- Geography, Global Systems Institute, College of Life and Environmental Sciences, University of Exeter, Exeter, United Kingdom
Dansgaard-Oeschger oscillations (DOs) are abrupt shifts in climate, which are dramatic temperature fluctuations observed in Greenland and recorded globally. These abrupt changes are associated with the slowing and shutting down of the Atlantic Meridional Overturning Circulation (AMOC), but despite their importance the driving forces of DOs are not fully understood. Here we assess the role of the AMOC during DOs, the Northern vs Southern Hemisphere control on AMOC, and the possibility of neotropical moisture as a driver for abrupt climate variability. During DOs, South America has recorded a disparity between the degree of warming, and the change in precipitation at different sites. Based on our current understanding, we propose likely oceanic and continental changes in tropical South America that can help disentangle the triggers of these events. With the margins of error associated with dating sources of palaeo-data, the need for an independent chronology with multiple proxies recorded in the same record, could offer the information needed to understand the driving forces of DOs.
Introduction
Global climate change in the 21st century is the largest challenge humanity has faced (IPCC, 2014) and is already resulting in extreme weather, reduced food security, and threats to biodiversity (Ripple et al., 2017). In spite of this urgency, our ability to make reliable predictions for the future is still hampered by our limited understanding of the climate system. We need accurate knowledge on natural climate variability to comprehend how human activities impact climate. We are yet to understand the natural triggers of rapid climate variability and how regional abrupt climate shifts can modify the global climate (Hodell and Channell, 2016; Sánchez Goñi et al., 2018).
Abrupt climate fluctuations have interrupted the baseline climate conditions of the Quaternary and are termed Dansgaard-Oeschger oscillations (DOs). These abrupt events are global, being recorded in diverse locations across both hemispheres, from Greenland and the North Atlantic to South America and Antarctica (Blunier and Brook, 2001; Correge et al., 2004; Harrison and Sanchez Goñi, 2010; Wolff et al., 2010; Zhang et al., 2015; Markle et al., 2017). DOs may induce glacial-interglacial transitions (Berger, 1992; Marino et al., 2015) and are linked to large fluctuations in global CO2 (Stauffer et al., 1998) and CH4 (Wolff et al., 2010). Abrupt climate events are also of critical interest because their magnitude and speed (Wolff et al., 2010; Orsi et al., 2014) correspond to climate projections under anthropogenic forcing (IPCC, 2014). Understanding DOs is therefore essential to constrain climate sensitivity (Rohling et al., 2012), improve our ability to predict future climate change (von Deimling et al., 2006; Collins et al., 2013), and to understand how climate perturbations in one region may affect climate in other parts of the world.
In this article we assess the potential of two main hypotheses for driving DOs: the changes to ocean circulation from the North Atlantic and the tropical moisture source from the equatorial Atlantic. South America has largely been understudied in research into DOs, resulting in a lack of data for the tropical moisture hypothesis. By looking at the modern South American climate, it is possible to explore how these climatic controls influence abrupt climate variability. Then we assess the critical knowledge gaps in our understanding of abrupt climate variability, and how the tropical trigger hypothesis could be tested using marine sediments from the tropical Atlantic.
Abrupt Climate Variability
Dansgaard-Oeschger oscillations consist of rapid warming events followed by gradual cooling (Figure 1A) and occur during glacial and deglacial periods. In the Greenland ice core record, DOs are recorded as dramatic shifts of Northern Hemisphere temperatures (Wolff et al., 2010; Figure 1A). During the warming events, Greenland temperatures can increase by 7 to 16°C in a few decades (Wolff et al., 2010) and are linked to major changes in ocean circulation and the configuration of tropical rain belts and monsoon systems (Broccoli et al., 2006; Clement and Peterson, 2008; Cruz et al., 2009; Gottschalk et al., 2015). DOs have a global impact, but the magnitude and direction of change can be regionally variable (Sánchez Goñi and Harrison, 2010; Wolff et al., 2010). For example, more humid conditions are observed in the Mediterranean (Budsky et al., 2019), precipitation changes occur in South America (Zhang Y. et al., 2017) and East Africa (Brown et al., 2007), the monsoonal system intensifies in Asia (Li et al., 2017; Dong et al., 2018; Liang et al., 2019) and shifts in northwest Africa (Itambi et al., 2009), drying occurs in northern Australia (Muller et al., 2008) and Indonesia (Griffiths et al., 2013), and while other records suggest very little or no climatic fluctuations occurred during DOs (Mulitza et al., 2007; Hessler et al., 2010).
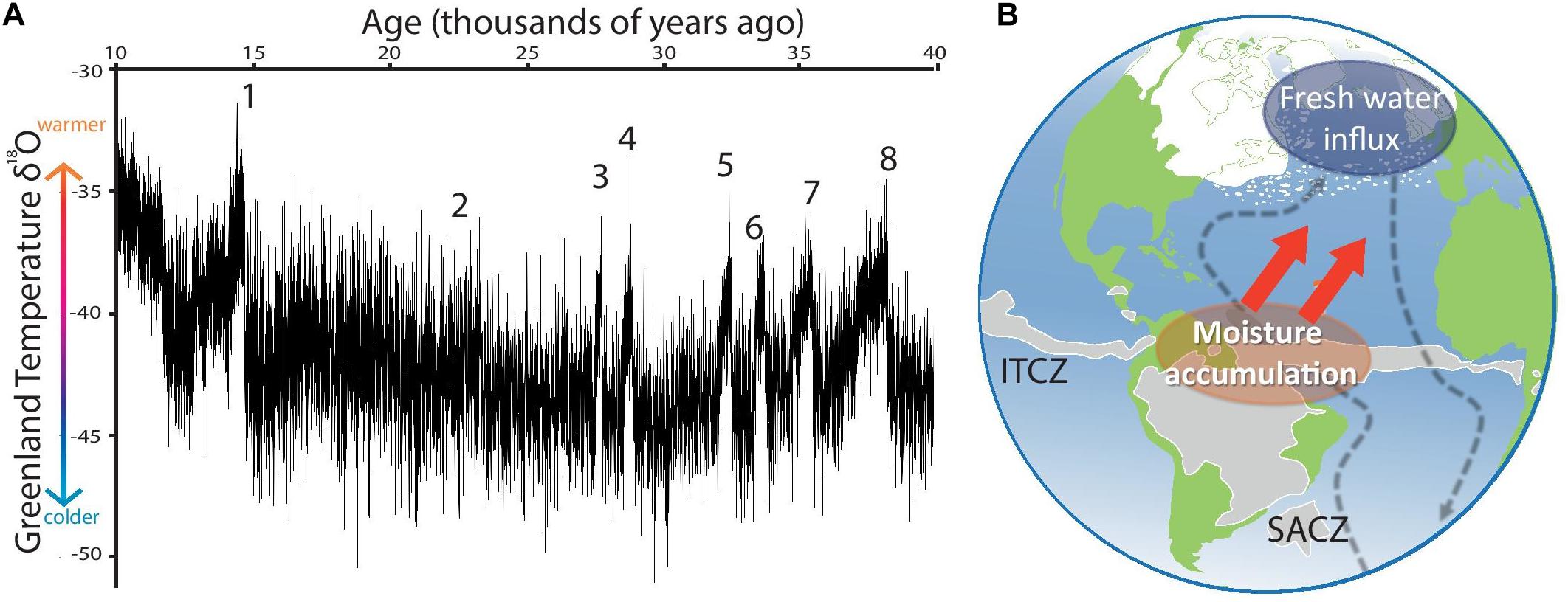
Figure 1. (A) Greenland ice core record and abrupt climate events (DOs) over the last 40,000 years (Andersen et al., 2004, 2006; Svensson et al., 2006; Vinther et al., 2006; Gkinis et al., 2014). Numbers indicate the onset of the last 8 DO events. (B) Contrasting hypotheses of DO triggers. Dashed lines indicate the Atlantic Meridional Overturning Circulation. Solid lines represent the hypothesised atmospheric transport from the American tropics to the North Atlantic.
Trigger Hypotheses of Abrupt Climate Events
The origin and distribution mechanisms of abrupt climate variability remains enigmatic despite their global importance. One hypothesis is that these events are triggered in high latitudes by interruptions to oceanic circulation. An influx of freshwater into the North Atlantic causes changes in the Atlantic Meridional Overturning Circulation (AMOC), which then distributes the climate perturbations to the rest of the planet (Figure 1B; Ganopolski and Rahmstorf, 2001; Clark et al., 2002; Kageyama et al., 2010). The AMOC is part of the temperature and salinity driven thermohaline circulation (Broecker, 1991). As warm surface waters migrate northwards, heat is released into the atmosphere, warming more northerly latitudes.
When freshwater, in the form of sea ice from ocean-cryosphere interactions, is injected into the North Atlantic, the density of the surface water is reduced, slowing downwelling, and slowing or shutting down the AMOC. With the AMOC slowed, global heat dispersal is reduced, and cooling at higher latitudes occurs (Heinrich, 1988; Bassis et al., 2017; Vettoretti and Peltier, 2018). An increase in freshwater release in the North Atlantic leading to a decrease in North Atlantic deep water formation is also captured by models (Ganopolski and Rahmstorf, 2001; Vettoretti and Peltier, 2018). The growth and collapse of an ice shelf could result in sea ice expansion, disturbing the AMOC and driving in the interstadial/stadial phases of the DOs cycles (Li et al., 2010, Li and Born, 2019; Petersen et al., 2013). Alternative drivers of AMOC instability have also been suggested. Ice surges from the Fennoscandian ice sheet may be a likely freshwater source (Dokken et al., 2013) during some DO cycles, while sea ice from Laurentide ice sheet may dominate others (Sánchez Goñi et al., 2020). Changes in the Southern Hemisphere have also been suggested to drive AMOC stability (Jaeschke et al., 2007; Buizert and Schmittner, 2015; Dima et al., 2018). Empirical data from obtained off the northeast Brazil coast coupled with model experiments also suggest that warming in the Southern Ocean and links with the South Atlantic western boundary current subsequently led to an abrupt restart of the AMOC during deglaciation (Jaeschke et al., 2007). The Southern Ocean can influence the AMOC by changes in the Antarctic Bottom Water formation, Atlantic density and pressure gradients, warm buoyant water entering the Southern Ocean and upwelling in the Southern Ocean (Buizert and Schmittner, 2015). Changes in these factors can lead to slowing down of the AMOC.
An alternative driver for DOs is a deep-decoupling oscillation within the North Atlantic (Schulz et al., 2002). Linked to surface water freshening, deep water formation fails to form and enters a deep-decoupled phase, with a reduced AMOC and less heat transfer. Import of heat and salt by advection and diffusion weakens and breaks down the halocline. This leads to convection and triggers a deep-coupled phase in the North Atlantic, resulting in deep water formation, and a strong AMOC and greater heat transfer (Schulz et al., 2002).
While shutting down of the AMOC is most accepted as the trigger of abrupt climate variability, discrepancies between model and proxy data suggest that our understanding of these events is still incomplete (Mecking et al., 2017; Goes et al., 2019; Gu et al., 2020). Some studies have shown disparate responses to different DOs, for example the Santos Basin shows DO signals during Marine Isotope Stage (MIS) 5 but absent or dampened millennial scale climate variability during MIS 3 (Santos et al., 2020). Modelling work also suggests that shutting down of AMOC and tropical SST changes and moisture accumulation are teleconnected (Lohmann, 2017, Zhang X. et al., 2017). In fact, some model simulations show that tropical climate perturbations could indeed precede, and potentially trigger, changes in the North Atlantic (Rodgers et al., 2003). Further understanding of these ocean-atmosphere teleconnections through combinations of empirical data and models could help in gaining a greater understanding of AMOC responses and the triggers of abrupt climate variability.
The propagation of abrupt climate events is another topic yet to be fully understood. Freshwater input into the North Atlantic can disturb the AMOC and produce climate shifts from cold to warm (Lippold et al., 2012) that then spread over the entire planet. However, it can take about 200 years for the oceans to redistribute heat from one hemisphere to the other (WAIS Divide Project Members, 2015), while the atmosphere (Markle et al., 2017) could allow for a quick transfer to distant parts of the world. This suggests that the AMOC might play a lesser role in transmitting DOs and that these events might originate outside the North Atlantic. Moreover, MIS 19 (800,000 years ago) is a period marked by abrupt climate shifts in the North Atlantic region, but these early shifts are not linked to AMOC perturbations (Sanchez Goñi et al., 2016). Instead, they may be explained by increased moisture transport from the tropical Atlantic to high latitudes (Sanchez Goñi et al., 2016), suggesting a low-latitude trigger. Remarkably, a tropical trigger for abrupt climate variability has hardly been explored (Arz et al., 1998; Cane and Clement, 1999; Clement and Peterson, 2008).
The low latitudes could trigger abrupt climate events because the tropics receive maximum solar irradiation and can become hotspots of heat accumulation due to orbital fluctuations (Berger et al., 2006). Precession and eccentricity are the primary orbital parameters modulating insolation or the amount of solar energy reaching the top of the atmosphere at the low latitudes (Laskar, 1990; Ruddiman, 2006). Phase coupling between precession, semiprecession and eccentricity, which originates in the tropics, have been essential in maintaining the ice sheet oscillations for the past 1.5 million years (Hagelberg et al., 1994; Rutherford and D’Hondt, 2000). Insolation changes linked to eccentricity and the precession harmonics can cause a decrease in the seasonal variations of equatorial insolation (McIntyre and Molfino, 1996; Berger et al., 2006). This may induce heat accumulation in the tropical regions and result in potential moisture build-up. Previous work has linked insolation and moisture availability in western Amazonia (Urrego et al., 2010), however, the paucity of other regional records has hindered the identification of a moisture source. A solar cycle combined with two much shorter freshwater cycles, has been modelled to produce the response observed in DOs (Braun et al., 2005). Coupled atmospheric-ocean models have shown that gradual increases in tropical CO2 can trigger abrupt warming and cooling events (Zhang X. et al., 2017), also supporting a tropical origin for these abrupt events.
Extra warmth in the tropics relative to the high latitudes may also enhance the latitudinal thermal gradient, strengthening, and shifting the westerlies northwards (Sanchez Goñi et al., 2016; Markle et al., 2017). Abrupt climate events have also been linked to the strength and latitudinal reach of tropical winds and the direct advection of low-latitude surface water heat to the North Atlantic (McIntyre and Molfino, 1996; Venancio et al., 2018). Reduced insolation seasonality and increased heat accumulation in the American tropics could therefore trigger abrupt climate events through (i) moisture accumulation from an enlarged land-sea thermal contrast, and (ii) increased moisture transport toward the North Atlantic from an amplified latitudinal thermal gradient and northward penetration of latent heat (Figure 1B).
An alternative scenario where abrupt climate changes are triggered by heat and moisture build-up in the understudied tropical regions and where both the ocean and atmosphere propagate these climate disruptions to the high latitudes is therefore likely (Arz et al., 1998; Cane and Clement, 1999; Jennerjahn et al., 2004; Berger et al., 2006; Clement and Peterson, 2008; Figure 1B). In fact, freshwater input in the North Atlantic is linked to iceberg discharges and ice growth (Ruddiman, 2006), but ice sheets necessitate moisture to grow. Moisture increases are recorded in Greenland preceding ice sheet growth (Steffensen et al., 2008; Sánchez Goñi et al., 2018), with suggestions that this is sourced from the North Atlantic (Nusbaumer et al., 2019). The tropical regions could be an alternative source for that moisture. The western tropical Atlantic is an important hot water reservoir of the global ocean conveyor (Wang and Enfield, 2001); and maximum solar irradiance linked to isolation cyclicity (Berger et al., 2006; Sánchez Goñi et al., 2018) make the American tropics a likely location for moisture build-up. Indeed, cooling in the high latitudes is suggested to be reinforced by tropical Atlantic warming and redistribution of heat in the surface of the oceans during the Cenozoic (Tremblin et al., 2016). Model studies have shown that SST changes in the tropical oceans can be linked to temperature changes in the Northern Hemisphere via atmospheric teleconnections (Rodgers et al., 2003; Lohmann, 2017; Zhang X. et al., 2017). Increases in tropical SST results in warming of Canada during the Boreal Summer and melting of the Laurentide Ice Sheet (Rodgers et al., 2003). Orbitally-driven heat accumulation and moisture build up in the tropics may therefore cause rapid global redistributions of warm waters and moisture, potentially triggering these rapid and large climate shifts.
One key area of research which could help understand whether tropical moisture injection or changes in ocean circulation can drive abrupt climate change, is to look at the timing of events. In the tropical moisture hypothesis, ice sheet formation would occur and, as the ice sheet grew, cooling would follow. Alternatively, if changes in ocean circulation are the driving forces, cooling would precede ice sheet growth. In support of the tropical moisture hypothesis, dust studies in the Greenland ice core suggest that changes to the low latitude atmospheric circulation precede the Greenland temperature change (Steffensen et al., 2008).
The Paleoclimate Modelling Intercomparison Project (PMIP) has explored various possible drivers for DOs. Some results suggest North Atlantic sea ice anomalies are important for creating the climate signal associated with DOs (Knorr et al., 2009; Li et al., 2010; Li and Born, 2019). Other results suggest North Atlantic sea ice brine rejection is a driving force on the stability of AMOC and DOs (Wang and Mysak, 2006). However, ocean temperatures likely influence the sea ice dynamics, and thus are the driving forces of DOs (Alvarez-Solas et al., 2010; Barker et al., 2015). Gradual oceanic cooling results in a non-linear climatic response, with sea ice acting as a positive feedback mechanism (Barker et al., 2015). Alternatively, the North Pacific has been suggested as a driver for ice sheet growth during DOs (Kiefer et al., 2002). Warm SST in the North Pacific provide moisture to East Greenland, replenishing ice sheets associated with DOs (Kiefer et al., 2002).
A tropical source for DOs has also been modelled by PMIP. Warming of tropical SST from boundary glacial conditions could result in changes to extratropical moisture and temperature. These changes in temperature and precipitation could result in the melting of ice sheets (Rodgers et al., 2003). The suggestion of a tropical methane source for driving DOs is unsupported by PMIP modelling. Simulations with two wetland models of different complexity show that tropical methane from wetlands alone is not enough to drive DOs, and that either missing wetland processes in the models are required, other methane sources are needed, or an alternative mechanism unrelated to methane release is required to explain DOs (Ringeval et al., 2013).
There is some uncertainty on whether current models can effectively represent the pathways driving DOs, as there is currently no PMIP common guidance for investigating DOs (Sime et al., 2021). Further developments into models for DOs and similar climatic events would provide further insights into this climate variability. Overall, we are yet to fully understand to what extent ocean-atmosphere interactions in the tropics can induce global climate shifts and whether they precede a fresh water influx in the North Atlantic.
Ocean-Atmosphere Interactions in Tropical South America
Understanding the current and past configuration of atmospheric and oceanic interactions in tropical South America is crucial to assess a tropical trigger for DOs. Several atmospheric mechanisms dominate and could influence moisture build up in this region. The Intertropical Convergence Zone (ITCZ) is a belt of warm, moist air that sits on the thermal equator, shifting seasonally to the warmer hemisphere (Nobre et al., 2012; Schneider et al., 2014). Similarly, the South Atlantic Convergence Zone (SACZ) is a precipitation band located over eastern Brazil and the South Atlantic which shifts with changes in sea surface temperature (SST; Chaves and Nobre, 2004; Cruz et al., 2005; Jorgetti et al., 2014). Warm South Atlantic SST cause the SACZ to intensify and migrate to a more northerly position (Chaves and Nobre, 2004; De Almeida et al., 2007). However, SACZ is further influenced by interactions between solar radiation (Nobre et al., 2012) and intraseasonal anomalies in outgoing longwave radiation within the American tropics (Carvalho et al., 2004). The SACZ can result in a negative feedback response, as increased cloud cover dampens the initial SST warming by decreasing incoming solar radiation (Venancio et al., 2020). The South American Monsoon System (SAMS) controls where precipitation occurs on the South American continent, influenced largely by the ITCZ and the SACZ but also influenced by Atlantic SST (Mechoso et al., 1990; Garreaud et al., 2009), trade winds (Garreaud et al., 2009), and Amazonian evapotranspiration (Trenberth, 1999). These ocean-atmosphere systems have been suggested to shift during the Quaternary due to interhemispheric temperature fluctuations (Peterson et al., 1991; Broccoli et al., 2006; Correa-Metrio et al., 2012; McGee et al., 2014). As atmospheric mechanisms respond to abrupt climate shifts in the Northern Hemisphere and oceans, the location of tropical precipitation bands would rearrange. If cooling occurs in the Northern Hemisphere, the ITCZ would move southward, and areas in the north of the current ITCZ location will receive less precipitation while areas to the south would receive more (Behling et al., 2000). Additionally, during Northern Hemisphere cold periods, the South Atlantic would warm and SACZ intensify (Stríkis et al., 2015). The intensified SACZ and more southerly ITCZ results in the intensification of the SAMS (Stríkis et al., 2015).
If tropical moisture accumulation was a trigger for abrupt warming and cooling events in the Northern Hemisphere, changes in precipitation patterns could result in negative feedback loops. Warming would intensify the SACZ and the more northerly position of the ITCZ would result in increased moisture accumulation in the tropical Atlantic. This in turn could result in more moisture being transported northward to the colder Northern Hemisphere where ice sheets would grow due to increased precipitation. Northern Hemisphere cooling would then occur, followed by changes to the AMOC. As the interhemispheric temperature differences increased, the ITCZ would then move southward again, resulting in less precipitation in the equatorial Atlantic. This reduction of moisture accumulation would result in less moisture reaching the ice sheets, halting ice growth.
An Unclear Picture of Abrupt Climate Variability in the American Tropics
Existing empirical data (Figure 2) show a range of environmental fluctuations in the American tropics during DOs, but integrating these into a consistent regional interpretation of abrupt climate variability remains notoriously difficult (Urrego et al., 2014). For moisture availability, studies have shown that some DOs are associated with decreased precipitation in western Amazonia (Urrego et al., 2010), while increased humidity is recorded in the Bolivian Altiplano (Baker et al., 2001; Paduano et al., 2003), and in offshore French Guyana (Zhang Y. et al., 2017). Speleothem records suggest a weakened South American monsoon and reduced precipitation during some DOs in subtropical Brazil (Cruz et al., 2005), but record no change during others in lowland Ecuador (Mosblech et al., 2012). DOs are also linked to increased precipitation and continental run-off in the Cariaco Basin (Peterson and Haug, 2006), and with decreased run-off in NE Brazil (Arz et al., 1998).
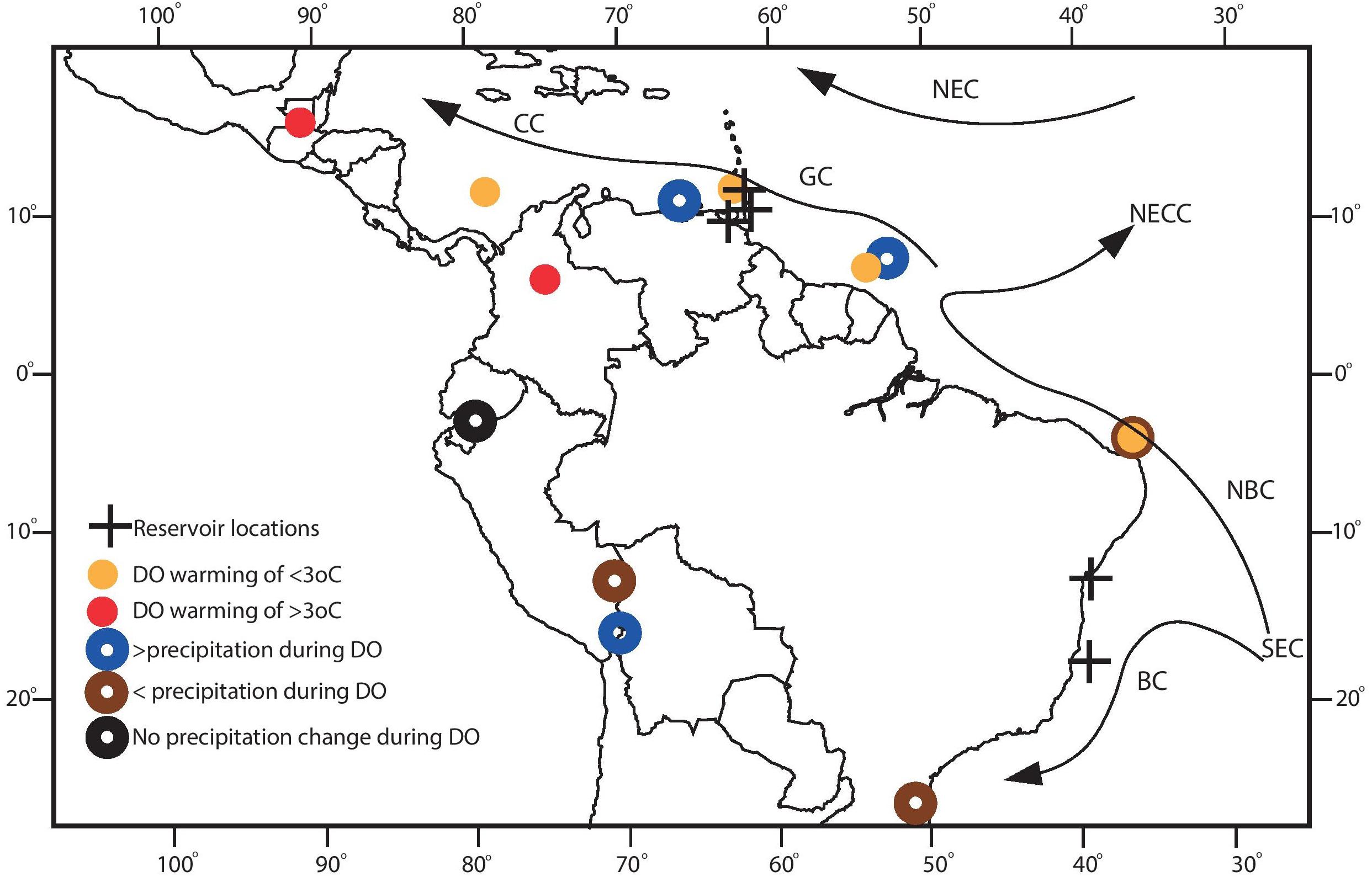
Figure 2. Warming and precipitation changes in South America during DOs showing the continental masses warming considerably more than the oceans. The location of ages used for regional reservoir corrections referred in Table 1. Precipitation shows contrasting responses across the continent. Modern currents have been added (CC, Caribbean Current; GC, Guyana Current; NEC, North Equatorial Current; NECC, North Equatorial Counter-current; NBC, North Brazil Current; and SEC, South Equatorial Current).
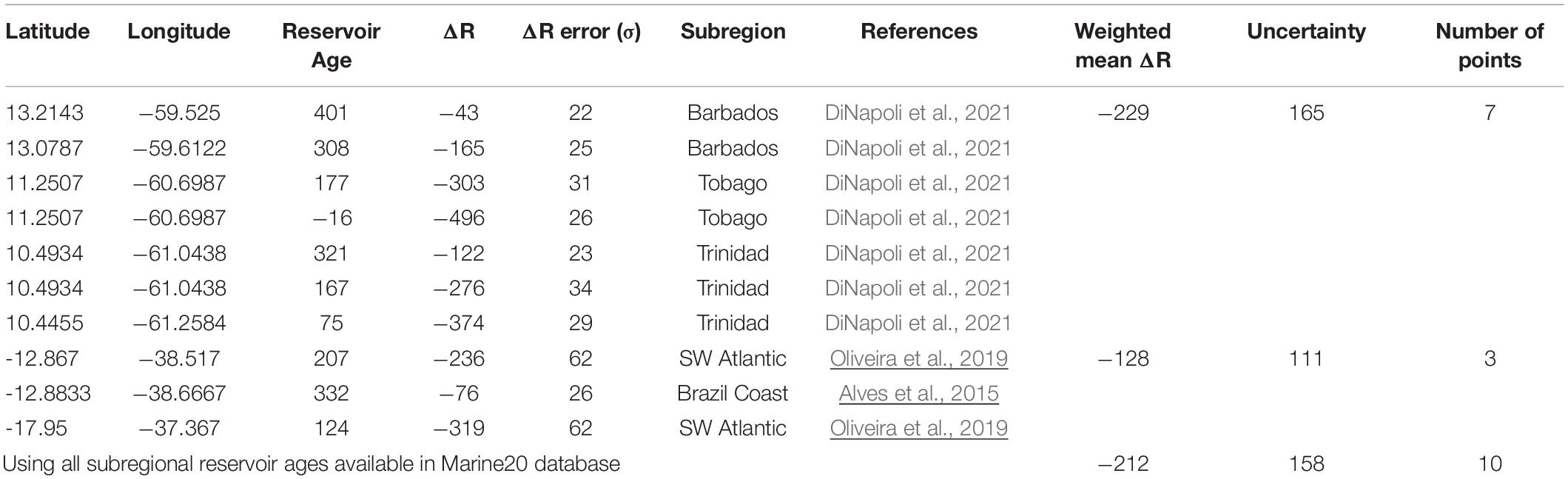
Table 1. Regional reservoir ages and corrections from the Caribbean and western tropical Atlantic extracted from the Marine20 database (Stuiver et al., 2021).
The migration of Andean vegetation during DOs has been shown to translate into air temperature changes which are consistent with ice-core records from tropical glaciers and tropical Atlantic surface water temperatures (Urrego et al., 2016), but the magnitude of temperature change in the atmosphere differs from that of the tropical Atlantic. Air temperature change from pollen records in the Central American lowlands (Correa-Metrio et al., 2012) and the Colombian Andes (Bogota-a et al., 2011; Groot et al., 2011) indicates warming between 3 and 13°C during DOs (Figure 2). Contrastingly, the magnitude of tropical Atlantic surface water warming remains minor compared to temperature change estimates over land, with a SST rise of 1°C recorded in the Tobago Basin (Rühlemann et al., 1999), 2°C in the Colombian Basin (Schmidt et al., 2004), and 2.6°C in the Guyana Basin (Rama-Corredor et al., 2015).
Overall, available palaeoclimate estimates for the American tropics are scarce (Hessler et al., 2010) but suggest significant land-sea temperature contrasts and atmospheric reorganisations that could result in moisture build-up. However, atmospheric reconstructions from speleothems and lakes are not directly comparable to oceanic changes measured in marine sediments because each relies on a different chronology with varying age uncertainties. This hinders simultaneous comparisons of air and sea temperatures to quantify land-sea thermal contrasts and identify moisture build-ups.
Ocean circulation also shifts during abrupt climate events. During periods of Northern Hemisphere cooling, the North Brazil Current will weaken during the initial cooling, continuously weakening as the ITCZ migrates southward (Arz et al., 1999; Bahr et al., 2018). Synchronously, the North Equatorial Countercurrent will intensify with southward migrations of the ITCZ (Wilson et al., 2011). These changes in the North Brazil Current and the North Equatorial Countercurrent, alongside the southward displacement of the ITCZ, result in the southward migration of the North Equatorial Current (Vink et al., 2001). An intensified North Equatorial Countercurrent will also decrease Guyana Current flow (Richardson et al., 1994).
Predicted Response to Abrupt Climate Change in Tropical South America
We summarise here the expected changes in vegetation, fire regimes, ocean currents and atmospheric systems in tropical South America during abrupt climate events (Figure 3). During periods of Northern Hemisphere cooling, a southward position of the ITCZ and intensified SACZ would result in decreased precipitation within northernmost South America (Figure 3D). This would result in the expansion of dry forests and savannas, and increased precipitation in Brazil and Bolivia. Due to the cooling experienced in northernmost South America, montane forests would migrate downslope. Predicted fire activity will reflect changes in precipitation and vegetation. Due to the change in fuel and precipitation intensity, fire activity would increase in northern South America, and decrease in Brazil and Bolivia (Figure 3C). Within the oceans, the North Brazil Current will weaken and the North Equatorial Countercurrent will intensify. The decreased water supply from the North Brazil Current to the Guyana Current will be replaced by the North Equatorial Current, which is displaced southward (Figure 3D).
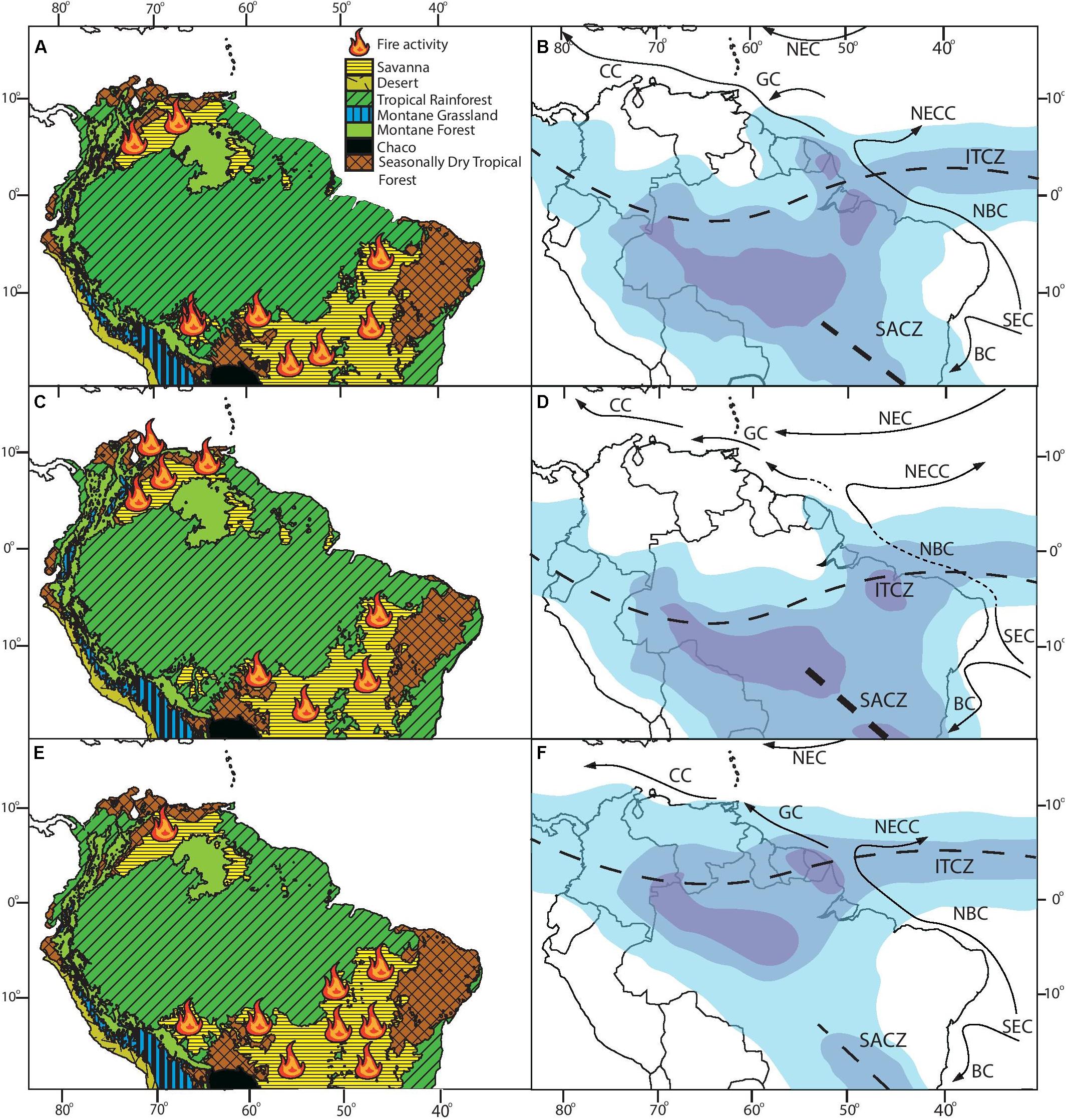
Figure 3. Predicted continental response of vegetation, ocean currents and precipitation during abrupt climate events. The (A) modern day vegetation and fire (adapted from Pennington and Lavin, 2016), (B) December-February (DJF) precipitation patterns (blue: darker blue indicates more intense precipitation; adapted from Stríkis et al., 2015) and ocean currents (arrows) in tropical South America (adapted from Vink et al., 2001). (C) Predicted vegetation and fire activity during periods of Northern Hemisphere cooling. (D) Predicted precipitation and ocean currents during Northern Hemisphere cooling. (E) Predicted vegetation and fire activity during Northern Hemisphere warming. (F) Predicted precipitation and ocean currents during Northern Hemisphere warming.
In contrast, during Northern Hemisphere warming, a more northward ITCZ and weakened SACZ would result in increased precipitation in northern South America (Figure 3F). This would result in the contraction of dry forests and savannas, and decreased precipitation in Brazil and Bolivia. Due to the warming experienced in northernmost South America, Andean forests will migrate upslope. Fire activity will decrease in northern South America due to changes in precipitation and fuel, and increase in the savannas of Brazil (Figure 3E). In the oceans, the North Brazil Current will intensify during Northern hemisphere warming, while the North Equatorial Countercurrent will weaken (Figure 3F).
Chronological Effects
A consistent identification of DOs outside the North Atlantic is still hampered by low-resolution chronologies, a lack of well-fingerprinted tephra for instantaneous dating, and poorly-constrained reservoir effects in marine records. The most updated regional reservoir corrections (Heaton et al., 2020) or ΔR (Stuiver and Braziunas, 1993; Reimer and Reimer, 2017) still rely on limited data points in the American tropics, including updated locations in the Caribbean (DiNapoli et al., 2021), and the west Atlantic off the Brazilian Coast (Alves et al., 2015; Oliveira et al., 2019). The weighted mean of regional differences in marine reservoir error can vary from −229 years using Caribbean ages to −128 years using ages off the Brazilian coast, while the uncertainty varies from 165 to 111 (Table 1). Even though these regional differences fall well within global ranges of −500 and 500 ΔR (Stuiver et al., 2021), they highlight regional variations in reservoir effects that can introduce further uncertainty to the chronology of short climate events such as DOs. Inconsistent chronologies between vegetation, speleothem, ice and marine records also hinder comparisons between records in the American tropics (Urrego et al., 2016; Adolphi et al., 2018). Recent studies on South American Uranium-Thorium (U/Th) dated speleothem records compared to the Greenland ice core record showed that South American abrupt events occur within the uncertainties of the Greenland core, though for some events dated shifts in speleothem records are at the limit of Greenland layer counting uncertainties (Adolphi et al., 2018). In the North Atlantic, unlike the American tropics, the chronology of DOs are well defined by layer counting in Greenland ice cores (Wolff et al., 2010), dating of loess records from western Europe (Moine et al., 2017), and direct dating of ice-rafted debris layers (Sánchez Goñi and Harrison, 2010).
The absence of such stratigraphic layers challenges the definition of a DO chronology for the American tropics (Urrego et al., 2014), and as a result available records adopt different chronologies and are not comparable. DO timing is correlated to the chronostratigraphy of the North Atlantic (McManus et al., 2004; Chiessi et al., 2009), the Iberian Peninsula chronology (Bard et al., 2000; Martrat et al., 2007; Sánchez Goñi and Harrison, 2010; Mollier-Vogel et al., 2013), and the Greenland chronology (Martrat et al., 2007; Correa-Metrio et al., 2012; Mosblech et al., 2012). In speleothem records, DO timing is defined as abrupt shifts in the oxygen isotope record (Cruz et al., 2005), which often involves large uncertainties from U/Th dating. Differences in chronological approaches result in age differences of up to 800 years for the timing of DOs and hinder any meaningful comparisons between records (Blaauw et al., 2010; Urrego et al., 2014).
The chronology of tropical records is sometimes tuned to Northern Hemisphere records eliminating the opportunity for analyses of synchronicity and asynchronicity (Figure 4). For instance, the Cariaco Basin sediments (Peterson and Haug, 2006) provide a unique tropical counterpart to high-latitude ice core records for rapid climate variability, however, their chronology is tuned to that of Greenland. This assumption of synchronicity between North Atlantic and tropical records invalidates any analysis of leads and lags (Urrego et al., 2014) and does not allow for hypothesis testing of the origin of DOs. Furthermore, the timing of oceanic changes cannot be assumed to apply to atmospheric change if the aim is to disentangle the role of the oceans vs the atmosphere in propagating abrupt climate events. A chronology that is independent of Greenland and North Atlantic records is therefore crucial to identify the trigger of abrupt climate events.
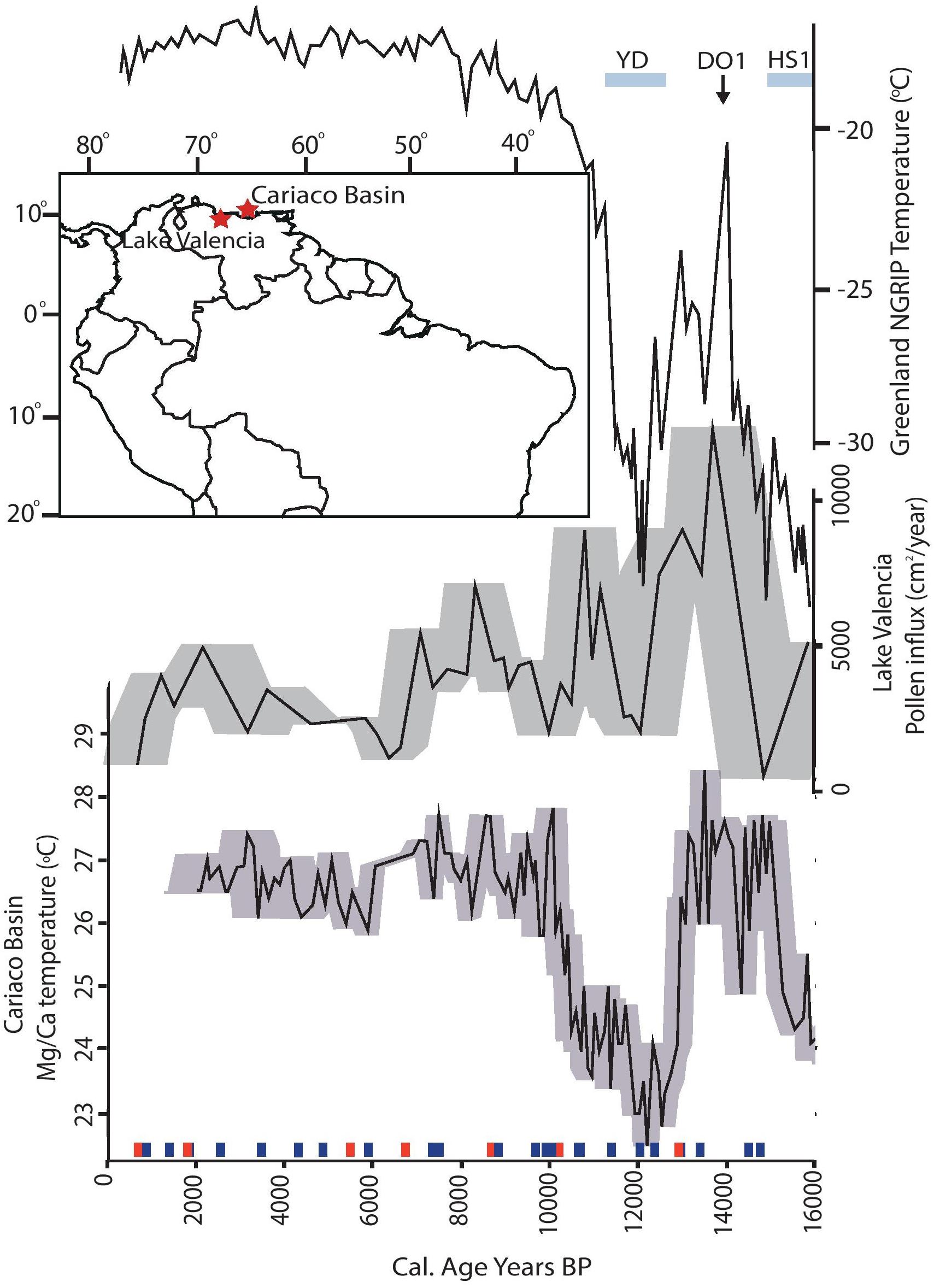
Figure 4. Effects of chronological uncertainties on two neighbouring continental and ocean records of abrupt climate changes. Top: Greenland NGRIP temperature reconstruction. Middle: vegetation change recorded in the pollen record of Lake Valencia (Bradbury et al., 1981). Bottom: sea surface Magnesium/Calcium temperature reconstruction from the Cariaco Basin (Lea et al., 2003), grey bands indicate the age ± error. Radiocarbon-dated intervals are represented by red (Bradbury et al., 1981) and blue (Lea et al., 2003) rectangles. Vertical bars indicate periods of warming and cooling using the North Atlantic chronology from Sánchez Goñi and Harrison (2010). DO1, Dansgaard-Oeschger event 1; YD, Younger Dryas; and HS1: Heinrich stadial 1.
A comparison between the Cariaco Basin and Lake Valencia records illustrates how different chronologies hinder the reconstruction of DOs signals in the American tropics (Figure 4). The Cariaco Basin provides a reconstruction of SST in the tropical Atlantic (Lea et al., 2003), while the pollen concentration from Lake Valencia (Bradbury et al., 1981) shows productivity on the vegetation linked to continental warming. The chronologies are derived from radiocarbon dating the two cores independently. The combined age uncertainty of the two records can lead to differences in interpretations of the DO signal. In the above example, the rise in pollen ∼14,000 years BP could be linked to a warming (BA), stable, or cooling climate, depending where you base data points within the margin of error. If using the median point for both, the rise in pollen concentration occurred with a 2°C rise in SST. If using the minimum point for both datasets, the increase in pollen occurs with a small rise of 0.5°C in SST. If using the maximum point for both, the pollen rise occurs with an increase in 3°C. This shows that by using two proxies from two different cores, interpretation of continental and oceanic responses may be inconclusive. In this example, a maximum in productivity of continental vegetation may be linked to a significant range of SST warming in the tropical Atlantic that derives only from the chronological uncertainties. These differences illustrate the challenges of reconstructing a coherent picture of DO signals in the tropics and the obstacles of identifying land-sea correlations that allow testing of alternative DO trigger hypotheses.
Testing the Tropical Trigger Hypothesis
To understand to what extent heat and moisture accumulation in the American tropics may trigger abrupt climate events and how the ocean and the atmosphere may transmit perturbations to the rest of the globe, we need to (i) quantify vegetation changes on land and across biomes to uncover fluctuations in the major atmospheric systems and potential moisture build-up, and (ii) quantify variations in surface water temperature and salinity along the Atlantic coast of South America to find potential evaporation sources, and to link surface water heat transport to major oceanic currents. Tropical vegetation records are ideal for tracking atmospheric change and moisture build-up because plants are highly sensitive to moisture availability (Phillips et al., 2009), and tropical biome boundaries (i.e., ecotones) are largely determined by precipitation (Ratter, 1992; Da Silveira and Lobo Sternberg, 2001). Atmospheric and oceanic records need to be high resolution to allow close investigation of these rapid and short climate events. A chronology that is independent from Greenland and North Atlantic records (Blaauw, 2012; Urrego et al., 2014) is also crucial to determine if climate changes in the American tropics precede climate perturbations in the North Atlantic region and are the likely triggers of abrupt climate events.
Integrated climate reconstructions from marine sedimentary sequences is therefore the best way to unravel terrestrial and oceanic changes in the American tropics during abrupt climate events (Figure 5). High-resolution terrestrial and marine tracers from the same sedimentary sequence can provide unique information to identify tropical moisture accumulation during DOs. Marine sediment sequences can be used to quantify land-sea climate contrasts and identify moisture build-ups. Marine sediments collected near continents are ideal as they provide high-quality records documenting past changes in the vegetation of the adjacent landmasses. They also contain marine indicators that can provide reconstructions of oceanic change (Sánchez Goñi et al., 2018). Terrestrial tracers include pollen and microcharcoal particles. Pollen documents vegetation changes that can be translated into air temperature, precipitation and rainfall seasonality. Charcoal particles document fluctuations in fire activity linked to vegetation type and precipitation. Marine tracers such as oxygen isotopes from benthic and planktonic foraminifera assemblages allow reconstructions of global ice volume and sea surface salinity (SSS), respectively. Additionally, organic compounds such as alkenones can be used to reconstruct SST and stratigraphic and sedimentological changes can be used to estimate changes in river runoff and wind intensity. All these marine and terrestrial tracers can be analysed from the same sample and thus same time slice (Figure 5) to achieve integrated ocean and atmospheric datasets. By quantifying terrestrial and marine indicators from the same sample, the reliance on external chronologies to determine leads and lags is minimised. Such an approach has proven invaluable to understand air and sea temperature coupling-decoupling in western Europe (Sanchez Goñi et al., 2013), the potential role of continental climates in the glacial inception (Desprat et al., 2005), orbital and millennial-scale changes in continental fires in western Europe (Daniau et al., 2007) and Southern Africa (Daniau et al., 2013), and Holocene land and ocean climates in the tropical Pacific (Seillès et al., 2016).
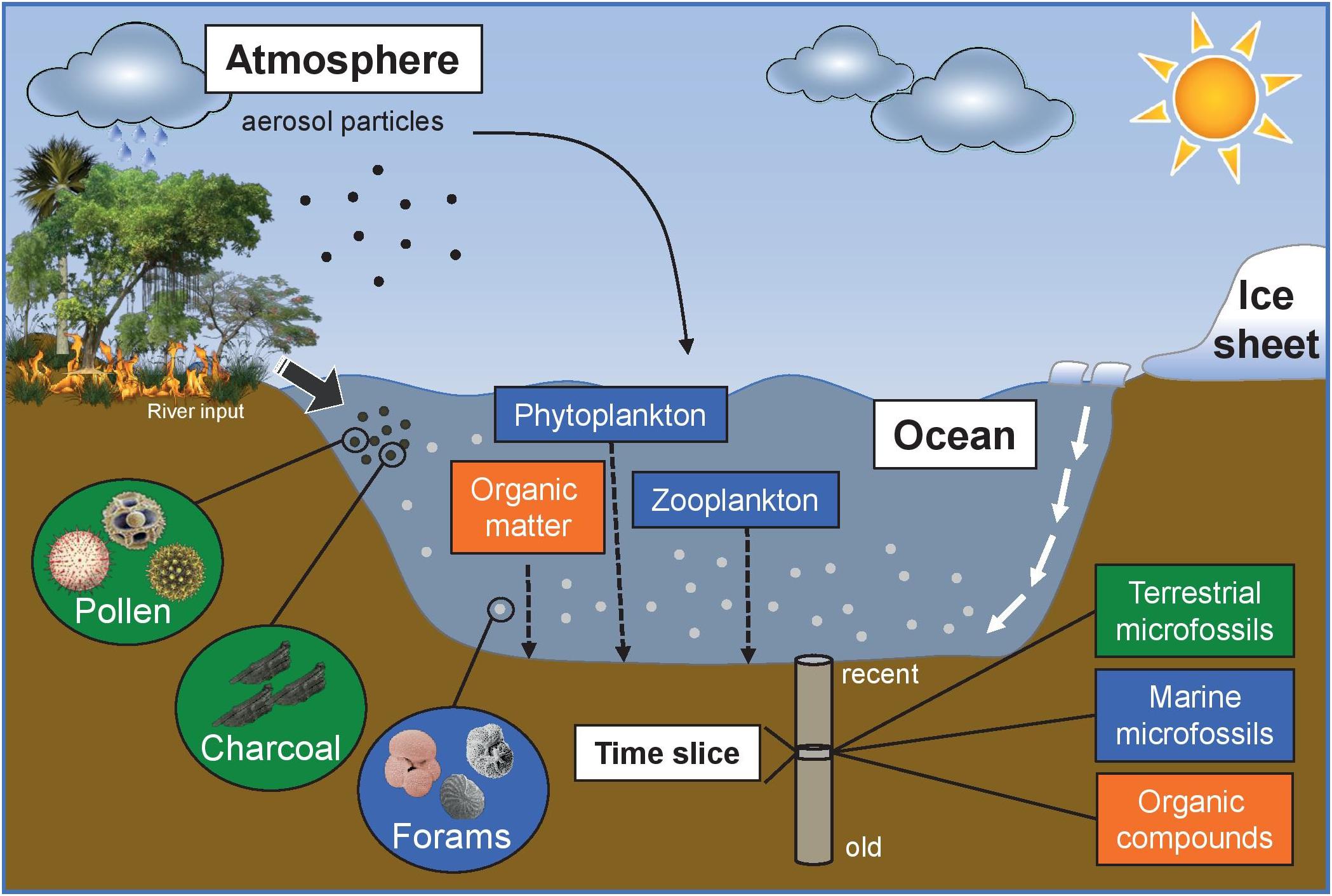
Figure 5. Integrated approach using marine sedimentary sequences to unravel terrestrial and oceanic changes during abrupt climate events and pathways of terrestrial and marine microfossil deposition. Forams: foraminifera. Modified from Center for Advanced Marine Core Research, Kochi University. (2020) https://www.kochi-u.ac.jp/marine-core/research/.
Conclusion
Despite abrupt climate events such as DOs being a global phenomenon, they are still poorly understood, especially how they are triggered. Several hypotheses have been suggested on what is driving changes in the ice sheets, and how changes outside the North Atlantic could trigger or propagate these events. The main two hypotheses for driving causes of DOs are changes to the AMOC in the North Atlantic and changes in tropical moisture accumulation and export. Modelling work suggests that these two mechanisms are linked and that tropical climate perturbations could indeed precede, and potentially trigger, changes in the North Atlantic.
Our projections of changes in vegetation and fire activity predict drying and fire increases in northernmost South America during Northern Hemisphere cooling, along with downslope migration of montane taxa. During Northern Hemisphere warming increased precipitation is projected to result in reduced fire activity and spread of rainforests.
The lack of a consistent and constant chronology for DOs outside the North Atlantic is one reason why the source of these changes is not fully understood. Due to the margin of error surrounding chronologies, it is challenging to reliably compare two different sites as the error can be larger than the duration of these abrupt events. To gain further insights into understanding the possible drivers of abrupt climate events such as Dansgaard-Oeschger cycles, multi-proxy approaches should be used on high resolution marine sequences. Marine sequences from the tropical Atlantic will produce integrated datasets of oceanic and terrestrial change that permit to explore the tropical trigger hypothesis of DOs.
A greater understanding of past abrupt climate events is crucial to better predict how the climate system may be responding to anthropogenic forcing in the 21st century and how it may respond in the future. By understanding the driving forces and responses of natural climate variability, we can mitigate and prepare for anthropogenic climate change. A better understanding of the climate system would provide the opportunity to manage current and future climate crises and their effects in food security, natural disasters and biodiversity loss.
Author Contributions
JO and DU conceived and developed the ideas in the manuscript. JO and DU wrote the manuscript and created the figures for the manuscript. Both authors contributed to the article and approved the submitted version.
Funding
JO is supported by a NERC GW4 + Doctoral Training Partnership studentship from the Natural Environmental Research Council (NE/L002434/1). DU acknowledges the project “Latin American Abrupt Climate Changes and Environmental Responses” (LaACER), funded by PAGES and INQUA.
Conflict of Interest
The authors declare that the research was conducted in the absence of any commercial or financial relationships that could be construed as a potential conflict of interest.
Acknowledgments
We thank the editor Michaël Hermoso and reviewers Gerrit Lohmann and Igor Venancio for their constructive feedback which has added to and improved our work. We also thank Maria Sanchez Goñi and Toby Pennington for insightful discussions.
References
Adolphi, F., Bronk Ramsey, C., Erhardt, T., Edwards, R. L., Cheng, H., Turney, C. S., et al. (2018). Connecting the Greenland ice-core and U/Th timescales via cosmogenic radionuclides: testing the synchroneity of Dansgaard–Oeschger events. Climate of the Past 14, 1755–1781. doi: 10.5194/cp-14-1755-2018
Alvarez-Solas, J., Charbit, S., Ritz, C., Paillard, D., Ramstein, G., and Dumas, C. (2010). Links between ocean temperature and iceberg discharge during Heinrich events. Nature Geoscience 3, 122–126. doi: 10.1038/NGEO752
Alves, E., Macario, K., Souza, R., Pimenta, A., Douka, K., Oliveira, F., et al. (2015). Radiocarbon reservoir corrections on the Brazilian coast from pre-bomb marine shells. Quaternary Geochronology 29, 30–35. doi: 10.1016/j.quageo.2015.05.006
Andersen, K. K., Azuma, N., Barnola, J. M., Bigler, M., Biscaye, P., Caillon, N., et al. (2004). High-resolution record of Northern Hemisphere climate extending into the last interglacial period. Nature 431, 147–151. doi: 10.1038/nature02805
Andersen, K. K., Svensson, A., Johnsen, S. J., Rasmussen, S. O., Bigler, M., Röthlisberger, R., et al. (2006). The Greenland ice core chronology 2005, 15–42 ka. Part 1: constructing the time scale. Quaternary Science Reviews 25, 3246–3257. doi: 10.1016/j.quascirev.2006.08.002
Arz, H. W., Pätzold, J., and Wefer, G. (1998). Correlated millennial-scale changes in surface hydrography and terrigenous sediment yield inferred from last-glacial marine deposits off northeastern Brazil. Quaternary Research 50, 157–166. doi: 10.1006/qres.1998.1992
Arz, H. W., Pätzold, J., and Wefer, G. (1999). The deglacial history of the western tropical Atlantic as inferred from high resolution stable isotope records off northeastern Brazil. Earth and Planetary Science Letters 167, 105–117. doi: 10.1016/s0012-821x(99)00025-4
Bahr, A., Hoffmann, J., Schönfeld, J., Schmidt, M. W., Nürnberg, D., Batenburg, S. J., et al. (2018). Low-latitude expressions of high-latitude forcing during Heinrich Stadial 1 and the Younger Dryas in northern South America. Global and Planetary Change 160, 1–9. doi: 10.1016/j.gloplacha.2017.11.008
Baker, P. A., Seltzer, G. O., Fritz, S. C., Dunbar, R. B., Grove, M. J., Tapia, P. M., et al. (2001). The history of South American tropical precipitation for the past 25,000 years. Science 291, 640–643. doi: 10.1126/science.291.5504.640
Bard, E., Rostek, F., Turon, J.-L., and Gendreau, S. (2000). Hydrological impact of Heinrich events in the subtropical northeast Atlantic. Science 289, 1321–1324. doi: 10.1126/science.289.5483.1321
Barker, S., Chen, J., Gong, X., Jonkers, L., Knorr, G., and Thornalley, D. (2015). Icebergs not the trigger for North Atlantic cold events. Nature 520, 333. doi: 10.1038/nature14330
Bassis, J. N., Petersen, S. V., and Mac Cathles, L. (2017). Heinrich events triggered by ocean forcing and modulated by isostatic adjustment. Nature 542, 332. doi: 10.1038/nature21069
Behling, H., Arz, H. W., Pätzold, J., and Wefer, G. (2000). Late Quaternary vegetational and climate dynamics in northeastern Brazil, inferences from marine core GeoB 3104-1. Quaternary Science Reviews 19, 981–994. doi: 10.1016/s0277-3791(99)00046-3
Berger, A. (1992). Astronomical theory of paleoclimates and the last glacial-interglacial cycle. Quaternary Science Reviews 11, 571–581. doi: 10.1016/0277-3791(92)90014-y
Berger, A., Loutre, M.-F., and Mélice, J. (2006). Equatorial insolation: from precession harmonics to eccentricity frequencies. Climate of the Past 2, 131–136. doi: 10.5194/cp-2-131-2006
Blaauw, M. (2012). Out of tune: the dangers of aligning proxy archives. Quaternary Science Reviews 36, 38–49. doi: 10.1016/j.quascirev.2010.11.012
Blaauw, M., Wohlfarth, B., Christen, J. A., Ampel, L., Veres, D., Hughen, K. A., et al. (2010). Were last glacial climate events simultaneous between Greenland and France? A quantitative comparison using non-tuned chronologies. Journal of Quaternary Science: Published for the Quaternary Research Association 25, 387–394. doi: 10.1002/jqs.1330
Blunier, T., and Brook, E. J. (2001). Timing of millennial-scale climate change in Antarctica and Greenland during the last glacial period. Science 291, 109–112. doi: 10.1126/science.291.5501.109
Bogota-a, R., Groot, M., Hooghiemstra, H., Lourens, L., Van der Linden, M., and Berrio, J. (2011). Rapid climate change from north Andean Lake Fúquene pollen records driven by obliquity: implications for a basin-wide biostratigraphic zonation for the last 284 ka. Quaternary Science Reviews 30, 3321–3337. doi: 10.1016/j.quascirev.2011.08.003
Bradbury, J. P., Leyden, B., Salgado-Labouriau, M., Lewis, W. M., Schubert, C., Binford, M. W., et al. (1981). Late quaternary environmental history of Lake Valencia. Venezuela. Science 214, 1299–1305.
Braun, H., Christl, M., Rahmstorf, S., Ganopolski, A., Mangini, A., Kubatzki, C., et al. (2005). Possible solar origin of the 1,470-year glacial climate cycle demonstrated in a coupled model. Nature 438, 208–211. doi: 10.1038/nature04121
Broccoli, A. J., Dahl, K. A., and Stouffer, R. J. (2006). Response of the ITCZ to Northern Hemisphere cooling. Geophysical Research Letters 2006, 33.
Broecker, W. S. (1991). The great ocean conveyor. Oceanography 4, 79–89. doi: 10.5670/oceanog.1991.07
Brown, E., Johnson, T., Scholz, C., Cohen, A., and King, J. (2007). Abrupt change in tropical African climate linked to the bipolar seesaw over the past 55,000 years. Geophysical Research Letters 34, 20.
Budsky, A., Wassenburg, J. A., Mertz-Kraus, R., Spötl, C., Jochum, K. P., Gibert, L., et al. (2019). Western Mediterranean climate response to Dansgaard/Oeschger events: New insights from speleothem records. Geophysical Research Letters 46, 9042–9053. doi: 10.1029/2019gl084009
Buizert, C., and Schmittner, A. (2015). Southern Ocean control of glacial AMOC stability and Dansgaard-Oeschger interstadial duration. Paleoceanography 30, 1595–1612. doi: 10.1002/2015pa002795
Cane, M., and Clement, A. C. (1999). ”A role for the tropical Pacific coupled ocean-atmosphere system on Milankovitch and millennial timescales. Part II: Global impacts,” in Mechanisms of Global Climate Change at Millennial Time Scales, 1999. Blackwell Publishing Ltd∗, 373–383.
Carvalho, L. M., Jones, C., and Liebmann, B. (2004). The South Atlantic convergence zone: Intensity, form, persistence, and relationships with intraseasonal to interannual activity and extreme rainfall. Journal of Climate 17, 88–108. doi: 10.1175/1520-04422004017
Center for Advanced Marine Core Research, Kochi University. (2020). Marine Cores. https://www.kochi-u.ac.jp/marine-core/en/index.html. [Accessed 10 Nov 2020]
Chaves, R. R., and Nobre, P. (2004). Interactions between sea surface temperature over the South Atlantic Ocean and the South Atlantic Convergence Zone. Geophysical Research Letters 31, 3.
Chiessi, C. M., Mulitza, S., Pätzold, J., Wefer, G., and Marengo, J. A. (2009). Possible impact of the Atlantic Multidecadal Oscillation on the South American summer monsoon. Geophysical Research Letters 36, 21.
Clark, P. U., Pisias, N. G., Stocker, T. F., and Weaver, A. J. (2002). The role of the thermohaline circulation in abrupt climate change. Nature 415, 863–869. doi: 10.1038/415863a
Clement, A. C., and Peterson, L. C. (2008). Mechanisms of abrupt climate change of the last glacial period. Reviews of Geophysics 46, 4.
Collins, M., Knutti, R., Arblaster, J., Dufresne, J.-L., Fichefet, T., Friedlingstein, P., et al. (2013). ”Long-term climate change: projections, commitments and irreversibility,” in Climate Change 2013-The Physical Science Basis: Contribution of Working Group I to the Fifth Assessment Report of the Intergovernmental Panel on Climate Change. Cambridge University Press∗, 1029–1136.
Correa-Metrio, A., Bush, M. B., Cabrera, K. R., Sully, S., Brenner, M., Hodell, D. A., et al. (2012). Rapid climate change and no-analog vegetation in lowland Central America during the last 86,000 years. Quaternary Science Reviews 38, 63–75. doi: 10.1016/j.quascirev.2012.01.025
Correge, T., Gagan, M. K., Beck, J. W., Burr, G. S., Cabioch, G., and Le Cornec, F. (2004). Interdecadal variation in the extent of South Pacific tropical waters during the Younger Dryas event. Nature 428, 927. doi: 10.1038/nature02506
Cruz, F. W., Wang, X., Auler, A., Vuille, M., Burns, S. J., Edwards, L. R., et al. (2009). ”Orbital and millennial-scale precipitation changes in Brazil from speleothem records,” in Past Climate Variability in South America and Surrounding Regions. Springer∗, 29–60.
CruzJr., Burns, S. J., Karmann, I., Sharp, W. D., Vuille, M., Cardoso, A. O., et al. (2005). Insolation-driven changes in atmospheric circulation over the past 116,000 years in subtropical Brazil. Nature 434, 63. doi: 10.1038/nature03365
Da Silveira, and Lobo Sternberg, L. (2001). Savanna–forest hysteresis in the tropics. Global Ecology and Biogeography 10, 369–378. doi: 10.1046/j.1466-822x.2001.00243.x
Daniau, A.-L., Sanchez Goñi, M. F., Martinez, P., Urrego, D. H., Bout-Roumazeilles, V., Desprat, S., et al. (2013). Orbital-scale climate forcing of grassland burning in southern Africa. Proceedings of the National Academy of Sciences 110, 5069–5073. doi: 10.1073/pnas.1214292110
Daniau, A.-L., Sánchez-Goñi, M., Beaufort, L., Laggoun-Défarge, F., Loutre, M.-F., and Duprat, J. (2007). Dansgaard–Oeschger climatic variability revealed by fire emissions in southwestern Iberia. Quaternary Science Reviews 26, 1369–1383. doi: 10.1016/j.quascirev.2007.02.005
De Almeida, R., Nobre, P., Haarsma, R., and Campos, E. (2007). Negative ocean-atmosphere feedback in the South Atlantic Convergence Zone. Geophysical Research Letters 34, 18.
Desprat, S., Sanchez Goñi, M. F., Turon, J.-L., McManus, J., Loutre, M.-F., Duprat, J., et al. (2005). Is vegetation responsible for glacial inception during periods of muted insolation changes? Quaternary Science Reviews 24, 1361–1374. doi: 10.1016/j.quascirev.2005.01.005
Dima, M., Lohmann, G., and Knorr, G. (2018). North Atlantic Versus Global Control on Dansgaard-Oeschger Events. Geophysical Research Letters 45(23) 12, 998.
DiNapoli, R. J., Fitzpatrick, S. M., Napolitano, M. F., Rick, T. C., Stone, J. H., and Jew, N. P. (2021). Marine reservoir corrections for the Caribbean demonstrate high intra-and inter-island variability in local reservoir offsets. Quaternary Geochronology 61, 101126. doi: 10.1016/j.quageo.2020.101126
Dokken, T. M., Nisancioglu, K. H., Li, C., Battisti, D. S., and Kissel, C. (2013). Dansgaard-Oeschger cycles: Interactions between ocean and sea ice intrinsic to the Nordic seas. Paleoceanography 28, 491–502. doi: 10.1002/palo.20042
Dong, J., Shen, C.-C., Kong, X., Wang, Y., and Duan, F. (2018). Asian monsoon dynamics at Dansgaard/Oeschger events 14–8 and Heinrich events 5–4 in northern China. Quaternary Geochronology 47, 72–80. doi: 10.1016/j.quageo.2018.05.012
Ganopolski, A., and Rahmstorf, S. (2001). Rapid changes of glacial climate simulated in a coupled climate model. Nature 409, 153. doi: 10.1038/35051500
Garreaud, R. D., Vuille, M., Compagnucci, R., and Marengo, J. (2009). Present-day south american climate. Palaeogeography, Palaeoclimatology, Palaeoecology 281, 180–195. doi: 10.1016/j.palaeo.2007.10.032
Gkinis, V., Simonsen, S. B., Buchardt, S. L., White, J., and Vinther, B. M. (2014). Water isotope diffusion rates from the NorthGRIP ice core for the last 16,000 years–Glaciological and paleoclimatic implications. Earth and Planetary Science Letters 405, 132–141. doi: 10.1016/j.epsl.2014.08.022
Goes, M., Murphy, L. N., and Clement, A. C. (2019). The stability of the AMOC during Heinrich events is not dependent on the AMOC strength in an Intermediate Complexity Earth System model ensemble. Paleoceanography and Paleoclimatology 34, 1359–1374. doi: 10.1029/2019pa003580
Gottschalk, J., Skinner, L. C., Misra, S., Waelbroeck, C., Menviel, L., and Timmermann, A. (2015). Abrupt changes in the southern extent of North Atlantic Deep Water during Dansgaard–Oeschger events. Nature geoscience 8, 950–954. doi: 10.1038/ngeo2558
Griffiths, M. L., Drysdale, R. N., Gagan, M. K., Hellstrom, J. C., Couchoud, I., Ayliffe, L. K., et al. (2013). Australasian monsoon response to Dansgaard–Oeschger event 21 and teleconnections to higher latitudes. Earth and Planetary Science Letters 369, 294–304. doi: 10.1016/j.epsl.2013.03.030
Groot, M., Bogotá, R., Lourens, L., Hooghiemstra, H., Vriend, M., Berrio, J., et al. (2011). Ultra-high resolution pollen record from the northern Andes reveals rapid shifts in montane climates within the last two glacial cycles. Climate of the Past 7, 299–316. doi: 10.5194/cp-7-299-2011
Gu, S., Liu, Z., Oppo, D. W., Lynch-Stieglitz, J., Jahn, A., Zhang, J., et al. (2020). Assessing the potential capability of reconstructing glacial Atlantic water masses and AMOC using multiple proxies in CESM. Earth and Planetary Science Letters 541, 116294. doi: 10.1016/j.epsl.2020.116294
Hagelberg, T. K., Bond, G., and Demenocal, P. (1994). Milankovitch band forcing of sub-Milankovitch climate variability during the Pleistocene. Paleoceanography 9, 545–558. doi: 10.1029/94PA00443
Harrison, S., and Sanchez Goñi, M. F. (2010). Global patterns of vegetation response to millennial-scale variability and rapid climate change during the last glacial period. Quaternary Science Reviews 29, 2957–2980. doi: 10.1016/j.quascirev.2010.07.016
Heinrich, H. (1988). Origin and consequences of cyclic ice rafting in the northeast Atlantic Ocean during the past 130,000 years. Quaternary research 29, 142–152. doi: 10.1016/0033-5894(88)90057-9
Heaton, T. J., Köhler, P., Butzin, M., Bard, E., Reimer, R. W., Austin, W. E., et al. (2020). Marine20—the marine radiocarbon age calibration curve (0–55,000 cal BP). Radiocarbon 62, 779–820. doi: 10.1017/RDC.2020.68
Hessler, I., Dupont, L., Bonnefille, R., Behling, H., González, C., Helmens, K. F., et al. (2010). Millennial-scale changes in vegetation records from tropical Africa and South America during the last glacial. Quaternary Science Reviews 29, 2882–2899. doi: 10.1016/j.quascirev.2009.11.029
Hodell, D., and Channell, J. E. (2016). Mode transitions in Northern Hemisphere glaciation: co-evolution of millennial and orbital variability in Quaternary climate∗.
IPCC (2014). Mitigation of climate change, Contribution of Working Group III to the Fifth Assessment Report of the Intergovernmental Panel on Climate Change 1454.
Itambi, A. C., von Dobeneck, T., Mulitza, S., Bickert, T., and Heslop, D. (2009). Millennial-scale northwest African droughts related to Heinrich events and Dansgaard-Oeschger cycles: Evidence in marine sediments from offshore Senegal. Paleoceanography 24, 1.
Jaeschke, A., Rühlemann, C., Arz, H., Heil, G., and Lohmann, G. (2007). Coupling of millennial-scale changes in sea surface temperature and precipitation off northeastern Brazil with high-latitude climate shifts during the last glacial period. Paleoceanography 22, 4. doi: 10.1029/2006PA001391
Jennerjahn, T. C., Ittekkot, V., Arz, H. W., Behling, H., Pätzold, J., and Wefer, G. (2004). Asynchronous terrestrial and marine signals of climate change during Heinrich events. Science 306, 2236–2239. doi: 10.1126/science.1102490
Jorgetti, T., da Silva, Dias, P. L., and de Freitas, E. D. (2014). The relationship between South Atlantic SST and SACZ intensity and positioning. Climate dynamics 42, 3077–3086. doi: 10.1007/s00382-013-1998-z
Kageyama, M., Paul, A., Roche, D. M., and Van Meerbeeck, C. J. (2010). Modelling glacial climatic millennial-scale variability related to changes in the Atlantic meridional overturning circulation: a review. Quaternary Science Reviews 29, 2931–2956. doi: 10.1016/j.quascirev.2010.05.029
Kiefer, T., Lorenz, S., Schulz, M., Lohmann, G., Sarnthein, M., and Elderfield, H. (2002). Response of precipitation over Greenland and the adjacent ocean to North Pacific warm spells during Dansgaard–Oeschger stadials. Terra Nova 14, 295–300. doi: 10.1046/j.1365-3121.2002.00420.x
Knorr, G., Lohmann, G., Prange, M., Barker, S., and Laepple, T. (2009). “Cooling Induced Instability of the Atlantic Overturning Circulation as a Cause for Rapid Climate Transitions,” in EGU General Assembly Conference Abstracts∗, 13351.
Laskar, J. (1990). The chaotic motion of the solar system: A numerical estimate of the size of the chaotic zones. Icarus 88, 266–291. doi: 10.1016/0019-1035(90)90084-m
Lea, D. W., Pak, D. K., Peterson, L. C., and Hughen, K. A. (2003). Synchroneity of tropical and high-latitude Atlantic temperatures over the last glacial termination. Science 301, 1361–1364. doi: 10.1126/science.1088470
Li, C., Battisti, D. S., and Bitz, C. M. (2010). Can North Atlantic sea ice anomalies account for Dansgaard–Oeschger climate signals? Journal of Climate 23, 5457–5475. doi: 10.1175/2010JCLI3409
Li, C., and Born, A. (2019). Coupled atmosphere-ice-ocean dynamics in Dansgaard-Oeschger events. Quaternary Science Reviews 203, 1–20. doi: 10.1016/j.quascirev.2018.10.031
Li, T.-Y., Han, L.-Y., Cheng, H., Edwards, R. L., Shen, C.-C., Li, H.-C., et al. (2017). Evolution of the Asian summer monsoon during Dansgaard/Oeschger events 13-17 recorded in a stalagmite constrained by high-precision chronology from southwest China. Quaternary Research 88, 121. doi: 10.1017/qua.2017.22
Liang, Y., Wang, Y., Wang, Q., Wu, J., Shao, Q., Zhang, Z., et al. (2019). East Asian summer monsoon climates and cave hydrological cycles over Dansgaard-Oeschger events 14 to 11 revealed by a new stalagmite record from Hulu Cave. Quaternary Research∗.
Lippold, J., Luo, Y., Francois, R., Allen, S. E., Gherardi, J., Pichat, S., et al. (2012). Strength and geometry of the glacial Atlantic Meridional Overturning Circulation. Nature Geoscience 5, 813–816.
Lohmann, G. (2017). Atmospheric bridge on orbital time scales. Theoretical and Applied Climatology 128, 709–718. doi: 10.1007/s00704-015-1725-2
Marino, G., Rohling, E., Rodríguez-Sanz, L., Grant, K., Heslop, D., Roberts, A., et al. (2015). Bipolar seesaw control on last interglacial sea level. Nature 522, 197. doi: 10.1038/nature14499
Markle, B. R., Steig, E. J., Buizert, C., Schoenemann, S. W., Bitz, C. M., Fudge, T., et al. (2017). Global atmospheric teleconnections during Dansgaard–Oeschger events. Nature Geoscience 10, 36. doi: 10.1038/ngeo2848
Martrat, B., Grimalt, J. O., Shackleton, N. J., de Abreu, L., Hutterli, M. A., and Stocker, T. F. (2007). Four climate cycles of recurring deep and surface water destabilizations on the Iberian margin. Science 317, 502–507. doi: 10.1126/science.1139994
McGee, D., Donohoe, A., Marshall, J., and Ferreira, D. (2014). Changes in ITCZ location and cross-equatorial heat transport at the last glacial maximum, Heinrich stadial 1, and the mid-Holocene. Earth Planet Sci. Lett. 390, 69–79. doi: 10.1016/j.epsl.2013.12.043
McIntyre, A., and Molfino, B. (1996). Forcing of Atlantic equatorial and subpolar millennial cycles by precession. Science 274, 1867–1870. doi: 10.1126/science.274.5294.1867
McManus, J. F., Francois, R., Gherardi, J.-M., Keigwin, L. D., and Brown-Leger, S. (2004). Collapse and rapid resumption of Atlantic meridional circulation linked to deglacial climate changes. Nature 428, 834–837. doi: 10.1038/nature02494
Mechoso, C. R., Lyons, S. W., and Spahr, J. A. (1990). The impact of sea surface temperature anomalies on the rainfall over northeast Brazil. Journal of climate 3, 812–826. doi: 10.1175/1520-0442(1990)003<0812:tiosst>2.0.co;2
Mecking, J., Drijfhout, S., Jackson, L., and Andrews, M. (2017). The effect of model bias on Atlantic freshwater transport and implications for AMOC bi-stability. Tellus A: Dynamic Meteorology and Oceanography 69, 1299910. doi: 10.1080/16000870.2017.1299910
Members, W. D. P. (2015). Precise interpolar phasing of abrupt climate change during the last ice age. Nature 520, 661–665. doi: 10.1038/nature14401
Moine, O., Antoine, P., Hatté, C., Landais, A., Mathieu, J., Prud’homme, C., et al. (2017). The impact of Last Glacial climate variability in west-European loess revealed by radiocarbon dating of fossil earthworm granules. Proceedings of the National Academy of Sciences 114, 6209–6214. doi: 10.1073/pnas.1614751114
Mollier-Vogel, E., Leduc, G., Böschen, T., Martinez, P., and Schneider, R. R. (2013). Rainfall response to orbital and millennial forcing in northern Peru over the last 18 ka. Quaternary Science Reviews 76, 29–38. doi: 10.1016/j.quascirev.2013.06.021
Mosblech, N. A., Bush, M. B., Gosling, W. D., Hodell, D., Thomas, L., Van Calsteren, P., et al. (2012). North Atlantic forcing of Amazonian precipitation during the last ice age. Nature Geoscience 5, 817. doi: 10.1038/ngeo1588
Mulitza, S., Paul, A., and Wefer, G. (2007). Late Pleistocene South Atlantic. Encyclopedia of Quaternary Science 3, 1816–1831. doi: 10.1016/b0-444-52747-8/00318-5
Muller, J., Kylander, M., Wüst, R. A., Weiss, D., Martinez-Cortizas, A., LeGrande, A. N., et al. (2008). Possible evidence for wet Heinrich phases in tropical NE Australia: the Lynch’s Crater deposit. Quaternary Science Reviews 27, 468–475. doi: 10.1016/j.quascirev.2007.11.006
Nobre, P., De Almeida, R. A., Malagutti, M., and Giarolla, E. (2012). Coupled ocean–atmosphere variations over the South Atlantic Ocean. Journal of Climate 25, 6349–6358. doi: 10.1175/JCLI-D-11-00444.1
Nusbaumer, J., Alexander, P. M., LeGrande, A. N., and Tedesco, M. (2019). Spatial shift of Greenland moisture sources related to enhanced Arctic warming. Geophysical Research Letters 46, 14723–14731. doi: 10.1029/2019gl084633
Oliveira, M. I., Carvalho, C., Macario, K., Evangelista, H., Lamounier, S., and Hammerschlag, I. (2019). Marine Reservoir Corrections for the Brazilian Northern Coast Using Modern Corals. Radiocarbon 61, 587–597. doi: 10.1017/RDC.2018.145
Orsi, A. J., Cornuelle, B. D., and Severinghaus, J. P. (2014). Magnitude and temporal evolution of Dansgaard–Oeschger event 8 abrupt temperature change inferred from nitrogen and argon isotopes in GISP2 ice using a new least-squares inversion. Earth and Planetary Science Letters 395, 81–90. doi: 10.1016/j.epsl.2014.03.030
Paduano, G. M., Bush, M. B., Baker, P. A., Fritz, S. C., and Seltzer, G. O. (2003). A vegetation and fire history of Lake Titicaca since the Last Glacial Maximum. Palaeogeography, Palaeoclimatology, Palaeoecology 194, 259–279. doi: 10.1016/s0031-0182(03)00281-5
Pennington, R. T., and Lavin, M. (2016). The contrasting nature of woody plant species in different neotropical forest biomes reflects differences in ecological stability. New Phytologist 210, 25–37. doi: 10.1111/nph.13724
Peterson, L. C., and Haug, G. H. (2006). Variability in the mean latitude of the Atlantic Intertropical Convergence Zone as recorded by riverine input of sediments to the Cariaco Basin (Venezuela). Palaeogeography, Palaeoclimatology, Palaeoecology 234, 97–113. doi: 10.1016/j.palaeo.2005.10.021
Petersen, S. V., Schrag, D. P., and Clark, P. U. (2013). A new mechanism for Dansgaard-Oeschger cycles. Paleoceanography 28, 24–30. doi: 10.1029/2012PA002364
Peterson, L. C., Overpeck, J., Kipp, N., and Imbrie, J. (1991). A high-resolution late Quaternary upwelling record from the anoxic Cariaco Basin, Venezuela. Paleoceanography 6, 99–119. doi: 10.1029/90pa02497
Phillips, O. L., Aragão, L. E., Lewis, S. L., Fisher, J. B., Lloyd, J., López-González, G., et al. (2009). Drought sensitivity of the Amazon rainforest. Science 323, 1344–1347.
Rama-Corredor, O., Martrat, B., Grimalt, J. O., López-Otalvaro, G. E., Flores, J. A., and Sierro, F. (2015). Parallelisms between sea surface temperature changes in the western tropical Atlantic (Guiana Basin) and high latitude climate signals over the last 140,000 years. Climate of the Past 11, 1297–1311. doi: 10.5194/cp-11-1297-2015
Ratter, J. (1992). Transiciones entre la vegetación de Cerrado y la de bosques en Brasil]. Transition between cerrado and forest vegetation in Brazil∗.
Reimer, R. W., and Reimer, P. J. (2017). An online application for [.] R calculation. Radiocarbon 59, 1623. doi: 10.1017/RDC.2016.117
Richardson, P., Hufford, G., Limeburner, R., and Brown, W. (1994). North Brazil current retroflection eddies. Journal of Geophysical Research: Oceans 99, 5081–5093. doi: 10.1029/93jc03486
Ripple, W. J., Wolf, C., Newsome, T. M., Galetti, M., Alamgir, M., Crist, E., et al. (2017). World scientists’ warning to humanity: A second notice. BioScience 67, 1026–1028.
Ringeval, B., Hopcroft, P., Valdes, P., Ciais, P., Ramstein, G., Dolman, A., et al. (2013). Response of methane emissions from wetlands to the Last Glacial Maximum and an idealized Dansgaard–Oeschger climate event: insights from two models of different complexity. Climate of the Past 9, 149–171. doi: 10.5194/cpd-8-3093-2012
Rodgers, K. B., Lohmann, G., Lorenz, S., Schneider, R., and Henderson, G. M. (2003). A tropical mechanism for Northern Hemisphere deglaciation. Geochemistry, Geophysics, Geosystems 4, 508. doi: 10.1029/2003GC000508
Rohling, E. J., Sluijs, A., Dijkstra, H. A., Köhler, P., van de Wal, R. S., and von, et al. (2012). Making sense of palaeoclimate sensitivity. Nature 491, 683–691. doi: 10.1038/nature11574
Ruddiman, W. F. (2006). Orbital changes and climate. Quaternary Science Reviews 25, 3092–3112. doi: 10.1016/j.quascirev.2006.09.001
Rühlemann, C., Mulitza, S., Müller, P. J., Wefer, G., and Zahn, R. (1999). Warming of the tropical Atlantic Ocean and slowdown of thermohaline circulation during the last deglaciation. Nature 402, 511–514. doi: 10.1038/990069
Rutherford, S., and D’Hondt, S. (2000). Early onset and tropical forcing of 100,000-year Pleistocene glacial cycles. Nature 408, 72–75. doi: 10.1038/35040533
Sanchez Goñi, M. F., Bard, E., Landais, A., Rossignol, L., and d’Errico, F. (2013). Air–sea temperature decoupling in western Europe during the last interglacial–glacial transition. Nature Geoscience 6, 837–841. doi: 10.1038/ngeo1924
Sánchez Goñi, M. F., Desprat, S., Fletcher, W. J., Morales-Molino, C., Naughton, F., Oliveira, D., et al. (2018). Pollen from the deep-sea: a breakthrough in the mystery of the Ice Ages. Frontiers in plant science 9:38.
Sánchez Goñi, M. F., and Harrison, S. P. (2010). Millennial-scale climate variability and vegetation changes during the Last Glacial: Concepts and terminology. Quaternary Science Reviews 29, 2823–2827. doi: 10.1016/j.quascirev.2009.11.014
Sánchez Goñi, M. F., Fourcade, T., Salonen, S., Lesven, J., Frigola, J., Swingedouw, D., et al. (2020). Muted cooling and drying of NW Mediterranean in response to the strongest last glacial North American ice surges. GSA Bulletin 133, 451–460. doi: 10.1130/b35736.1
Sanchez Goñi, M. F., Rodrigues, T., Hodell, D. A., Polanco-Martinez, J. M., Alonso-Garcia, M., Hernández-Almeida, I., et al. (2016). Tropically-driven climate shifts in southwestern Europe during MIS 19, a low eccentricity interglacial. Earth and Planetary Science Letters 448, 81–93. doi: 10.1016/j.epsl.2016.05.018
Santos, T. P., Ballalai, J. M., Franco, D. R., Oliveira, R. R., Lessa, D. O., Venancio, I. M., et al. (2020). Asymmetric response of the subtropical western South Atlantic thermocline to the Dansgaard-Oeschger events of Marine Isotope Stages 5 and 3. Quaternary Science Reviews 237, 106307. doi: 10.1016/j.quascirev.2020.106307
Schmidt, M. W., Spero, H. J., and Lea, D. W. (2004). Links between salinity variation in the Caribbean and North Atlantic thermohaline circulation. Nature 428, 160–163. doi: 10.1038/nature02346
Schneider, T., Bischoff, T., and Haug, G. H. (2014). Migrations and dynamics of the intertropical convergence zone. Nature 513, 45. doi: 10.1038/nature13636
Schulz, M., Paul, A., and Timmermann, A. (2002). Relaxation oscillators in concert: A framework for climate change at millennial timescales during the late Pleistocene. Geophysical Research Letters 29, 41–46. doi: 10.1029/2002GL016144
Seillès, B., Sánchez Goñi, M. F., Ledru, M.-P., Urrego, D. H., Martinez, P., Hanquiez, V., et al. (2016). Holocene land–sea climatic links on the equatorial Pacific coast (Bay of Guayaquil. Ecuador). The Holocene 26, 567–577. doi: 10.1177/0959683615612566
Sime, L., Vallet, I. M., and Valdes, P. (2021). “Dansgaard-Oeschger Tipping Events (TEs): Towards determining if IPCC-relevant models represent these TEs,” in Copernicus Meetings∗.
Stauffer, B., Blunier, T., Dällenbach, A., Indermühle, A., Schwander, J., Stocker, T., et al. (1998). Atmospheric CO 2 concentration and millennial-scale climate change during the last glacial period. Nature 392, 59. doi: 10.1038/32133
Steffensen, J. P., Andersen, K. K., Bigler, M., Clausen, H. B., Dahl-Jensen, D., Fischer, H., et al. (2008). High-resolution Greenland ice core data show abrupt climate change happens in few years. Science 321, 680–684. doi: 10.1126/science.1157707
Stríkis, N. M., Chiessi, C. M., Cruz, F. W., Vuille, M., Cheng, H., de Souza Barreto, E. A., et al. (2015). Timing and structure of Mega-SACZ events during Heinrich Stadial 1. Geophysical Research Letters 42, 5477A–5484A.
Stuiver, M., and Braziunas, T. F. (1993). Modeling atmospheric 14C influences and 14C ages of marine samples to 10,000 BC. Radiocarbon 35, 137–189. doi: 10.1017/S0033822200013874
Stuiver, M., Reimer, P. J., and Reimer, R. W. (2021). CALIB 8.2 [WWW program]. http://calib.org, accessed 2021-3-30∗
Svensson, A., Andersen, K. K., Bigler, M., Clausen, H. B., Dahl-Jensen, D., Davies, S. M., et al. (2006). The Greenland ice core chronology 2005, 15–42 ka. Part 2: comparison to other records. Quaternary Science Reviews 25, 3258–3267. doi: 10.1016/j.quascirev.2006.08.003
Tremblin, M., Hermoso, M., and Minoletti, F. (2016). Equatorial heat accumulation as a long-term trigger of permanent Antarctic ice sheets during the Cenozoic. Proceedings on the National Academy of Sciences 133, 11782. doi: 10.1073/pnas.1608100113
Trenberth, K. E. (1999). Atmospheric moisture recycling: Role of advection and local evaporation. Journal of Climate 12, 1368–1381. doi: 10.1175/1520-0442(1999)012<1368:amrroa>2.0.co;2
Urrego, D., Bernal, J., Chiessi, C., Cruz, F., Sanchez Goñi, M., and Power, M. (2014). Hooghiemstra and LaACER participants: Millennial-scale climate variability in the American tropics and subtropics. PAGES Mag 22, 94–95. doi: 10.22498/pages.22.2.94
Urrego, D. H., Bush, M. B., and Silman, M. R. (2010). A long history of cloud and forest migration from Lake Consuelo. Peru. Quaternary Research 73, 364–373. doi: 10.1016/j.yqres.2009.10.005
Urrego, D. H., Hooghiemstra, H., Rama-Corredo, O., Martrat, B., Grimalt, J. O., Thompson, L., et al. (2016). Millennial-scale vegetation changes in the tropical Andes using ecological grouping and ordination methods∗.
Venancio, I., Mulitza, S., Govin, A., Santos, T., Lessa, D., Albuquerque, A., et al. (2018). Millennial-to Orbital-Scale Responses of Western Equatorial Atlantic Thermocline Depth to Changes in the Trade Wind System Since the Last Interglacial. Paleoceanography and Paleoclimatology 33, 1490–1507. doi: 10.1029/2018PA003437
Venancio, I., Shimizu, M., Santos, T., Lessa, D., Dias, B., Chiessi, C., et al. (2020). Ocean-atmosphere interactions over the western South Atlantic during Heinrich stadials. Global and Planetary Change 195, 103352. doi: 10.1016/j.gloplacha.2020.103352
Vettoretti, G., and Peltier, W. R. (2018). Fast Physics and Slow Physics in the Nonlinear Dansgaard–Oeschger Relaxation Oscillation. Journal of Climate 31, 3423–3449. doi: 10.1175/jcli-d-17-0559.1
Vinther, B. M., Clausen, H. B., Johnsen, S. J., Rasmussen, S. O., Andersen, K. K., Buchardt, S. L., et al. (2006). A synchronized dating of three Greenland ice cores throughout the Holocene. Journal of Geophysical Research: Atmospheres 111, 6921. doi: 10.1029/2005JD006921
Vink, A., Rühlemann, C., Zonneveld, K. A., Mulitza, S., Hüls, M., and Willems, H. (2001). Shifts in the position of the North Equatorial Current and rapid productivity changes in the western Tropical Atlantic during the last glacial. Paleoceanography 16, 479–490. doi: 10.1029/2000pa000582
von Deimling, T. S., Held, H., Ganopolski, A., and Rahmstorf, S. (2006). Climate sensitivity estimated from ensemble simulations of glacial climate. Climate Dynamics 27, 149–163. doi: 10.1007/s00382-006-0126-8
Wang, C., and Enfield, D. B. (2001). The tropical Western Hemisphere warm pool. Geophysical research letters 28, 1635–1638. doi: 10.1029/2000gl011763
Wang, Z., and Mysak, L. A. (2006). Glacial abrupt climate changes and Dansgaard-Oeschger oscillations in a coupled climate model. Paleoceanography 21, 2. doi: 10.1029/2005PA001238
Wilson, K. E., Maslin, M. A., and Burns, S. J. (2011). Evidence for a prolonged retroflection of the North Brazil Current during glacial stages. Palaeogeography, Palaeoclimatology, Palaeoecology 301, 86–96. doi: 10.1016/j.palaeo.2011.01.003
Wolff, E. W., Chappellaz, J., Blunier, T., Rasmussen, S. O., and Svensson, A. (2010). Millennial-scale variability during the last glacial: The ice core record. Quaternary Science Reviews 29, 2828–2838. doi: 10.1016/j.quascirev.2009.10.013
Zhang, X., Knorr, G., Lohmann, G., and Barker, S. (2017). Abrupt North Atlantic circulation changes in response to gradual CO 2 forcing in a glacial climate state. Nature Geoscience 10, 518–523. doi: 10.1038/NGEO2974
Zhang, Y., Chiessi, C. M., Mulitza, S., Sawakuchi, A. O., Häggi, C., Zabel, M., et al. (2017). Different precipitation patterns across tropical South America during Heinrich and Dansgaard-Oeschger stadials. Quaternary Science Reviews 177, 1–9. doi: 10.1016/j.quascirev.2017.10.012
Zhang, Y., Chiessi, C. M., Mulitza, S., Zabel, M., Trindade, R. I., Hollanda, M. H. B., et al. (2015). Origin of increased terrigenous supply to the NE South American continental margin during Heinrich Stadial 1 and the Younger Dryas. Earth and Planetary Science Letters 432, 493–500. doi: 10.1016/j.epsl.2015.09.054
Keywords: Dansgaard-Oeschger, chronology, tropical trigger, South America, vegetation, marine records
Citation: Oughton JW and Urrego DH (2021) Testing the Tropical Trigger Hypothesis of Abrupt Climate Variability. Front. Earth Sci. 9:669885. doi: 10.3389/feart.2021.669885
Received: 19 February 2021; Accepted: 14 April 2021;
Published: 13 May 2021.
Edited by:
Michaël Hermoso, UMR 8187 Laboratoire d’océanologie et de géosciences (LOG), FranceReviewed by:
Gerrit Lohmann, Alfred Wegener Institute Helmholtz Centre for Polar and Marine Research (AWI), GermanyIgor Venancio, Fluminense Federal University, Brazil
Copyright © 2021 Oughton and Urrego. This is an open-access article distributed under the terms of the Creative Commons Attribution License (CC BY). The use, distribution or reproduction in other forums is permitted, provided the original author(s) and the copyright owner(s) are credited and that the original publication in this journal is cited, in accordance with accepted academic practice. No use, distribution or reproduction is permitted which does not comply with these terms.
*Correspondence: Jack W. Oughton, am8zNTlAZXhldGVyLmFjLnVr