the reviewers. Any product that may be evaluated in this article, or claim that may be made by its manufacturer, is not guaranteed or endorsed by the publisher.
Acknowledgments
Research permits for this work included permit # GRCA-2016-SCI-0033 from the Grand Canyon National Park, and a research permit from the U.S. Department of Agriculture, Forest Service, for study in flagstone quarries in the Williams District of the Kaibab National Forest, Arizona. Big Boquillas Ranch provided access to the Seligman outcrops, and we thank Michael Reidhead for access to outcrops on his land near Holbrook. Thanks to Ronny Nalin, Kevin Nick, Geraint Owen, Balázs Törö, John Whitmore, Jasper Knight, and reviewers for suggestions that improved previous versions of the manuscript.
Abbreviations
SSD, soft-sediment deformation; SSDS, soft-sediment deformation structure; SSDSs, soft-sediment deformation structures.
References
Allen, J. R. L. (1977). The Possible Mechanics of Convolute Lamination in Graded Sand Beds. J. Geol. Soc. 134, 19–31. doi:10.1144/gsjgs.134.1.0019
Alvarez, W., Staley, E., O'Connor, D., and Chan, M. A. (1998). Synsedimentary Deformation in the Jurassic of southeastern Utah-A Case of Impact Shaking? Geol 26, 579–582. doi:10.1130/0091-7613(1998)026<0579:sditjo>2.3.co;2
Baars, D. L. (1961). “Permian Blanket Sandstones of Colorado Plateau,” in Geometry of Sandstone Bodies. Editors J. A. Peterson, and J. C. Osmond (Tulsa, OK: Amer. Assoc. of Petr. Geol.), 179–207.
Blakey, R. C., and Knepp, R. (1989). Pennsylvanian and Permian Geology of Arizona, in. Editors J. P. Jenney, and S. J. Reynolds. Tucson, AZ: Arizona Geological Society. 17, 313–347.
Blakey, R. C., and Middleton, L. T. (1983). “Permian Shoreline Eolian Complex in central Arizona: Dune Changes in Response to Cyclic Sealevel Changes,” in Eolian Sediments and Processes. Editors M. E. Brookfield, and T. S. Ahlbrandt (Amsterdam: Elsevier), 551–581. doi:10.1016/s0070-4571(08)70813-6
Blakey, R. C. (1990). Stratigraphy and Geologic History of Pennsylvanian and Permian Rocks, Mogollon Rim Region, central Arizona and Vicinity. Geol. Soc. Am. Bull. 102, 1189–1217. doi:10.1130/0016-7606(1990)102<1189:saghop>2.3.co;2
Blakey, R. C. (2003). Supai Group and Hermit Formation, in Grand Canyon Geology, 2nd. ed. Editors S. S. Beus, and M. Morales (Oxford University Press, New York), 136–162.
Blakey, R. C. (1988). Superscoops: Their Significance as Elements of Eolian Architecture. Geol 16, 483–487. doi:10.1130/0091-7613(1988)016<0483:stsaeo>2.3.co;2
Brand, L. (1979). Field and Laboratory Studies on the Coconino sandstone (Permian) Vertebrate Footprints and Their Paleoecological Implications. Palaeogeogr. Palaeoclimatol. Palaeoecol. 28, 25–38. doi:10.1016/0031-0182(79)90111-1
Brand, L. R., and Kramer, J. (1996). Underprints of Vertebrate and Invertebrate Trackways in the Coconino SS (Permian) in Northern Arizona. Ichnos 4, 1–6. doi:10.1080/10420949609380129
Brand, L. R. (1992). Reply to Comments on "fossil Vertebrate Footprints in the Coconino SS (Permian) of Northern Arizona: Evidence for Underwater Origin. Geology 20, 668–670. doi:10.1130/0091-7613(1992)020%3C0666:CAROFV%3E2.3.CO;2
Brand, L. R., and Tang, T. (1991). Fossil Vertebrate Footprints in the Coconino Sandstone (Permian) of Northern Arizona: Evidence for Underwater Origin. Geol 19, 1201–1204. doi:10.1130/0091-7613(1991)019<1201:fvfitc>2.3.co;2
Bryant, G., Cushman, R., Nick, K., and Miall, A. (2016). Paleohydrologic Controls on Soft-Sediment Deformation in the Navajo Sandstone. Sediment. Geology. 344, 205–221. doi:10.1016/j.sedgeo.2016.06.005
Bryant, G., and Miall, A. (2010). Diverse Products of Near-Surface Sediment Mobilization in an Ancient Eolianite: Outcrop Features of the Early Jurassic Navajo Sandstone. Basin Res. 22, 578–590. doi:10.1111/j.1365-2117.2010.00483.x
Chan, M. A., and Bruhn, R. L. (2014). Dynamic Liquefaction of Jurassic Sand Dunes: Processes, Origins, and Implications. Earth Surf. Process. Landforms 39, 1478–1491. Oxford University Press, New York. doi:10.1002/esp.3539
Chan, M., Kocurek, G., Netoff, D., Alvarez, W., and Blakey, R. (2007). “Clastic-injection Pipes and Syndepositional Deformation Structures in Jurassic Eolian Deposits: Examples from the Colorado Plateau,” in Sand Injectites: Implications for Hydrocarbon Exploration and Production. Editors A. Hurst, and J. Cartwright (Tulsa, Oklahoma, United States: Amer. Assoc. of Petrol. Geol. Memoir), 87, 233–244.
Citton, P., Sacchi, E., and Nicosia, U. (2012). Sometimes They Come Back: Recovery and Reinterpretation of a Trackway Slab from the Permian Coconino Sandstone of the Southwestern United States. Ichnos 19, 165–174. doi:10.1080/10420940.2012.702607
Cojan, I., and Thiry, M. (1992). Seismically Induced Deformation Structures in Oligocene Shallow-marine and Aeolian Coastal Sands (Paris Basin). Tectonophysics 206, 79–89. doi:10.1016/0040-1951(92)90369-h
Davis, G. H., and Bump, A. P. (2009). “Structural Geological Evolution of the Colorado Plateau,” in Backbone of the Americas: Shallow Subduction, Plateau Uplift, and Ridge and Terrane Collision. Editors S. M. Kay, V. A. Ramos, and W. R. Dickinson (Boulder, CO: Geol. Soc. of Amer. Memoir), 204, 99–124.
Doe, T. W., and Dott, R. H. (1980). Genetic Significance of Deformed Cross-Bedding—With Examples from the Navajo and Webber Sandstones of Utah. J. Sed. Petrol. 50, 793–812. doi:10.1306/212f7aef-2b24-11d7-8648000102c1865d
Elcock, S. (1993). A Study of the Early Permian Coconino SS, Arizona. Thesis. West Midlands, UK: University of Wolverhampton, 79.
Elliott, D. K., and Bartlett, R. W. (2013). A New Trace Fossil from the Permian Coconino SS of Northern Arizona. in The Carboniferous-Permian Transition. Editors S.G Lucas, W.A DiMichelle, J.A Barrick, J.W Schneider, and J.S Spielman New Mexico, USA: New Mexico Mus. Nat. Hist. Sci. Bull. 60, 79–80.
Fernandes, L. A., de Castro, A. B., and Basilici, G. (2007). Seismites in continental Sand Sea Deposits of the Late Cretaceous Caiuá Desert, Bauru Basin, Brazil. Sediment. Geology. 199, 51–64. doi:10.1016/j.sedgeo.2005.12.030
Fisher, W. L. (1961). Upper Paleozoic and Lower Mesozoic Stratigraphy of Parashant and Andrus Canyons, Mohave County, Northwestern Arizona. Northwestern Arizona: Dissertation, University of Kansas, 345.
Glennie, K., and Hurst, A. (2007). “Fluidization and Associated Soft-Sediment Deformation in Eolian Sandstones: Hopeman Sandstone (Permian), Scotland, and Rotliegend, North Sea,” in Sand Injectites: Implications for Hydrocarbon Exploration and Production. Editors A. Hurst, and J. Cartwright (Tulsa, Oklahoma, United States: Amer. Assoc. of Petrol. Geol. Memoir), 87, 245–252.
Horowitz, D. H. (1982). Geometry and Origin of Large-Scale Deformation Structures in Some Ancient Wind-Blown Sand Deposits. Sedimentology 29, 155–180. doi:10.1111/j.1365-3091.1982.tb01717.x
Jones, A. P., and Omoto, K. (2000). Towards Establishing Criteria for Identifying Trigger Mechanisms for Soft-Sediment Deformation: a Case Study of Late Pleistocene Lacustrine Sands and Clays, Onikobe and Nakayamadaira Basins, Northeastern Japan. Sedimentology 47, 1211–1226. doi:10.1046/j.1365-3091.2000.00355.x
Kocurek, G. (1981). Significance of Interdune Deposits and Bounding Surfaces in Aeolian Dune Sands. Sedimentology 28, 753–780. doi:10.1111/j.1365-3091.1981.tb01941.x
Liesa, C. L., Rodríguez-López, J. P., Ezquerro, L., Alfaro, P., Rodríguez-Pascua, M. Á., Lafuente, P., et al. (2016). Facies Control on Seismites in an Alluvial-Aeolian System: The Pliocene Dunefield of the Teruel Half-Graben basin (Eastern Spain). Sediment. Geology. 344, 237–252. doi:10.1016/j.sedgeo.2016.05.009
Lockley, M., and Hunt, A. P. (1995). Dinosaur Tracks and Other Fossil Footprints of the Western United States. NY: Columbia University Press, 40–56.
Loope, D. B. (1984). Eolian Origin of Upper Paleozoic Sandstones, southeastern Utah. J. Sed. Petrol. 54, 563–580. doi:10.1306/212f846d-2b24-11d7-8648000102c1865d
Loope, D. B., Rowe, C. M., and Joeckel, R. M. (2001). Annual Monsoon rains Recorded by Jurassic Dunes. Nature 412, 64–66. doi:10.1038/35083554
Lowe, D. R. (1976). Subaqueous Liquefied and Fluidized Sediment Flows and Their Deposits. Sedimentology 23, 285–308. doi:10.1111/j.1365-3091.1976.tb00051.x
Lowe, D. R. (1975). Water Escape Structures in Coarse-Grained Sediments. Sedimentology 22, 157–204. doi:10.1111/j.1365-3091.1975.tb00290.x
Lundy, W. L. (1973). The Stratigraphy and Evolution of the Coconino SS of Northern Arizona. Tulsa, OK: Thesis, University of Tulsa, 106.
Maithel, S. A., Brand, L. R., and Whitmore, J. H. (2019). A Methodology for Disaggregation and Textural Analysis of Quartz-Cemented Sandstones. J. Sediment. Res. 89, 599–609. doi:10.2110/jsr.2019.35
Maithel, S. A., Brand, L. R., and Whitmore, J. H. (2021). Characterization of Hard‐to‐differentiate Dune Stratification Types in the Permian Coconino Sandstone (Arizona, USA). Sedimentology 68, 238–265. doi:10.1111/sed.12774
Maithel, S. A., Garner, P. A., and Whitmore, J. H. (2015). Preliminary Assessment of the Petrology of the Hopeman Sandstone (Permo-Triassic), Moray Firth Basin, Scotland. Scottish J. Geology. 51, 177–184. doi:10.1144/sjg2014-028
Martín-Chivelet, J., Palma, R. M., López-Gómez, J., and Kietzmann, D. A. (2011). Earthquake-induced Soft-Sediment Deformation Structures in Upper Jurassic Open-marine Microbialites (Neuquén Basin, Argentina). Sediment. Geology. 235, 210–221. doi:10.1016/j.sedgeo.2010.09.017
McKee, E. D., and Bigarella, J. J. (1979). “Ancient Sandstones Considered to Be Eolian,” in A Study of Global Sand Seas. Editor E. D. McKee (Washington, D.C.: U. S. Geol. Survey Prof. Paper 1052), 429.
McKee, E. D., Douglass, J. R., and Rittenhouse, S. (1971). Deformation of lee-side Laminae in Eolian Dunes. Geol. Soc. America Bull. 82, 359–378. doi:10.1130/0016-7606(1971)82[359:dollie]2.0.co;2
McKee, E. D. (1974). “Paleozoic Rocks of Grand Canyon,” in Geology of Northern Arizona with Notes on Archaeology and Paleoclimate, Part 1 - Regional Studies. Editors T. N. V. Karlstrom, G. A. Swann, and R. L. Eastwood (Flagstaff, AZ): Annual Meeting), 119–154.
McKee, E. D. (1945). Small-Scale Structures in the Coconino Sandstone of Northern Arizona. J. Geology. 53, 313–325. doi:10.1086/625291
McKee, E. D. (1934). The Coconino SS – its History and Origin, 440. Carnegie Instit. of Wash., Publ., 77–115.
McKeever, P. J. (1991). Trackway Preservation in Eolian Sandstones from the Permian of Scotland. Geol 19, 726–729. doi:10.1130/0091-7613(1991)019<0726:tpiesf>2.3.co;2
Middleton, L. T., and Blakey, R. C. (1983). “Processes and Controls on the Intertonguing of the Kayenta and Navajo Formations, Northern Arizona: Eolian-Fluvial Interactions,” in Eolian Sediments and Processes. Editors M. E. Brookfield, and T. S. Ahlbrandt (Amsterdam): Elsevier), 613–634. doi:10.1016/s0070-4571(08)70815-x
Middleton, L. T., Elliot, D. K., and Morales, M. (2003). “Coconino SS,” in Grand Canyon Geology. Editors S. S. Beus, and M. Morales (Morales NY: Oxford University Press), 163–179.
Millhouse, A. M. (2009). Analysis of Trackways in the Permian Coconino SS of Ash Fork and Grand Canyon, Arizona. M.S. Thesis. Flagstaff, AZ: Northern Arizona University.
Mills, P. C. (1983). Genesis and Diagnostic Value of Soft-Sediment Deformation Structures-A Review. Sediment. Geology. 35, 83–104. doi:10.1016/0037-0738(83)90046-5
Moretti, M., Alfaro, P., Caselles, O., and Canas, J. A. (1999). Modelling Seismites with a Digital Shaking Table. Tectonophysics 304, 369–383. doi:10.1016/s0040-1951(98)00289-3
Moretti, M., and Ronchi, A. (2011). Liquefaction Features Interpreted as Seismites in the Pleistocene Fluvio-Lacustrine Deposits of the Neuquén Basin (Northern Patagonia). Sediment. Geology. 235, 200–209. doi:10.1016/j.sedgeo.2010.09.014
Moretti, M. (2000). Soft-sediment Deformation Structures Interpreted as Seismites in Middle-Late Pleistocene Aeolian Deposits (Apulian Foreland, Southern Italy). Sediment. Geology. 135, 167–179. doi:10.1016/s0037-0738(00)00070-1
Netoff, D. (2002). Seismogenically Induced Fluidization of Jurassic Erg Sands, South-central Utah. Sedimentology 49, 65–80. doi:10.1046/j.1365-3091.2002.00432.x
Nichols, R. J. (1995). “The Liquification and Remobilization of sandy Sediments,” in Characterization of Deep Marine Clastic Systems. Editors A. J. Hartley, and D. J. Prosser (London, United Kingdom: Geol. Soc. Special Publications), 94, 63–76. doi:10.1144/gsl.sp.1995.094.01.06
Obermeier, S. F. (1996). Use of Liquefaction-Induced Features for Paleoseismic Analysis - an Overview of How Seismic Liquefaction Features Can Be Distinguished from Other Features and How Their Regional Distribution and Properties of Source Sediment Can Be Used to Infer the Location and Strength of Holocene Paleo-Earthquakes. Eng. Geology. 44, 1–76. doi:10.1016/s0013-7952(96)00040-3
Owen, G. (1987). “Deformation Processes in Unconsolidated Sands,” in Deformation of Sediments and Sedimentary Rocks. Editors M. E. Jones, and R. M. F. Preston (London, United Kingdom: Geol. Soc. of London Special Publications), 29, 11–24. doi:10.1144/gsl.sp.1987.029.01.02
Owen, G. (1996). Experimental Soft-Sediment Deformation: Structures Formed by the Liquefaction of Unconsolidated Sands and Some Ancient Examples. Sedimentology 43, 279–293. doi:10.1046/j.1365-3091.1996.d01-5.x
Owen, G., Moretti, M., and Alfaro, P. (2011). Recognising Triggers for Soft-Sediment Deformation: Current Understanding and Future Directions. Sediment. Geology. 235, 133–140. doi:10.1016/j.sedgeo.2010.12.010
Owen, G., and Moretti, M. (2011). Identifying Triggers for Liquefaction-Induced Soft-Sediment Deformation in Sands. Sediment. Geology. 235, 141–147. doi:10.1016/j.sedgeo.2010.10.003
Owen, G. (1995). Soft-sediment Deformation in Upper Proterozoic Torridonian Sandstones (Applecross Formation) at Torridon, Northwest Scotland. J. Sediment. Res. A65, 495–504. doi:10.1306/d4268108-2b26-11d7-8648000102c1865d
Reiche, P. (1938). An Analysis of Cross-Lamination the Coconino Sandstone. J. Geology. 46, 905–932. doi:10.1086/624709
Rodríguez-lópez, J. P., Meléndez, N., De Boer, P. L., and Soria, A. R. (2008). Aeolian Sand Sea Development along the Mid-cretaceous Western Tethyan Margin (Spain): Erg Sedimentology and Palaeoclimate Implications. Sedimentology 55, 1253–1292. doi:10.1111/j.1365-3091.2007.00945.x
Rodríguez-Pascua, M. A., Calvo, J. P., De Vicente, G., and Gómez-Gras, E. (2000). Soft-sediment Deformation Structures Interpreted as Seismites in Lacustrine Sediments of the Prebetic Zone, SE Spain, and Their Potential Use as Indicators of Earthquake Magnitudes during the Late Miocene. Sed. Geol. 135, 117–135.
Rossetti, D. F., and Góes, A. M. (2000). Deciphering the Sedimentological Imprint of Paleoseismic Events: an Example from the Aptian Codó Formation, Northern Brazil. Sediment. Geology. 135, 137–156. doi:10.1016/s0037-0738(00)00068-3
Sanderson, I. A. (1974). Sedimentary Structures and Their Environmental Significance in the Navajo Sandstone, San Rafael Swell, Utah. Salt Lake City, Utah: Brigham Young University. M.S. Thesis.
Schindelin, J., Arganda-Carreras, I., Frise, E., Kaynig, V., Longair, M., Pietzsch, T., et al. (2012). Fiji: an Open-Source Platform for Biological-Image Analysis. Nat. Methods 9 (7), 676–682. doi:10.1038/nmeth.2019
Schneider, C. A., Rasband, W. S., and Eliceiri, K. W. (2012). NIH Image to ImageJ: 25 Years of Image Analysis. Nat. Methods 9 (9), 671–675. doi:10.1038/nmeth.2089
Shanmugam, G. (2017). Global Case Studies of Soft-Sediment Deformation Structures (SSDS): Definitions, Classifications, Advances, Origins, and Problems. J. Palaeogeogr. 6, 251–320. doi:10.1016/j.jop.2017.06.004
Shanmugam, G. (2016). The Seismite Problem. J. Palaeogeogr. 5, 318–362. doi:10.1016/j.jop.2016.06.002
Sorauf, J. E. (1962). Structural Geology and Stratigraphy of the Whitmore Area, Mohave County, Arizona. Lawrence, KS: Dissertation, University of Kansas, 361.
Sumner, C. D. (1999). Stratigraphy of the Northern Depositional Margin of the Coconino SS, Northern Arizona and Southern Utah. Nacogdoches, TX: Austin State University, 161. Thesis, Stephen F.
Toro, B., and Pratt, B. R. (2015). “Characteristics and Implications of Sedimentary Deformation Features in the Green River Formation (Eocene) in Utah and Colorado,” in Geology of Utah’s Uinta Basin and Uinta Mountains. Editors M. D. Vanden Berg, R. Ressetar, and L. P. Birgenheier (Salt Lake City, UT: Utah Geol. Assoc. Publication), 44, 371–422.
Uygur, K., and Picard, M. D. (1980). “Reservoir Characteristics of Jurassic Navajo Sandstone, Southern Utah,” in Henry Mountains Symposium. Editor M. D. Picard (Salt Lake City, UT): Utah Geol. Assoc. Publication. Utah Geol. Assoc.), 277–286.
van Loon, A. J., and Brodzikowski, K. (1987). Problems and Progress in the Research on Soft-Sediment Deformations. Sediment. Geology. 50, 167–193. doi:10.1016/0037-0738(87)90032-7
van Loon, A. J. (2009). Soft-sediment Deformation Structures in Siliclastic Sediments: an Overview. Geologos 15, 3–55.
Keywords: soft-sediment deformation, permian, cross-bedded sandstone, coconino sandstone, earthquake
Citation: Brand L and Maithel S (2021) Small-Scale Soft-Sediment Deformation Structures in the Cross-Bedded Coconino Sandstone (Permian; Arizona, United States); Possible Evidence for Seismic Influence. Front. Earth Sci. 9:723495. doi: 10.3389/feart.2021.723495
Received: 10 June 2021; Accepted: 02 November 2021;
Published: 19 November 2021.
Edited by:
Barbara Mauz, University of Salzburg, AustriaReviewed by:
David Mark Hodgson, University of Leeds, United KingdomYvonne Therese Spychala, Leibniz University Hannover, Germany
Copyright © 2021 Brand and Maithel. This is an open-access article distributed under the terms of the Creative Commons Attribution License (CC BY). The use, distribution or reproduction in other forums is permitted, provided the original author(s) and the copyright owner(s) are credited and that the original publication in this journal is cited, in accordance with accepted academic practice. No use, distribution or reproduction is permitted which does not comply with these terms.
*Correspondence: Leonard Brand, lbrand@llu.edu