- 1Department of Civil Engineering, University of Alaska Anchorage, Anchorage, AK, United States
- 2Department of Geological Sciences, University of Alaska Anchorage, Anchorage, AK, United States
Two prominent arctic coastal erosion mechanisms affect the coastal bluffs along the North Slope of Alaska. These include the niche erosion/block collapse mechanism and the bluff face thaw/slump mechanism. The niche erosion/block collapse erosion mechanism is dominant where there are few coarse sediments in the coastal bluffs, the elevation of the beach below the bluff is low, and there is frequent contact between the sea and the base of the bluff. In contrast, the bluff face thaw/slump mechanism is dominant where significant amounts of coarse sediment are present, the elevation of the beach is high, and contact between the sea and the bluff is infrequent. We show that a single geologic parameter, coarse sediment areal density, is predictive of the dominant erosion mechanism and is somewhat predictive of coastal erosion rates. The coarse sediment areal density is the dry mass (g) of coarse sediment (sand and gravel) per horizontal area (cm2) in the coastal bluff. It accounts for bluff height and the density of coarse material in the bluff. When the areal density exceeds 120 g cm−2, the bluff face thaw/slump mechanism is dominant. When the areal density is below 80 g cm−2, niche erosion/block collapse is dominant. Coarse sediment areal density also controls the coastal erosion rate to some extent. For the sites studied and using erosion rates for the 1980–2000 period, when the sediment areal density exceeds 120 g cm−2, the average erosion rate is low or 0.34
Introduction
The Arctic is experiencing high and accelerating coastal erosion rates. For example, Mars and Houseknecht (2007) used remote sensing techniques to study coastal erosion-derived land loss on a 60-km segment of the Beaufort Sea coast (between Drew Point and Cape Halkett, Alaska, Figure 1) and found that the amount of land loss was significantly greater in 1985–2005 (1.08 km2 yr−1) relative to the loss in 1955–1985 (0.48 km2yr−1). Jones et al. (2009) working in the same area determined that the average rate of erosion increased from 6.8 m yr−1 (1955–1979), to 8.7 m yr−1 (1979–2002), and to 13.6 m yr−1 (2002–2007). Erosion rates are high in this location because of the high ice content of the coastal bluffs and the absence of coarse material (sand and gravel). At other locations, erosion rates are often lower but still accelerating. For example, on Barter Island, where coastal bluffs contain significant amounts of coarse material, bluff retreat rate averaged 1.8 myr−1 between 1955 and 2004 and 3.8 m yr−1 between 2004 and 2010 (Gibbs et al., 2010). Erosion rates are generally accelerating because of 1) greater spatial extent of open water, which allows for the generation of larger waves, 2) greater open water period, and 3) increased rate of coastal permafrost thaw (Barnhart et al., 2014a; Barnhart et al., 2014b; Frederick et al., 2016). Erosion threatens coastal infrastructure throughout the Arctic including governmental assets and community infrastructure. The US Army Corps of Engineers (2009) has designated 26 Alaska communities (including Barrow) “Priority Action Communities” due to the threat of erosion.
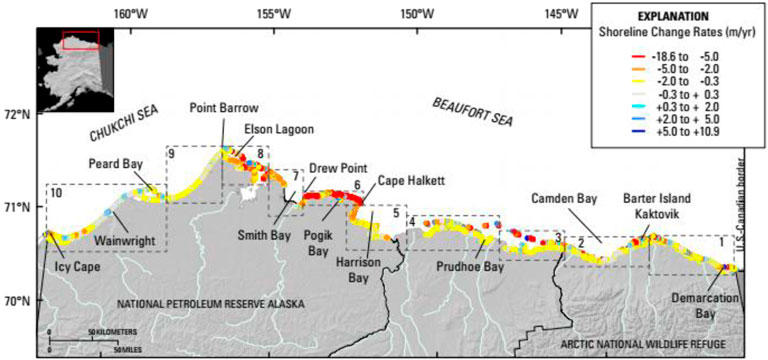
FIGURE 1. Map of the north coast of Alaska showing color-coded shoreline change rates for the period circa-1940s (1947 and 1949) to circa-2000s (1997–2012, Gibbs and Richmond, 2015).
A number of arctic coastal erosion mechanisms affecting high coastal bluffs in the Arctic have been identified including niche erosion/block collapse (prevalent in the Drew Point area (Ravens et al., 2012; Barnhart et al., 2014a)) and bluff face thaw/slump (also referred to as translational-shear ice-thaw, Gibbs et al., 2013, and thermal denudation, Barankaya et al., 2021). The erosion mechanisms affecting Arctic coastal bluffs differ from the erosion of non-Arctic bluffs (e.g., Carter and Guy 1988) because of the role played by thermal processes in the Arctic. With the niche erosion/block collapse erosion mechanism, typically a small beach is present before the bluff (Figures 2, 3). During a storm surge event, waters rise allowing contact between sea and the base of the bluff. Waves and currents thermally and mechanically carve a niche at the base of the bluff (Kobayashi 1985). Niche growth undermines the bluffs leading to block collapse due to an overturning failure (Hoque and Pollard 2016). The lower failure plane intersects with a shore parallel ice wedge (Figure 4). The upper failure plane is at interface of the ice wedge and the soil. The failure is governed by the tensile strength of the frozen soil, as well as the niche depth, the ice wedge location, and the depth of the ice wedge. Niche erosion/block collapse is the predominant erosion mechanism in settings where the coastal bluffs have high ice content (∼70%, Ping et al., 2011), and where the bluffs lack significant amounts of coarse material (sand and gravel). The lack of coarse material leads to a low elevation beach at the base of the bluff and frequent contact between the sea and the coastal bluffs (Ravens et al., 2011; Ravens and Peterson 2018).
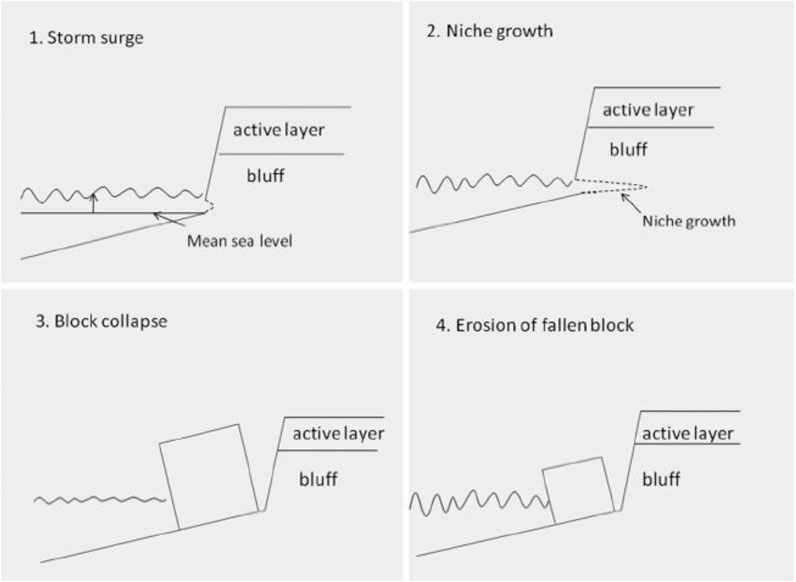
FIGURE 2. Conceptual model of the niche erosion/block collapse erosion mechanism (from Ravens et al., 2012).
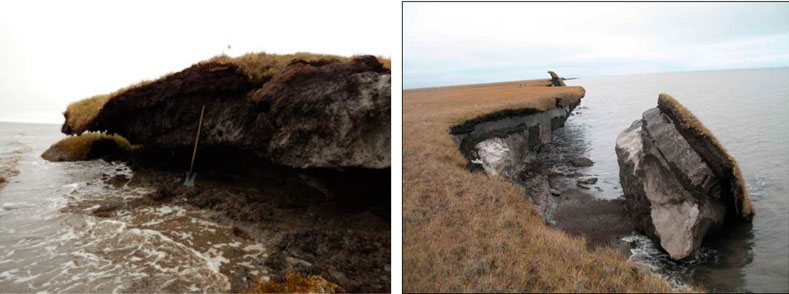
FIGURE 3. Photos of (A) an erosional niche from Elson Lagoon Alaska and (B) a fallen block by Drew Point, Alaska (image courtesy of Christopher Arp of the Alaska Science Center, U.S. Geological Survey).
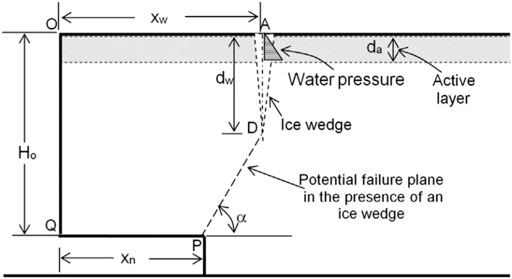
FIGURE 4. Sketch of the bluff cross-section assumed by Hoque and Pollard (2016) in their analysis of overturning failure.
Bluff face thaw/slump is the predominant erosion mechanism in settings where significant amounts of coarse sediments are common (e.g., at Barter Island, Ravens et al., 2011; Ravens and Peterson 2018). With significant amounts of coarse sediments in the coastal bluffs, the elevation of the beach before the bluff is relatively high (1–2 m above mean sea level) and contact between the sea and the base of the bluff—and niche erosion—is infrequent. For example, data provided by the USGS (Ann Gibbs, personal communication) indicates that only a single significant niche erosion/block collapse event occurred in the 1955–2010 time period at Barter Island which has significant amounts of coarse sediments (Figure 5). The bluff face warms due to the combined effect of a number of heat transfer processes including solar (shortwave) radiation, longwave radiation emission from the Earth’s surface, absorption of downward longwave radiation from the atmosphere, sensible heat flux, and latent heat flux (Westermann et al., 2009; Ravens and Ulmgren, 2020). When the bluff face is warmed sufficiently, it thaws and material slumps to the beach face (Figures 5,6). Relatively small storms (e.g., the 1-year return period storm) are sufficient to remove the sediment that accumulates on the beach (Ravens et al., 2011).
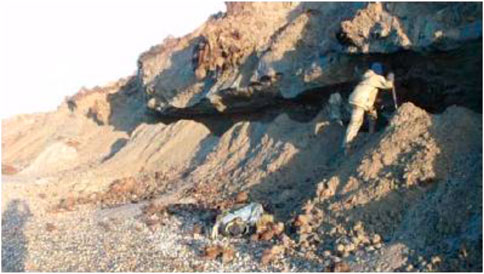
FIGURE 5. Photo showing material that has slumped onto the beach face following bluff face thaw at Barter Island (2011 image courtesy of Li Erikson, U.S. Geological Survey). The bluff height is about 10 m and the sediment areal density is about 600 g/cm2, based on USGS data. Interestingly, the photo was taken soon after the 2008 niche erosion/block collapse event and the niche is still in evidence.
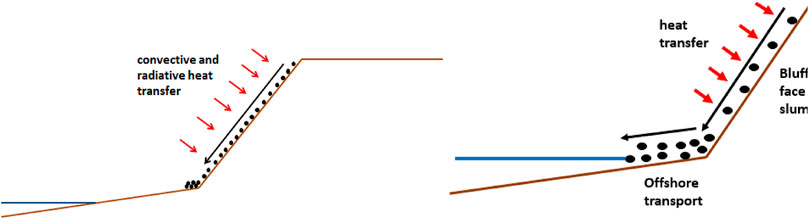
FIGURE 6. Conceptual depiction of the bluff face thaw/slump erosion mechanism, which includes 1) the thawing of the bluff face, followed by 2) the slumping and deposition on the beach face, followed by 3) the offshore transport due to storm surge and waves.
Ravens et al. (2011) defined a parameter, the “coarse sediment areal density”, and they hypothesized that this parameter determined whether the bluffs at a given coastal site were controlled by niche erosion/block collapse or by bluff face thaw/slumping. The sediment areal density is the dry mass of coarse sediment (sand and gravel) contained in a column of bluff sediment/soil per unit horizontal area (g cm-2). If there was a virtual column in the bluff extending from mean sea level to the bluff top, the coarse sediment areal density would be the dry mass of coarse sediment (sand and gravel) per unit horizontal area in the column. In this paper, we test this hypothesis by examining the extent to which coarse sediment areal density can predict coastal erosion mechanism. We also examine the relationship between coarse sediment areal density and coastal erosion rate.
Methodology
Coastal locations with both sediment data and aerial photo data from the north coast of Alaska between Utqiagvik (formerly Barrow) and the Canadian border were sought. Data on sediment grain size distribution (percent sand, silt, and clay) as a function of depth into the bluffs, sediment bulk density, and bluff height were obtained from 22 coastal sites according to Ping et al. (2011). Note, Ping et al. (2011) did not report on the presence of gravel so we concluded that it was negligible in their samples. However, the USGS, working at their Barter Island site, found significant gravel (Gibbs et al., 2010). The samples were collected from undisturbed areas between ice wedges after removal of slumped material. We examined oblique aerial photos from Gibbs and Richmond (2009) at locations proximal to the sites with sediment data to determine if the coastal erosion mechanism was niche erosion/block collapse or bluff face thaw/slump (Table 1). On average, the distance between location with sediment data and photos was about 6 km. For each photo, sand and gravel content data from one proximal core or bluff sample was used to determine the sediment areal density (Figure 7). Locations experiencing niche erosion/block collapse were readily determined based on the characteristic erosional blocks (Figure 8). Locations dominated by bluff face thaw/slump were evident based on the presence of a high elevation beach before the coastal bluff and the presence of material (e.g., vegetation) that was slumping on the bluff face (Figure 9). The coarse sediment areal density (g cm-2) was calculated as the product of the coarse sediment (sand and gravel) content (%), sediment bulk density (g cm-3) and the bluff height (cm), using data from Ping et al., 2011. The ice content of the bluffs was implicitly included in the sediment bulk density.
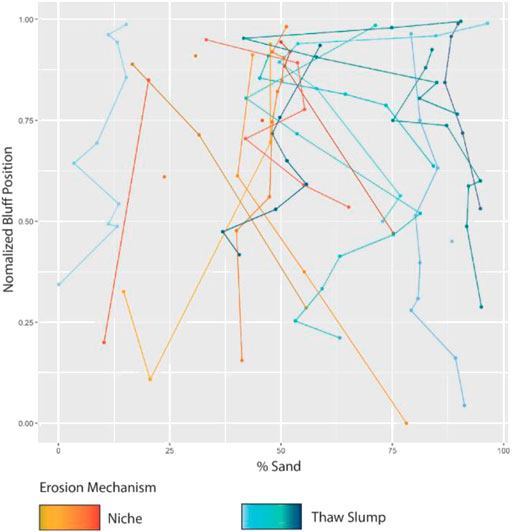
FIGURE 7. Plot showing sand content (%) as a function of normalized bluff position (depth/bluff. height) at the various sites for which sediment data was available. The plot also identifies the erosion mechanism inferred based on aerial photo analysis. Note, in some instances, only a single bluff sample was analyzed and these data are plotted as dots. Note, the low sand content of one core (BSC41b in Table 1), identified as a site of bluff face thaw/slump erosion, appears to be an outlier. However, the coarse sediment areal density of this site (82.7 g/cm2, Table 1) is similar to that calculated for other bluff face thaw/slump sites.
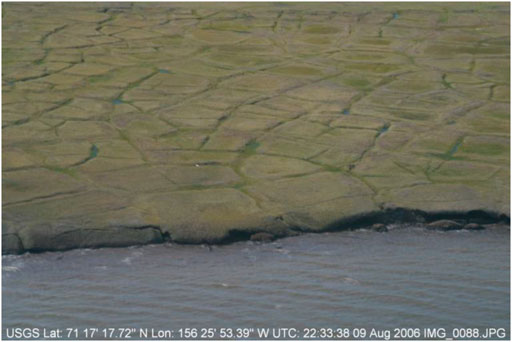
FIGURE 8. Example photo of coastal bluffs where niche erosion/block collapse was the predominant mechanism (image courtesy of Ann Gibbs, U.S. Geological Survey).
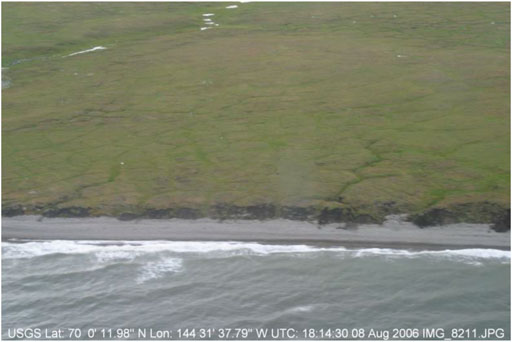
FIGURE 9. Example photo of coastal bluffs where bluff face thaw/slumping was the predominant erosion mechanism (image courtesy of Ann Gibbs, U.S. Geological Survey).
Results and Discussion
The locations of the 19 coastal sites subject to analysis, as well as the erosion mechanisms attributed to those sites based on the analysis of the aerial photos, are shown in Figure 10. It is noteworthy that the majority of the sites experiencing niche erosion/block collapse are on the western side of the study domain, whereas the sites experiencing bluff face thaw/slump are mainly on the eastern side. Note also that there was relatively little variation of erosion mechanism with position according to our analysis. The frequency of occurrence of the niche erosion/block collapse mechanism and the bluff face thaw/slump mechanism relative to the course sediment areal density (g cm−2, Figure 11) shows that with sediment areal density greater than 120 g cm−2, the dominant erosion mechanism was bluff face thaw/slumping. With sediment areal density less than 80 g cm−2, the dominant erosion mechanism was niche erosion/block collapse. One might wonder whether the erosion mechanism at specific sites, inferred based on the 2006 areal photos, might vary over time. It is noteworthy that, for example, Elson Lagoon, Drew Point, and Barter Island have been subject to numerous research papers over the past few decades, and there has been no mention of a change in erosion mechanism although there are some caveats. First, Barter Island has eroded mainly due to bluff face thaw/slump (as expected due to its high sediment areal density), but it was subject to a significant niche erosion/block collapse event during a large 2008 storm (Gibbs et al., 2010; Ravens et al., 2011). Also, Gibbs et al. (2019) point out the seasonality of erosion mechanism. In early to mid-summer, there tends to be more bluff face thaw/slumping because of the high levels of solar (short wave) radiation. In the second half of the summer, after the thaw of sea ice, storm surges and wave action bring aggressive mechanical forces to the coast removing previously thawed and deposited material, and potentially causing niche erosion if the beach elevation is sufficiently low.
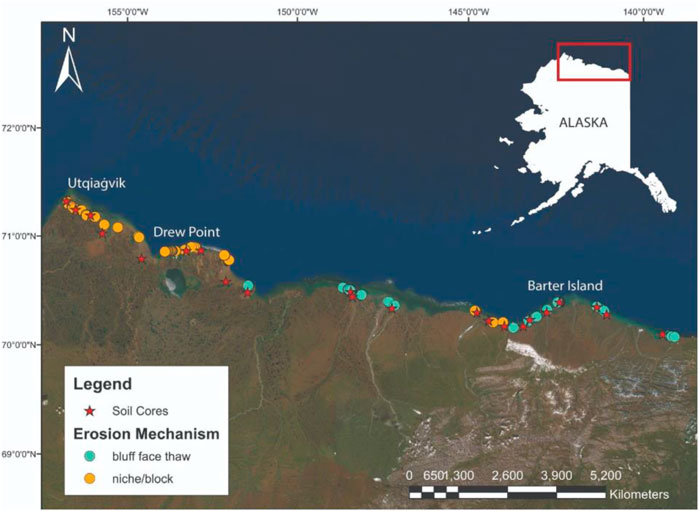
FIGURE 10. Map of the north coast of Alaska showing the locations of the coastal sites studied as well as the erosion mechanism attributed to those sites. Base map imagery courtesy of Esri.
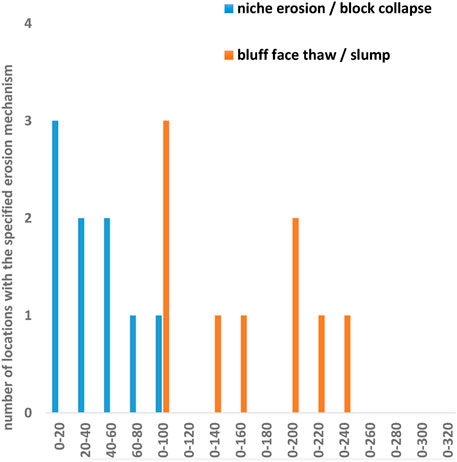
FIGURE 11. A histogram showing the frequency of occurrence of the niche erosion/block collapse erosion mechanism and bluff face thaw/slump mechanism as a function of coarse sediment areal density.
Erosion rates for the 1980–2000 period (from Ping et al., 2011) are plotted relative to coarse sediment areal density (Figure 12). For sediment areal density values greater than 120 g cm−2 (coincident with the bluff face thaw/slump mechanism), erosion rates ranged from 1.24 m/yr to −1.55 m/yr (i.e., an accretion of 1.55 m/yr) with an average erosion rate of 0.34
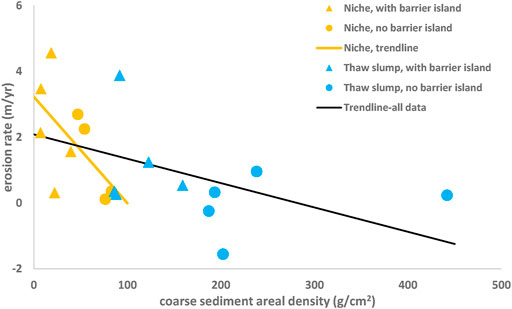
FIGURE 12. Dependence of coastal erosion rates for the 1980–2000 time period on coarse sediment areal density, for sites experiencing niche erosion/block collapse and bluff face thaw/slump. Note, the figure provides data on coastal sites that are protected by barrier islands as well as ones without protection as indicated in the legend. Trend lines are provided for sites with niche erosion/block collapse (orange line, R2 = 0.37) as well as considering all sites (black line, R2 = 0.25). For bluff face thaw/slump sites, the correlation was negligible (R2 = 0.09) and no trend line is provided.
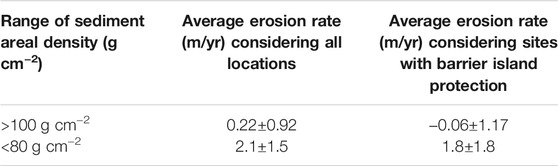
TABLE 2. Average erosion rates (for 1980–2000 period) for different ranges of coarse sediment areal density.
Analysis was also performed to determine whether the presence of barrier island protection translated to reduced erosion rates for the two ranges of sediment areal density and the associated erosion mechanisms. For locations with coarse sediment areal density above 120 g cm−2 (i.e., bluff face thaw/slump sites), the average erosion rate was reduced from 0.34
A significant amount of the variance in the measured erosion rate could not be explained using the coarse sediment areal density alone. Various explanations for the unexplained variance exist. First, we had to work with a significant distance (order 1 km) between the location of the erosion measurement and the borehole from which the sediment areal density was derived. Given spatial non-uniformity in the coastal stratigraphy, it is reasonable to suggest that the sediment areal density at the location of the erosion measurement differed from the density at the borehole. Second, there are many environmental variables that affect erosion but were not included in the regression including: nearshore water surface elevation, nearshore wave condition, and nearshore water and air temperature. Third, the way in which environmental variables affect arctic coastal erosion can be quite complex as indicated by process-based approaches to determine erosion rate (Ravens et al., 2012; Barnhart et al., 2014a).
The analysis presented above focuses on the predictability of Arctic coastal erosion mechanism based on sediment areal density. However, once this relationship has been established, it is noteworthy that sediment character can be inferred to some extent based on the erosion mechanism. For example, in locations where niche erosion/block collapse is dominant, we can infer that the coarse sediment in the eroding bluffs in limited. Such insights could be used in sediment transport and other studies.
Conclusion
The research presented here suggests that a single geologic parameter, the coarse sediment areal density, controls the dominant arctic coastal erosion mechanism of coastal bluffs on the North Slope (i.e., north coast) of Alaska. The coarse sediment areal density is the dry mass (g) of coarse sediment (sand) per horizontal area (cm2) in the coastal bluff. When the coarse sediment areal density exceeds 120 g cm−2, the bluff face thaw/slump erosion mechanism is dominant. When the coarse sediment areal density is below 80 g cm−2, the niche erosion/block collapse erosion mechanism is dominant. The coarse sediment areal density also has some influence on coastal erosion rates. Considering the 22 sites addressed in this study, the sediment areal density was found to have a controlling effect on erosion rate. Using erosion rates for the 1980–2000 period, when the sediment areal density exceeds 120 g cm−2, the average erosion rate was of 0.34
Data Availability Statement
The original contributions presented in the study are included in the article/Supplementary Material, further inquiries can be directed to the corresponding author.
Author Contributions
TR conceived the overall manuscript and did the majority of the writing. SP did the analysis of the aerial photos and sediment data.
Funding
Funding for this research came from the National Science Foundation (Award # 7416374) and from the Bureau of Ocean Energy Management (NSL# AK-17-01).
Conflict of Interest
The authors declare that the research was conducted in the absence of any commercial or financial relationships that could be construed as a potential conflict of interest.
Publisher’s Note
All claims expressed in this article are solely those of the authors and do not necessarily represent those of their affiliated organizations, or those of the publisher, the editors and the reviewers. Any product that may be evaluated in this article, or claim that may be made by its manufacturer, is not guaranteed or endorsed by the publisher.
References
Baranskaya, A., Novikova, A., Shabanova, N., Belova, N., MaznevJones, S. B., Ogorodov, S., et al. (2021). The Role of Thermal Denudation in Erosion of Ice-Rich Permafrost Coasts in an Enclosed Bay (Gulf of Kruzenstern, Western Yamal, Russia). Front. Earth Sci. 8, 2021. doi:10.3389/feart.2020.566227
Barnhart, K. R., Anderson, R. S., Overeem, I., Wobus, C., Clow, G. D., and Urban, F. E. (2014a). Modeling Erosion of Ice-Rich Permafrost bluffs along the Alaskan Beaufort Sea Coast. J. Geophys. Res. Earth Surf. 119, 1155–1179. doi:10.1002/2013jf002845
Barnhart, K. R., Overeem, I., and Anderson, R. S. (2014b). The Effect of Changing Sea Ice on the Physical Vulnerability of Arctic Coasts. The Cryosphere 8, 1777–1799. doi:10.5194/tc-8-1777-2014
Carter, C. H., and E. Guy, D. (1988). Coastal Erosion: Processes, Timing and Magnitudes at the bluff Toe. Mar. Geology. 84, 1–17. Available at: https://pubs.er.usgs.gov/publication/70014360. doi:10.1016/0025-3227(88)90121-1
Frederick, J. M., Thomas, M. A., BullJones, D. L. C. A., and Roberts, J. (2016). “The Arctic Coastal Erosion Problem,”. SAND2016-9762. doi:10.2172/1431492
Gibbs, A. E., Erikson, L. H., Jones, B. M., and Richmond, B. M. (2010). Characterizing Morphology and Erosional Trends of Permafrost Bluffs, Barter Island. Alaska: AGU Fall Meeting. EP23A-0772.
Gibbs, A. E., and Richmond, B. M. (2009). Oblique Aerial Photography of the Arctic Coast of Alaska, Nulavik to Demarcation Point, August 7 -10, 2006. U.S. Geol. Surv. Data Ser. 436 6 (databases), 4. Available at: http://pubs.usgs.gov/ds/436/. doi:10.3133/ds436
Gibbs, A. E., Richmond, B. M., Palaseanu-Lovejoy, M., Erikson, L. H., Jones, B. M., and Brock, J. (2013). Remote Sensing of the Arctic Coast of Alaska Using Airborne Lidar Data. San Francisco, CA: American Geophysical Union Fall Meeting. B51H-0406.
Gibbs, A. E., and Richmond, B. M. (2015). National Assessment of Shoreline Change: Historical Change along the north Coast of Alaska, U.S.-Canadian Border to Icy Cape. Geol. Surv. Open-File Rep. 2015 1048, 96. doi:10.3133/ofr20151048
Hoque, M. A., and Pollard, W. H. (2016). Stability of Permafrost Dominated Coastal Cliffs in the Arctic. Polar Sci. 10, 79–88. doi:10.1016/j.polar.2015.10.004
Jones, B. M., Arp, C. D., Jorgenson, M. T., Hinkel, K. M., Schmutz, J. A., and Flint, P. L. (2009). Increase in the Rate and Uniformity of Coastline Erosion in Arctic Alaska. Geophys. Res. Lett. 36, L03503. doi:10.1029/2008gl036205
Kobayashi, N. (1985). Formation of Thermoerosional Niches into Frozen bluffs Due to Storm Surges on the Beaufort Sea Coast. J. Geophys. Res. 90 (C6), 11983–11988. doi:10.1029/jc090ic06p11983
Mars, J. C., and Houseknecht, D. W. (2007). Quantitative Remote Sensing Study Indicates Doubling of Coastal Erosion Rate in Past 50 Yr along a Segment of the Arctic Coast of Alaska. Geol 35 (7), 583–586. doi:10.1130/g23672a.1
Ping, C.-L., Michaelson, G. J., Guo, L., Jorgenson, M. T., Kanevskiy, M., Shur, Y., et al. (2011). Soil Carbon and Material Fluxes across the Eroding Alaska Beaufort Sea Coastline. J. Geophys. Res. 116, G02004. doi:10.1029/2010JG001588
Ravens, T., Kartezhnikova, M., Ulmgren, M., Yager, G., Jones, B., Erikson, L., et al. (2011). Arctic Coastal Erosion Modeling. San Francisco, CA: Presented at the American Geophysical Union Fall Meeting.
Ravens, T. M., Jones, B. M., Zhang, J., Arp, C. D., and Schmutz, J. A. (2012). Process-based Coastal Erosion Modeling for Drew Point, North Slope, Alaska. J. Waterway, Port, Coastal, Ocean Eng. 138 (2), 122–130. doi:10.1061/(asce)ww.1943-5460.0000106
Ravens, T. M., and Peterson, S. (2018). “Arctic Coastal Erosion Modeling,” in A Chapter in Advances In Coastal Hydraulics. Editors P. Vijay, and K. Jim (London Singapore: World Scientific). doi:10.1142/9789813231283_0002
Ravens, T., and Ulmgren, M. (2020). Arctic Coastal Geomorphic Change Modeling with Arctic Xbeach. Anchorage, AK: AGU Fall Meeting.
Keywords: arctic, coastal erosion, mechanism, coarse sediment, areal density
Citation: Ravens TM and Peterson S (2021) Geologic Controls on Erosion Mechanism on the Alaska Beaufort Coast. Front. Earth Sci. 9:693824. doi: 10.3389/feart.2021.693824
Received: 12 April 2021; Accepted: 24 September 2021;
Published: 15 October 2021.
Edited by:
Scott Raymond Dallimore, Geological Survey of Canada, CanadaReviewed by:
Henry Patton, Arctic University of Norway, NorwayJennifer Frederick, Sandia National Laboratories, United States
Copyright © 2021 Ravens and Peterson. This is an open-access article distributed under the terms of the Creative Commons Attribution License (CC BY). The use, distribution or reproduction in other forums is permitted, provided the original author(s) and the copyright owner(s) are credited and that the original publication in this journal is cited, in accordance with accepted academic practice. No use, distribution or reproduction is permitted which does not comply with these terms.
*Correspondence: Thomas M. Ravens, tmravens@alaska.edu
†ORCID: Thomas M. Ravens, orcid.org/0000-0002-4613-4632
‡Present address: Sasha Peterson, Department of Environmental Science and Engineering, University of Texas at El Paso, El Paso, TX, United States