- 1Warner College of Natural Resources, Colorado State University, Fort Collins, CO, United States
- 2US Fish and Wildlife Service, Vancouver, WA, United States
- 3National Marine Fisheries Service, Santa Rosa, CA, United States
- 4Department of Earth and Environment, Franklin and Marshall College, Lancaster, PA, United States
- 5US Forest Service, Bend, OR, United States
- 6US Forest Service, Portland, OR, United States
- 7School of Geography, University of Nottingham, Nottingham, United Kingdom
River-wetland corridors form where a high degree of connectivity between the surface (rheic) and subsurface (hyporheic) components of streamflow creates an interconnected system of channels, wetlands, ponds, and lakes. River-wetland corridors occur where the valley floor is sufficiently wide to accommodate a laterally unconfined river planform that may feature morphologically complex, multi-threaded channels with vegetated bars, islands, and floodplains. River-wetland corridors can develop anywhere there is valley expansion along a drainage network, from the headwaters to estuaries or deltas, and they are found across all latitudes and within all biomes and hydroclimates. River-wetland corridors may be longitudinally continuous but are commonly interspersed with single-thread reaches in narrower portions of the valley. The development and persistence of river-wetland corridors is driven by combinations of geologic, biotic, and geomorphic processes that create a river environment that is diverse, heterogeneous, patchy, and dynamically stable, and within which patterns of flow, sediment features, and habitats shift continually. Hence, we describe these polydimensional river corridors as “kaleidoscope rivers.” Historically, river-wetland corridors were pervasive in wide, alluvial valley reaches, but their presence has been so diminished worldwide (due to a diverse range of anthropogenic activities and impacts) that the general public and even most river managers are unaware of their former pervasiveness. Here, we define river-wetland corridors as a river type; review paleoenvironmental and historical records to establish their past ubiquity; describe the geologic, biotic, and geomorphic processes responsible for their formation and persistence; and provide examples of river-wetland corridor remnants that still survive. We close by highlighting the significance of the diverse river functions supported by river-wetland corridors, the consequences of diminution and neglect of this river type, and the implications for river restoration.
Introduction
We define a river-wetland corridor as a relatively wide valley floor within which there is space for persistent alluvial deposits and sufficient connectivity between surface and subsurface hydrology to create and maintain an interacting system of channels, wetlands, and floodplain ponds and lakes. Salient characteristics are that the water table is at or close to ground elevation much of the time, and the floodplain is inundated and/or saturated frequently and for long periods. This corridor always includes within it the active channel(s), geomorphic floodplain, underlying hyporheic aquifer, and associated riverine wetlands (Harvey and Gooseff, 2015). The corridor may occupy the entirety of an alluvial valley floor, or its extent may be limited naturally (e.g., by river terraces) or anthropogenically (e.g., by levees).
Our focus is on understanding river-wetland corridors at the reach scale. In this context, we define a reach of a river-wetland corridor as the length of contiguous alluvial valley between topographic or geological controls, within which the channel planform is laterally unconfined and free to adjust and evolve in response to the influences of stream flow, sediment load, large wood, vegetation, and animals (e.g., beaver (Castor spp.)). A reach may include more than one channel planform. Indeed, as noted by (Leopold and Wolman 1957, p. 169), “A given reach of river may exhibit both braiding and meandering. In fact, Russell (1954) points out that the Meander River in Turkey, which gave us the term “meandering,” has both braided and straight reaches.” River-wetland corridors are then, discrete reaches along river networks, with spatial boundaries defined by distinctive characteristics of valley morphology and hydrology. “River” here includes all sizes of naturally flowing bodies of water, from creeks and brooks to the largest lotic ecosystems. The active channels are flow paths confined by streambanks and/or natural levees (Hey, 1979). Stream flow in a river-wetland corridor may predominantly follow a single channel that may be straight or meandering, it may follow multi-threaded channels (we use this term to include anastomosing, anabranching, and braiding), or stream flow may be unchanneled, with net movement down-valley occurring via a diffuse and shifting network of preferential flow paths. The geomorphic floodplain is composed of layers of fluvially deposited sediment and organic matter and is inundated and/or saturated for varying timespans from continuously to frequently. The floodplain surface is not flat, being composed of natural levees, levee back-slopes, splay deposits, abandoned channels, ridge and swale topography, back swamps, and open water bodies (ponds and lakes). Fluvial wetlands in the river-wetland corridor include seasonally saturated and inundated areas that may be linked to stream channels via surface (rheic) connections, subsurface (hyporheic) connection, or both (Ward, 1989; Stanford and Ward, 1993; Petts and Amoros, 1996). River-wetland corridors are, like the floodplains within which they exist, built and maintained by net deposition of sediment. That said, although dynamic adjustments of the river-wetland corridor are led by deposition, sediment erosion, transport, and exchange processes also play significant roles. Rates of net sedimentation may be imperceptible or matched by those of sediment compaction and floodplain subsidence, so that river-wetland corridors adjust continually over timespans of years to decades. We refer to this scenario as being dynamically stable in that the fundamental characteristics of abundant wetlands and substantial channel-floodplain hydrologic connectivity persist, although the detailed morphology of the river corridor is continually adjusting to and accommodating inputs of water and sediment, and internal feedbacks with biota. Conversely, a river-wetland corridor cannot long persist if its river is actively incising (Cluer and Thorne, 2014).
River-wetland corridors are fundamentally groundwater-dependent (Boulton, 2005). Subsurface water may be derived from influent flow from the river (especially during floods), infiltration of local precipitation and surface water, or percolation from the regional aquifer (Loheide et al., 2009). This range of recharge sources is important because a characteristic attribute of river-wetland corridors is that they can maintain their wetlands through inflow and infiltration, even in the absence of inputs from regional aquifers (Melly et al., 2017). Recognizing this, a river-wetland corridor can be described as rheos (from the Greek rhéos—which translates as “stream”) in that it constantly exchanges water with, and is therefore part of, the river. The point here is that not all of the river (rheos) flows above ground. The part of the river that flows beneath the ground is the hypo-rheos (literally, the river below); hence, we refer to the alluvial aquifer as being hyporheic, in that it is characterized by flow paths that originate from, and terminate in, the river corridor above (Gooseff, 2010). Laterally beyond and vertically beneath the hyporheic aquifer is the perirheic zone, which is the domain of water that is not part of the river, being sourced from and exchanged with that in other systems, such as valley side-slope hydrology and regional groundwater (Mertes, 1997) (Figure 1).
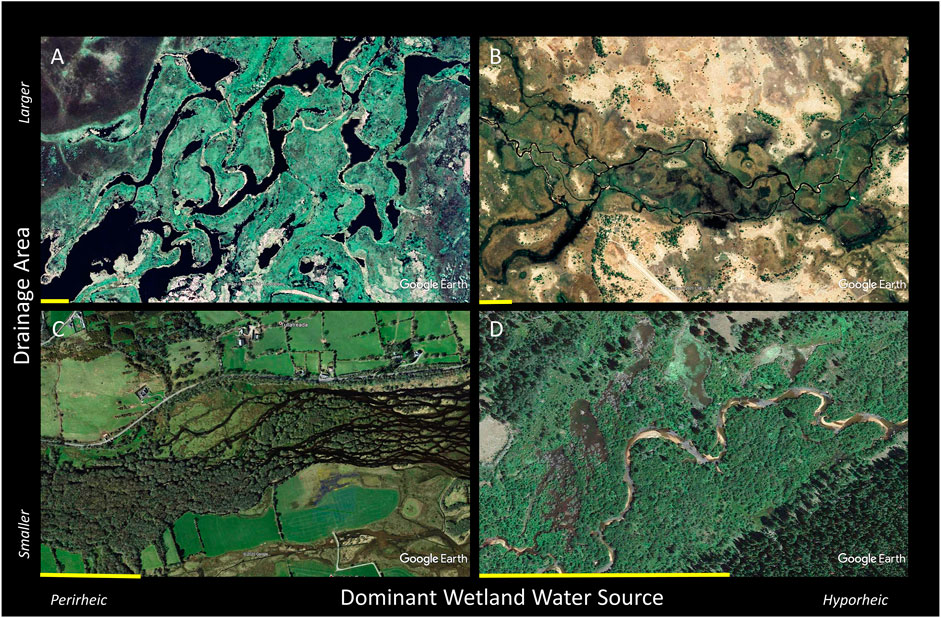
FIGURE 1. Examples of “contemporary remnants” in which fully functional river-wetland corridors still exist: (A) Pantanal, River Paraguay, Brazil; (B) Okavango Delta, Okavango River, Botswana; (C) The Gearagh, River Lee, Ireland; (D) North St. Vrain Creek, Colorado, United States. Each scale bar is approximately 300 m long.
River-wetland corridors can be laterally expansive and tend to be longitudinally discontinuous, alternating downstream with other river corridor configurations (e.g., relatively steep, laterally confined reaches) as valley geometry and/or fluxes into and out of the river corridor vary through space and time. River-wetland corridors can develop throughout the drainage network, from small, headwater streams in which meadows cover fractions of a hectare, to the lowermost courses and deltas of major rivers that drain basins with areas of thousands of square kilometers (e.g., the wet grasslands of the Florida Everglades or (historically) the bottomland forests and wood-dominated channels around the “Great Raft” on Louisiana’s Red River (Triska, 1984)).
Some areas of river-wetland corridors are perennially inundated, with aquatic vegetation ranging from algae to aquatic macrophytes. Larger areas are seasonally inundated and/or saturated, supporting a wide variety of wetland obligate, facultative, and riparian vegetation types including, but not limited to, woody species such as cottonwoods (Populus spp.), alder (Alnus spp.), and willows (Salix spp.), as well as sedges (Cyperaceae), rushes (Juncaceae), and grasses (Gramineae). Our definition of a river-wetland corridor thus subsumes beaver-meadows, riverine carr (a type of wetland dominated by shrubs; Whittow, 1984), sloughs, swamps, muskeg, bottomland forests (e.g., the várzea and igapó of the Amazon basin), wet meadows, wet woodlands, bosques (gallery forests), sausals, and ciénega (wetlands in the arid United States Southwest), along with open water ponds and lakes. A characteristic of all these vegetation assemblages is that plant cover in river-wetland corridors is typically dense, which contributes to sediment deposition and relatively high vegetative resistance to flow and fluvial erosion.
We conceptualize river-wetland corridors as falling on a continuum of types of river reach that form in valley segments with less lateral confinement and a lower gradient relative to reaches up- and downstream in the river network (Figure 2). The continuum of river types that extends from relatively dry, braided stream corridors to perennially wet river-wetland corridors is one of increasing hydrologic connectivity and complexity when integrated across three dimensions: longitudinally along the channel(s) and floodplain; vertically between the surface and subsurface components of river flow, and laterally between the channel, wetlands, floodplain, the valley sides, and adjacent uplands.
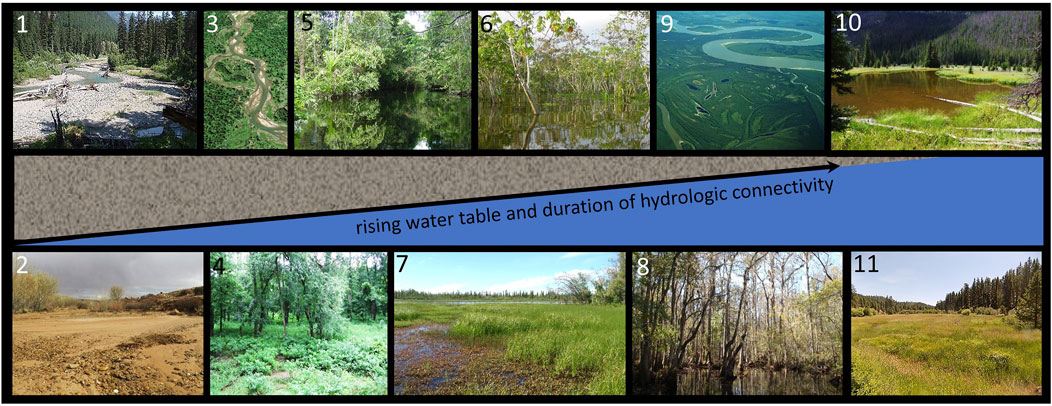
FIGURE 2. A schematic illustration of the continuum of channel planform, vegetation communities, and floodplain water table within wide, low-gradient valley segments. Inset illustrations are (1) Park Creek, MT, United States, (2) Harris Wash, UT, United States, (3) Swan River, MT, United States (4) bottomland hardwood forest along the Illinois River, IL, United States, (5) igapo forest, Amazon River, Brazil, (6) várzea forest on the Amazon River floodplain, Brazil, (7) wet meadow and lake, Yukon River, Alaska, United States (8) swamp on the floodplain of the Congaree River, SC, United States, (9) muskeg along the floodplain of the Yukon River, AK, United States, (10) an abandoned beaver pond in a wet-meadow stream corridor, CO, United States, and (11) wet-meadow stream corridor at Deep Creek, OR, United States.
In a river-wetland corridor, downstream passage of water, sediment, and organic material (e.g., large wood, detritus) is slowed due to lack of lateral confinement (resulting in wider, shallower flows), coupled with high flow resistance and permeable, in-channel obstructions (e.g., vegetation, logjams, beaver dams). This is especially the case in multi-threaded rivers, where the absence of a single, large, high-conveyance channel both reflects and enhances natural tendencies for sediment deposition and large wood retention. In essence, modulated longitudinal stream connectivity results in longer residence times for water, solutes, sediment, and organic matter in river-wetland corridors compared to river types with more concentrated flow paths and less spatial heterogeneity (Helton et al., 2014; Wohl, 2017).
Wide valley floors provide space for laterally extensive floodplains and channel migration zones that accommodate the full spectrum of channel planforms including straight, meandering, and multi-threaded (braided, anastomosing, anabranching). Braided rivers typically form where stream bank resistance to erosion is low compared to that of the stream bed; channel and valley gradients are relatively steep; and discharges relative to size of material in the channel (i.e., dimensionless discharges) are comparatively high (Eaton et al., 2010). Braided rivers can form in both wet and dry climates. A braided river floodplain can be well drained because of high hydraulic conductivity in the coarse sediment in which the floodplain is formed, or the floodplain can be sufficiently wet to fit our criteria for a river-wetland corridor. As the content of fine, cohesive sediment in floodplain soils increases and/or dense vegetation colonizes the riparian zone, the erosional resistance of stream banks increases. Both field observation (e.g., Schumm, 1985) and laboratory experimentation (e.g., Tal and Paola, 2010) show that cohesive soils and vegetation can transform a braided channel planform into one characterized by elements of meandering, anastomosing, and/or anabranching.
To provide context for current river-wetland corridor management and restoration efforts, we start by reviewing the historical and paleoenvironmental evidence of their past abundance and ubiquity and documenting their dramatic decline over the last two centuries. We examine the temporally and spatially dynamic roles of geology, biology, and geomorphology in their formation and persistence, and elucidate our characterization of polydimensional river-wetland corridors as “kaleidoscope rivers.” We close by establishing the functional significance of river-wetland corridors and consider the implications of their rediscovery for river restoration. We conclude that river-wetland corridors: 1) were wetter, more abundant, and widespread globally prior to intensive human alteration of catchments, valley floors, and channels; 2) support river functions that are compromised or lost when river-wetland corridors are simplified, degraded, or drained; and 3) should be both conserved through sustainable river management and recognized as a potentially legitimate and desirable target condition for river restoration that is necessary for the recovery of wetland obligate species as well as species that derive benefits from wetlands.
Former Abundance
Evidence of the antiquity, former abundance, and widespread distribution of river-wetland corridors exists in geological, paleoenvironmental, archeological, and historical records. For example, stratigraphy indicative of deposition by rivers with anastomosing or anabranching planforms suggests that rivers of this type began replacing braided rivers during the middle Devonian, ∼400 million years B.P. Coincidence between planform metamorphosis and colonization of river valleys by forests led (Davies and Gibling, 2011, p. 629) to conclude that, “…expansion of tree habitats led to the crossing of a threshold in vegetative control of floodplain and river morphology”. Paleoenvironmental information in the form of buried hydrosols, wetland plant macrofossils, and pollen (e.g., Brown, 2002; Davis et al., 2002) confirms that heavily vegetated, multi-threaded systems were much wetter than the contemporary, artificially drained river corridors to which we have become accustomed.
Archeological records are also useful for inferring the former existence of river-wetland corridors because people have settled near the rich resources of this valley type for millennia (e.g., Coles and Orme, 1983; Coles, 2000; Kim et al., 2008). Interpretations of several archeological sites in the United Kingdom, for example, suggest that some prehistoric human groups preferentially settled in valleys modified by beavers in order to exploit the fertile wet-meadows for grazing and cropland, re-use beaver-cut timber in building houses, and take advantage of abundant fish stocks (Liarsou, 2013).
Historical records in the form of photographs, maps (e.g., Kaatz, 1955; Hohensinner et al., 2021), written descriptions (e.g., Christy and Alverson, 2011), place names (Brown et al., 2018), and drawings provide evidence of the more recent existence of river-wetland corridors (e.g., Baker et al., 1993). Although photographs commonly serve as a primary source, they provide little insight into river systems that were modified prior to the advent of photography in the mid-1800s. Maps and written documents provide a longer record. In the United States, records of exploration and General Land Office (GLO) maps made between 1812 and 1946 provide reliable sources of information, especially in the western United States. The GLO surveyed and mapped lands in support of land division and sales to settlers and immigrants. Although the mapping of river corridors was not the intended purpose of GLO maps, river channels commonly served as property boundaries and reference points. Written records accompanied many expeditions and provide a source of supporting information. An example comes from a report submitted to the United States Secretary of War in 1875 describing the valley of the Willamette River, OR, United States, as quoted in Sedell and Froggatt (1984), p. 1830: “[the floodplain] during floods was covered with swiftly-running water to a depth of 1.5–3 m. Each year new channels were opened, old ones closed; new chutes cut, old ones obstructed by masses of drifts; sloughs became the main bed while the latter assumed the characteristics of the former; extensive [wood] rafts are piled up by one freshet only to be displaced by a succeeding one; the formation of islands and bars is in constant progress where the velocity of the current receives a sudden check only to disappear at the very next high water.”
There are, however, significant limitations to using contemporary or historical maps and photographs to determine planform characteristics for small watercourses. Many topographic maps are based on aerial photographs, in which small, well-vegetated streams are typically indiscernible. As a result, stream characteristics such as sinuosity and planform pattern may be missing from historical maps, with small streams represented as simple, straight blue lines that follow the valley centerline. This may give the misleading impression that small streams had, or still have, channels that were straight, single-thread, and static (Beechie et al., 2006; Hall et al., 2007). High-resolution topographic data from Light Detection and Ranging (LiDAR) technology now provides evidence that, prior to anthropogenic disturbance, small watercourses were capable of developing and maintaining river-wetland corridors with multi-threaded planforms (Figure 3).
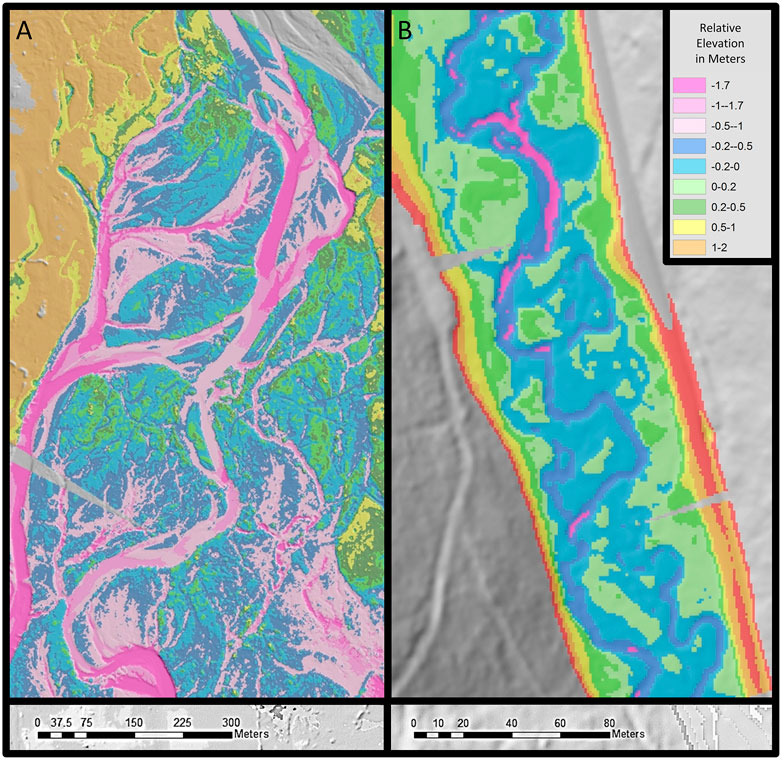
FIGURE 3. Complex, multi-threaded channel planforms can develop in relatively large and small river-wetland corridors: (A) Methow River, Washington, United States; (B) Summit Creek, Oregon, United States.
Historic place names can be more persistent, definitive, and revealing than maps and photographs (Brown et al., 2018). In North America, Native Americans have long used place names related to the presence of river-wetland corridors. Examples include the Entiat River in WA, United States, which means “place of grassy water” in Columbia-Moses, a Southern Interior Salish language; and the Muskegon River, Michigan, derived from the Odawa word “masquigon” for marshy river (Bright, 2004). Early European colonists introduced their own descriptive place names. The term “sausal,” which means “willow” in old, vernacular Spanish, is found in place names in California. GLO maps depict sausals in many California valleys (e.g., Grossinger, 2012) and the place name Sausalito literally means the place with “little willows.” The name of the Embarras River in Illinois has its roots in a French word indicating an “obstacle,” in this case referring to logjams and wood rafts that characterized the river when the French fur-trappers arrived. It is notable that every US state except Hawaii includes at least one Beaver Creek.
In Europe, and particularly in the United Kingdom, “Carr” is a common, ancient place name. It derives from old Norse and means “brushy marsh.” Star Carr in northern Yorkshire, United Kingdom, is an Early Mesolithic archaeological site that was occupied continuously for 200–500 years (Milner et al., 2011). Its valley location, artifacts, and name reveal the pre-historical landscape even though the watercourse is now just a series of highly modified ponds. The United Kingdom place name “Beverley” derives from the Old English words for beaver and lea(h), the latter indicating a “meadow or clearing.”
Current Sparsity
Evidence that river-wetland corridors were wet, widespread, and ecologically productive in the geologic, prehistoric, and recent past is irrefutable. It is well documented that until about two centuries ago, riverine wetlands were still widespread throughout northwestern and central Europe (Grootjans and Verbeek, 2002). However, most of Europe’s lowland rivers had been systematically channelized and their floodplains drained by the end of the 20th century. To understand why, it is necessary only to quote Johann Gottfried Tulla, the purposeful ‘Tamer of the Rhine’, who stated that, “As a rule, no river or stream needs more than one bed, not even the Rhine” (Cioc, 2002, p. 38). With respect to wetlands, between 50 and 90% of wetland ecosystems with organic soils have been lost (Klimkowska et al., 2007), and Krause et al. (2011) estimated that >80% of the remaining floodplain wetlands in northern Germany have been substantially altered since the 1950s.
European colonists carried their propensity for draining valleys and channelizing rivers with them to all corners of the globe. For example, the once-common “chain-of-ponds and meadows” valley floor form largely disappeared from southeastern Australia following European settlement (e.g., Mactaggart et al., 2008). An estimated 90% of New Zealand’s wetlands disappeared within 150 years of the onset of European settlement in 1840 (Ausseil et al., 2007).
Loss of river-wetland corridors is perhaps best documented in the United States. Between the 1780s and the 1980s, six states drained more than 85% of their wetlands and a further 16 drained at least 50% (Dahl and Allord, 1996). In the central prairies, >75% of riverine wetlands along the Platte River have been drained (Riggins et al., 2009) and half of all riparian areas in the arid to semiarid western United States, including their riverine wetlands, have diminished vegetation cover (Silverman et al., 2019). A similarly high degree of riparian disturbance and analogous wetland losses have occurred in montane wet meadows (Loheide et al., 2009).
River-wetland corridors across the United States have also been lost due to channel instability and planform metamorphosis (usually, from multi-thread to single-thread) attributable to a wide variety of anthropogenic actions. Losses may be traced to channelization, dam construction, river regulation, floodplain drainage, artificial levees, restoration to a stabilized single-thread channel, and urbanization (De Becker et al., 1999; Lewis, 2001; Grootjans and Verbeek, 2002). River-wetland corridors may also be degraded by intensive riparian grazing that causes loss of vegetation (Trimble and Mendel, 1995) or extirpation of beaver populations (Wolf et al., 2007; Loheide et al., 2009).
Many of the former river-wetland corridors that remain have been rendered dysfunctional due to dehydration following channel incision that disconnected the stream from its floodplain and drained the hyporheic aquifer (Cluer and Thorne, 2014). Others have been buried under legacy sediment associated with farming (e.g., Happ et al., 1940; Knox, 1987; Booth et al., 2009; Trimble, 2009), mining and logging (Pavlowsky et al., 2017), and other primary industries. Countless river-wetland systems lie buried beneath post-settlement valley fills in the heavily mill-dammed, mid-Atlantic region of the United States (Walter and Merritts, 2008; Merritts et al., 2011; Merritts et al., 2013). In this context, (Walter and Merritts 2008, p. 299) noted that, “… before European settlement, the streams were small anabranching channels within extensive vegetated wetlands.” Only patches of relict Holocene wet meadows remain extant, at locations where dams were not constructed, such as at sparse locations along Gunpowder Falls, Maryland (Merritts et al., 2011) and at Great Marsh, Pennsylvania (Martin, 1958).
Joyce (2014), p. 91 provides a stark assessment of the magnitude of historical loss of wetlands and their ecosystems, “it is likely that the wet grassland resource experienced losses of at least 80% during the 20th century…. In some countries or regions, such as Finland, eastern England, and mid/western United States, it is thought that less than 1% of the former area of wet grassland remains.”
Fundamental Drivers
Somewhat counterintuitively, a functional river-wetland corridor is not predicated by a wet climate, hence these corridors can be found even in an extremely arid zone like that around the River Nile between Khartoum, Sudan and Lake Nassar, Egypt. To understand how this works, consider that, as (Jeffres et al., 2020, p. 7) recently pointed out for California’s Central Valley, “Mean water residence times calculated for the floodplain agricultural wetland, perennial drainage canal and Sacramento River were 2.15 days, 23.5, and 1.7 s, respectively.” It is the longer relative residence time of the water in connected floodplain systems, along with their ability to store groundwater and support riparian vegetation, that give the impression of abundant flow. Thus, a functional river-wetland corridor reflects the presence of a floodplain water table at or near the ground surface throughout most of the year. This high groundwater table and associated abundant surface water can result directly from geologic, biotic, or geomorphic controls, or some combination of these influences (Figure 4). In essence, we view geology, biota, and geomorphology as primary drivers of river-wetland corridors and hydrology as a secondary driver.
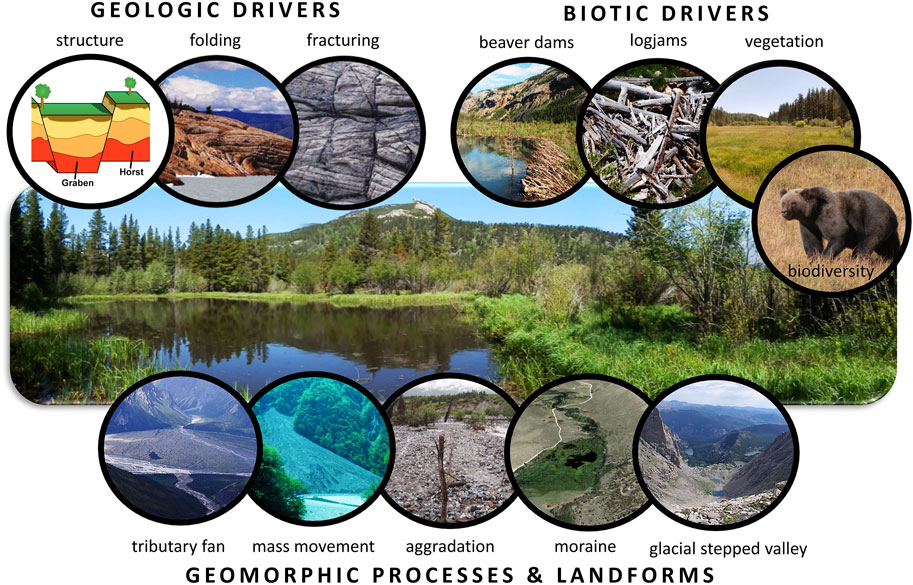
FIGURE 4. Geologic drivers, biotic drivers, and geomorphic processes and landforms that can promote formation of a river-wetland stream corridor (inset “structure” diagram from usgs.gov).
Geologically, the distinguishing characteristics of a river-wetland corridor are:
(i) a valley floor sufficiently wide to support partially to completely laterally unconfined channel planforms,
(ii) a shallow alluvial aquifer with sufficient capacity and connectivity to keep the river corridor persistently wet, and
(iii) a downstream grade control or valley constriction that acts as the local base level for the upstream reach and modulates longitudinal sediment movement.
The water that hydrates the river-wetland corridor may be predominantly hyporheic (i.e., a component of flow in the river), but may also come from perirheic sources controlled by geology. Geological drivers of upwelling in regional groundwater include grabens that retain groundwater inputs from adjacent uplands (e.g., Koltzer et al., 2019). For example, in the Pantanal of Brazil, Bolivia, and Paraguay, which may be the largest tropical river-wetland corridor, differential subsidence along active faults has created a vast, low-lying sedimentary basin featuring both seasonally and permanently flooded areas (Assine et al., 2015). Similarly, fault scarps in rift valleys can produce drainage systems with wet floodplains that terminate in endorheic lakes like Natron, Eyasi, Manyara, Rukwa, and Balangida in Tanzania (Mwanukuzi, 1993).
Smaller-scale geological drivers of river-wetland formation include:
(i) folding of aquifers (e.g., DesRoches et al., 2014);
(ii) lithologic contacts between units of differing hydraulic conductivity (e.g., Muldoon et al., 2001);
(iii) resistant lithologies that form a local base level and create wet, low-gradient depositional zones upstream (e.g. Klip River, South Africa (Tooth et al., 2002; Tooth and McCarthy, 2007)); and
(iv) undulating bedrock topography that facilitates high water tables where the depth to bedrock decreases locally with distance downstream along the river (e.g., Hardie et al., 2012).
Reach-scale variations in fracture density can also influence valley geometry. Densely fractured bedrock lowers resistance to weathering and erosion, allowing a relatively wide valley floor to form (Ehlen and Wohl, 2002). Dense fractures also support a bedrock aquifer that can generate upwelling of deep groundwater (Briggs and Hare, 2018). Conversely, a relatively shallow impermeable layer or fragipan, such as might be created by lacustrine deposits (e.g., Sudan’s Sudd wetlands along the White Nile upstream of Khartoum (Salama, 1987)) or silica-rich layers, can create a perched aquifer that may be recharged by hyporheic or shallow, perirheic flows (e.g., Bockheim and Hartemink, 2013).
Biologically, the salient features of a functional river-wetland corridor are:
(i) dense riparian and wetland vegetation;
(ii) high organic matter content in floodplain soil; and
(iii) biodiversity.
Aquatic, riparian, and floodplain plants increase drag and surface roughness, resist erosion, and create obstructions to flow both within active channels and across the valley floor (e.g., Collins et al., 2012; Aberle and Järvelä, 2013). In so doing, plants slow surficial flows, augment overbank flows, and promote the influent stream conditions that help to hydrate a river-wetland corridor. Other biotic features documented as being capable of driving hyporheic exchange and influent stream conditions include: beaver dams (Polvi and Wohl, 2012); large wood, especially in the form of logjams or wood rafts (e.g., Triska, 1984; Miller et al., 2012; Larsen et al., 2016); and dense stands of aquatic, herbaceous, and/or shrubby vegetation (Larsen and Harvey, 2011; Larsen, 2019). The capacity of woody and shrubby vegetation to “clog” streams has long been recognized—hence the penchant of drainage authorities for channel maintenance that involves “clearing and snagging” (Shields and Nunnally, 1984; Harmon et al., 1986; Gippel, 1995). However, in low gradient fluvial systems like the Florida Everglades (Larsen and Harvey, 2010) and many mountain meadows, dense covers of native grasses create sufficient resistance to flow and erosion to mediate channel form and migration, promote sediment retention, and support a diverse and dynamically stable river-wetland corridor (Micheli and Kirchner, 2002a; Micheli and Kirchner, 2002b; Slocombe and Davis, 2014).
High organic matter content in floodplain soils can increase soil cohesion (Zhang and Hartge, 1990) and tensile strength (Rahimi et al., 2000) and thus resistance to erosion. High organic matter content can also increase porosity and permeability and therefore surface-subsurface hydrologic connectivity.
Biodiversity refers to the diversity of organisms that can influence river processes, from biofilms and macroinvertebrates that help to stabilize fine sediment (Albertson et al., 2019; Gerbersdorf et al., 2020), through crustaceans (Johnson et al., 2011) and fish (Hassan et al., 2008) that alter the grain-size distribution and cohesion of streambed sediments, to apex predators that limit grazing intensity in the river corridor and therefore influence the vegetation communities and food and habitat for other animals such as beaver that are dependent on riparian vegetation (Beschta and Ripple, 2012).
Geomorphic processes and landforms can promote formation of river-wetland corridors and they commonly generate within-reach variability by creating local or temporary base levels and/or valley width constrictions. Landforms that may constrict the valley floor include:
(i) alluvial tributary fans (e.g., Miller et al., 2012) and
(ii) run-out deposits, fans, and lobes generated by valley side slope failures such as
a. rock falls (Schrott et al., 2003),
b. rock avalanches,
c. landslides,
d. solifluction lobes, and
e. debris flows (Swanger et al., 2010; Booth et al., 2020).
A geomorphically-generated valley floor constriction must be relatively large and enduring in order to impede flow and sediment transport in the trunk stream sufficiently to create an upstream depositional reach with a high water table (Fryirs et al., 2007). Elevated sediment inputs from tributaries and valley side slopes that can create such relatively large landforms may be triggered by particular disturbances such as volcanic eruptions (e.g., Del Moral, 1999) or wildfires (e.g., Heede et al., 1988; Benda et al., 2003). Excessive and protracted sediment inputs from the catchment and trunk stream upstream can also cause so much downstream valley alluviation that surficial runoff switches from single-channeled to multi-threaded or even sheet flow spread across the valley floor - a scenario that can persist for decades in river corridors where high sediment loads prevail (e.g., Fryirs et al., 2007; Martin-Vide et al., 2014; Major et al., 2019).
Figure 5 relates planform variation to the influence of geomorphic landforms, which generate local variability in valley floor width, hydrologic connectivity, and partitioning of water between surface and subsurface flows within a single, contiguous river-wetland corridor. The expression of diverse planforms in this example is dynamic in time as well as space, changing in response to fluctuations in discharge, sediment flux (fluvial and upland inputs), vegetation growth and dieback, and the actions of animals ranging in size from algae and macroinvertebrates to large herbivores and beaver (Johnson et al., 2019). The hyporheic segment of river flow is in very close proximity to the valley-floor surface and at base flow supports a mosaic of features with and without surface hydrologic connectivity. This river-wetland corridor includes valley-spanning wetland complexes, a single-thread channel, a segment with no channel, spring brook heads, and anabranching channels.
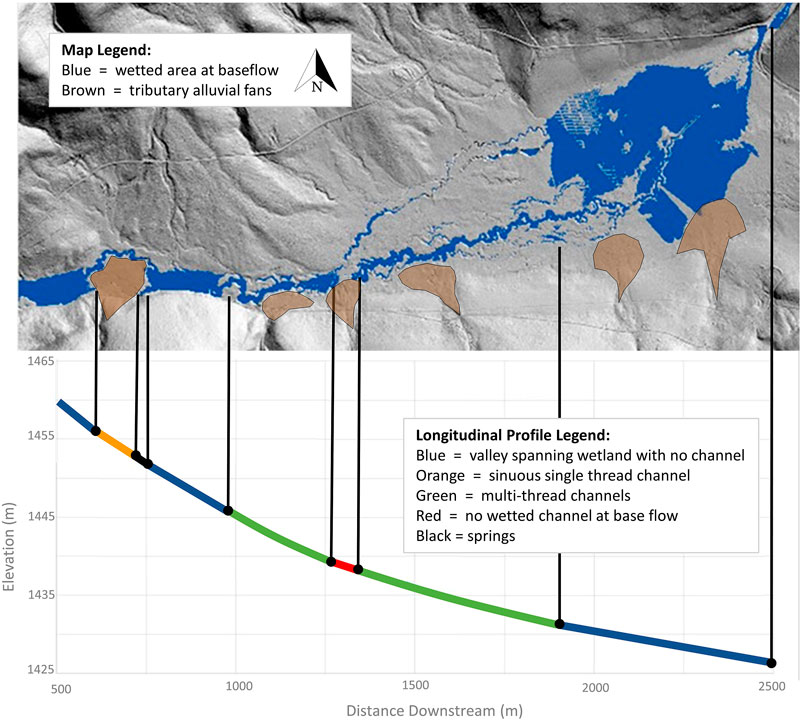
FIGURE 5. Example of variability in channel planform and partitioning of water between channels, wetlands, and the shallow aquifer within a single, contiguous river-wetland corridor. Large tributary fans on the northern boundary are easily discernible, while smaller fans on the southern boundary have been highlighted to show their influence on fluvial processes within this continuous valley. North Fork Crooked River, Oregon, United States.
Glacial moraines that act as a local base level and temporarily dam a stream can create a depositional zone upstream from the moraine (Cooper et al., 2012) and glacio-fluvial deposits in valley sandurs and outwash plains usually have a high water table (e.g., Scheffel, 2018; Perera, 2020), making them potential reaches for river-wetland corridor formation. Alpine glaciers also tend to create a stepped valley floor topography, with low-gradient and steeper segments of valley floor alternating downstream (MacGregor et al., 2000). The low-gradient valley segments may support river-wetland corridors (e.g., Arp et al., 2007).
Interactions and Feedbacks
Although it was convenient to introduce and describe the attributes, drivers, processes, and landforms associated with river-wetland corridors separately, it is their interactions that govern hydrologic connectivity, channel form and evolution, and vegetation dynamics. Continuous changes in the relative influences of the drivers and processes, coupled with the complexity of nested feedback loops between river-wetland corridor morphologies and ecosystems, make these systems both responsive to change and resilient to natural disturbance. Nevertheless, river-wetland corridors are vulnerable to the impacts of anthropogenic activities. We first describe the interactions that occur in natural systems and then discuss how a wide array of human activities alter these interactions.
In a pristine river-wetland corridor, geology and geomorphic processes interact to create a characteristic valley geometry with a relatively wide valley floor and gentle downstream valley gradient. Geology, biotic communities, and geomorphic processes then interact to create degrees of lateral and vertical connectivity that are similar in magnitude to that of longitudinal connectivity. These same fundamental drivers also govern the inputs of water, sediment, and organic matter to the river corridor and, in turn, these inputs interact with the valley characteristics to influence river form and process (Figure 6).
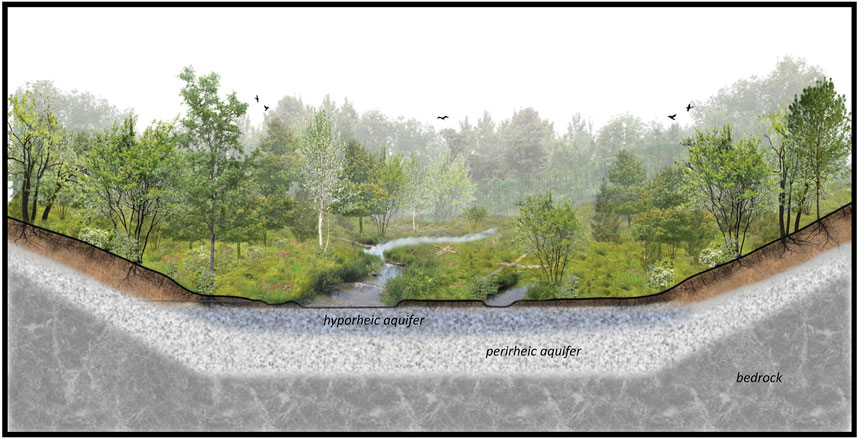
FIGURE 6. Schematic representation of a fully connected stream corridor where biogeomorphic processes (e.g. large wood, beaver, vegetation) and river-wetland attributes (e.g. valley geometry, channel planforms, channel migration, hyporheic and regional aquifers) interact in complex ways, via multiple, nested feedback loops. Original illustration provided by LandStudies, Inc., Pennsylvania (with permission from Land Studies, 2021).
Channel form, conveyance capacity, and boundary conditions then interact with water, sediment, and organic inputs to influence rates and distributions of lateral channel migration. However, the relationship between processes driving and resisting lateral migration is complicated by two, competing feedback loops. In the first feedback loop, lateral migration influences the distribution, density, and erosional resistance of floodplain vegetation by determining the turnover time and spatial heterogeneity of valley-floor surfaces (e.g., Fetherston et al., 1995; Beechie et al., 2006). In the second feedback loop, vegetation moderates the influence of lateral migration by increasing hydraulic roughness and reinforcing floodplain soils, which act to increase the erosional resistance of channel banks, while also facilitating deposition of coarser sediments that form natural levees and fine, cohesive sediments that resist re-entrainment (e.g., Micheli and Kirchner, 2002a; Allen et al., 2018).
Vegetation type and density, which reflect the regional climate, biome, and depth to alluvial aquifer, also influence the amount and spatial distribution of large wood in the stream corridor (e.g., Wohl et al., 2017b) and, in the northern hemisphere, the presence of beaver (Castor canadensis in North America, C. fiber in Eurasia) (Pollock et al., 2017). Obstructions within the active channel(s) and floodplain associated with the presence of vegetation, large wood, and beaver dams increase subsurface hydrologic connectivity and hyporheic exchange flows (e.g., Sawyer and Cardenas, 2012; Wang et al., 2018; Doughty et al., 2020). Similarly, these obstructions can further influence surface hydrologic connectivity by promoting lateral channel migration (e.g., Eaton and Hassan, 2013), formation of a multi-threaded channel planform (John and Klein, 2004; Collins et al., 2012; Polvi and Wohl, 2013), and enhanced overbank flows (Brummer et al., 2006; Westbrook et al., 2006). Local variability in hydrologic connectivity, in turn, influences the elevation of the floodplain water table, which directly affects the types, densities, and vitality of vegetation. This reflects an additional, strongly positive feedback between enhanced hydrologic connectivity and biologic processes.
Stratigraphic and geochronological studies of river-wetland corridors indicate that feedbacks among water, sediment, and biota can create and maintain a dynamically stable alluvial valley floor that may endure for centuries to millennia (e.g., Gibling et al., 1998; Polvi and Wohl, 2012). This is evidence that a fully functioning river-wetland corridor is not a transient state but a dynamically stable river type that is naturally resilient to all but major, step-change disturbances (such as tectonic uplift or sea level decline).
This resilience to disturbance stems from the bio-geomorphological complexity and nested feedback loops that create and maintain river-wetland corridors. Hey (1978) proposed that a single channel has nine degrees of freedom in adjusting its morphology to accommodate changes in boundary conditions and inputs. In a multi-threaded river-wetland corridor the number of degrees of freedom increases linearly with the number of channels and the number of possible combinations of ways of accommodating disturbance grows rapidly to hundreds of thousands or even millions (see Supplementary Material). Leopold (1994) demonstrated that river responses to disturbance are distributed among all available degrees of freedom. In essence, when subject to an external perturbation, a river-wetland corridor can diffuse the impact of that disturbance through thousands of small adjustments that are distributed throughout the complex river corridor. This helps explain why a river-wetland corridor is capable of absorbing substantial disturbances while staying within the bounds of its dynamic stability.
In a fully functional river-wetland corridor, this intrinsic, geomorphic resilience to disturbance is further enhanced by riverine biota. Beaver modifications of the river corridor increase resilience to drought (Hood and Bayley, 2008) and wildfire (Fairfax and Whittle, 2020). The dense vegetation of river-wetland corridors can enhance the resistance of these systems to flood-induced erosion and deposition (Heffernan, 2008). Dense wetland vegetation can also create a condition of dynamic stability in which changes in hydrology drive shifts in the areas of emergent vegetation versus open water (Larsen and Harvey, 2010). Additionally, and significantly, the recuperative capacity of vibrant river-wetland ecosystems gives the system a capacity to “self-repair” that simpler, water- and sediment-dominated streams do not exhibit to the same degree. This not only hastens post-disturbance recovery and shortens relaxation times, but can also increase ecosystem resilience to future disturbances (Johnson et al., 2019).
The dynamically stable and resilient nature of river-wetland corridors stems from their capacity for continuous, small adjustments that accommodate fluctuations in the influences of hydrology, geology, and biota on river form and process (Castro and Thorne, 2019). Externally driven, episodic changes such as major floods, volcanic eruptions, or wildfires significantly disturb the intricate balance between water, sediment, and biota (Kleindl et al., 2015), but the path by which the biogeomorphic system has evolved triggers systemic interactions that diffuse, mitigate, and ultimately absorb all but the most profound and persistent shocks. For example, when successive generations of beavers shift the locations of dams and ponds across a river corridor over periods of decades to centuries, they create a spatially heterogeneous river corridor (Laurel and Wohl, 2019). Many investigators have described the characteristics and importance of spatial heterogeneity in river-floodplain systems (Wohl, 2016), leading to descriptions such as “shifting habitat mosaics” (Arscott et al., 2002; Stanford et al., 2005). In a river-wetland corridor, the details of the riverscape change daily, giving it greater fluidity even than a shifting mosaic. We therefore propose the term “kaleidoscope river” to describe the self-organizing tessellation of the polydimensional river-wetland corridor, a system continually adjusting to internal and external perturbations in ways that collectively maintain configurations that are robust and self-reinforcing. A kaleidoscope is essentially a continually changing mosaic, so using the word kaleidoscope in this context emphasizes the continually dynamic nature of river-wetland corridors.
Anthropogenic Modifications and Contemporary Remnants
Despite their natural resilience, river-wetland corridors can be destabilized by diverse forms of human-induced disturbance. River-wetland corridors worldwide have been and still are commonly altered for flood control, navigation, hydropower generation, downstream transport of commodities such as cut logs, and to open access to agriculturally rich bottomlands through land drainage (e.g., Schrautzer et al., 1996; Schneider and Eugster, 2007). Channel modification routinely involves channelization (e.g., Brookes, 1988; Pisut, 2002; Mitsch and Day, 2006; Brown et al., 2018) and removal of naturally occurring wood rafts, logjams, and individual wood pieces (e.g., Harmon et al., 1986; Wohl, 2014). The almost complete eradication of beaver populations for the commercial fur trade (e.g., Nolet and Rosell, 1998; Pollock et al., 2017) also caused widespread hydrologic disconnection and simplification of river-wetland corridors. In the United States, national programs such as the Swamp Land Act of 1850 transferred title of federally owned wetlands to states that would agree to drain the land and convert it to agricultural uses. This federal legislation hastened the destruction of millions of hectares of wetlands (e.g., 8.1 million ha in Florida, 3.5 million ha in Louisiana) (Guirard and Brassieur, 2007). Analogously, landscape drainage overseen by Dutch engineers resulted in widespread degradation of the English Fens during the middle decades of the 17th century (Williamson, 2006).
Even if riverscapes are not altered directly, changes in land use and water management can result in significant changes to flow regimes, sediment loads, valley floor and channel geometries, water surface slopes, and riparian biota. This is evidenced particularly by human impacts on catchment inputs to drainage networks and the degrees to which water, sediment, and wood moving through the river corridor are exchanged between the channel, floodplain, and hyporheic zones of the river.
In this context, it is difficult to overstate the significance of the complex, nested feedback loops outlined above, within which a change in any of the driving variables can be accommodated through myriad, subtle changes in the configuration and dynamics of the river-wetland corridor, while maintaining continuity of the river functions. Removing or converting vegetation, large wood accumulations or beaver dams, for example, can reduce lateral surface and subsurface hydrologic connectivity, reduce channel migration rates, and prompt a multi-threaded system with low-conveyance and limited downstream connectivity to metamorphose into a single-thread, high-conveyance channel (Collins et al., 2002; Wolf et al., 2007). Similarly, removal of logjams, beaver dams, and other temporary, local base level controls can trigger channel incision and lowering of the floodplain water table, which drains floodplain aquifers and wetlands (e.g., Larsen et al., 2016) and converts riparian vegetation to upland species. Removal of apex predators can result in unnaturally intensive riparian grazing by large ungulates, leading to loss of vegetation and beaver that can result in desiccation of the river-wetland corridor and channel incision (e.g., Beschta and Ripple, 2006; Beschta and Ripple, 2012).
Construction of levees or embanked roads artificially reduces valley floor width, which may transform a multi-thread planform to a single-thread, high-conveyance channel. Reduced flood flows and sediment supply in regulated rivers can also decrease channel migration rates (e.g., Bradley and Smith, 1984), limit surface and subsurface hydrologic connectivity (e.g., Kingsford, 2000), lower the floodplain water table (Mahoney and Rood, 1991), and allow xeric, upland vegetation to encroach into the floodplain (e.g., Caskey et al., 2015). In other circumstances, increased base flows and reduced flood peaks can allow floodplain vegetation to increase bank erosional resistance, raise the floodplain water table, and cause a braided river to assume a meandering or anastomosing planform (e.g., Williams, 1978; Nadler and Schumm, 1981).
Less direct, but perhaps no less consequential impacts to river-wetland corridors may stem from landscape-scale changes in vegetation beyond the valley floor. Benda et al. (2003), for example, concluded that timber harvest and fire suppression can substantially alter the frequency and magnitude of natural disturbances, such as fire-induced mass movements that create or enlarge the alluvial fans that sustain some river-wetland corridors. These types of effects are pervasive across large, relatively dry and fire-prone areas of the world, such as the Mediterranean region and the western United States (Shakesby and Doerr, 2006).
Despite extensive modification and loss of river-wetland corridors, examples of this type of riverine environment can still be found. Typically, functional river-wetland corridors remain in mountainous or high-latitude areas of the world that are too cold for farming; in exceptionally wet areas where either the land could not be effectively drained or salinization destroyed soil fertility; and in extremely large river systems where channelization was not economically feasible (e.g., portions of the Amazon and Congo River basins; Keddy et al., 2009).
Contemporary examples can be found in locations ranging from the few remaining relatively natural river deltas, such as those of the Yukon, Mackenzie, and Lena Rivers, through estuarine floodplains and tidal marshes (e.g., Salmon River, OR, United States), to headwater streams and wet meadows. Many of these, however, are relatively small remnants of formerly more longitudinally and laterally extensive river-wetland corridors, Larger, intact river-wetland corridors also remain in parts of Africa, Central Asia, Siberia, and South America. Table 1 and Figure 1 highlight a few, diverse examples of these remnants.
Significance
The significance of river-wetland corridors as a river type extends from local scales to cumulative effects at regional and global scales. This significance stems from the diverse river functions that river-wetland corridors provide (Figure 7). Major river functions can be grouped into conveyance, storage and processing, habitat and biodiversity, and resilience.
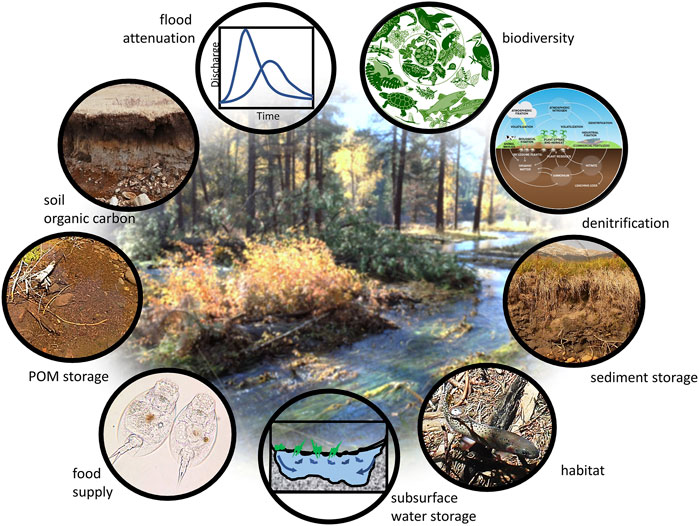
FIGURE 7. Schematic illustration of river functions associated with river-wetland corridors (“biodiversity” image modified from Nelson et al. (2006); “denitrification” diagram from ibiologia.com; “food supply” image (rotifers) from Matthew A. Robinson, Wikimedia Commons).
The primary function of a river is to convey water, sediments, and organic materials from upstream sources to downstream sinks. In this context, based on the arguments and explanations set out in previous sections, river-wetland corridors play an important role in modulating downstream connectivity and locally promoting enhanced lateral and vertical connectivity. Studies of contemporary rivers with valley segments occupied by remnant river-wetland corridors indicate the important contributions of this river type to the geomorphic and ecological functionality of a river system (Poff et al., 2007; Peipoch et al., 2015).
Intact river-wetland corridors attenuate downstream fluxes of water, solutes, sediment, and particulate organic matter (POM) and, in so doing, enhance organic carbon storage (e.g., Robertson et al., 1999; Johnston, 2014; Sutfin et al., 2016; Laurel and Wohl, 2019; Sutfin and Wohl, 2019) and denitrification (e.g., Richardson et al., 2004; Racchetti et al., 2010). The temporally limited longitudinal connectivity that river-wetland corridors impart to the fluvial system can be beneficial, especially when accompanied by enhanced lateral and vertical connectivity (Battin et al., 2008; Burchsted et al., 2010; Wohl, 2017). Increased lateral and vertical connectivity amplifies the capacity of river-wetland corridors to store sediment and adsorbed contaminants, and the saturated, reducing environment and high primary productivity typical of a river-wetland corridor (Edwards et al., 2020) can support high concentrations of soil organic carbon (e.g., Keddy et al., 2009; Sutfin et al., 2016; Lininger et al., 2018). Field quantification of river corridor carbon storage establishes that the reaches in a stream network with well-connected, wet floodplains can have disproportionately high soil organic carbon stock compared to other, drier valley-floor segments (Wohl et al., 2012; Wohl et al., 2017a; Sutfin and Wohl, 2019). They can also be disproportionately important at the catchment-scale in terms of storing far more organic carbon per unit area than adjacent uplands (Wohl et al., 2012), suggesting that river-wetland corridors could, and probably should, be effectively targeted for restoration intended to enhance carbon sequestration (Wohl et al., 2018).
Hydraulic complexity and the abundance of low-velocity areas within a river-wetland corridor can facilitate retention of POM (e.g., Jones and Smock, 1991), especially where extensive backwater areas are created by logjams (Beckman and Wohl, 2014; Livers et al., 2018) or beaver dams (e.g., Naiman et al., 1986). In turn, POM retention increases the capacity of these systems to support fish and wildlife because food availability is a first-order control on biological populations (Lindeman, 1942; Odum et al., 2005).
Some river-wetland corridors are predominantly forested and these shaded systems rely on POM shed by floodplain forests as an energy source (e.g., Mulholland, 1981; Thompson and Townsend, 2005). Rivers in wet meadows are less shaded than those in forests, so that instream photosynthesis by algae, bryophytes, and aquatic macrophytes, along with the presence of decaying floodplain vegetation, provide the base for the aquatic food web (e.g., Thorp et al., 1998). Retention of POM remains important, however, both as a food source and as habitat for microbial and macroinvertebrate communities (Tank et al., 2010; Edwards et al., 2020).
By facilitating in-channel and, especially, overbank storage of fine sediment and adsorbed contaminants such as heavy metals, synthetic chemicals (Wohl, 2015), and excess phosphorus (Reddy et al., 1999), river-wetland corridors also provide opportunities for microbial communities that promote denitrification to access more slowly moving surface and subsurface waters, which helps to improve water quality. High rates of hyporheic exchange in river-wetland corridors can also dampen seasonal and daily temperature fluctuations in surface waters (e.g., Loheide and Gorelick, 2006; Weber et al., 2017) in a manner beneficial to fish and other aquatic organisms.
Functional river-wetland corridors provide both abundant and diverse aquatic and riparian habitat (e.g., Sayer, 2014; Entwistle et al., 2019) and corridors for species migration and dispersal (e.g., Antas, 1994). The spatial and temporal sequence of disturbances associated with processes such as lateral channel movement, formation of logjams (Collins et al., 2012), and construction and then infilling of beaver ponds (Wright et al., 2002; Stevens et al., 2006) creates a patchy, shifting pattern of surface and subsurface habitats. These habitats have diverse grain-size distribution, elevation, hydraulic conductivity, hydrologic connectivity, inundation regimes, organic carbon concentrations, and nutrient availability. Because of this diversity, floodplain wetlands are a rich source of food for juvenile salmonids (Katz et al., 2017), which can be acquired directly by the fish accessing the floodplain or can be delivered to the channel network by floodplain return flows (Jeffres et al., 2020).
Wetlands and beaver ponds of varying age and size support juvenile salmonids, but also create critical habitat diversity for wetland plants, amphibians, and other species that require a reliable water source (Stevens et al., 2006; Popescu and Gibbs, 2009). This increased “density diversity” enhances the opportunity for aquatic species to find the appropriate life-history habitat within a relatively small area, effectively reducing migration distances and energy expenditure. The hydraulic and habitat heterogeneity associated with functioning river-wetland corridors decreases the distances between various habitat niches so that aquatic and wetland species do not need to move as far to fulfill diverse thermal and trophic needs (Armstrong and Schindler, 2013). Spatial variations in flow depth and velocity also allow aquatic and wetland species to self-segregate. For example, juvenile salmonids can find refuge in shallow water areas away from piscivorous fish (Brown and Moyle 1991), while different species of pond-breeding amphibians have higher survival rates where beaver ponds of differing age and hydrologic connectivity provide habitats with and without fish (Cunningham et al., 2007).
The river functions described above result in expansive areas with higher densities of biomass and higher biodiversity within river-wetland corridors than may be present in more laterally confined portions of a stream network (e.g., Thorp et al., 2010; Bellmore and Baxter, 2014; Shi et al., 2017). Beaver-modified valley floors, for example, have higher biodiversity of aquatic and wetland plants (Wright et al., 2002), aquatic and terrestrial insects (e.g., Hood and Larson, 2014), amphibians (e.g., Karraker and Gibbs, 2009), fish (e.g., Smith and Mather, 2013), birds (e.g., Aznar and Desrochers, 2008), and mammals (Rosell et al., 2005; Hauer et al., 2016). Greater biodiversity, coupled with the higher levels of habitat complexity and diversity described previously, may increase the stability of biological populations subject to significant environmental variability (Bellmore et al., 2015). This in turn increases the range, value, and reliability of a suite of ecosystem services highly valued by society (Ekka et al., 2020).
An important, tertiary river function provided by river-wetland corridors is that of enhancing the resistance and resilience of the river corridor to both natural and human disturbances. Resistance describes the ability of a system to remain essentially unchanged when subject to disturbance (e.g., Simon, 2009). Resilience is the persistence of relationships within a system and is a measure of the ability of the system to absorb changes of state and driving variables (Holling, 1973). River-wetland corridors are generally more resistant to drought and wildfire because of the elevated water table and relative abundance of standing water (e.g., Hood and Bayley, 2008; Fairfax and Whittle, 2020). Sediment pulses that can severely damage dysfunctional channels in the aftermath of wildfire are instead distributed and attenuated across functional river-wetland corridors, resulting in less significant impacts. River-wetland corridors can also be more resistant to floods because wide, vegetation-stabilized floodplains and, in some cases, the presence of multiple channels, attenuate flood peaks and reduce hydraulic force per unit area exerted against the channel and floodplain boundaries (e.g., Hillman, 1998; Nilsson et al., 2018). Related to these characteristics, river-wetland corridors are particularly suited to subsurface water storage (e.g., Loheide and Gorelick, 2007), a river function that may help to support or enhance local and downstream base flows (Wegener et al., 2017).
Implications for River Restoration
River restoration, as commonly practiced today, reflects an aesthetic preference for a well-defined single channel that is relatively stable (Kondolf, 2006; Le Lay et al., 2013; Medel et al., 2020; Wilson et al., 2020), with channel conveyance that limits the frequency and duration of floodplain inundation. This results in restored rivers with channel geometries, planforms, and capacities for conveying water, sediment, and organic matter that mimic those characteristic of transport reaches (i.e., reaches that pass excess sediment downstream; Montgomery and Buffington, 1997). Channels with these characteristics are suitable restoration targets for valley segments with relatively narrow valley floors and floodplains, relatively high channel gradients, strong downstream connectivity, and limited capacity for surface or subsurface storage (Jeffres et al., 2020). However, mimicking transport channels is less suited to wider, lower-gradient, depositional valley reaches that, prior to anthropogenic disturbance, featured the multi-threaded, response channels (i.e., reaches that were likely to accumulate excess sediment; Montgomery and Buffington, 1997) that are characteristic of river-wetland corridors.
Maintenance or restoration of a high-conveyance, transport channel where there would naturally be a response channel limits lateral and vertical connectivity within the river corridor, missing the opportunity to re-create the functions of the lost river-wetland corridor and perpetuating the conditions within which a defined single-thread channel is bounded by a relatively dry, former floodplain. What could be above- and below-ground storage areas for water, sediment, organic matter, and nutrients remain sources as the valley floor continues to be functionally disconnected and efficiently drained. This inhibits recovery of the complex interactions and feedback loops between biogeomorphic processes and river-wetland attributes described above and illustrated in Figures 4 and 7, as well as degrading the habitat networks provided by hydrologically connected and dynamically stable channels and floodplain wetlands (Sayer, 2014). In essence, replacing a dynamically stable river-wetland corridor with a more static channel that is largely disconnected from the floodplain changes a kaleidoscope river to the fluvial equivalent of a monochromatic photograph.
In the past, most river networks included response reaches featuring river-wetland corridors that were interspersed with source and transport reaches. Response reaches are known to provide a range of intrinsically valuable river functions. This suggests that river management and restoration should recognize the former abundance of this river type in diverse landscape settings. Management and restoration should include river-wetland corridors in conceptualizations of desirable river types and should, where appropriate and feasible, consider river-wetland corridors as potential, alternative targets for river conservation and restoration projects. Given the multi-faceted significance of river-wetland corridors, investing in their re-creation can represent a wise use of the limited funds available to support river restoration.
Restoration explicitly designed to restore river-wetland corridors is gradually starting to occur, as exemplified by wet-meadow restoration in Europe (Grootjans and Verbeek, 2002; Klimkowska et al., 2007) and the United States (Booth et al., 2009; Riggins et al., 2009; Hartranft et al., 2011; Silverman et al., 2019; Voosen, 2020), “stage 0” restoration projects in the United States Pacific Northwest (Powers et al., 2019), and beaver reintroduction and mimicry efforts throughout the United States (Pollock et al., 2014; Bouwes et al., 2016; Pollock et al., 2017), Canada (Muni and Westbrook, 2021), Europe (e.g., Nolet and Rosell, 1998; Fustec et al., 2001), and across the United Kingdom (Puttock et al., 2015). To be successful, restoration requires a sound understanding of past and present conditions and trajectories, informed by analyses of historical records and key biogeomorphic processes across a range of spatial and temporal scales (e.g., Woelfle-Erskine et al., 2012; Rathburn et al., 2013; Brierley and Fryirs, 2016). Many existing restoration efforts focus on small to medium-sized rivers. Although large floodplain rivers have already been extensively modified in the temperate latitudes and are threatened by projected human alterations in both tropical and high latitudes (e.g., Erӧs et al., 2019), these rivers can be more difficult to restore because of the presence of multiple land owners and jurisdictions, as well as numerous constraints created by infrastructure and consumptive water uses. Nevertheless, larger scale manipulations of river-corridor topography and flow regime designed to restore the hydrologic connectivity that supports river-wetland corridors can be undertaken to beneficial effect, as in the case of the Kissimmee River in Florida, United States (Koebel and Bousquin, 2014).
In closing, we stress that not every reach in a river network is a candidate for river-wetland corridor restoration, or even river-floodplain reconnection. However, in order for impaired systems to fulfill their potential to deliver multiple functions and benefits, at least some of the wider, flatter, alluvial valley segments that punctuate source and transport reaches should feature river-wetland corridors. This is achievable if restoration practitioners and regulators embrace two paradigm shifts. The first hinges on acknowledging the past abundance of river-wetland corridors and appreciating their potential to contribute to the functionality and resilience of river systems in what is an uncertain future. The second rests on broadening our view of the river, by thinking outside the channel.
Author Contributions
All of the authors contributed to writing the text and compiling the information in the table by adding relevant information, examples, and references; JC, EW, and PP are primarily responsible for the figures with the exception of Figure 6, which is used courtesy of LandStudies.
Funding
In part, this work was supported by the Engineering and Physical Sciences Research Council, United Kingdom (grant number EP/P004180/1).
Disclaimer
The findings and conclusions in this manuscript are those of the authors and do not necessarily represent the views of NOAA-Fisheries, the US Fish and Wildlife Service, or the US Forest Service.
Conflict of Interest
The authors declare that the research was conducted in the absence of any commercial or financial relationships that could be construed as a potential conflict of interest.
Acknowledgments
We would like to thank Ryan Bellmore for his insights on the capacity of ecosystems to support fish and how habitat complexity and diversity confer stability to biological populations. We also would like to thank special issue editors Fernando Nardi, Ryan Morrison, Paola Passalacqua, and Ryan McManamay for the invitation to contribute this paper. We thank Lyle Zevenbergen for preparing the spreadsheet on river degrees of freedom that is included in the Supplemental Material.
Supplementary Material
The Supplementary Material for this article can be found online at: https://www.frontiersin.org/articles/10.3389/feart.2021.653623/full#supplementary-material
References
Aberle, J., and Järvelä, J. (2013). Flow Resistance of Emergent Rigid and Flexible Floodplain Vegetation. J. Hydraul. Res. 51, 33–45. doi:10.1080/00221686.2012.754795
Albertson, L. K., Sklar, L. S., Cooper, S. D., and Cardinale, B. J. (2019). Aquatic Macroinvertebrates Stabilize Gravel Bed Sediment: A Test Using Silk Net-Spinning Caddisflies in Seminatural River Channels. PLoS One 14, e0209087. doi:10.1371/journal.pone.0209087
Allen, D. C., Wynn-Thompson, T. M., Kopp, D. A., and Cardinale, B. J. (2018). Riparian Plant Biodiversity Reduces Stream Channel Migration Rates in Three Rivers in Michigan, USA. Ecohydrology 11, e1972. doi:10.1002/eco.1972
Antas, P. T. Z. (1994). Migration and Other Movements Among the Lower Paraná River valley Wetlands, Argentina, and the South Brazil/Pantanal Wetlands. Bird Conserv. Int. 4, 181–190. doi:10.1017/S0959270900002768
Armstrong, J. B., and Schindler, D. E. (2013). Going With the Flow: Spatial Distributions of Juvenile Coho Salmon Track an Annually Shifting Mosaic of Water Temperature. Ecosystems 16, 1429–1441. doi:10.1007/s10021-013-9693-9
Arp, C. D., Schmidt, J. C., Baker, M. A., and Myers, A. K. (2007). Stream Geomorphology in a Mountain Lake District: Hydraulic Geometry, Sediment Sources and Sinks, and Downstream Lake Effects. Earth Surf. Process. Landf. 32, 525–543. doi:10.1002/esp.1421
Arscott, D. B., Tockner, K., Van der Nat, D., and Ward, J. V. (2002). Aquatic Habitat Dynamics along a Braided Alpine River Ecosystem (Tagliamento River, Northeast Italy). Ecosystems 5, 0802–0814. doi:10.1007/s10021-002-0192-7
Assine, M. L., Merino, E. R., Pupim, F. N., Warren, L. V., Guerreiro, R. L., and McGlue, M. M. (2015). “Geology and Geomorphology of the Pantanal Basin,” in Dynamics of the Pantanal Wetland in South America. Editors Bergier, I., and Assine, M. L. (Cham, Switzerland: Springer), 23–50.
Ausseil, A.-G. E., Dymond, J. R., and Shepherd, J. D. (2007). Rapid Mapping and Prioritisation of Wetland Sites in the Manawatu-Wanganui Region, New Zealand. Environ. Manage. 39, 316–325. doi:10.1007/s00267-005-0223-1
Aznar, J.-C., and Desrochers, A. (2008). Building for the Future: Abandoned Beaver Ponds Promote Bird Diversity. Écoscience 15, 250–257. doi:10.2980/15-2-3107
Baker, R. G., Schwert, D. P., Bettis, E. A., and Chumbley, C. A. (1993). Impact of Euro-American Settlement on a Riparian Landscape in Northeast Iowa, Midwestem USA: An Integrated Approach Based on Historical Evidence, Floodplain Sediments, Fossil Pollen, Plant Macrofossils and Insects. The Holocene 3, 314–323. doi:10.1177/095968369300300403
Battin, T. J., Kaplan, L. A., Findlay, S., Hopkinson, C. S., Marti, E., Packman, A. I., et al. (2008). Biophysical Controls on Organic Carbon Fluxes in Fluvial Networks. Nat. Geosci. 1, 95–100. doi:10.1038/ngeo101
Beckman, N. D., and Wohl, E. (2014). Carbon Storage in Mountainous Headwater Streams: The Role of Old-Growth Forest and Logjams. Water Resour. Res. 50, 2376–2393. doi:10.1002/2013wr014167
Beechie, T. J., Liermann, M., Pollock, M. M., Baker, S., and Davies, J. (2006). Channel Pattern and River-Floodplain Dynamics in Forested Mountain River Systems. Geomorphology 78 (1-2), 124–141. doi:10.1016/j.geomorph.2006.01.030
Bellmore, J. R., Baxter, C. V., and Connolly, P. J. (2015). Spatial Complexity Reduces Interaction Strengths in the Meta‐Food Web of a River Floodplain Mosaic. Ecology 96 (1), 274–283. doi:10.1890/14-0733.1
Bellmore, J. R., and Baxter, C. V. (2014). Effects of Geomorphic Process Domains on River Ecosystems: A Comparison of Floodplain and Confined Valley Segments. River Res. Appl. 30, 617–630. doi:10.1002/rra.2672
Benda, L., Miller, D., Bigelow, P., and Andras, K. (2003). Effects of Post-Wildfire Erosion on Channel Environments, Boise River, Idaho. For. Ecol. Manag. 178, 105–119. doi:10.1016/s0378-1127(03)00056-2
Beschta, R. L., and Ripple, W. J. (2006). River Channel Dynamics Following Extirpation of Wolves in Northwestern Yellowstone National Park, USA. Earth Surf. Process. Landf. 31, 1525–1539. doi:10.1002/esp.1362
Beschta, R. L., and Ripple, W. J. (2012). The Role of Large Predators in Maintaining Riparian Plant Communities and River Morphology. Geomorphology 157–158, 88–98. doi:10.1016/j.geomorph.2011.04.042
Blumentritt, D. J., Wright, H. E., and Stefanova, V. (2009). Formation and Early History of Lakes Pepin and St. Croix of the Upper Mississippi River. J. Paleolimnol. 41, 545–562. doi:10.1007/s10933-008-9291-6
Bockheim, J. G., and Hartemink, A. E. (2013). Soils with Fragipans in the USA. Catena 104, 233–242. doi:10.1016/j.catena.2012.11.014
Booth, D. B., Ross-Smith, K., Haddon, E. K., Dunne, T., Larsen, E. W., Roche, J. W., et al. (2020). Opportunities and Challenges for Restoration of the Merced River Through Yosemite Valley, Yosemite National Park, USA. River Res. Appl. 36, 1803–1816. doi:10.1016/j.catena.2012.11.014
Booth, E. G., Loheide, S. P., and Hansis, R. D. (2009). Postsettlement Alluvium Removal: A Novel Floodplain Restoration Technique (Wisconsin). Ecol. Restor. 27, 136–139. doi:10.3368/er.27.2.136
Boulton, A. J. (2005). Chances and Challenges in the Conservation of Groundwaters and Their Dependent Ecosystems. Aquat. Conserv: Mar. Freshw. Ecosyst. 15, 319–323. doi:10.1002/aqc.712
Bouwes, N., Weber, N., Jordan, C. E., Saunders, W. C., Tattam, I. A., Volk, C., et al. (2016). Ecosystem experiment Reveals Benefits of Natural and Simulated Beaver Dams to a Threatened Population of Steelhead (Oncorhynchus mykiss). Sci. Rep. 6, 28581. doi:10.1038/srep28581
Bradley, C., and Smith, D. G. (1984). Meandering Channel Response to Altered Flow Regime: Milk River, Alberta and Montana. Water Resour. Res. 20, 1913–1920. doi:10.1029/wr020i012p01913
Brierley, G. J., and Fryirs, K. A. (2016). The Use of Evolutionary Trajectories to Guide 'Moving Targets' in the Management of River Futures. River Res. Appl. 32, 823–835. doi:10.1002/rra.2930
Briggs, M. A., and Hare, D. K. (2018). Explicit Consideration of Preferential Groundwater Discharges as Surface Water Ecosystem Control Points. Hydrol. Process. 2018, 1–6. doi:10.1002/hyp.13178
Bright, W. (2004). Native American Placenames of the United States. Norman, OK: University of Oklahoma Press.
Brookes, A. (1988). Channelized Rivers: Perspectives for Environmental Management. Chichester, United Kingdom: Wiley.
Brown, A. G. (2002). Learning From the Past: Palaeohydrology and Palaeoecology. Freshw. Biol. 47, 817–829. doi:10.1046/j.1365-2427.2002.00907.x
Brown, A. G., Lespez, L., Sear, D. A., Macaire, J.-J., Houben, P., Klimek, K., et al. (2018). Natural vs Anthropogenic Streams in Europe: History, Ecology and Implications for Restoration, River-Rewilding and Riverine Ecosystem Services. Earth Sci. Rev. 180, 185–205. doi:10.1016/j.earscirev.2018.02.001
Brown, L. R., and Moyle, P. B. (1991). Changes in Habitat and Microhabitat Partitioning Within an Assemblage of Stream Fishes in Response to Predation by Sacramento Squawfish (Ptychocheilus grandis). Can. J. Fish. Aquat. Sci. 48 (5), 849–856. doi:10.1139/f91-101
Brummer, C. J., Abbe, T. B., Sampson, J. R., and Montgomery, D. R. (2006). Influence of Vertical Channel Change Associated With Wood Accumulations on Delineating Channel Migration Zones, Washington, USA. Geomorphology 80, 295–309. doi:10.1016/j.geomorph.2006.03.002
Burchsted, D., Daniels, M., Thorson, R., and Vokoun, J. (2010). The River Discontinuum: Applying Beaver Modifications to Baseline Conditions for Restoration of Forested Headwaters. Bioscience 60, 908–922. doi:10.1525/bio.2010.60.11.7
Caskey, S. T., Blaschak, T. S., Wohl, E., Schnackenberg, E., Merritt, D. M., and Dwire, K. A. (2015). Downstream Effects of Stream Flow Diversion on Channel Characteristics and Riparian Vegetation in the Colorado Rocky Mountains, USA. Earth Surf. Process. Landf. 40, 586–598. doi:10.1002/esp.3651
Castro, J. M., and Thorne, C. R. (2019). The Stream Evolution Triangle: Integrating Geology, Hydrology, and Biology. River Res. Applic 35, 315–326. doi:10.1002/rra.3421
Christy, J. A., and Alverson, E. R. (2011). Historical Vegetation of the Willamette Valley, Oregon, Circa 1850. Northwest Sci. 85, 93–107. doi:10.3955/046.085.0202
Cioc, M. (2002). The Rhine: An Eco-Biography, 1815-2000. Seattle, WA: University of Washington Press.
Cluer, B., and Thorne, C. (2014). A Stream Evolution Model Integrating Habitat and Ecosystem Benefits. River Res. Applic. 30, 135–154. doi:10.1002/rra.2631
Coles, B. (2000). “Beaver Territories: the Resource Potential for Humans,” in Human Ecodynamics: Proceedings of the Association for Environmental Archaeology Conference 1998. Editors G. Bailey, R. Charles, and N. Winder (Oxford, UK: Oxbow Books), 80–89.
Coles, J. M., and Orme, B. J. (1983). Home Sapiens or Castor Fiber?. Antiquity 57, 95–102. doi:10.1017/s0003598x00055265
Collins, B. D., Montgomery, D. R., Fetherston, K. L., and Abbe, T. B. (2012). The Floodplain Large-Wood Cycle Hypothesis: A Mechanism for the Physical and Biotic Structuring of Temperate Forested Alluvial Valleys in the North Pacific Coastal Ecoregion. Geomorphology 139–140, 460–470. doi:10.1016/j.geomorph.2011.11.011
Collins, B. D., Montgomery, D. R., and Haas, A. D. (2002). Historical Changes in the Distribution and Functions of Large Wood in Puget Lowland Rivers. Can. J. Fish. Aquat. Sci. 59, 66–76. doi:10.1139/f01-199
Cooper, D. J., Chimner, R. A., and Merritt, D. M. (2012). “Western Mountain Wetlands,” in Wetland Habitats of North America. Editors D. P. Batzer, and A. H Baldwin (Berkeley, CA: University of California Press), 313–328.
Cunningham, J. M., Calhoun, A. J. K., and Glanz, W. E. (2007). Pond-Breeding Amphibian Species Richness and Habitat Selection in a Beaver-Modified Landscape. J. Wildl. Manag. 71, 2517–2526. doi:10.2193/2006-510
Dahl, T. E., and Allord, G. J. (1996). Technical Aspects of Wetlands; History of Wetlands in the Conterminous United States, U.S. Geological Survey Water-Supply Paper 2425. Springfield: US Geological Survey.
Davies, N. S., and Gibling, M. R. (2011). Evolution of Fixed-Channel Alluvial plains in Response to Carboniferous Vegetation. Nat. Geosci. 4, 629–633. doi:10.1038/ngeo1237
Davis, O. K., Minckley, T., Moutoux, T., Jull, T., and Kalin, B. (2002). The Transformation of Sonoran Desert Wetlands Following the Historic Decrease of Burning. J. Arid Environ. 50, 393–412. doi:10.1006/jare.2001.0914
De Becker, P., Hermy, M., and Butaye, J. (1999). Ecohydrological Characterization of a Groundwater-Fed Alluvial Floodplain Mire. Appl. Veg. Sci. 2, 215–228. doi:10.2307/1478985
Del Moral, R. (1999). Predictability of Primary Successional Wetlands on Pumice, Mount St. Helens. Madroño 46, 177–186.
DesRoches, A., Danielescu, S., and Butler, K. (2014). Structural Controls on Groundwater Flow in a Fractured Bedrock Aquifer Underlying an Agricultural Region of Northwestern New Brunswick, Canada. Hydrogeol. J. 22, 1067–1086. doi:10.1007/s10040-014-1134-0
Dickinson, W. R., Klute, M. A., Hayes, M. J., Janecke, S. U., Lundin, E. R., McKittrick, M. A., et al. (1988). Paleogeographic and Paleotectonic Setting of Laramide Sedimentary Basins in the Central Rocky Mountain Region. Geol. Soc. Am. Bull. 100, 1023–1039. doi:10.1130/0016-7606(1988)100<1023:papsol>2.3.co;2
Doughty, M., Sawyer, A. H., Wohl, E., and Singha, K. (2020). Mapping Increases in Hyporheic Exchange from Channel-Spanning Logjams. J. Hydrol. 587, 124931. doi:10.1016/j.jhydrol.2020.124931
Eaton, B. C., Millar, R. G., and Davidson, S. (2010). Channel Patterns: Braided, Anabranching, and Single-Thread. Geomorphology 120 (3–4), 353–364. doi:10.1016/j.geomorph.2010.04.010
Eaton, B. C., and Hassan, M. A. (2013). Scale-dependent Interactions Between Wood and Channel Dynamics: Modeling Jam Formation and Sediment Storage in Gravel-Bed Streams. J. Geophys. Res. Earth Surf. 118, 2500–2508. doi:10.1002/2013jf002917
Edwards, P. M., Pan, Y., Mork, L., and Thorne, C. (2020). Using Diatoms to Assess River Restoration: A Pilot Study in Whychus Creek, Oregon, USA. River Res. Appl. 36, 2089–2095. doi:10.1002/rra.3712
Ehlen, J., and Wohl, E. (2002). Joints and Landform Evolution in Bedrock Canyons. Trans. Japan. Geomorphol. Union 23, 237–255.
Ekka, A., Pande, S., Jiang, Y., and der Zaag, P. v. (2020). Anthropogenic Modifications and River Ecosystem Services: A Landscape Perspective. Water 12, 2706. doi:10.3390/w12102706
Entwistle, N., Heritage, G., and Milan, D. (2019). Ecohydraulic Modelling of Anabranching Rivers. River Res. Applic 35 (4), 353–364. doi:10.1002/rra.3413
Erӧs, T., Kuehne, L., Dolezsai, A., Sommerwerk, N., and Wolter, C. (2019). A Systematic Review of Assessment and Conservation Management in Large Floodplain Rivers–Actions Postponed. Ecol. Indic. 98, 453–461. doi:10.1016/j.ecolind.2018.11.026
Fairfax, E., and Whittle, A. (2020). Smokey the Beaver: Beaver-Dammed Riparian Corridors Stay Green During Wildfire Throughout the Western United States. Ecol. Appl. 30, e02225. doi:10.1002/eap.2225
Faulkner, D. J., Larson, P. H., Jol, H. M., Running, G. L., Loope, H. M., and Goble, R. J. (2016). Autogenic Incision and Terrace Formation Resulting From Abrupt Late-Glacial Base-Level Fall, Lower Chippewa River, Wisconsin, USA. Geomorphology 266, 75–95. doi:10.1016/j.geomorph.2016.04.016
Fetherston, K. L., Naiman, R. J., and Bilby, R. E. (1995). Large Woody Debris, Physical Process, and Riparian Forest Development in Montane River Networks of the Pacific Northwest. Geomorphology 13, 133–144. doi:10.1016/0169-555x(95)00033-2
Fryirs, K. A., Brierley, G. J., Preston, N. J., and Kasai, M. (2007). Buffers, Barriers and Blankets: The (Dis)connectivity of Catchment-Scale Sediment Cascades. Catena 70, 49–67. doi:10.1016/j.catena.2006.07.007
Fustec, J., Lode, T., Le Jacques, D., and Cormier, J. P. (2001). Colonization, Riparian Habitat Selection and Home Range Size in a Reintroduced Population of European Beavers in the Loire. Freshw. Biol. 46, 1361–1371. doi:10.1046/j.1365-2427.2001.00756.x
Gerbersdorf, S. U., Koca, K., de Beer, D., Chennu, A., Noss, C., Risse-Buhl, U., et al. (2020). Exploring Flow-Biofilm-Sediment Interactions: Assessment of Current Status and Future Challenges. Water Res. 185, 116182. doi:10.1016/j.watres.2020.116182
Gibling, M. R., Nanson, G. C., and Maroulis, J. C. (1998). Anastomosing River Sedimentation in the Channel Country of Central Australia. Sedimentology 45, 595–619. doi:10.1046/j.1365-3091.1998.00163.x
Gippel, C. J. (1995). Environmental Hydraulics of Large Woody Debris in Streams and Rivers. J. Environ. Eng. 121, 388–395. doi:10.1061/(asce)0733-9372(1995)121:5(388)
Gooseff, M. N. (2010). Defining Hyporheic Zones - Advancing Our Conceptual and Operational Definitions of Where Stream Water and Groundwater Meet. Geogr. Compass 4, 945–955. doi:10.1111/j.1749-8198.2010.00364.x
Grootjans, A. P., and Verbeek, S. K. (2002). A Conceptual Model of European Wet Meadow Restoration. Ecol. Restor. 20, 6–9. doi:10.3368/er.20.1.6
Grossinger, R. (2012). Napa Valley Historical Ecology Atlas: Exploring a Hidden Landscape of Transformation and Resilience. Berkeley, CA: University of California Press.
Guirard, G., and Brassieur, C. R. (2007). Inherit the Atchafalaya. Lafayette, LA: University of Louisiana Press.
Hall, J. E., Holzer, D. M., and Beechie, T. J. (2007). Predicting River Floodplain and Lateral Channel Migration for Salmon Habitat Conservation. J. Am. Water Resour. Assoc. 43, 786–797. doi:10.1111/j.1752-1688.2007.00063.x
Happ, S. C., Rittenhouse, G., and Dobson, G. C. (1940). Some Principles of Accelerated Stream and valley Sedimentation. Washington, DC: US Department of Agriculture.
Hardie, M. A., Doyle, R. B., Cotching, W. E., and Lisson, S. (2012). Subsurface Lateral Flow in Texture-Contrast (Duplex) Soils and Catchments With Shallow Bedrock. Appl. Environ. Soil Sci. 2012, 861358. doi:10.1155/2012/861358
Harmon, M. E., Franklin, J. F., Swanson, F. J., Sollins, P., Gregory, S. V., Lattin, J. D., et al. (1986). Ecology of Coarse Woody Debris in Temperate Ecosystems. Adv. Ecol. Res. 15, 133–302. doi:10.1016/s0065-2504(08)60121-x
Hartranft, J. L., Merritts, D. J., Walter, R. C., and Rahnis, M. (2011). Big Spring Run Restoration experiment: Policy, Geomorphology, and Aquatic Ecosystems in the Big Spring Run Watershed. Lancaster County, PA: Sustain.
Harvey, J., and Gooseff, M. (2015). River Corridor Science: Hydrologic Exchange and Ecological Consequences From Bedforms to Basins. Water Resour. Res. 51, 6893–6922. doi:10.1002/2015WR017617
Harwood, K., and Brown, A. G. (1993). Fluvial Processes in a Forested Anastomosing River: Flood Partitioning and Changing Flow Patterns. Earth Surf. Process. Landf. 18, 741–748. doi:10.1002/esp.3290180808
Hassan, M. A., Gottesfeld, A. S., Montgomery, D. R., Tunnicliffe, J. F., Clarke, G. K. C., Wynn, G., et al. (2008). Salmon-Driven Bed Load Transport and Bed Morphology in Mountain Streams. Geophys. Res. Lett. 35, L04405. doi:10.1029/2007gl032997
Hauer, F. R., Locke, H., Dreitz, V. J., Hebblewhite, M., Lowe, W. H., Muhlefeld, C. C., et al. (2016). Gravel-bed River Floodplains Are the Ecological Nexus of Glaciated Mountain Landscapes. Sci. Adv. 2, e1600026. doi:10.1126/sciadv.1600026
Heede, B. H., Harvey, M. D., and Laird, J. R. (1988). Sediment Delivery Linkages in a Chaparral Watershed Following a Wildfire. Environ. Manage. 12, 349–358. doi:10.1007/bf01867524
Heffernan, J. B. (2008). Wetlands as an Alternative Stable State in Desert Streams. Ecology 89, 1261–1271. doi:10.1890/07-0915.1
Helton, A. M., Poole, G. C., Payn, R. A., Izurieta, C., and Stanford, J. A. (2014). Relative Influences of the River Channel, Floodplain Surface, and Alluvial Aquifer on Simulated Hydrologic Residence Time in a Montane River Floodplain. Geomorphology 205, 17–26. doi:10.1016/j.geomorph.2012.01.004
Hey, R. D. (1978). Determinate Hydraulic Geometry of River Channels. J. Hydr. Div. 104, 869–885. doi:10.1061/jyceaj.0005010
Hey, R. D. (1979). Dynamic Process-Response Model of River Channel Development. Earth Surf. Process. 4, 59–72. doi:10.1002/esp.3290040106
Hillman, G. R. (1998). Flood Wave Attenuation by a Wetland Following a Beaver Dam Failure on a Second Order Boreal Stream. Wetlands 18, 21–34. doi:10.1007/bf03161439
Hohensinner, S., Egger, G., Muhar, S., Vaudor, L., and Piégay, H. (2021). What Remains Today of Pre‐industrial Alpine Rivers? Census of Historical and Current Channel Patterns in the Alps. River Res. Appl. 37, 128–149. doi:10.1002/rra.3751
Holling, C. S. (1973). Resilience and Stability of Ecological Systems. Annu. Rev. Ecol. Syst. 4, 1–23. doi:10.1146/annurev.es.04.110173.000245
Hood, G. A., and Bayley, S. E. (2008). Beaver (Castor canadensis) Mitigate the Effects of Climate on the Area of Open Water in Boreal Wetlands in Western Canada. Biol. Conserv. 141, 556–567. doi:10.1016/j.biocon.2007.12.003
Hood, G. A., and Larson, D. G. (2014). Beaver-Created Habitat Heterogeneity Influences Aquatic Invertebrate Assemblages in Boreal Canada. Wetlands 34, 19–29. doi:10.1007/s13157-013-0476-z
Jeffres, C. A., Holmes, E. J., Sommer, T. R., and Katz, J. V. E. (2020). Detrital Food Web Drives Aquatic Ecosystem Productivity in a Managed Floodplain. Plos One 15 (9), e0216019. doi:10.1371/journal.pone.0216019
John, S., and Klein, A. (2004). Hydrogeomorphic Effects of Beaver Dams on Floodplain Morphology: Avulsion Processes and Sediment Fluxes in Upland Valley Floors (Spessart, Germany). Quaternaire 15, 219–231. doi:10.3406/quate.2004.1769
Johnson, M. F., Rice, S. P., and Reid, I. (2011). Increase in Coarse Sediment Transport Associated with Disturbance of Gravel River Beds by Signal Crayfish (Pacifastacus leniusculus). Earth Surf. Process. Landf. 36, 1680–1692. doi:10.1002/esp.2192
Johnson, M. F., Thorne, C. R., Castro, J. M., Kondolf, G. M., Mazzacano, C. S., Rood, S. B., et al. (2019). Biomic River Restoration: A New Focus for River Management. River Res. Applic 36, 3–12. doi:10.1002/rra.3529
Johnston, C. A. (2014). Beaver Pond Effects on Carbon Storage in Soils. Geoderma 213, 371–378. doi:10.1016/j.geoderma.2013.08.025
Jones, J. B., and Smock, L. A. (1991). Transport and Retention of Particulate Organic Matter in Two Low-Gradient Headwater Streams. J. North Am. Benthol. Soc. 10, 115–126. doi:10.2307/1467572
Joyce, C. B. (2014). Ecological Consequences and Restoration Potential of Abandoned Wet Grasslands. Ecol. Eng. 66, 91–102. doi:10.1016/j.ecoleng.2013.05.008
Kaatz, M. R. (1955). The Black Swamp: a Study in Historical Geography. Ann. Assoc. Am. Geogr. 45, 1–35. doi:10.1111/j.1467-8306.1955.tb01481.x
Karraker, N. E., and Gibbs, J. P. (2009). Amphibian Production in Forested Landscapes in Relation to Wetland Hydroperiod: A Case Study of Vernal Pools and Beaver Ponds. Biol. Conserv. 142, 2293–2302. doi:10.1016/j.biocon.2009.05.002
Katz, J. V. E., Jeffres, C., Conrad, J. L., Sommer, T. R., Martinez, J., Brumbaugh, S., et al. (2017). Floodplain Farm fields Provide Novel Rearing Habitat for Chinook salmon. PLoS One 12, e0177409. doi:10.1371/journal.pone.0177409
Keddy, P. A., Fraser, L. H., Solomeshch, A. I., Junk, W. J., Campbell, D. R., Arroyo, M. T. K., et al. (2009). Wet and Wonderful: The World’s Largest Wetlands are Conservation Priorities. Bioscience 59, 39–51. doi:10.1525/bio.2009.59.1.8
Kim, J. Y., Yang, D. Y., Nahm, W. H., Yi, S. H., Kim, J. C., Hong, S.-S., et al. (2008). Last Glacial and Holocene Fluvial Wetland Sedimentary Stratigraphy: Comparison Between Soro-ri and Jangheung-ri Archeological Sites, Korea. Quat. Int. 176–177, 135–142. doi:10.1016/j.quaint.2007.05.013
Kingsford, R. T. (2000). Ecological Impacts of Dams, Water Diversions and River Management on Floodplain Wetlands in Australia. Aust. Ecol. 25, 109–127. doi:10.1046/j.1442-9993.2000.01036.x
Kleindl, W. J., Rains, M. C., Marshall, L. A., and Hauer, F. R. (2015). Fire and Flood Expand the Floodplain Shifting Habitat Mosaic Concept. Freshw. Sci. 34, 1366–1382. doi:10.1086/684016
Klimkowska, A., Van Diggelen, R., Bakker, J. P., and Grootjans, A. P. (2007). Wet Meadow Restoration in Western Europe: A Quantitative Assessment of the Effectiveness of Several Techniques. Biol. Conserv. 140, 318–328. doi:10.1016/j.biocon.2007.08.024
Knox, J. C. (1987). Historical Valley Floor Sedimentation in the Upper Mississippi Valley. Ann. Assoc. Am. Geogr. 77, 224–244. doi:10.1111/j.1467-8306.1987.tb00155.x
Koebel, J. W., and Bousquin, S. G. (2014). The Kissimmee River Restoration Project and Evaluation Program, Florida, U.S.A. Restor. Ecol. 22, 345–352. doi:10.1111/rec.12063
Koltzer, N., Scheck-Wenderoth, M., Cacace, M., Frick, M., and Bott, J. (2019). Regional Hydraulic Model of the Upper Rhine Graben. Adv. Geosci. 49, 197–206. doi:10.5194/adgeo-49-197-2019
Kondolf, G. M. (2006). River Restoration and Meanders. Ecol. Soc. 11, 42. doi:10.5751/es-01795-110242
Krause, B., Culmsee, H., Wesche, K., Bergmeier, E., and Leuschner, C. (2011). Habitat Loss of Floodplain Meadows in north Germany since the 1950s. Biodivers. Conserv. 20, 2347–2364. doi:10.1007/s10531-011-9988-0
Larsen, A., May, J.-H., Moss, P., and Hacker, J. (2016). Could Alluvial Knickpoint Retreat rather Than Fire Drive the Loss of Alluvial Wet Monsoon Forest, Tropical Northern Australia?. Earth Surf. Process. Landf. 41, 1583–1594. doi:10.1002/esp.3933
Larsen, L. G., and Harvey, J. W. (2010). How Vegetation and Sediment Transport Feedbacks Drive Landscape Change in the Everglades and Wetlands Worldwide. Am. Nat. 176, E66–E79. doi:10.1086/655215
Larsen, L. G., and Harvey, J. W. (2011). Modeling of Hydroecological Feedbacks Predicts Distinct Classes of Landscape Pattern, Process, and Restoration Potential in Shallow Aquatic Ecosystems. Geomorphology 126, 279–296. doi:10.1016/j.geomorph.2010.03.015
Larsen, L. G. (2019). Multiscale Flow-Vegetation-Sediment Feedbacks in Low-Gradient Landscapes. Geomorphology 334, 165–193. doi:10.1016/j.geomorph.2019.03.009
Laurel, D., and Wohl, E. (2019). The Persistence of Beaver‐Induced Geomorphic Heterogeneity and Organic Carbon Stock in River Corridors. Earth Surf. Process. Landf. 44, 342–353. doi:10.1002/esp.4486
Le Lay, Y.-F., Piégay, H., and Rivière-Honegger, A. (2013). Perception of Braided River Landscapes: Implications for Public Participation and Sustainable Management. J. Environ. Manage. 119, 1–12. doi:10.1016/j.jenvman.2013.01.006
Leopold, L. B., and Wolman, M. G. (1957). River Flood plains: Some Observations on Their Formation. Washington, DC: U.S. Geological Survey.
Lewis, W. M. (2001). Wetlands Explained: Wetland Science, Policy, and Politics in America. New York: Oxford University Press.
Liarsou, A. (2013). Interactions Between the Beaver (Castor fiber L.) and Human Societies: A Long-Term Archaeological and Historical Approach. Archaeol. Rev. Camb. 28, 171–185.
Lindeman, R. L. (1942). The Trophic-Dynamic Aspect of Ecology. Ecology 23 (4), 399–417. doi:10.2307/1930126
Lininger, K. B., Wohl, E., and Rose, J. R. (2018). Geomorphic Controls on Floodplain Soil Organic Carbon in the Yukon Flats, Interior Alaska, From Reach to River basin Scales. Water Resour. Res. 54, 1934–1951. doi:10.1002/2017wr022042
Livers, B., Wohl, E., Jackson, K. J., and Sutfin, N. A. (2018). Historical Land Use as a Driver of Alternative States for Stream Form and Function in Forested Mountain Watersheds of the Southern Rocky Mountains. Earth Surf. Process. Landf. 43, 669–684. doi:10.1002/esp.4275
Loheide, S. P., Deitchman, R. S., Cooper, D. J., Wolf, E. C., Hammersmark, C. T., and Lundquist, J. D. (2009). A Framework for Understanding the Hydroecology of Impacted Wet Meadows in the Sierra Nevada and Cascade Ranges, California, USA. Hydrogeol. J. 17, 229–246. doi:10.1007/s10040-008-0380-4
Loheide, S. P., and Gorelick, S. M. (2006). Quantifying Stream−Aquifer Interactions Through the Analysis of Remotely Sensed Thermographic Profiles and In Situ Temperature Histories. Environ. Sci. Technol. 40, 3336–3341. doi:10.1021/es0522074
Loheide, S. P., and Gorelick, S. M. (2007). Riparian Hydroecology: A Coupled Model of the Observed Interactions Between Groundwater Flow and Meadow Vegetation Patterning. Water Resour. Res. 43, W07414. doi:10.1029/2006wr005233
MacGregor, K. R., Anderson, R. S., Anderson, S. P., and Waddington, E. D. (2000). Numerical Simulations of Glacial-Valley Longitudinal Profile Evolution. Geology 28, 1031–1034. doi:10.1130/0091-7613(2000)028<1031:nsogvl>2.3.co;2
Mactaggart, B., Bauer, J., Goldney, D., and Rawson, A. (2008). Problems in Naming and Defining the Swampy Meadow-An Australian Perspective. J. Environ. Manage. 87, 461–473. doi:10.1016/j.jenvman.2007.01.030
Mahoney, J. M., and Rood, S. B. (1991). A Device for Studying the Influence of Declining Water Table on Poplar Growth and Survival. Tree Physiol. 8, 305–314. doi:10.1093/treephys/8.3.305
Major, J. J., Zheng, S., Mosbrucker, A. R., Spicer, K. R., Christianson, T., and Thorne, C. R. (2019). Multidecadal Geomorphic Evolution of a Profoundly Disturbed Gravel Bed River System-A Complex, Nonlinear Response and Its Impact on Sediment Delivery. J. Geophys. Res. Earth Surf. 124, 1281–1309. doi:10.1029/2018jf004843
Martin, P. S. (1958). Taiga-Tundra and the Full-Glacial Period in Chester County, Pennsylvania. Am. J. Sci. 256, 470–502. doi:10.2475/ajs.256.7.470
Martín-Vide, J. P., Amarilla, M., and Zárate, F. J. (2014). Collapse of the Pilcomayo River. Geomorphology 205, 155–163. doi:10.1016/j.geomorph.2012.12.007
McCarthy, T. S., and Ellery, W. N. (1998). The Okavango Delta. Trans. R. Soc. South Africa 53, 157–182. doi:10.1080/00359199809520384
Medel, I. D., Stubblefield, A. P., and Shea, C. (2020). Sedimentation and Erosion Patterns within Anabranching Channels in a Lowland River Restoration Project. Int. J. River Basin Manage. 18 1–11. doi:10.1080/15715124.2020.1809435
Melly, B. L., Schael, D. M., and Gama, P. T. (2017). Perched Wetlands: an Explanation to Wetland Formation in Semi-Arid Areas. J. Arid Environ. 141, 34–39. doi:10.1016/j.jaridenv.2017.02.004
Merritts, D., Walter, R., Rahnis, M., Cox, S., Hartranft, J., Scheid, C., et al. (2013). “The Rise and Fall of Mid-Atlantic Streams: Millpond Sedimentation, Milldam Breaching, Channel Incision, and Stream Bank Erosion,” in The Challenges of Dam Removal and River Restoration. Editors J. V. DeGraff, and J. E. Evans. 21st Edn. Boulder, CO: Geological Society of America Reviews in Engineering Geology, 183–203.
Merritts, D., Walter, R., Rahnis, M., Hartranft, J., Cox, S., Gellis, A., et al. (2011). Anthropocene Streams and Base-Level Controls From Historic Dams in the Unglaciated Mid-Atlantic Region, USA. Phil. Trans. R. Soc. A. 369 (1938), 976–1009. doi:10.1098/rsta.2010.0335
Mertes, L. A. K. (1997). Documentation and Significance of the Perirheic Zone on Inundated Floodplains. Water Resour. Res. 33, 1749–1762. doi:10.1029/97wr00658
Micheli, E. R., and Kirchner, J. W. (2002a). Effects of Wet Meadow Riparian Vegetation on Streambank Erosion. 1. Remote Sensing Measurements of Streambank Migration and Erodibility. Earth Surf. Process. Landf. 27 (6), 627–639. doi:10.1002/esp.338
Micheli, E. R., and Kirchner, J. W. (2002b). Effects of Wet Meadow Riparian Vegetation on Streambank Erosion. 2. Measurements of Vegetated Bank Strength and Consequences for Failure Mechanics. Earth Surf. Process. Landf. 27 (7), 687–697. doi:10.1002/esp.340
Miller, J. R., Lord, M. L., Villarroel, L. F., Germanoski, D., and Chambers, J. C. (2012). Structural Organization of Process Zones in Upland Watersheds of Central Nevada and its Influence on Basin Connectivity, Dynamics, and Wet Meadow Complexes. Geomorphology 139–140, 384–402. doi:10.1016/j.geomorph.2011.11.004
Milner, N., Lane, P., Taylor, B., Conneller, C., and Schadla-Hall, T. (2011). Star Carr in a Postglacial Lakescape: 60 Years of Research. J. Wetl. Archaeol. 11, 1–19. doi:10.1179/jwa.2011.11.1.1
Milzow, C., Kgotlhang, L., Bauer-Gottwein, P., Meier, P., and Kinzelbach, W. (2009). Regional Review: The Hydrology of the Okavango Delta, Botswana-Processes, Data and Modelling. Hydrogeol. J. 17, 1297–1328. doi:10.1007/s10040-009-0436-0
Mitsch, W. J., and Day, J. W. (2006). Restoration of Wetlands in the Mississippi-Ohio-Missouri (MOM) River Basin: Experience and Needed Research. Ecol. Eng. 26, 55–69. doi:10.1016/j.ecoleng.2005.09.005
M., M., Simo, J., and K., B. (2001). Correlation of Hydraulic Conductivity with Stratigraphy in a Fractured-Dolomite Aquifer, Northeastern Wisconsin, USA. Hydrogeol. J. 9, 570–583. doi:10.1007/s10040-001-0165-5
Montgomery, D. R., and Buffington, J. M. (1997). Channel-reach Morphology in Mountain Drainage Basins. Geol. Soc. Am. Bull. 109, 596–611. doi:10.1130/0016-7606(1997)109<0596:crmimd>2.3.co;2
Mulholland, P. J. (1981). Organic Carbon Flow in a Swamp‐Stream Ecosystem. Ecol. Monogr. 51, 307–322. doi:10.2307/2937276
Muni, T. M., and Westbrook, C. J. (2021). Beaver Dam Analogue Configurations Influence Stream and Riparian Water Table Dynamics of a Degraded spring-fed Creek in the Canadian Rockies. River Res. Appl. 37, 1–13. doi:10.1002/rra.3753
Mwanukuzi, P. P. K. (1993). “Origin and Geomorphology of the Wetlands of Tanzania,” in Proceedings of a Seminar on the Wetlands of Tanzania. Editors G. L. Kamukala, and S. A. Crafter (Gland, Switzerland: IUCN), 27–36.
Nadler, C. T., and Schumm, S. A. (1981). Metamorphosis of South Platte and Arkansas Rivers, Eastern Colorado. Phys. Geogr. 2, 95–115. doi:10.1080/02723646.1981.10642207
Nelson, P., White, R., and Molina, R. (2006). The Pacific Northwest Research Station’s Biodiversity Initiative: Collaborating for Biodiversity Management. Gen. Tech. Rep. PNW-GTR-670. Portland, OR: U.S. Department of Agriculture, Forest Service, Pacific Northwest Research Station Available at: https://www.fs.fed.us/pnw/pubs/pnw_gtr670.pdf.
Naiman, R. J., Melillo, J. M., and Hobbie, J. E. (1986). Ecosystem Alteation of Boreal Forest Streams by Beaver (Castor Canadensis). Ecology 67, 1254–1269. doi:10.2307/1938681
Nilsson, C., Riis, T., Sarneel, J. M., and Svavarsdóttir, K. (2018). Ecological Restoration as a Means of Managing Inland Flood Hazards. Bioscience 68, 89–99. doi:10.1093/biosci/bix148
Nolet, B. A., and Rosell, F. (1998). Comeback of the Beaver Castor fiber: an Overview of Old and New Conservation Problems. Biol. Conserv. 83, 165–173. doi:10.1016/s0006-3207(97)00066-9
Pavlowsky, R. T., Lecce, S. A., Owen, M. R., and Martin, D. J. (2017). Legacy Sediment, Lead, and Zinc Storage in Channel and Floodplain Deposits of the Big River, Old Lead Belt Mining District, Missouri, USA. Geomorphology 299, 54–75. doi:10.1016/j.geomorph.2017.08.042
Peipoch, M., Brauns, M., Hauer, F. R., Weitere, M., and Valett, H. M. (2015). Ecological Simplification: Human Influences on Riverscape Complexity. BioScience 65, 1057–1065. doi:10.1093/biosci/biv120
Perera, E. A. (2020). Surface Hydrology Characteristics of Inland Patchy Wetlands in Southern and Western Iceland. MS thesis. York (United Kingdom): York University.
Petts, G. E., and Amoros, C. (1996). “The Fluvial Hydrosystem,” in Fluvial Hydrosystems. Editors G. E. Petts, and C. Amoros (London, United Kingdom: Chapman and Hall), 1–12.
Pisut, P. (2002). Channel Evolution of the Pre-Channelized Danube River in Bratislava, Slovakia (1712-1886). Earth Surf. Process. Landf. 27, 369–390. doi:10.1007/978-94-009-1491-9_1
Poff, N. L., Olden, J. D., Merritt, D. M., and Pepin, D. M. (2007). Homogenization of Regional River Dynamics by Dams and Global Biodiversity Implications. Proc. Natl. Acad. Sci. 104, 5732–5737. doi:10.1073/pnas.0609812104
Pollock, M. M., Beechie, T. J., Wheaton, J. M., Jordan, C. E., Bouwes, N., Weber, N., et al. (2014). Using Beaver Dams to Restore Incised Stream Ecosystems. Bioscience 64, 279–290. doi:10.1093/biosci/biu036
M. M. Pollock, G. M. Lewallen, K. Woodruff, C. E. Jordan, and J. M. Castro (2017). The Beaver Restoration Guidebook: Working With Beaver to Restore Streams, Wetlands, and Floodplains, v. 2.0. Portland, OR: US Fish and Wildlife Service.
Polvi, L. E., and Wohl, E. (2012). The beaver Meadow Complex Revisited - the Role of Beavers in post-glacial Floodplain Development. Earth Surf. Process. Landf. 37, 332–346. doi:10.1002/esp.2261
Polvi, L. E., and Wohl, E. (2013). Biotic Drivers of Stream Planform. Bioscience 63, 439–452. doi:10.1525/bio.2013.63.6.6
Popescu, V. D., and Gibbs, J. P. (2009). Interactions Between Climate, beaver Activity, and Pond Occupancy by the Cold-Adapted Mink Frog in New York State, USA. Biol. Conserv. 142, 2059–2068. doi:10.1016/j.biocon.2009.04.001
Powers, P. D., Helstab, M., and Niezgoda, S. L. (2019). A Process‐based Approach to Restoring Depositional River Valleys to Stage 0, an Anastomosing Channel Network. River Res. Appl. 35, 3–13. doi:10.1002/rra.3378
Prudic, D. E., Niswonger, R. G., Harrill, J. R., and Wood, J. L. (2007). “Streambed Infiltration and Ground-Water Flow From the Trout Creek Drainage, an Intermittent Tributary to the Humboldt River, North-Central Nevada,” in Chapter K in Ground-Water Recharge in the Arid and Semiarid Southwestern United States. Editors D. A. Stonestrom, J. Constantz, T. P. Ferre, and S. A. Leake (Tucson, AZ: U.S. Geological Survey). doi:10.3133/pp1703K
Puttock, A. K., Cunliffe, A. M., Anderson, K., and Brazier, R. E. (2015). Aerial Photography Collected With a Multirotor Drone Reveals Impact of Eurasian Beaver Reintroduction on Ecosystem Structure. J. Unmanned Veh. Sys. 3, 123–130. doi:10.1139/juvs-2015-0005
Racchetti, E., Bartoli, M., Soana, E., Longhi, D., Christian, R. R., Pinardi, M., et al. (2010). Influence of Hydrological Connectivity of Riverine Wetlands on Nitrogen Removal via Denitrification. Biogeochemistry 103, 335–354. doi:10.1007/s10533-010-9477-7
Rahimi, H., Pazira, E., and Tajik, F. (2000). Effect of Soil Organic Matter, Electrical Conductivity and Sodium Adsorption Ratio on Tensile Strength of Aggregates. Soil Tillage Res. 54, 145–153. doi:10.1016/s0167-1987(00)00086-6
Rathburn, S. L., Rubin, Z. K., and Wohl, E. E. (2013). Evaluating Channel Response to an Extreme Sedimentation Event in the Context of Historical Range of Variability: Upper Colorado River, USA. Earth Surf. Process. Landf. 38, 391–406. doi:10.1002/esp.3329
Reddy, K. R., Kadlec, R. H., Flaig, E., and Gale, P. M. (1999). Phosphorus Retention in Streams and Wetlands: A Review. Crit. Rev. Environ. Sci. Technol. 29, 83–146. doi:10.1080/10643389991259182
Richardson, W. B., Strauss, E. A., Bartsch, L. A., Monroe, E. M., Cavanaugh, J. C., Vingum, L., et al. (2004). Denitrification in the Upper Mississippi River: Rates, Controls, and Contribution to Nitrate Flux. Can. J. Fish. Aquat. Sci. 61, 1102–1112. doi:10.1139/f04-062
Riggins, J. J., Davis, C. A., and Hoback, W. W. (2009). Biodiversity of Belowground Invertebrates as an Indicator of Wet Meadow Restoration success (Platte River, Nebraska). Restor. Ecol. 17, 495–505. doi:10.1111/j.1526-100x.2008.00394.x
Robertson, A. I., Bunn, S. E., Boon, P. I., and Walker, K. (1999). Sources, Sinks and Transformation of Organic Carbon in Australian Floodplain Rivers. Mar. Freshw. Res. 50, 813–829. doi:10.1071/mf98039
Rosell, F., Bozser, O., Collen, P., and Parker, H. (2005). Ecological Impact of Beavers Castor fiber and Castor canadensis and Their Ability to Modify Ecosystems. Mammal Rev. 35, 248–276. doi:10.1111/j.1365-2907.2005.00067.x
Salama, R. B. (1987). The Evolution of the River Nile. The Buried saline Rift Lakes in Sudan-I. Bahr El Arab Rift, the Sudd Buried saline lake. J. Afr. Earth Sci. (1983) 6, 899–913. doi:10.1016/0899-5362(87)90049-2
Sawyer, A. H., and Cardenas, M. B. (2012). Effect of Experimental Wood Addition on Hyporheic Exchange and thermal Dynamics in a Losing Meadow Stream. Water Resour. Res. 48, W10537. doi:10.1029/2011wr011776
Sayer, C. D. (2014). Conservation of Aquatic Landscapes: Ponds, Lakes, and Rivers as Integrated Systems. WIREs Water 1, 573–585. doi:10.1002/wat2.1045
Scheffel, H. (2018). The Hydrology of a Sandur-Wetland in a Volcanic Environment, Southeast Iceland. MS thesis. York (United Kingdom): York University.
Schneider, N., and Eugster, W. (2007). Climatic Impacts of Historical Wetland Drainage in Switzerland. Clim. Change 80, 301–321. doi:10.1007/s10584-006-9120-8
Schrautzer, J., Asshoff, M., and Müller, F. (1996). Restoration Strategies for Wet Grasslands in Northern Germany. Ecol. Eng. 7, 255–278. doi:10.1016/s0925-8574(96)00021-3
Schrott, L., Hufschmidt, G., Hankammer, M., Hoffmann, T., and Dikau, R. (2003). Spatial Distribution of Sediment Storage Types and Quantification of valley Fill Deposits in an Alpine Basin, Reintal, Bavarian Alps, Germany. Geomorphology 55, 45–63. doi:10.1016/s0169-555x(03)00131-4
Schumm, S. A. (1985). Patterns of Alluvial Rivers. Annu. Rev. Earth Planet. Sci. 13, 5–27. doi:10.1146/annurev.ea.13.050185.000253
Sedell, J. R., and Froggatt, J. L. (1984). Importance of Streamside Forests to Large Rivers: The Isolation of the Willamette River, Oregon, U. S. A., From its Floodplain by Snagging and Streamside Forest Removal. SIL Proc. 1922-2010 22, 1828–1834. doi:10.1080/03680770.1983.11897581
Shakesby, R., and Doerr, S. (2006). Wildfire as a Hydrological and Geomorphological Agent. Earth Sci. Rev. 74, 269–307. doi:10.1016/j.earscirev.2005.10.006
Shi, L., Wang, Y., Jia, Y., Lu, C., Lei, G., and Wen, L. (2017). Vegetation Cover Dynamics and Resilience to Climatic and Hydrological Disturbances in Seasonal Floodplain: the Effects of Hydrological Connectivity. Front. Plant Sci. 8, 2196. doi:10.3389/fpls.2017.02196
Shields, F. D., and Nunnally, N. R. (1984). Environmental Aspects of Clearing and Snagging. J. Environ. Eng. 110, 152–165. doi:10.1061/(asce)0733-9372(1984)110:1(152)
Silverman, N. L., Allred, B. W., Donnelly, J. P., Chapman, T. B., Maestas, J. D., Wheaton, J. M., et al. (2019). Low-Tech Riparian and Wet Meadow Restoration Increases Vegetation Productivity and Resilience Across Semiarid Rangelands. Restor. Ecol. 27, 269–278. doi:10.1111/rec.12869
Slocombe, M. L., and Davis, J. D. (2014). Morphology of Small, Discontinuous Montane Meadow Streams in the Sierra Nevada. Geomorphology 219, 103–113. doi:10.1016/j.geomorph.2014.04.040
Smith, J. M., and Mather, M. E. (2013). Beaver Dams Maintain Fish Biodiversity by Increasing Habitat Heterogeneity Throughout a Low-Gradient Stream Network. Freshw. Biol. 58, 1523–1538. doi:10.1111/fwb.12153
Stanford, J. A., Lorang, M. S., and Hauer, F. R. (2005). The Shifting Habitat Mosaic of River Ecosystems. SIL Proc. 1922-2010 29, 123–136. doi:10.1080/03680770.2005.11901979
Stanford, J. A., and Ward, J. V. (1993). An Ecosystem Perspective of Alluvial Rivers: Connectivity and the Hyporheic Corridor. J. North Am. Benthol. Soc. 12, 48–60. doi:10.2307/1467685
Stevens, C. E., Paszkowski, C. A., and Scrimgeour, G. J. (2006). Older is Better: Beaver Ponds on Boreal Streams as Breeding Habitat for the Wood Frog. J. Wildl. Manage. 70, 1360–1371. doi:10.2193/0022-541x(2006)70[1360:oibbpo]2.0.co;2
Sutfin, N. A., and Wohl, E. (2019). Elevational Differences in Hydrogeomorphic Disturbance Regime Influence Sediment Residence Times within Mountain River Corridors. Nat. Commun. 10, 2221. doi:10.1038/s41467-019-09864-w
Sutfin, N. A., Wohl, E. E., and Dwire, K. A. (2016). Banking Carbon: A Review of Organic Carbon Storage and Physical Factors Influencing Retention in Floodplains and Riparian Ecosystems. Earth Surf. Process. Landf. 41, 38–60. doi:10.1002/esp.3857
Swanger, K. M., Marchant, D. R., Kowalewski, D. E., and Head, J. W. (2010). Viscous Flow Lobes in central Taylor Valley, Antarctica: Origin as Remnant Buried Glacial Ice. Geomorphology 120, 174–185. doi:10.1016/j.geomorph.2010.03.024
Tal, M., and Paola, C. (2010). Effects of Vegetation on Channel Morphodynamics: Results and Insights From Laboratory Experiments. Earth Surf. Process. Landf. 35, 1014–1028. doi:10.1002/esp.1908
Tank, J. L., Rosi-Marshall, E. J., Griffiths, N. A., Entrekin, S. A., and Stephen, M. L. (2010). A Review of Allochthonous Organic Matter Dynamics and Metabolism in Streams. J. North Am. Benthol. Soc. 29, 118–146. doi:10.1899/08-170.1
Thompson, R. M., and Townsend, C. R. (2005). Energy Availability, Spatial Heterogeneity and Ecosystem Size Predict Food-Web Structure in Streams. Oikos 108, 137–148. doi:10.1111/j.0030-1299.2005.11600.x
Thorp, J. H., Delong, M. D., Greenwood, K. S., and Casper, A. F. (1998). Isotopic Analysis of Three Food Web Theories in Constricted and Floodplain Regions of a Large River. Oecologia 117, 551–563. doi:10.1007/s004420050692
Thorp, J. H., Flotemersch, J. E., Delong, M. D., Casper, A. F., Thoms, M. C., Ballantyne, F., et al. (2010). Linking Ecosystem Services, Rehabilitation, and River Hydrogeomorphology. Bioscience 60, 67–74. doi:10.1525/bio.2010.60.1.11
Tooth, S., McCarthy, T. S., Brandt, D., Hancox, P. J., and Morris, R. (2002). Geological Controls on the Formation of Alluvial Meanders and Floodplain Wetlands: the Example of the Klip River, Eastern Free State, South Africa. Earth Surf. Process. Landf. 27, 797–815. doi:10.1002/esp.353
Tooth, S., and McCarthy, T. S. (2007). Wetlands in Drylands: Geomorphological and Sedimentological Characteristics, With Emphasis on Examples From Southern Africa. Prog. Phys. Geogr. Earth Environ. 31, 3–41. doi:10.1177/0309133307073879
Trimble, S. W. (2009). Fluvial Processes, Morphology and Sediment Budgets in the Coon Creek Basin, WI, USA, 1975-1993. Geomorphology 108, 8–23. doi:10.1016/j.geomorph.2006.11.015
Trimble, S. W., and Mendel, A. C. (1995). The Cow as a Geomorphic Agent - A Critical Review. Geomorphology 13, 233–253. doi:10.1016/0169-555x(95)00028-4
Triska, F. J. (1984). Role of Wood Debris in Modifying Channel Geomorphology and Riparian Areas of a Large Lowland River under Pristine Conditions: A Historical Case Study. SIL Proc. 1922-2010 22, 1876–1892. doi:10.1080/03680770.1983.11897589
Walter, R. C., and Merritts, D. J. (2008). Natural Streams and the Legacy of Water-Powered Mills. Science 319, 299–304. doi:10.1126/science.1151716
Wang, X., Shaw, E. L., Westbrook, C. J., and Bedard-Haughn, A. (2018). Beaver Dams Induce Hyporheic and Biogeochemical Changes in Riparian Areas in a Mountain Peatland. Wetlands 38, 1017–1032. doi:10.1007/s13157-018-1059-9
Ward, J. V. (1989). The Four-Dimensional Nature of Lotic Ecosystems. J. North Am. Benthol. Soc. 8, 2–8. doi:10.2307/1467397
Weber, N., Bouwes, N., Pollock, M. M., Volk, C., Wheaton, J. M., Wathen, G., et al. (2017). Alteration of Stream Temperature by Natural and Artificial beaver Dams. PLoS One 12 (5), e0176313. doi:10.1371/journal.pone.0176313
Wegener, P., Covino, T., and Wohl, E. (2017). Beaver‐Mediated Lateral Hydrologic Connectivity, Fluvial Carbon and Nutrient Flux, and Aquatic Ecosystem Metabolism. Water Resour. Res. 53, 4606–4623. doi:10.1002/2016wr019790
Westbrook, C. J., Cooper, D. J., and Baker, B. W. (2006). Beaver Dams and Overbank Floods Influence Groundwater-Surface Water Interactions of a Rocky Mountain Riparian Area. Water Resour. Res. 42, W06404. doi:10.1029/2005wr004560
Williams, G. P. (1978). The Case of the Shrinking Channels – the North Platte and the Platte Rivers in Nebraska. Arlington, VA: U.S. Geological Survey Circular.
Williamson, T. (2006). Dutch Engineers and the Draining of the Fens in Eastern England. Geografische Stud. 338, 103–119.
Wilson, K. N., Baker, S. L., and Kondolf, G. M. (2020). The Ideal Meander: Exploring Freshwater Scientist Drawings of River Restoration. Freshw. Sci. 39, 349–355. doi:10.1086/709012
Woelfle‐Erskine, C., Wilcox, A. C., and Moore, J. N. (2012). Combining Historical and Process Perspectives to Infer Ranges of Geomorphic Variability and Inform River Restoration in a Wandering Gravel‐Bed River. Earth Surf. Process. Landf. 37 (12), 1302–1312. doi:10.1002/esp.3276
Wohl, E., Dwire, K., Sutfin, N. A., Polvi, L., and Bazan, R. (2012). Mechanisms of Carbon Storage in Mountainous Headwater Rivers. Nat. Commun. 3, 1263. doi:10.1038/ncomms2274
Wohl, E. (2014). A Legacy of Absence. Prog. Phys. Geogr. Earth Environ. 38, 637–663. doi:10.1177/0309133314548091
Wohl, E. (2017). Connectivity in Rivers. Prog. Phys. Geogr. Earth Environ. 41, 345–362. doi:10.1177/0309133317714972
Wohl, E., Hall, R. O., Lininger, K. B., Sutfin, N. A., and Walters, D. M. (2017a). Carbon Dynamics of River Corridors and the Effects of Human Alterations. Ecol. Monogr. 87, 379–409. doi:10.1002/ecm.1261
Wohl, E. (2015). Legacy Effects on Sediments in River Corridors. Earth-Science Rev. 147, 30–53. doi:10.1016/j.earscirev.2015.05.001
Wohl, E., Lininger, K. B., Fox, M., Baillie, B. R., and Erskine, W. D. (2017b). Instream Large Wood Loads Across Bioclimatic Regions. Forest Ecol. Manag. 404, 370–380. doi:10.1016/j.foreco.2017.09.013
Wohl, E., Lininger, K. B., and Scott, D. N. (2018). River Beads as a Conceptual Framework for Building Carbon Storage and Resilience to Extreme Climate Events Into River Management. Biogeochemistry 141, 365–383. doi:10.1007/s10533-017-0397-7
Wohl, E. (2016). Spatial Heterogeneity as a Component of River Geomorphic Complexity. Prog. Phys. Geogr. Earth Environ. 40, 598–615. doi:10.1177/0309133316658615
Wolf, E. C., Cooper, D. J., and Hobbs, N. T. (2007). Hydrologic Regime and Herbivory Stabilize an Alternative State in Yellowstone National Park. Ecol. Appl. 17, 1572–1587. doi:10.1890/06-2042.1
Wright, J. P., Jones, C. G., and Flecker, A. S. (2002). An Ecosystem Engineer, the beaver, Increases Species Richness at the Landscape Scale. Oecologia 132, 96–101. doi:10.1007/s00442-002-0929-1
Keywords: connectivity, floodplain, hyporheic, kaleidoscope rivers, perirheic, river restoration, river-wetland corridor, wetland
Citation: Wohl E, Castro J, Cluer B, Merritts D, Powers P, Staab B and Thorne C (2021) Rediscovering, Reevaluating, and Restoring Lost River-Wetland Corridors. Front. Earth Sci. 9:653623. doi: 10.3389/feart.2021.653623
Received: 14 January 2021; Accepted: 11 June 2021;
Published: 30 June 2021.
Edited by:
Fernando Nardi, University for Foreigners Perugia, ItalyReviewed by:
Jeremie Riquier, Université Jean Monnet, FranceBruce Rhoads, University of Illinois at Urbana-Champaign, United States
Copyright © 2021 Wohl, Castro, Cluer, Merritts, Powers, Staab and Thorne. This is an open-access article distributed under the terms of the Creative Commons Attribution License (CC BY). The use, distribution or reproduction in other forums is permitted, provided the original author(s) and the copyright owner(s) are credited and that the original publication in this journal is cited, in accordance with accepted academic practice. No use, distribution or reproduction is permitted which does not comply with these terms.
*Correspondence: Colin Thorne, Y3Rob3JuZUB3b2xmd2F0ZXJyZXNvdXJjZXMuY29t
†ORCID: Janine Castro, orcid.org/0000-0002-1951-7507; Colin Thorne, orcid.org/0000-0002-2450-9624