- 1Department of Earth and Space Sciences, University of Washington, Seattle, WA, United States
- 2Department of Marine, Earth, & Atmospheric Sciences, North Carolina State University, Raleigh, NC, United States
The Colorado Plateau’s complex landscape has motivated over a century of debate, key to which is understanding the timing and processes of surface uplift of the greater Colorado Plateau region, and its interactions with erosion, drainage reorganization, and landscape evolution. Here, we evaluate what is known about the surface uplift history from prior paleoelevation estimates from the region by synthesizing and evaluating estimates 1) in context inferred from geologic, geomorphic, and thermochronologic constraints, and 2) in light of recent isotopic and paleobotanical proxy method advancements. Altogether, existing data and estimates suggest that half-modern surface elevations were attained by the end of the Laramide orogeny (∼40 Ma), and near-modern surface elevations by the mid-Miocene (∼16 Ma). However, our analysis of paleoelevation proxy methods highlights the need to improve proxy estimates from carbonate and floral archives including the ∼6–16 Ma Bidahochi and ∼34 Ma Florissant Formations and explore understudied (with respect to paleoelevation) Laramide basin deposits to fill knowledge gaps. We argue that there are opportunities to leverage recent advancements in temperature-based paleoaltimetry to refine the surface uplift history; for instance, via systematic comparison of clumped isotope and paleobotanical thermometry methods applied to lacustrine carbonates that span the region in both space and time, and by use of paleoclimate model mediated lapse rates in paleoelevation reconstruction.
Introduction
Scientists have long debated the uplift of the Colorado Plateau region (CP), including the modern Colorado River (CR) drainage from the Rocky Mountains to where it exits the modern plateau southwest of Grand Canyon (GC; Figure 1). This region was a tectonically stable sedimentary basin near sea-level until the Laramide orogeny initiated in the Late Cretaceous (Hunt, 1956; Nations et al., 1991) and now resides ∼2–3 km above sea level. Proposed mechanisms for plateau rise make testable predictions of uplift patterns due to processes including: lower-crustal flow (McQuarrie and Chase, 2000) or shear-removal of lithospheric mantle by the Farallon slab during the Laramide (Hernández-Uribe and Palin, 2019); lithospheric foundering (Bird, 1979) or hydration (Porter et al., 2017) in the mid-Cenozoic; and mantle upwelling (e.g., Moucha et al., 2009) or isostatic response to denudation (e.g., Lazear et al., 2013) in the late Cenozoic. Accurate paleoelevation constraints can evaluate hypothesized uplift mechanisms and provide context for CR evolution.
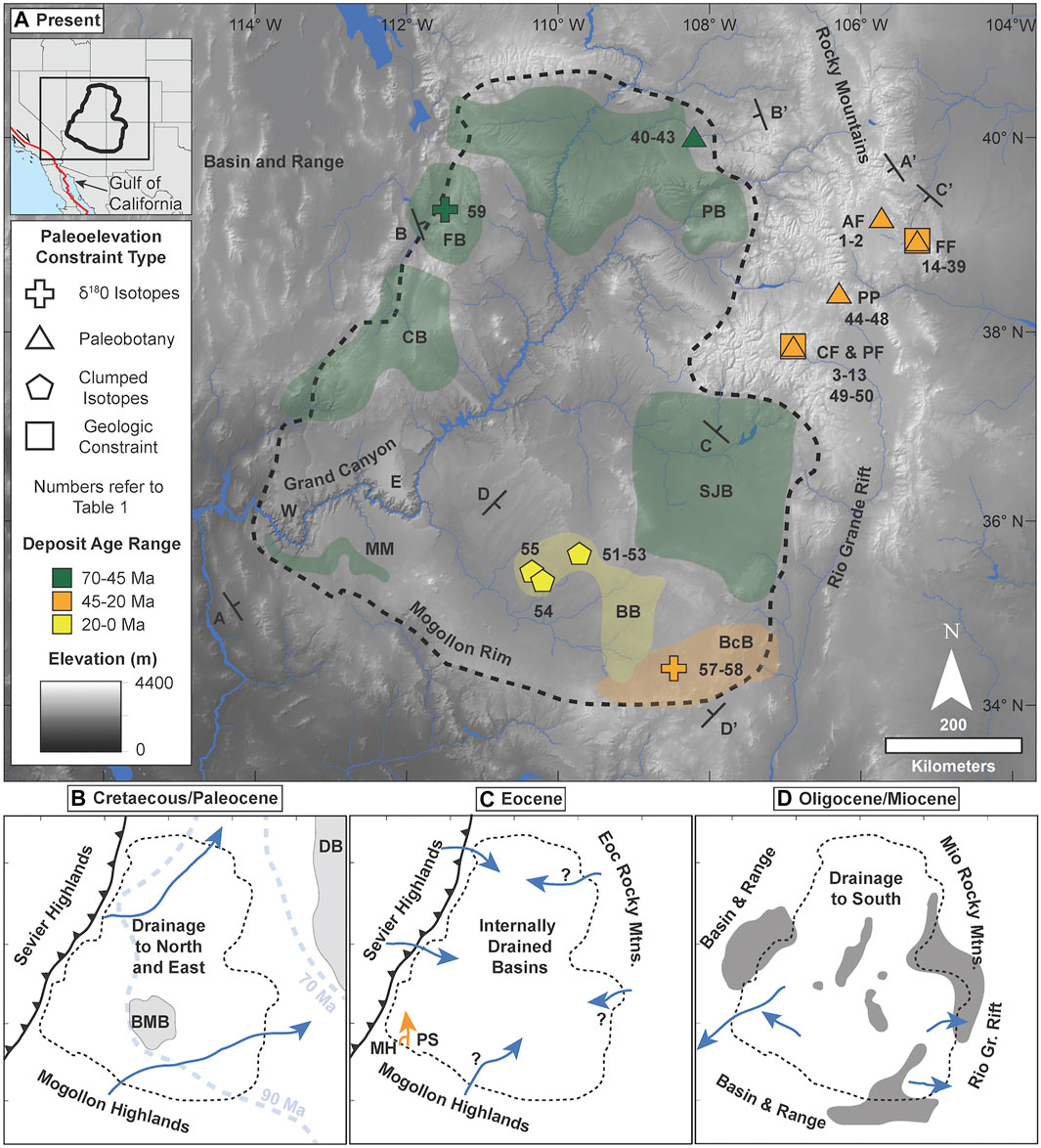
FIGURE 1. (A) Modern elevation of the Colorado Plateau region with relevant geographic features and depositional basins, locations of paleoaltimetry estimates, and transects corresponding to Figure 2. Symbols represent the paleoaltimetry method applied at each location, and superscripts refer to paleo-elevation estimate ID in Supplementary Table S1. SJB = San Juan Basin; BcB = Baca Basin; BB = Bidahochi Basin; MM = Music Mountain Formation; CB = Claron Basin; FB = Flagstaff Basin; UB = Uinta Basin; PB = Piceance Basin; CF = Creede Formation; AF = Antero Formation; FF = Florissant Formation; PP = Pitch-Pinnacle Formation; PF = Platoro Formation. (B–D) Relevant paleogeographic reconstructions to provide context for interpreting uplift of the Colorado Plateau region (see Overview section for discussion). (B) In the Late Cretaceous-Paleocene, evidence of the Laramide orogeny and uplift of the CP is marked by the disappearance of marine deposits (gray shading with inferred paleo-shoreline contours based on deposit ages; BMB = Black Mesa Basin; DB = Denver Basin - Fox Hills Group). The Sevier thrust front schematically shows the edge of the Sevier highlands and marks the western boundary of the CP. (C) By the Eocene, North-flowing paleocanyons had incised the southwestern plateau (orange arrows; MH = Milkweed-Hindu, PS = Peach Springs). Uplift to the north and east caused internal drainage and drainage reorganization (blue arrows). (D) In the Oligocene-Miocene, there was widespread volcanism (gray shading) on the edges of the CP. Continental extension and normal faulting to the West and South (Basin and Range, Rio Grande Rift) caused a drop in base-level and aided drainage reversal to the southwest (blue arrows). Paleogeographic reconstructions were adapted from these compilations: Hunt, 1956; Potochnik et al., 2001; Davis et al., 2009; Cather et al., 2012; Young and Crow, 2014; Hill et al., 2016; Karlstrom et al., 2020.
Here, we build on previous syntheses (e.g., Cather et al., 2012) to examine proposed CP paleoelevation estimates and highlight opportunities to refine the elevation history in light of recent proxy method advances. Decades of paleoelevation reconstructions combined with constraints from geology, geomorphology, and thermochronology support the interpretation that the CP was elevated through multi-stage, spatially differential uplift since the Laramide. Paleobotanical and geochemical proxy methods and the use of climate models to infer paleoelevation from proxy data have advanced significantly in the past 10–15 years and can be leveraged to refine and expand temperature-based paleoelevation reconstructions across the CP region.
Constraints on Colorado Plateau Paleotopography
Numerous geologic, thermochronologic, and geomorphic investigations constrain CP paleotopography, providing context for evaluating paleoaltimetry estimates (for details, see reviews by Hunt, 1956; Young and Spamer, 2001; Beard et al., 2011; Cather et al., 2012; Karlstrom et al., 2014; Ranney, 2014; Karlstrom et al., 2017; Crow et al., 2019; Karlstrom et al., 2019; Leopold and Zaborac-Reed, 2019; Hill and Polyak, 2020; Karlstrom et al., 2020).
Overview
CP pre-Cenozoic stratigraphy is characterized by relatively flat-lying sedimentary sequences, which suggests that the region was a comparatively stable sedimentary basin near or below sea-level for most of the Paleozoic-Mesozoic (Hunt, 1956). Coincident with onset of the Laramide (≥90 Ma; Carrapa et al., 2019), CP uplift likely swept from southwest to northeast (Figure 1B) suggested by the diachronous disappearance of Cretaceous Interior Seaway deposits (Nations et al., 1991; Raynolds and Johnson, 2003); barbed NE-flowing tributaries along sections of GC; and substantial (∼2 km) Paleozoic-Mesozoic to late Cretaceous-Paleogene denudation on the southwestern plateau indicated by erosional unconformities (e.g., Young, 2001; Hill et al., 2016). Imbricated gravels deposited in incised paleo-canyons (e.g., Milkweed-Hindu, Peach Springs, Salt River) on the southwestern CP margin in the late Cretaceous and Paleocene-Eocene support this view (e.g., Young, 2001; Potochnik et al., 2011), although the magnitude of Paleogene relief is debated (e.g., Karlstrom et al., 2014; Young and Crow, 2014).
The mid-Cenozoic (Figure 1C) was a period of internal drainage from the Sevier highlands to the west (e.g., Davis et al., 2009; Ibarra et al., 2021) and uplifts to the northeast (e.g., Cather et al., 2012). Drainage reorganization and tilting are evidenced by widespread fluvial and lacustrine deposits across the CP (Hunt, 1956) and lacustrine carbonate oxygen isotopic records (Davis et al., 2009). Late Cenozoic (Figure 1D) continental extension caused subsidence on the western and southern CP margins (McQuarrie and Wernicke, 2005). Drainage reversed to the southwest (e.g., Potochnik et.al., 2001), and a scarcity of late Cenozoic deposits suggests regional erosion (Hunt, 1956).
The modern CR was integrated from its headwaters in the Rocky Mountains through GC coincident with development of the Salton Trough by ∼6 Ma, recorded by CR deposits in the Muddy Creek Formation (e.g., Longwell, 1946; Lucchitta, 1972; Karlstrom et al., 2014). The CR reached its current base-level in the Gulf of California at 4.80–4.63 Ma (Crow et al., 2021).
Additional Uplift Constraints
Studies have investigated CP paleoelevation through reconstructions of erosion and river incision, which may occur in response to relief, drainage, and base-level changes that accompany surface uplift. Erosional exhumation may drive cooling of crustal rocks, which can be recorded by mineral thermal histories reconstructed from thermochronology (Reiners and Shuster, 2009), or constrained by other burial and thermal history information (Nuccio and Condon, 1996). For instance, thermochronometer cooling ages and peak burial temperature constraints from the southwestern CP surface suggests >1.5 km of denudation during the Laramide (e.g., Flowers et al., 2008), and ∼1.9–2.5 km of denudation prior to the Neogene (Ryb et al., 2021), supporting the interpretation that uplift of the southwestern CP margin initiated during the Laramide and may have continued into the late Cenozoic. Recent (<10 Ma) cooling of the southwestern CP surface rocks may reflect an erosional response to integration of the CR and a drop in base-level (e.g., Lee et al., 2013; Murray et al., 2016).
Cooling histories from canyon-rim and river-level samples track paleo-relief of GC (Flowers et al., 2008; Flowers and Farley, 2012; Flowers and Farley, 2013; Lee et al., 2013; Karlstrom et al., 2014; Winn et al., 2017) and constrain minimum plateau paleoelevation. A synthesis of thermochronologic datasets by Karlstrom et al. (2020) indicates segments of the GC were incised at different times before becoming integrated 6–5 Ma; the Western GC was likely a paleo-canyon with substantial relief incised by a northeast-flowing river by ∼65 Ma, whereas the Eastern GC was likely incised by a NW-flowing paleo-river by ∼20 Ma. These trends are compatible with multi-stage uplift prior to ∼20 Ma. Additional erosion constraints from volcanic deposits (e.g., Aslan et al., 2008; Donahue et al., 2013), cave deposits (Karlstrom et al., 2008; Polyak et al., 2008), and strath terraces show increased incision rates since ∼6 Ma, for example in eastern GC (Crow et al., 2014) and the Lower Colorado River (Karlstrom et al., 2017), potentially reflecting small-scale (<400 m) uplift of the southwestern plateau related to Neogene volcanism (Crow et al., 2011; Crow et al., 2019).
River longitudinal profiles may also constrain CP uplift timing (Roberts et al., 2012). However, complications due to varying rock erodibility (Cook et al., 2009; Pederson and Tressler, 2012), normal faulting and paleocanyon integration (Karlstrom et al., 2014), as well as drainage reorganization and resulting changes to stream power (Schwanghart and Scherler, 2020) limit straightforward correlation between river profiles and uplift. Nevertheless, an inverse correlation between channel steepness and upper mantle p-wave velocity in the Virgin River drainage suggests epeirogenic uplift affects modern CP river profiles (Walk et al., 2019). Projection of relict channels buried by Miocene and younger basalt (Hamblin et al., 1981), and knickpoint-bound upper reaches in CR tributaries (Darling and Whipple, 2015), indicate ∼1 km relief growth both prior to and since the Miocene. Such constraints do not distinguish between relief growth in response to CP uplift or subsidence at the plateau margin (Ott et al., 2018).
Previous Paleoaltimetry
Many workers have developed and applied direct paleoelevation proxies to resolve CP paleoelevation, though little agreement on methods or results has been reached (Figure 2; Supplementary Table S1). For instance, CP paleoelevation estimates based on basalt-vesicle paleobarometry (Sahagian et al., 2002a; Sahagian et al., 2002b; Sahagian et al., 2003), while elegant in theory, are problematic (Bondre, 2003; Libarkin and Chase, 2003), and the approach has not been replicated. Below, we evaluate CP paleoelevation estimates from oxygen-isotopic and paleothermometry-based methodologies.
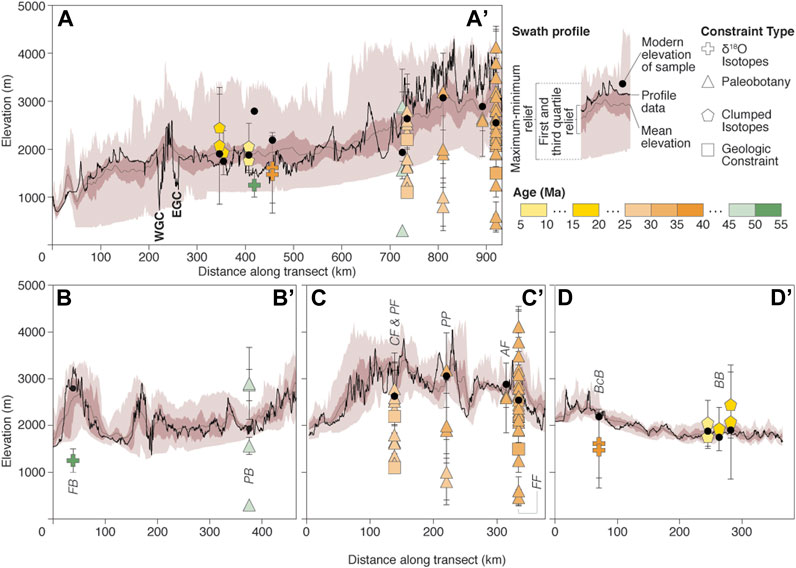
FIGURE 2. Compilation of paleoelevation estimates for the Colorado Plateau region. Error bars shown where available and represent reported uncertainties or range of possible elevations. Symbols correspond to proxy-type and color corresponds to age (consistent with Figure 1). For all panels, elevation profiles (black line) correspond to transects in Figure 1. Swath profiles of these transects are shown by mean elevation (gray line), the first and third quartile relief (dark shading), and the full maximum-minimum relief (light shading). (A) Compilation of all estimates projected onto elevation transect (A-A’); swath profile is cropped to the CP region and is 660 km across at its widest extent (330 km on either side of the transect). (B-D) Regional topographic swath profiles are 60 km wide (30 km on either side of the transect). Estimates are labeled by name of their geologic unit consistent with Figure 1 (BcB = Baca Basin; BB = Bidahochi Basin; FB = Flagstaff Basin; PB = Piceance Basin; CF = Creede Formation; AF = Antero Formation; FF = Florissant Formation; PP = Pitch-Pinnacle Formation; PF = Platoro Formation). (B) Estimates from the northern plateau projected onto elevation transect B-B’. (C) Estimates from the present-day Rocky Mountains projected onto elevation transect (C-C’). (D) Estimates from the southern plateau projected onto elevation transect (D-D’). All data and references are presented in Supplementary Table S1; data from MacGinitie, 1953; Steven and Ratté, 1965; MacGinitie, 1969; Axelrod and Bailey, 1976; Meyer, 1986; Axelrod, 1987; Wolfe and Schorn, 1989; Gregory and Chase, 1992; Meyer, 1992; Wolfe, 1992a; Wolfe, 1992b; Gregory, 1994; Wolfe, 1994; Gregory and McIntosh, 1996; Axelrod, 1998; Wolfe et al., 1998; Gregory-Wodziki, 2001; Leopold and Claypoole 2001; Boyle et al., 2008; Leopold et al., 2008; Huntington et al., 2010; Cather et al., 2012; Baumgartner and Meyer, 2014; Leopold and Zaborac-Reed, 2014; VanDevelde and Bowen, 2014; Zaborac-Reed and Leopold, 2016; Licht et al., 2017; Leopold and Zaborac-Reed, 2019; Allen et al., 2020.
δ18O-Based Paleoaltimetry
δ18O of precipitation decreases as air masses traverse increasing elevation (e.g., Rowley and Garzione, 2007). δ18O of authigenic carbonates (δ18Oc) precipitated from meteoric waters can be used to infer δ18O of precipitation and thus elevation, but several factors must be considered (Botsyun and Ehlers, 2021). In southwestern North America, summer and winter precipitation originate from different sources and commonly exhibit different δ18O values (Douglas et al., 1993; Blasch and Bryson, 2007), and summer monsoon precipitation is impacted by the “amount effect” (i.e., heavy precipitation is depleted in 18O whereas sparse precipitation is enriched in 18O; Dansgaard, 1964). Changing atmospheric conditions can further complicate precipitation δ18O through time (Licht et al., 2017). Moreover, various factors can blur the δ18O-elevation relationship preserved in δ18Oc, including temperature (e.g., Kim and O’Neil, 1997), evaporation (e.g., Licht et al., 2017), seasonal-bias (e.g., Breecker et al., 2009), and/or drainage reorganization (Davis et al., 2008).
Novel carbonate isotopic approaches have been developed to circumvent some complexities of reconstructing water δ18O values, for example by providing independent information on temperature (e.g., clumped isotopes; Huntington et al., 2010) and on unevaporated catchment water composition from triple-oxygen isotopes (Passey and Ji, 2019) or 13C-excess (Horton and Oze, 2012). Licht et al. (2017) and VanDeVelde and Bowen (2014) used these methods to estimate ∼1.5 km paleoelevation for the Baca Formation (∼38 Ma) and 1–1.5 km mean hypsometric paleoelevation for the Flagstaff Formation (∼50 Ma), respectively (Figure 2). Despite limitations, δ18Oc values from CP Eocene north-western basins may record increased mean hypsometric elevation (Figure 1A), lake closures resulting from local topographic growth diverting flows, and southward progression of drainage integration (Davis et al., 2009).
Paleobotanical Thermometry-Based Paleoaltimetry
The steepest temperature gradients on Earth are vertical, making surface temperature proxies attractive paleoaltimeters. Proxies have been used to estimate depositional mean annual surface temperature (paleo-MAST), which may be combined with coeval near-sea-level temperature reference data and an estimated rate of temperature decrease with elevation (“lapse-rate”) to infer paleoelevation.
Paleobotanical methods are perhaps the oldest and most widely used paleotemperature proxies (e.g., Bailey and Sinnott, 1915; Wolfe, 1979; Wolfe, 1994; Greenwood et al., 2004) and have been applied throughout the Cenozoic of North America (e.g., Retallack et al., 2004; Wing et al., 2005; Peppe et al., 2010). Bioclimatic proxies identify climate “envelopes” defined by the physical tolerances of sampled plant assemblages or their nearest living relatives (e.g., Mosbrugger and Utescher, 1997; Boyle et al., 2008; Thompson et al., 2012). Leaf physiognomic proxies are based on empirical correlations between temperature and physical properties of modern leaves, applied quantitatively to ancient floras using several different methods (e.g., Wolfe, 1993; Wilf, 1997; Spicer et al., 2009; Peppe et al., 2011). Paleobotanical proxies have been used widely and resulted in widely varying temperature and elevation reconstructions—as well as debate over when and where they are applicable in the geologic past (e.g., Kowalski and Dilcher, 2003; Royer and Wilf, 2006; Little et al., 2010; Peppe et al., 2010).
Studies of key Eocene-Oligocene sites (Figure 1) highlight this variability and permit several CP uplift histories (Figure 2; Supplementary Table S1 and references therein). MAST estimates based on different methodologies applied to the same fossil assemblage span a ∼13°C range for the Florissant flora, and other key floral sites at Creede and Pitch-Pinnacle have similarly wide ranges of temperature estimates (Leopold and Zaborac-Reed, 2019). Existing paleoelevation reconstructions based on paleobotanical-proxy temperatures and estimated lapse-rates (either study-specific lapse-rates, or using Wolfe, 1992a; Meyer, 1986; Meyer, 1992; discussed in Challenges and Recent Advances section) contain significant uncertainty—in some cases estimates range from <0.5 to >4 km for the same flora (e.g., Florissant; Figure 2C). Additional estimates calculated here using the refined Meyer (2007) approach (i.e., sea-level correction, local lapse-rate), based on paleobotanical-proxy temperature estimates that lack published paleoelevation evaluations, show similarly broad possibilities (Supplementary Table S1). This highlights the need for an evaluation of paleobotanical methods and their application, as well as the independent verification of temperature estimates and paleoelevation approaches.
Clumped Isotope Thermometry-Based Paleoaltimetry
Carbonate clumped isotope (Δ47) thermometry (Ghosh et al., 2006; Eiler, 2007; Eiler, 2011) is commonly used for reconstructing surface temperature and paleoelevation (Huntington and Lechler, 2015). The Δ47 value refers to the ratio of “clumped” carbonate molecules containing heavy isotopes 13C and 18O, relative to the random distribution of isotopes among isotopologues. Heavy isotope clumping is directly temperature-dependent, and the carbonate temperature estimates (TΔ47) are thermodynamically based; thus, the method can be applied in the geologic past without concern for plant evolutionary changes or variable paleobotanical-proxy methods, provided authigenic carbonates are available and well preserved. Clumped isotope data also constrain water δ18O values, and were used by Licht et al. (2017) to assess diagenesis and complement δ18O-based CP paleoaltimetry. TΔ47 for the Miocene lacustrine Bidahochi Formation (16–6 Ma), combined with TΔ47 of coeval near-sea-level deposits and a modern-lake-carbonate TΔ47 lapse-rate, estimated a paleoelevation of ∼1.9 km, suggesting that near-modern surface elevations of the southern CP were attained by 16 Ma (Figure 2; Huntington et al., 2010), consistent with thermochronology data (Karlstrom et al., 2017).
Discussion
Existing Estimates and Remaining Questions
Existing paleoelevation estimates are compatible with multi-stage, spatially differential uplift (Figure 2). Laramide-age deposits imply ≳1 km rise of the northwestern and southeastern CP by ∼50 and ∼38 Ma, respectively, consistent with paleo-canyon and paleo-relief studies. Geologic constraints from mid-Cenozoic (∼38 Ma) deposits suggest half- to near-modern elevations in the northeastern CP (Steven and Ratté, 1965; Cather et al., 2012), consistent with some paleobotanical-based estimates. Late-Cenozoic (∼16–6 Ma) deposits suggest near-modern elevations in the southwestern CP. Altogether, this suggests half-modern surface elevations by ∼38 Ma, and near-modern surface elevations by ∼16–6 Ma. The timing supports hypothesized Laramide and mid-Cenozoic uplift mechanisms but does not preclude more recent modest surface uplift or subsidence, or rock uplift that is balanced by erosion (<6 Ma on the southern CP).
Except for some paleobotanical-based estimates in the southern Rocky Mountains, for all regions and time periods paleoaltimetry data suggest that elevations were likely lower than or close to modern; this is consistent with geodynamic models and other constraints (e.g., Cather et al., 2012) and implies multi-stage uplift without a complex late-Cenozoic uplift and subsidence (c.f., Wolfe, 1994). The following remain to be resolved: the 1) amount and spatio-temporal pattern of Laramide-related surface uplift; 2) rate and timing of southern Rocky Mountains uplift, with implications for drainage reversal; 3) magnitude of possible ≲16 Ma CP elevation change; and 4) cause of variation in paleobotanical paleoelevation estimates.
A Path Forward With Temperature-Based Paleoaltimetry
Challenges and Recent Advances
Temperature-based paleoaltimetry methodologies are challenging, but have advanced substantially in the last decade and have potential to help address these remaining questions. Multiple factors independent of elevation influence MAST, including short- (e.g., El-Nino Southern Oscillation) and long-term climate changes (e.g., Cenozoic cooling). Additionally, climate variability on decadal to millennial time scales may be integrated differently depending on the resolution of the proxy, and seasonal bias may impact different proxy types and settings (e.g., Burgener et al., 2016). MAST varies spatially due to local vegetation cover, aspect, precipitation regime, or proximity to water bodies/the ocean (e.g., Kelson et al., 2020), which can change with secular or periodic changes in climate—potentially complicating comparison of known near-sea-level deposits with contemporaneous inland deposits of unknown elevation. Workers have addressed such complications in various ways; e.g., Huntington et al. (2010) analyzed the Bouse Formation as a near-sea-level anchor in two sub-basins, and opted to use estimates from the warmer sub-basin farther from the coast instead of the marine-biased colder sub-basin proximal to the coast. Feng and Poulsen (2016) used paleoclimate simulations of Eocene North America to make improved predictions of contemporaneous sea-level temperatures for Cordilleran floral sites.
There have also been varied approaches to determining lapse-rates, such as applying the average modern global (5.5°C/km), regional (3°C/km; Wolfe, 1992b), or local (e.g., 5.9°C/km; Meyer, 1986) lapse rate for the CP (e.g., Meyer, 2007). Lapse-rate may change with aridity/humidity and latitude (e.g., Neumann, 1955; Li et al., 2015), leading to skepticism about whether modern or global lapse-rates are applicable to ancient reconstructions. Paleoclimate models have advanced significantly since initial rates were proposed and demonstrate that significant error can be generated by applying linear modern lapse-rates to ancient climates and paleogeographies; model-mediated lapse-rates should be a key component of future temperature-based paleoaltimetry studies (e.g., Feng and Poulsen, 2016; Farnsworth et al., 2021; Botsyun and Ehlers, 2021).
Confidence and accuracy in paleobotanical temperature estimates also have improved significantly. Advances include new standardizations in leaf physiognomic methods (e.g., Peppe et al., 2011), regional corrections and character re-evaluations in multivariate methods (e.g., Spicer et al., 2021), larger comparative datasets and refined statistical methods in bioclimatic analyses (e.g., Chevalier, 2019), and improvements in paleofloral identifications and taxonomy (e.g., Manchester, 2014). Additionally, the number of available CP-region collections has increased; local and regional stratigraphy refinements have improved context and relationships between existing floras (e.g., Prothero, 2008), allowing for subsampling and clear division in collection units; and geochronology has refined ages and durations of distinct floras (e.g., Lipman and Bachmann, 2015), allowing for higher-resolution reconstructions and more precise application of corrections/lapse-rates.
Challenges of TΔ47 paleoaltimetry include: the need for up to tens of milligrams of carbonate to achieve precise temperature estimates, and relatively large elevation uncertainties (∼0.5 km; Huntington and Lechler, 2015); time integration during carbonate precipitation that can vary depending on climate (e.g., Kelson et al., 2020); and potential for carbonate temperature resetting by post-depositional diagenesis and heating (e.g., Huntington et al., 2011). Despite this, confidence and accuracy in Δ47 measurements have improved significantly in the last decade—with capability for higher sample throughput, more robust calibrations, new carbonate standards, and improved instrument precision and data normalization (e.g., Bernasconi et al., 2018; Petersen et al., 2019; Saenger et al., 2021). Evaluation of carbonate diagenesis also has improved (e.g., Lacroix and Niemi, 2019), and recent work indicates that the burial/exhumation history can be reconstructed from reset TΔ47 values with implications for paleotopography (e.g., Ning et al., 2019).
While transfer functions have been proposed to translate carbonate TΔ47 to MAST by assuming the seasonal timing of carbonate formation (Hren and Sheldon, 2012), using modern-carbonate-based lapse-rates may capture similar seasonal bias of ancient samples and reduce assumptions (e.g., Li et al., 2021). Quaternary lacustrine carbonates from southwest North America apparently record summer-biased temperature instead of MAST (Huntington et al., 2010). However, this bias may not hold for the paleo-record, particularly under Cretaceous and early Paleogene greenhouse climates. Novel isotopic approaches (triple-oxygen isotopes, 13C-excess) can help correct for evaporative effects and estimate the unevaporated δ18O of catchment waters, which may aid in constraining paleo-hydrology and provide context for estimating carbonate temperature seasonality and lapse-rates.
Future Opportunities
These advances can be leveraged to refine temperature-based proxy reconstructions and expand their use in the CP region. The region is well suited to temperature-based paleoaltimetry because its paleo-latitude has changed little for ∼80 Ma; the mid-latitude setting allows for a steeper and better-constrained temperature-elevation gradient, thus minimizing elevation uncertainty; and target deposits are widespread in space and time and unlikely to have been deeply buried and diagenetically altered.
Essential to resolving paleobotanical estimates is independent comparison of carbonate TΔ47 and well-studied floral records from the same lacustrine formations (e.g., Florissant, Creede, Antero). Systematic comparison could expand the applicability and comparability of techniques across additional Laramide-age localities (Figure 1A) that may contain either carbonates or fossil floras (Claron, Flagstaff, Uinta, Piceance, and San Juan Basins), and potentially provide context for interpreting 14–19°C early calcite cements from the ∼64 Ma southwestern CP Music Mountain Formation (Huntington et al., 2011). Additionally, there is opportunity to re-evaluate late-Cenozoic Bidahochi and Bouse Formation paleotemperatures (Huntington et al., 2010) with higher temporal/spatial resolution. In all cases, we recommend using paleoclimate model-mediated lapse-rates and sea-level corrections to minimize uncertainty and align methods for extrapolating temperature to elevation. Our analysis suggests that temperature-based paleoaltimetry methods are key to refining CP paleoelevation, with implications for understanding uplift mechanisms and CR drainage evolution.
Author Contributions
EOH and KH (corresponding authors) conceived of the manuscript, with input from EGH. EOH did much of the literature review, first drafts of writing, and compilation of datasets. KH contributed to the writing and literature review on the Colorado Plateau, paleoaltimetry, and clumped isotopes. EGH contributed to the writing and literature review for paleobotanical methods. PSG drafted Figure 1 and contributed to the writing and literature review of river profile modelling. CB drafted Figure 2. All authors provided input on data analysis, interpretation, and writing.
Conflict of Interest
The authors declare that the research was conducted in the absence of any commercial or financial relationships that could be construed as a potential conflict of interest.
Acknowledgments
We acknowledge funding from the University of Washington, Quaternary Research Center, and Endowed Professorship for the College of the Environment in Earth Systems. We thank Dave Sharrow, Elena Stiles, the IsoLab Clumped Group, Alex Lechler, Megan Mueller and Susannah Morey for insightful conversations. We thank Alexis Licht and Paolo Ballato for encouraging this submission and Associate Editor Heiko Pingel for editorial support and suggestions. We also thank Laura Crossey, Cari Johnson, and Richard Ott for their insightful comments that greatly improved this manuscript.
Supplementary Material
The Supplementary Material for this article can be found online at: https://www.frontiersin.org/articles/10.3389/feart.2021.648605/full#supplementary-material
References
Allen, S. E., Lowe, A. J., Peppe, D. J., and Meyer, H. W. (2020). Paleoclimate and Paleoecology of the Latest Eocene Florissant flora of central Colorado, U.S.A. Palaeogeogr. Palaeoclimatol. Palaeoecol. 551, 109678. doi:10.1016/j.palaeo.2020.109678
Aslan, A., Karlstrom, K., Hood, W. C., Cole, R. D., Oesleby, T. W., Betton, C., et al. (2008). River Incision Histories of the Black Canyon of the Gunnison and Unaweep Canyon: Interplay between Late Cenozoic Tectonism, Climate Change, and Drainage Integration in the Western Rocky Mountains. GSA Field Guide 10: Roaming the Rocky Mountains and Environs: Geological Field Trips. Colorado: Geological Society of America, 175–202. doi:10.1130/2008.fld010(09)
Axelrod, D. I. (1987). The Late Oligocene Creede flora. Colorado: University of California Press (LosAngeles, CA), 235.
Axelrod, D. I., and Bailey, H. P. (1976). Tertiary Vegetation, Climate, and Altitude of the Rio Grande Depression, New Mexico-Colorado. Paleobiology 2, 235–254. doi:10.1017/s0094837300004814
Axelrod, D. I. (1998). The Oligocene Haynes Flora of Eastern Idaho. Colorado: University of California Publications Geol. Sci. 143, 1–235.
Bailey, I. W., and Sinnott, E. W. (1915). A Botanical index of Cretaceous and Tertiary Climates. Science 41, 831–834. doi:10.1126/science.41.1066.831
Baumgartner, K. A., and Meyer, H. W. (2014). Coexistence Climate Analysis of the Late Eocene Florissant Flora, Colorado. GSA Abstracts with Programs 490, 46.
Beard, L. S., Karlstrom, K. E., Young, R. A., and Billingsley, G. H. (2011). in C Revolution 2—Origin and evolution of the Colorado River system, workshop abstracts: U.S. Geological Survey Open-File Report 2011–1210, 300p Available at https://pubs.usgs.gov/of/2011/1210/ (Accessed December 16, 2020).
Bernasconi, S. M., Müller, I. A., Bergmann, K. D., Breitenbach, S. F. M., Fernandez, A., Hodell, D. A., et al. (2018). Reducing Uncertainties in Carbonate Clumped Isotope Analysis through Consistent Carbonate-Based Standardization. Geochem. Geophys. Geosyst. 19, 2895–2914. doi:10.1029/2017GC007385
Bird, P. (1979). Continental Delamination and the Colorado Plateau. J. Geophys. Res. 84, 7561–7571. doi:10.1029/JB084iB13p07561
Blasch, K. W., and Bryson, J. R. (2007). Distinguishing Sources of Ground Water Recharge by Using ?2H and ?18O. Ground Water 45, 294–308. doi:10.1111/j.1745-6584.2006.00289.x
Bondre, N. R. (2003). Analysis of Vesicular Basalts and Lava Emplacement Processes for Application as a Paleobarometer/Paleoaltimeter: A Discussion. J. Geology. 111, 499–502. doi:10.1086/375279
Botsyun, S., and Ehlers, T. A. (2021). How Can Climate Models Be Used in Paleoelevation Reconstructions?. Front. Earth Sci.9, 624542. doi:10.3389/feart.2021.624542
Boyle, B., Meyer, H. W., Enquist, B., and Salas, S. (2008). Higher Taxa as Paleoecological and. Paleoclimatic Indicators: A Search for the Modern Analog of the Florissant Fossil flora. Geol. S. Am. S. 435, 33–51. doi:10.1130/2008.2435(03)
Breecker, D. O., Sharp, Z. D., and McFadden, L. D. (2009). Seasonal Bias in the Formation and Stable Isotopic Composition of Pedogenic Carbonate in Modern Soils from central New Mexico, USA. Geol. Soc. America Bull. 121, 630–640. doi:10.1130/B26413.1
Burgener, L., Huntington, K. W., Hoke, G. D., Schauer, A., Ringham, M. C., Latorre, C., et al. (2016). Variations in Soil Carbonate Formation and Seasonal Bias over >4 Km of Relief in the Western Andes (30°S) Revealed by Clumped Isotope Thermometry. Earth Planet. Sci. Lett. 441, 188–199. doi:10.1016/j.epsl.2016.02.033
Carrapa, B., DeCelles, P. G., and Romero, M. (2019). Early Inception of the Laramide Orogeny in Southwestern Montana and Northern Wyoming: Implications for Models of Flat-Slab Subduction. J. Geophys. Res. Solid Earth 124, 2102–2123. doi:10.1029/2018JB016888
Cather, S. M., Chapin, C. E., and Kelley, S. A. (2012). Diachronous Episodes of Cenozoic Erosion in Southwestern North America and Their Relationship to Surface Uplift, Paleoclimate, Paleodrainage, and Paleoaltimetry. Geosphere 8, 1177–1206. doi:10.1130/GES00801.1
Chevalier, M. (2019). Enabling Possibilities to Quantify Past Climate from Fossil Assemblages at Aglobal Scale. Glob. Planet. Change 175, 27–35. doi:10.3917/dunod.cheva.2019.01
Cook, K. L., Whipple, K. X., Heimsath, A. M., and Hanks, T. C. (2009). Rapid Incision of the Colorado River in Glen Canyon - Insights from Channel Profiles, Local Incision Rates, and Modeling of Lithologic Controls. Earth Surf. Process. Landforms 34 (7), 994–1010. doi:10.1002/esp.1790
Crow, R., Karlstrom, K., Asmerom, Y., Schmandt, B., Polyak, V., and DuFrane, S. A. (2011). Shrinking of the Colorado Plateau via Lithospheric Mantle Erosion: Evidence from Nd and Sr Isotopes and Geochronology of Neogene Basalts. Geology 39, 27–30. doi:10.1130/G31611.1
Crow, R., Karlstrom, K., Darling, A., Crossey, L., Polyak, V., Granger, D., et al. (2014). Steady Incision of Grand Canyon at the Million Year Timeframe: A Case for Mantle-Driven Differential Uplift. Earth Planet. Sci. Lett. 397, 159–173. doi:10.1016/j.epsl.2014.04.020
Crow, R. S., Howard, K. A., Beard, L. S., Pearthree, P. A., House, P. K., Karlstrom, K. E., et al. (2019). Insights into post-Miocene Uplift of the Western Margin of the Colorado Plateau from the Stratigraphic Record of the Lower Colorado River. Geosphere 15, 1826–1845. doi:10.1130/GES02020.1
Crow, R. S., Schwing, J., Karlstrom, K. E., Heizler, M., Pearthree, P. A., House, P. K., et al. (2021). Redefining the Age of the Lower Colorado River, Southwestern United States. Geology 49, 7. doi:10.1130/G48080.1
Dansgaard, W. (1964). Stable Isotopes in Precipitation. Tellus 16, 436–468. doi:10.3402/tellusa.v16i4.8993
Darling, A., and Whipple, K. (2015). Geomorphic Constraints on the Age of the Western Grand Canyon. Geosphere 11, 958–976. doi:10.1130/GES01131.1
Davis, S. J., Mulch, A., Carroll, A. R., Horton, T. W., and Chamberlain, C. P. (2006). Paleogene Landscape Evolution of the central North American Cordillera: Developing Topography and Hydrology in the Laramide Foreland. Geol. Soc. America Bull. preprint, 1–116. doi:10.1130/B26308.1
Davis, S. J., Wiegand, B. A., Carroll, A. R., and Chamberlain, C. P. (2008). The Effect of Drainage Reorganization on Paleoaltimetry Studies: An Example from the Paleogene Laramide Foreland. Earth Planet. Sci. Lett. 275, 258–268. doi:10.1016/j.epsl.2008.08.009
Donahue, M., Karlstrom, K. E., Aslan, A., Darling, A., Granger, D., Wan, E., et al. (2013). Incision History of the Black Canyon of Gunnison, Colorado, over the Past ∼1 ma Inferred from Dating of Fluvial Gravel Deposits. Geosphere 9, 815–826. doi:10.1130/GES00847.1
Douglas, M. W., Maddox, R. A., Howard, K., and Reyes, S. (1993). The Mexican Monsoon. J. Clim. 6, 1665–1677. doi:10.1175/1520-0442(1993)006<1665:TMM>2.0.CO;2
Eiler, J. M. (2007). "Clumped-isotope" Geochemistry-The Study of Naturally-Occurring, Multiply-Substituted Isotopologues. Earth Planet. Sci. Lett. 262, 309–327. doi:10.1016/j.epsl.2007.08.020
Eiler, J. M. (2011). Paleoclimate Reconstruction Using Carbonate Clumped Isotope Thermometry. Quat. Sci. Rev. 30, 3575–3588. doi:10.1016/j.quascirev.2011.09.001
Farnsworth, A., Valdes, P. J., Spicer, R. A., Ding, L., Witkowski, C., Lauretano, V., et al. (2021). Paleoclimate Model-Derived thermal Lapse Rates: Towards Increasing Precision in Paleoaltimetry Studies. Earth Planet. Sci. Lett. 564, 116903. doi:10.1016/j.epsl.2021.116903
Feng, R., and Poulsen, C. J. (2016). Refinement of Eocene Lapse Rates, Fossil-Leaf Altimetry, and North American Cordilleran Surface Elevation Estimates. Earth Planet. Sci. Lett. 436, 130–141. doi:10.1016/j.epsl.2015.12.022
Flowers, R. M., and Farley, K. A. (2012). Apatite 4He/3He and (U-Th)/He Evidence for an Ancient Grand Canyon. Science 338, 1616–1619. doi:10.1126/science.1229390
Flowers, R. M., and Farley, K. A. (2013). Response to Comments on "Apatite 4He/3He and (U-Th)/He Evidence for an Ancient Grand Canyon". Science 340, 143. doi:10.1126/science.1234203
Flowers, R. M., Wernicke, B. P., and Farley, K. A. (2008). Unroofing, Incision, and Uplift History of the Southwestern Colorado Plateau from Apatite (U-Th)/He Thermochronometry. Geol. Soc. America Bull. 120, 571–587. doi:10.1130/B26231.1
Ghosh, P., Adkins, J., Affek, H., Balta, B., Guo, W., Schauble, E. A., et al. (2006). 13C-18O Bonds in Carbonate Minerals: A New Kind of Paleothermometer. Geochimica et Cosmochimica Acta 70, 1439–1456. doi:10.1016/j.gca.2005.11.014
Greenwood, D. R., Wilf, P., Wing, S. L., and Christophel, D. C. (2004). Paleotemperature Estimation Usingleaf-Margin Analysis: Is Australia Different? Palaios 19, 129–142. doi:10.4324/9780203500804
Gregory, K. M., and Chase, C. G. (1992). Tectonic Significance of Paleobotanically Estimated Climate and Altitude of the Late Eocene Erosion Surface, Colorado. Geology 20, 581–585. doi:10.1130/0091-7613(1992)020<0581:TSOPEC>2.3.CO;2
Gregory, K. M., and McIntosh, W. C. (1996). Paleoclimate and Paleoelevation of the Oligocene Pitch-Pinnacle flora, Sawatch Range. Colo. GSA Bull. 108, 545–561. doi:10.1130/0016-7606(1996)108<0545:PAPOTO>2.3.CO;2
Gregory, K. M. (1994). Paleoclimate and Paleoelevation of the 35 Ma Florissant flora. Front. Range, Coloradopaleoclimates 1, 23–57.
Gregory-Wodziki, K. M. (2001). “Paleoclimatic Implications of Tree-Ring Growth Characteristics of 34.1 Ma Sequoioxylon Pearsallii from Florissant, Colorado,” in Fossil Flora and Stratigraphy of the Florissant Formation, Colorado. Editors E. Evanoff, K. M. Gregory-Wodziski, and K. R. Johnson (Denver, CO: Proceedings of the Denver Museum of Nature and Science Series), 4, 163–186.
Hamblin, W. K., Damon, P. E., and Bull, W. B. (1981). Estimates of Vertical crystal Strain Rates Alongthe Western Margins of the Colorado Plateau. Geology 9 (7), 293–298. doi:10.1130/0091-7613(1981)9<293:EOVCSR>2.0.CO;2
Hernández-Uribe, D., and Palin, R. M. (2019). Catastrophic Shear-Removal of Subcontinental Lithospheric Mantle beneath the Colorado Plateau by the Subducted Farallon Slab. Sci. Rep. 9, 1–10. doi:10.1038/s41598-019-44628-y
Hill, C. A., and Polyak, V. J. (2020). A Karst Hydrology Model for the Geomorphic Evolution of Grand Canyon, Arizona, USA. Earth-Science Rev. 208, 103279. doi:10.1016/j.earscirev.2020.103279
Hill, C. A., Polyak, V. J., Asmerom, Y., and P. Provencio, P. (2016). Constraints on a Late Cretaceous Uplift, Denudation, and Incision of the Grand Canyon Region, Southwestern Colorado Plateau, USA, from U-Pb Dating of Lacustrine limestone. Tectonics 35, 896–906. doi:10.1002/2016TC004166
Horton, T. W., and Oze, C. (2012). Are Two Elements Better Than One? Dual Isotope-Ratio Detrending of Evaporative Effects on lake Carbonate Paleoelevation Proxies. Geochem. Geophys. Geosyst. 13 (6), Q0AK05. doi:10.1029/2012GC004132
Hren, M. T., and Sheldon, N. D. (2012). Temporal Variations in lake Water Temperature: Paleoenvironmental Implications of lake Carbonate δ18O and Temperature Records. Earth Planet. Sci. Lett. 337-338, 77–84. doi:10.1016/j.epsl.2012.05.019
Hunt, C. B. (1956). Cenozoic Geology of the Colorado Plateau. in (USGS Numbered Series No. 279). Professional Paper 279, 103. doi:10.3133/pp279
Huntington, K. W., Budd, D. A., Wernicke, B. P., and Eiler, J. M. (2011). Use of Clumped-Isotope Thermometry to Constrain the Crystallization Temperature of Diagenetic Calcite. J. Sediment. Res. 81, 656–669. doi:10.2110/jsr.2011.51
Huntington, K. W., and Lechler, A. R. (2015). Carbonate Clumped Isotope Thermometry in continental Tectonics. Tectonophysics 647-648, 1–20. doi:10.1016/j.tecto.2015.02.019
Huntington, K. W., Wernicke, B. P., and Eiler, J. M. (2010). Influence of Climate Change and Uplift on Colorado Plateau Paleotemperatures from Carbonate Clumped Isotope Thermometry. Tectonics 29 (3), TC3005. doi:10.1029/2009TC002449
Ibarra, D. E., Kukla, T., Methner, K. A., Mulch, A., and Chamberlain, C. P. (2021). Reconstructing Past Elevations From Triple Oxygen Isotopes of Lacustrine Chert: Application to the Eocene Nevadaplano, Elko Basin, Nevada, United States. Front. Earth Sci.9, 628868. doi:10.3389/feart.2021.628868
Karlstrom, K. E., Crossey, L. J., Embid, E., Crow, R., Heizler, M., Hereford, R., et al. (2017). Cenozoic Incision History of the Little Colorado River: Its Role in Carving Grand Canyon and Onset of Rapid Incision in the Past Ca. 2 Ma in the Colorado River System. Geosphere 13, 49–81. doi:10.1130/GES01304.1
Karlstrom, K. E., Crossey, L. J., Humphreys, E., Shuster, D., and Whipple, K. (2019). AGE AND CARVING OF GRAND CANYON TOWARDS A RESOLUTION OF 150 yearS OF DEBATE. GSA Annual Meeting in Phoenix. USA-: Arizona, September 22–25, 2019. doi:10.1130/abs/2019am-338944
Karlstrom, K. E., Crow, R., Crossey, L. J., Coblentz, D., and Van Wijk, J. W. (2008). Model for Tectonically Driven Incision of the Younger Than 6 Ma Grand Canyon. Geol 36, 835–838. doi:10.1130/G25032A.1
Karlstrom, K. E., Jacobson, C. E., Sundell, K. E., Eyster, A., Blakey, R., Ingersoll, R. V., et al. (2020). Evaluating the Shinumo-Sespe Drainage Connection: Arguments against the "old" (70-17 Ma) Grand Canyon Models for Colorado Plateau Drainage Evolution. Geosphere 16, 1425–1456. doi:10.1130/GES02265.1
Karlstrom, K. E., Lee, J. P., Kelley, S. A., Crow, R. S., Crossey, L. J., Young, R. A., et al. (2014). Formation of the Grand Canyon 5 to 6 Million Years Ago through Integration of Older Palaeocanyons. Nat. Geosci 7, 239–244. doi:10.1038/ngeo2065
Kelson, J. R., Huntington, K. W., Breecker, D. O., Burgener, L. K., Gallagher, T. M., Hoke, G. D., et al. (2020). A Proxy for All Seasons? A Synthesis of Clumped Isotope Data from Holocene Soil Carbonates. Quat. Sci. Rev. 234, 106259. doi:10.1016/j.quascirev.2020.106259
Kim, S.-T., and O'Neil, J. R. (1997). Equilibrium and Nonequilibrium Oxygen Isotope Effects in Synthetic Carbonates. Geochimica et Cosmochimica Acta 61, 3461–3475. doi:10.1016/S0016-7037(97)00169-5
Kowalski, E. A., and Dilcher, D. L. (2003). Warmer Paleotemperatures for Terrestrial Ecosystems. Natl. Acad. Sci. USA, 100, 167–170. doi:10.1073/pnas.232693599
Lacroix, B., and Niemi, N. A. (2019). Investigating the Effect of Burial Histories on the Clumped Isotope Thermometer: An Example from the Green River and Washakie Basins, Wyoming. Geochimica et Cosmochimica Acta 247, 40–58. doi:10.1016/j.gca.2018.12.016
Lazear, G., Karlstrom, K., Aslan, A., and Kelley, S. (2013). Denudation and Flexural Isostatic Response of the Colorado Plateau and Southern Rocky Mountains Region since 10 Ma. Geosphere 9, 792–814. doi:10.1130/GES00836.1
Lee, J. P., Stockli, D. F., Kelley, S. A., Pederson, J. L., Karlstrom, K. E., and Ehlers, T. A. (2013). New Thermochronometric Constraints on the Tertiary Landscape Evolution of the central and Eastern Grand Canyon, Arizona. Geosphere 9, 216–228. doi:10.1130/GES00842.1
Leopold, E. B., and Claypoole, S. T. (2001). “Florissant Leaf and Pollen Floras of Colorado Compared Climatic Implications,” in Fossil flora and Stratigraphy of the Florissant Formation, Colorado. Editors E. Evanoff, K. M. Gregory-Wodzicki, and K. Johnson (Denver, CO): Denver Museum of Nature and Science), 17–70.
Leopold, E. B., Manchester, S., and Meyer, H. W. (2008). “Phytogeography of the Late EoceneFlorissant flora Reconsidered,”. Paleontology of the Upper Eocene Florissant Formation. Editors H. W. Meyer, and D. M. Smith (Colorado: Geological Society of America Special Paper), 435, 53–70. doi:10.1130/2008.2435(04)
Leopold, E. B., and Zaborac-Reed, S. (2019). Pollen Evidence of Floristic Turnover Forced by Cool Aridity during the Oligocene in Colorado. Geosphere 15, 254–294. doi:10.1130/GES01689.1
Leopold, E., and Zaborac-Reed, S. (2014). “Biogeographic History of Abies bracteata (D. Don) A. Poit. in the Western United States,”. Paleobotany and Biogeography. Editors W. D. Stevens, O. M. Montiel, and P. H. Raven (St. Louis, MO: Missouri Botanical Garden Press), 252–286.
Li, H., Liu, X., Arnold, A., Elliott, B., Flores, R., Kelley, A. M., et al. (2021). Mass 47 Clumped Isotope Signatures in Modern Lacustrine Authigenic Carbonates in Western China and Other Regions and Implications for Paleotemperature and Paleoelevation Reconstructions. Earth Planet. Sci. Lett. 562, 116840. doi:10.1016/j.epsl.2021.116840
Li, Y., Zeng, Z., Zhao, L., and Piao, S. (2015). Spatial Patterns of Climatological Temperature Lapse Rate in mainland China: A Multi-Time Scale Investigation. J. Geophys. Res. Atmos. 120, 2661–2675. doi:10.1002/2014JD022978
Libarkin, J. C., and Chase, C. G. (2003). Timing of Colorado Plateau Uplift: Initial Constraints from Vesicular basalt-derived Paleoelevations: Comment and ReplyCOMMENT. Geology 31, 191. doi:10.1130/0091-7613(2003)031<0191:TOCPUI>2.0.CO;2
Licht, A., Quade, J., Kowler, A., de los Santos, M., Hudson, A., Schauer, A., et al. (2017). Impact of the North American Monsoon on Isotope Paleoaltimeters: Implications for the Paleoaltimetry of the American Southwest. Am. J. Sci. 317, 1–33. doi:10.2475/01.2017.01
Lipman, P. W., and Bachmann, O. (2015). Ignimbrites to Batholiths: Integrating Perspectives from Geological, Geophysical, and Geochronological Data. Geosphere, 11, 705–743. doi:10.1130/GES01091.1
Little, S. A., Kembel, S. W., and Wilf, P. (2010). Paleotemperature Proxies from Leaf Fossils Reinterpreted in Light of Evolutionary History. PLoS One 5, E15161. doi:10.1017/cbo9780511709371
Longwell, C. R. (1946). How Old Is the Colorado River? Am. J. Sci. 244, 817–835. doi:10.2475/ajs.244.12.817
Lucchitta, I. (1972). Early History of the Colorado River in the Basin and Range Province. GSA Bull. 83, 1933–1948. doi:10.1130/0016-7606(1972)83[1933:EHOTCR]2.0.CO;2
MacGinitie, H. D. (1953). Fossil Plants of the Florissant Beds, Colorado. Carnegie Wash. Pub. 599, 1–188.
MacGinitie, H. D. (1969). The Eocene green River flora of Northwestern Colorado and Northeastern. Utah. University of California Press. doi:10.1037/e463452008-093
Manchester, S. R. (2014). Revisions to Roland Brown's North American Paleocene flora. Acta Musei. Nationalis. Pragae, Series B-Historia. Naturalis 70, 153–210. doi:10.14446/AMNP.2014.153
McQuarrie, N., and Chase, C. G. (2000). Raising the Colorado Plateau. Geology 28, 91–94. doi:10.1130/0091-7613(2000)028<0091:RTCP>2.0.CO;2
McQuarrie, N., and Wernicke, B. P. (2005). An Animated Tectonic Reconstruction of Southwestern North America since 36 Ma. Geosphere 1, 147–172. doi:10.1130/GES00016.1
Meyer, H. W. (2007). A Review of Paleotemperature Lapse Rate Methods for Estimating Paleoelevation from Fossil Floras. Rev. Mineralogy Geochem. 66, 155–171. doi:10.2138/rmg.2007.66.6
Meyer, H. W. (1986). An Evaluation of the Methods for Estimating Paleoaltitudes Using Tertiary Floras from the Rio Grande Rift Vicinity. in New Mexico and Colorado(Oligocene Mountain Climates) (Ph.D) (University of California, Berkeley, United States -- California.)
Meyer, H. W. (1992). Lapse Rates and Other Variables Applied to Estimating Paleoaltitudes from Fossil, Floras. Palaeogeogr. Palaeocl. 99, 71–99. doi:10.3386/w4197
Mosbrugger, V., and Utescher, T. (1997). The Coexistence Approach – A Method for Quantitative Reconstructions of Tertiary Terrestrial Paleoclimate Data Using Plant Fossils. Palaeogeogr. Palaeocl., 134, 203–214. doi:10.1016/S0031-0182(96)00154-X
Moucha, R., Forte, A. M., Rowley, D. B., Mitrovica, J. X., Simmons, N. A., and Grand, S. P. (2009). Deep Mantle Forces and the Uplift of the Colorado Plateau. Geophys. Res. Lett. 36 (19), L19310. doi:10.1029/2009GL039778
Murray, K. E., Reiners, P. W., and Thomson, S. N. (2016). Rapid Pliocene-Pleistocene Erosion of the central Colorado Plateau Documented by Apatite Thermochronology from the Henry Mountains. Geology 44, 483–486. doi:10.1130/G37733.1
Nations, D., Nations, J. D., and Eaton, J. G. (1991). Stratigraphy, Depositional Environments, and Sedimentary Tectonics of the Western MarginCretaceous Western Interior Seaway. Colorado: Geological Society of America.
Neumann, J. (1955). Latitudinal Variation of Tropospheric Temperature Lapse Rate. Arch. Met. Geoph. Biokl. A. 8, 351–353. doi:10.1007/BF02247093
Ning, Z., Zhang, L., Huntington, K. W., Wang, C., Dai, J., Han, Z., et al. (2019). The Burial and Exhumation History of the Liuqu Conglomerate in the Yarlung Zangbo Suture Zone, Southern Tibet: Insights from Clumped Isotope Thermometry. J. Asian Earth Sci. 174, 205–217. doi:10.1016/j.jseaes.2018.12.009
Nuccio, V. F., and Condon, S. M. (1996). Burial and thermal History of the Paradox Basin, Utah and Colorado, and Petroleum Potential of the Middle Pennsylvanian Paradox Formation. U.S. Geological Survey Bulletin 2000-O. 57–76.
Ott, R. F., Whipple, K. X., and van Soest, M. (2018). Incision History of the Verde Valley Region and Implications for Uplift of the Colorado Plateau (central Arizona). Geosphere 14, 1690–1709. doi:10.1130/GES01640.1
Passey, B. H., and Ji, H. (2019). Triple Oxygen Isotope Signatures of Evaporation in lake Waters and Carbonates: A Case Study from the Western United States. Earth Planet. Sci. Lett. 518, 1–12. doi:10.1016/j.epsl.2019.04.026
Pederson, J. L., and Tressler, C. (2012). Colorado River Long-Profile Metrics, Knickzones and Their Meaning. Earth Planet. Sci. Lett. 345-348, 171–179. doi:10.1016/j.epsl.2012.06.047
Peppe, D. J., Royer, D. L., Cariglino, B., Oliver, S. Y., Newman, S., Leight, E., et al. (2011). Sensitivity of leaf size and shape to climate: Global patterns and paleoclimatic applications. New Phytol. 190, 724–739. doi:10.1037/t44508-000
Peppe, D. J., Royer, D. L., Wilf, P., and Kowalski, E. A. (2010). Quantification of Large Uncertainties in Fossil Leaf Paleoaltimetry. Tectonics 29 (3), TC3015. doi:10.1029/2009TC002549
Petersen, S. V., Defliese, W. F., Saenger, C., Daëron, M., Huntington, K. W., John, C. M., et al. (2019). Effects of Improved 17 O Correction on Interlaboratory Agreement in Clumped Isotope Calibrations, Estimates of Mineral-Specific Offsets, and Temperature Dependence of Acid Digestion Fractionation. Geochem. Geophys. Geosyst. 20, 3495–3519. doi:10.1029/2018GC008127
Polyak, V., Hill, C., and Asmerom, Y. (2008). Age and Evolution of the Grand Canyon Revealed by U-Pb Dating of Water Table-type Speleothems. Science 319, 1377–1380. doi:10.1126/science.1151248
Porter, R., Hoisch, T., and Holt, W. E. (2017). The Role of Lower-Crustal Hydration in the Tectonic Evolution of the Colorado Plateau. Tectonophysics 712-713, 221–231. doi:10.1016/j.tecto.2017.05.025
Potochnik, A. R., Beard, L. S., Karlstrom, K. E., Young, R. A., and Billingsley, G. H. (2011). Ancestral Colorado River Exit from the Plateau Province—Salt River Hypothesis. CRevolution2: US Geological Survey Open-File Report 1210, 222–231.
Potochnik, A. R., Young, R. A., and Spamer, E. E. (2001). Paleogeomorphic Evolution of the Salt River region: Implications for Cretaceous-Laramide Inheritance for Ancestral Colorado River Drainage. Colorado River Origin and Evolution: Grand Canyon, Arizona. Grand Canyon Association, 17–24.
Prothero, D. R. (2008). Magnetic Stratigraphy of the Eocene-Oligocene Floral Transition in westernPaleontology of the Upper Eocene Florissant Formation North America. Paleontology of the Upper Eocene Florissant Formation, Colorado 435, 71. doi:10.1130/2008.2435(05)
Ranney, W. (2014). A Pre-21st century History of Ideas on the Origin of the Grand Canyon. Geosphere 10, 233–242. doi:10.1130/GES00960.1
Raynolds, R. G., and Johnson, K. R. (2003). Synopsis of the Stratigraphy and Paleontology of the Uppermost Cretaceous and Lower Tertiary Strata in the Denver Basin, Colorado. Rocky Mountain Geology. 38, 171–181. doi:10.2113/gsrocky.38.1.171
Reiners, P. W., and Shuster, D. L. (2009). Thermochronology and Landscape Evolution. Phys. Today 62, 31–36. doi:10.1063/1.3226750
Orr, G. J., Prothero, W. N., Duncan, D. R., Kester, R. A., Ambers, P. R., and au, C. P. (2004). Eocene-Oligocene Extinction and Paleoclimatic Change Near Eugene, Oregon. Geol. Soc. America Bull. 116, 817–839. doi:10.1130/b25281.1
Roberts, G. G., White, N. J., Martin-Brandis, G. L., and Crosby, A. G. (2012). An Uplift History of the Colorado Plateau and its Surroundings from Inverse Modeling of Longitudinal River Profiles. Tectonics 31 (4), TC4022. doi:10.1029/2012TC003107
Rowley, D. B., and Garzione, C. N. (2007). Stable Isotope-Based Paleoaltimetry. Annu. Rev. Earth Planet. Sci. 35, 463–508. doi:10.1146/annurev.earth.35.031306.140155
Royer, D. L., and Wilf, P. (2006). Why Do Toothed Leaves Correlate with Cold Climates? Gas Exchange Atleaf Margins Provides New Insights into a Classic Paleotemperature Proxy. Int. J. Plant Sci. 167, 11–18.
Ryb, U., Lloyd, M. K., and Eiler, J. M., (2021). Carbonate Clumped Isotope Constraints on Burial, Uplift and exhumation histories of the Colorado Plateau. Earth Planet. Sci. Lett., 566, 116964. doi:10.1016/j.epsl.2021.116964
Saenger, C. P., Schauer, A. J., Heitmann, E. O., Huntington, K. W., and Steig, E. J. (2021). How 17O excessIn Clumped Isotope Reference-Frame Materials and ETH Standards Affects Reconstructed Temperature. Chem. Geology., 563, 120059. doi:10.1007/s11002-021-09561-z
Sahagian, D. L., Proussevitch, A. A., and Carlson, W. D., (2002a). Analysis of Vesicular Basalts and Lava Emplacement Processes for Application as a Paleobarometer/Paleoaltimeter. J. Geology. 110, 671–685. doi:10.1086/342627
Sahagian, D., Proussevitch, A., and Carlson, W., (2002b). Timing of Colorado Plateau Uplift: Initial Constraints from Vesicular basalt-derived Paleoelevations. Geology 30, 807–810. doi:10.1130/0091-7613(2002)030<0807:TOCPUI>2.0.CO;2
Sahagian, D., Proussevitch, A., and Carlson, W. (2003). Analysis of Vesicular Basalts and Lava Emplacement Processes for Application as a Paleobarometer/Paleoaltimeter: A Reply. J. Geology. 111, 502–504. doi:10.1086/375278
Schwanghart, W., and Scherler, D. (2020). Divide Mobility Controls Knickpoint Migration on the RoanPlateau (Colorado, USA). Geology 48 (7), 698–702. doi:10.1130/G47054.1
Spicer, R. A., Su, T., Valdes, P. J., Farnsworth, A., Wu, F. X., Shi, G., et al. (2021). The Topographic Evolution of the Tibetan Region as Revealed by Palaeontology. Palaeobiodiversity and Palaeoenvironments 101 (1), 213–243. doi:10.1007/s12549-020-00452-1
Spicer, R. A., Valdes, P. J., Spicer, T. E. V., Craggs, H. J., Srivastava, G., Mehrotra, R. C., et al. (2009). New Developments in CLAMP: Calibration Using Global Gridded Meteorological Data: Palaeogeogr. Palaeocl 283, 91–98. doi:10.1016/j.palaeo.2009.09.009
Steven, T. A., and Ratte, J. C. (1965). Geology and Structural Control of Ore Deposition in the Creede District, San Juan Mountains, Colorado. Professional Paper. U.S. G.P.O. 487, 95. doi:10.3133/pp487
Thompson, R., Anderson, K., Pelltier, R., Strickland, L., Bartlein, P., and Shafer, S. (2012). Quantitative estimationOf Climatic Parameters from Vegetation Data in North America by the Mutual Climatic Range Technique. Quat. Sci. Rev., 51, 18–39. doi:10.3133/pp1650f
VanDeVelde, J. H., and Bowen, G. J. (2014). Isotope Hydrology of Early Paleogene Lake Flagstaff, central Utah: Implications for Cordilleran Evolution. Am. J. Sci. 314, 1436–1461. doi:10.2475/10.2014.02
Walk, C. J., Karlstrom, K. E., Crow, R. S., and Heizler, M. T. (2019). Birth and Evolution of the Virgin River Fluvial System: ∼1 km of post–5 ma Uplift of the Western Colorado Plateau. Geosphere, 15(3), 759–782.
Wilf, P. (1997). When Are Leaves Good Thermometers? A New Case for Leaf Margin Analysis. Palaeobiology, 23, 373–390.
Wing, S. L., Harrington, G. J., Smith, F. A., Bloch, J. I., Boyer, D. M., and Freeman, K. H. (2005). Transient floral Change and Rapid Global Warming at the Paleocene-Eocene Boundary. Science 310, 993–996. doi:10.1126/science.1116913
Winn, C., Karlstrom, K. E., Shuster, D. L., Kelley, S., and Fox, M. (2017). 6 Ma Age of Carving Westernmost Grand Canyon: Reconciling Geologic Data with Combined AFT, (U-Th)/He, and 4He/3He Thermochronologic Data. Earth Planet. Sci. Lett. 474, 257–271. doi:10.1016/j.epsl.2017.06.051
Wolfe, J. A. (1993). A Method of Obtaining Climatic Parameters from Leaf Assemblages. USGS Bull 2040, 1–73. doi:10.1117/12.1438371–73
Wolfe, J. A., (1992a). An Analysis of Present-Day Terrestrial Lapse Rates in the Western Conterminous United States and Their Significance to Paleoaltitudinal Estimates: U.S. Geol. Surv. Bull. 1964, 35. doi:10.3133/b1964
Wolfe, J. A., (1992b). “Climatic, Floristic, and Vegetational Changes Near the Eocene/Oligocene Boundary in North America,”. Late Eocene-Oligocene Climatic and Biotic Evolution. Editors D. R. Prothero, and W. A. Berggren (Princeton, NJ: Princeton University Press), 421–436.
Wolfe, J. A., Forest, C. E., and Molnar, P. (1998). Paleobotanical Evidence of Eocene and Oligocene Paleoaltitudes in Midlatitude Western North America. GSA Bull. 110, 664–678. doi:10.1130/0016-7606(1998)110<0664:PEOEAO>2.3.CO;2
Wolfe, J. A. (1979). Temperature Parameters of Humid to Mesic Forests of Eastern Asia and Relation to Forests of Other Regions in the Northern Hemisphere and Australasia. USGS Bull. 1964, 1–35. doi:10.3133/pp1106
Wolfe, J. A., and Schorn, H. E. (1989). Paleoecologic, Paleoclimatic, and Evolutionary Significance of the Oligocene Creede flora, Colorado. Paleobiology 15, 180–198. doi:10.1017/s0094837300009350
Wolfe, J. A. (1994). Tertiary Climatic Changes at Middle Latitudes of Western North America. Palaeogeogr. Palaeoclimatol. Palaeoecol. 108, 195–205. doi:10.1016/0031-0182(94)90233-X
Young, R. A., and Crow, R. (2014). Paleogene Grand Canyon Incompatible with Tertiary Paleogeography and Stratigraphy. Geosphere 10, 664–679. doi:10.1130/GES00973.1
Young, R. A. (2001). “The Laramide-Paleogene History of the Western Grand Canyon Region: Setting the Stage,”. Colorado River Origin and Evolution. Editors R. A. Young, and E. E. Spamer (Grand Canyon, Arizona: Grand Canyon Association), 7–16.
Young, R. A., and Spamer, E. E. (2001). Colorado River Origin and Evolution. Grand Canyon, Arizona: Grand Canyon Association Monograph 12, 280.
Keywords: Colorado Plateau, paleoaltimetry, clumped isotopes, paleobotany, temperature proxies, Southern Rocky Mountains
Citation: Heitmann EO, Hyland EG, Schoettle-Greene P, Brigham CAP and Huntington KW (2021) Rise of the Colorado Plateau: A Synthesis of Paleoelevation Constraints From the Region and a Path Forward Using Temperature-Based Elevation Proxies. Front. Earth Sci. 9:648605. doi: 10.3389/feart.2021.648605
Received: 01 January 2021; Accepted: 14 May 2021;
Published: 10 June 2021.
Edited by:
Heiko Pingel, University of Potsdam, GermanyReviewed by:
Cari Johnson, The University of Utah, United StatesRichard Ott, German Research Center for Geosciences, Germany
Laura Crossey, University of New Mexico, United States
Copyright © 2021 Heitmann, Hyland, Schoettle-Greene, Brigham and Huntington. This is an open-access article distributed under the terms of the Creative Commons Attribution License (CC BY). The use, distribution or reproduction in other forums is permitted, provided the original author(s) and the copyright owner(s) are credited and that the original publication in this journal is cited, in accordance with accepted academic practice. No use, distribution or reproduction is permitted which does not comply with these terms.
*Correspondence: Emma O. Heitmann, ZW9oMzIyQHV3LmVkdQ==; Katharine W. Huntington, a2F0ZTFAdXcuZWR1