- 1Department of Earth Sciences, University of Geneva, Geneva, Switzerland
- 2Department of Architecture, Built Environment and Construction Engineering, Politecnico di Milano, Milan, Italy
- 3Istituto Nazionale di Geofisica e Vulcanologia, Sezione di Bologna, Bologna, Italy
Understanding the complexity of future volcanic impacts that can be potentially induced by the large variability of volcanic hazards and the multiple dimensions of vulnerability of the increasingly interdependent and interconnected societies, requires an in-depth analysis of past events. A structured and inclusive post-event impact assessment framework is proposed and applied for the evaluation of damage and disruption on critical infrastructures caused by the eruption of the Cordón Caulle volcano (Chile) in 2011–2012. This framework is built on the forensic analysis of disasters combined with the techniques of the root cause analysis that converge in a bow-tie tool. It consists of a fault tree connected to subsequent event trees to describe the causal order of impacts. Considering the physical and systemic dimensions of vulnerability, four orders of impact have been identified: i) the first order refers to the physical damage or the primary impact on a component of the critical infrastructure; ii) the second order refers to the loss of functionality in the system due to a physical damage on key components of the system; iii) the third order refers to the systemic impact due to the interdependency and connectivity among different critical infrastructures; and iv) a higher order is related to the consequences on the main economic sectors and to social disruption that can activate an overall damage to the economy of the country or countries affected. Our study in the Argentinian Patagonia shows that the long-lasting impact of the 2011–2012 Cordón Caulle eruption is mostly due to a secondary hazard (i.e., wind remobilisation of ash), which exacerbated the primary impact affecting significantly larger areas and for longer time with respect to primary tephra deposition. In addition, systemic vulnerability, particularly the intrinsic dependencies within and among systems, played a major role in the cascading impact of the analysed communities.
1 Introduction
The increasing complexity of modern societies makes volcanic eruptions more impactful on several sectors at multiple spatial and time scales, requiring a shift in how we understand and manage natural risk. The recent eruptions of Eyjafjallajökull, Iceland (2010) (Oxford Economics, 2010; Scaini et al., 2014) and of Cordón Caulle, Chile (2011–2012) (Craig et al., 2016a, Craig et al., 2016b; Elissondo et al., 2016) demonstrated that our societies face high risk from even small to moderate size volcanic eruptions, not only due to an increase in exposed and vulnerable systems, but also to a more globalised and infrastructure-dependent world. Investigations on risk assessment strategies have shown how complexities due to multi-hazard scenarios and compounded vulnerabilities require an integrative approach considering the complexity of cascading impacts that can be unravelled by investigating the connections among systems within a system-of-systems approach (Bonadonna et al., 2009; Sapountzaki et al., 2009; Eusgeld et al., 2011; Menoni et al., 2012; Menoni et al., 2017). In fact, in the last 50 years, the fundamental concept of risk has evolved from a natural hazard perspective towards a risk-informed sustainable development, through the inclusion of exposure and social components of risk as well as the physical and systemic dimensions of vulnerability (UNDRR, 2019).
We selected the 2011–2012 eruption of the Cordón Caulle (CC) volcano (Chile) as a case study, as it represents the perfect example to investigate long-lasting and cascading impacts, over years after the end of the eruption, in communities highly dependent on critical infrastructures. Here with long-lasting impact we refer to the impact associated with tephra fallout and ash remobilisation that can last several years up to a decade throughout the paper. The 2011–2012 CC eruption was a rhyolitic eruption classified as small-moderate size to sub-Plinian that generated a widespread tephra-fallout deposit covering more than 100,000 km2 towards the Atlantic Ocean in Argentina (Pistolesi et al., 2015; Bonadonna et al., 2015). The eruption lasted from the 4 June 2011 to December 2012 (OVDAS, 2012) and generated significant impacts, due to tephra fall out and subsequent remobilisation of ash, in the short, medium and long term in all sectors mainly in the provinces of Río Negro, Neuquén and Chubut (Argentina, Figure 1A). Although most of the volume of the tephra-fallout deposit was produced during less than two days (climactic phase of 4–5 June 2011 lasted 24–30 h), the remobilisation of ash by water (i.e., lahars) and by wind maximised and exacerbated the impacts on the affected localities for long periods, not only due to the socio-economic repercussions and previous vulnerabilities (most of the communities are geographically isolated), but also to the longevity of wind-remobilisation of ash (Wilson et al., 2013; Craig et al., 2016a, Craig et al., 2016b; Elissondo et al., 2016; Forte et al., 2018; Dominguez et al., 2020a). About 462 million USD of economic losses were calculated in relation to the eruption (Elissondo et al., 2016); but the losses associated with the long term consequences, particularly in the power, telecommunications and water systems as well as the cost of cleaning processes and the impact on all economic sectors, are harder to determine.
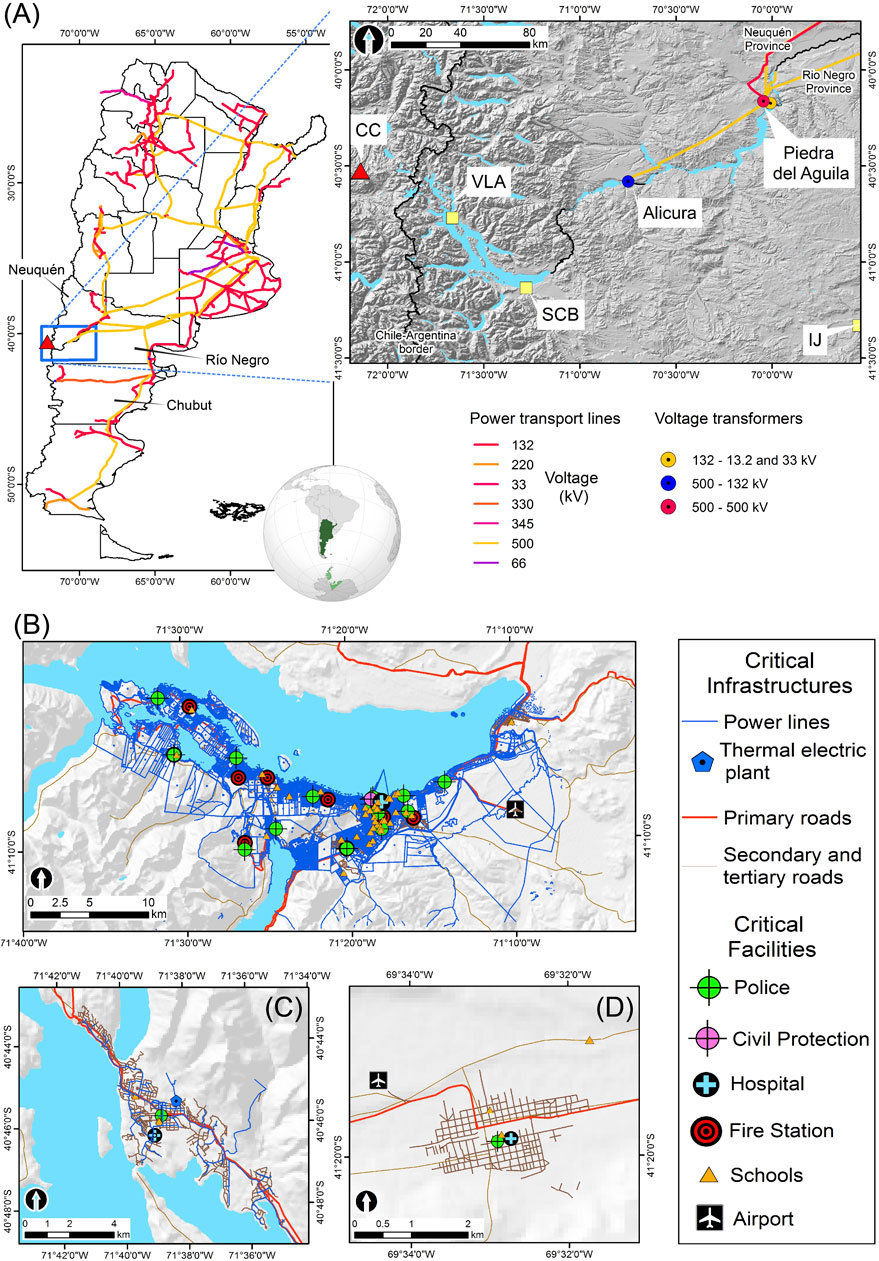
FIGURE 1. (A) Location map and characteristics of the power system of Argentina and of the target localities of this study (San Carlos de Bariloche (SCB), Villa La Angostura (VLA), and Ingeniero Jacobacci (IJ) indicated by the yellow squares). CC indicates the Cordón Caulle volcano (red triangle). Power transport lines connected to the National System, transformers and nodes (Alicura, Piedra del Aguila) are shown (orange, blue and red circles). Critical infrastructures (CI) and facilities (CF) for (B) SCB, (C) VLA, and (D) IJ.
Post-event impact assessments on critical infrastructure systems (e.g., power, water, transportation, telecommunication systems) have demonstrated that impacts are usually amplified by a sequence of events associated with dynamic and multiple dimensions of vulnerability (i.e., physical, systemic, economic, social) which evolve and overlap over time (Rinaldi et al., 2001; Rinaldi, 2004; Eusgeld et al., 2011). Impacts are thus amplified not only by the intensity of the hazard and by the level of vulnerabilities, but also by the interactions of the hazard with vulnerability and response, driving to complex cascading effects (also known as ripple effects or domino effects, Gasparini and Garcia-Aristizabal, 2014; Pescaroli and Alexander, 2015; Menoni et al., 2017; Zuccaro et al., 2018) in other systems or subsystems that result in functional, social or economic disruptions (Pescaroli and Alexander, 2015). The significant interconnections and interdependencies among systems, which trigger a chain of cascading effects, are not easy to disentangle (Rinaldi et al., 2001; Rinaldi, 2004; Rose, 2004; Pescaroli and Alexander, 2015). Therefore, identifying the role of each risk component (e.g., hazard type and intensity, vulnerability dimensions and capacity to respond) on the generated impacts, and associated cascading effects, is fundamental in order to prioritise mitigation actions and strengthen the response of the exposed communities. This is particularly challenging in the case of volcanic eruptions that involve multiple primary and secondary hazards (e.g., tephra fallout, pyroclastic density currents, lahars, wind remobilisation of ash) combined with multiple dimensions of vulnerability (e.g., physical, systemic, social, economic) in increasingly growing and interconnected societies in volcanic areas.
This research proposes a new post-event impact assessment framework based on the forensic analysis of disasters in order to disentangle the relations between volcanic eruptions and human systems. The forensic analysis represents a very effective approach to identify root causes and understand complex interactions, largely applied in engineering and judicial purposes, crisis emergency management, incident and industrial risk assessment, and most recently to natural disasters (Mendoza, 2019). Within a natural disaster context, the forensic investigation approach aims at building resilience and reducing risk through learning from past experiences (Burton, 2010). The FORensic INvestigation of Disasters program (FORIN) was then developed to answer the what, who, when, how and why the different impacts occur, through a systematic and integrative analysis of the main drivers, or the so-called, root causes of impacts, from a multi-disciplinary perspective (IRDR, 2011). Another framework for post-impact analysis, the Post-Event Review Capability (PERC) is based on a synergistic effort bringing together governments and stakeholders from various sectors (i.e., academia, private companies and public sectors) to dynamically analyse the different stages of the risk management cycle in a short and long term (i.e., preparedness, response, recovery, and mitigation) (Venkateswaran et al., 2015).
Even though FORIN represents the cornerstone of forensic investigation of disasters, most of the applications were not deepened on the technical aspects of the root cause analysis such as the identification and quantification of key vulnerability aspects Mendoza (2019). From an engineering perspective, this analysis can be conducted through the material reliability (Schultz et al., 2010) and industrial incident analysis (Stamatelatos and Vesely, 2002; IAEA, 2015). Root causes techniques (in particular logical tools) have been widely applied to the analysis of failure of critical components, which are of particular relevance to investigate the failure of critical infrastructures (Livingston and Jackson, 2001). One interesting EU-funded project applied to natural hazards is the SYNER-G framework (Systemic Seismic Vulnerability and Risk Analysis for Buildings, Lifeline Networks and Infrastructures Safety Gain, 2009–2012) that provides insights into how to describe and quantify cascading effects due to seismic hazard accounting for systemic vulnerability through several methods (e.g., logical tools, network analysis) (Pitilakis et al., 2011).
Forensic Investigation methods have been applied in volcanology only to a very limited extent. Pioneering studies have been conducted by the STREVA project (Strengthening resilience in volcanic areas, 2012–2019). Most of forensic STREVA outputs are social-oriented analysis of various case studies (e.g., Armijos and Few, 2017; Armijos et al., 2017; Few et al., 2017; Hicks and Few, 2015; Pyle et al., 2018; Barclay et al., 2019) with the exception of the study of Sword-Daniels et al. (2015) that provides an interesting relational analysis for the health care system and associated essential services affected by the recent Soufrière Hills eruption (1995–2015) on Montserrat Island (United Kingdom, West Indies). A comprehensive application of forensic analysis of the short and long-term cascading effects associated with the 1999 Mount Cameroon eruption (Cameroon) has been conducted by Wantim et al. (2018). This study clearly classifies direct (physical damage) and indirect (systemic and long-term) impacts that become more evident over time, highlighting that enchained effects were more severe than the immediate consequences of the eruption.
Even though volcanic risk approaches have evolved with time and important international projects have focused on different components of risk (including systemic and social vulnerabilities, e.g., ENSURE, 2008–2011; DEVORA, 2008–2015; STREVA, 2012–2019), no risk framework exists that encompasses the complexity of cascading impacts associated with volcanic eruptions. Based on a coupled approach including the fundamental concepts of the FORIN (IRDR, 2011) and PERC (Venkateswaran et al., 2015) methodologies, combined with the most innovative techniques used in reliability and incident analysis (e.g., Livingston and Jackson, 2001; Andersen and Fagerhaug, 2006; Sutton, 2015) and more recently in multi-risk analysis (e.g., Garcia-Aristizabal et al., 2017; Garcia-Aristizabal et al., 2019), the conceptual post-event impact assessment framework proposed in this study seeks to contribute on the understanding and quantification of cascading impacts associated with volcanic eruptions.
The CC eruption represents an exceptional opportunity to demonstrate the capabilities of the proposed approach to i) analyse the cascading effects associated with the ground accumulation of tephra and wind-remobilisation of ash, ii) identify priorities of intervention and the key aspects contributing to risk reduction, and iii) recommend a damage collection template. A comprehensive analysis of impacts on critical infrastructures (CI), critical services (CS), and critical facilities (CF) with particular emphasis on the power system of the three of the most affected localities in Argentina: Villa La Angostura (VLA) in the province of Neuquén, San Carlos de Bariloche (SCB) and Ingeniero Jacobacci (IJ) in the province of Río Negro is presented (Figure 1). The variable physical characteristics of the primary tephra-fallout deposit (Bonadonna et al., 2015; Pistolesi et al., 2015) combined with a climate gradient from the Andes Range to the Patagonia steppe resulted in a variety of environmental scenarios with different hazard intensities. Whilst the humid and vegetated Andes areas, where VLA is located, were impacted by a thick coarse-ash-rich tephra-fallout deposit, the arid and windy steppe areas, where IJ is located, were impacted by a thin fine-ash-rich tephra-fallout deposit (Dominguez et al., 2020b). Outcomes associated with different combinations of environmental conditions and tephra-fallout deposit characteristics are described in detail. The manuscript is organised as follows: the methodology associated with the proposed impact assessment framework and data requirements are outlined in the Methods. Impact data and results of the application of the framework are presented in the Results. The main findings, applicability and limitations are discussed in the Discussion. Conclusions are presented in the Conclusion.
2 Methods
2.1 Proposed Conceptual Framework for an Integrative Impact Assessment
Since impact assessment frameworks that encompass the interactions of cascading effects associated with volcanic phenomena are still very limited (e.g., Marzocchi et al., 1995), we propose here a new integrative framework that relies on a series of logical steps summarized in Figure 2 and detailed as follows:
2.1.1 Step I–Definition of Objectives, Sectors, and Relationships
The initial phase of the study consists in clearly defining the objectives, sectors and the level of detail (resolution) at which the analysis can be conducted. Subsequently, the identification of the connections and the degree of dependency among different systems of each analyzed sector need to be established. In practice, even without a physical connection between certain components of systems, consequences in one system may affect another one, especially in the case of CI, CF, and CS. Here we distinguish between dependencies among elements of the same system (intra-dependency), or dependencies among different systems (inter-dependency). Depending on the level of knowledge and available data, categories of dependency could be also identified (i.e., physical reliance, cyber transfer, geographic proximity, logical relations, policy and societal dependencies; Rinaldi et al., 2001; Dudenhoeffer et al., 2006). The use of relational diagrams (Rinaldi et al., 2001; Sword-Daniels et al., 2015; Wild et al., 2019) or dependency matrices (Pitilakis et al., 2014) provide a global overview of connections and are very helpful to identify the criticality level among the different analysed systems.
2.1.2 Step II–Find the Evidence of Impact
The basis of the forensic investigation is to find the evidences required to answer questions concerning the what, who, when, how, and why the different impacts have occurred. To do this, it is necessary to identify and locate the impact in time and space as well as to determine all the possible pieces of evidence that connect the impact on an exposed element to a given hazardous event (e.g., tephra fallout, ash remobilisation) and its intensity.
2.1.3 Step III–Structure and Quantify
The core of this framework is to structure and quantify all the possible causes and subsequent consequences (cascading effects) associated with each impact. We introduce a classification of impacts in four causal orders (Table 1): a first order refers to the physical damage, a second order refers to the loss of functionality, a third order is associated with the systemic impact among CI and other CF (e.g., hospitals), and a higher order is associated with the impact on individual economic sectors and on the overall economy as well as on the social sector. This sequence of impacts is inherently related to different temporal (quantified here as duration) and spatial (quantified here as % of area affected) scales. The physical damage on a system component usually induces local scale impacts and a short duration (seconds-days). However, complications due to the degree of response (e.g., speed of detection, accessibility, repairing resources) and the systemic vulnerability factors (e.g., connectivity, redundancy, transferability) will induce the loss of functionality of the system or part of the system, potentially increasing the duration of the impact (up to months). The level of interaction and dependency among CI added to the possibility of transferring the functions or not will induce, in turn, a systemic impact, potentially increasing the size of the affected area and the duration (hours-years). Finally, a higher order of impact due to the level of dependency of the economic and social sectors on CI will trigger broader and longer consequences (local-to-national, months-to-years) (Table 1).
However, the sequence of impacts associated with natural hazards is not linear. There is often an overlapping among several systems impacted at the same time. The multiple sources of causes can be related to various vulnerability dimensions, variable hazard conditions or different degree of response; and the drivers of impact remain, therefore, unclear. As a consequence, we need to structure the sequence of consequences and to clearly discretise each impact to better understand its root causes. We can unravel these complex relations through the use of root cause tools broadly used in reliability and incident analysis. There is a large variability of logical tools; a detailed description and examples of these tools are presented in the Electronic Supplementary Material (Supplementary Figure S1). Here we focus on the use of fishbones, or diagrams of cause and effects, fault and event trees. The fishbone consists of branches connected to a central spine, in order to classify thet causes by category (e.g., hazard-related, vulnerability-related, etc.) associated with a given effect. The fault and event trees are analytical tools constructed by a deductive and an inductive approach, respectively. Deductive indicates a backward thinking exploring all the possible causes of a failure. Inductive refers to a forward thinking to dig all the possible pathways or scenarios triggered by a given event. These logical tools can be connected in what it is known as the bow-tie structure where the top event of a fault tree is connected to the trigger event of an event tree (Ruijter and Guldenmund, 2015). Figure 3 shows the proposed bow-tie structure to link the different orders of impact of this framework. This structure consists of a fault tree describing the physical damage (i.e., first order of impact), and subsequent event trees describing the loss of functionality and systemic impact (i.e., second and third order of impact). The top event of the fault tree will serve as the trigger event of the second impact order (event tree-2nd), and subsequently, each resulting scenario from this event tree will serve as a trigger event of a new event tree describing a third impact order (event tree-3rd) (Figure 3). The level of detail of this analysis strongly depends on the impact assessment objectives and the quality and accuracy of data that can be obtained for each component. Qualitative or semi-quantitative assessments allow for a better understanding of the intrinsic processes and the efficiency of response actions as well as to identify the lessons learnt to be better prepared for the next volcanic event. Quantitative assessments of the fault and event trees could allow to the numerical estimation of the probability of physical damage, the conditional probability of dysfunction given a physical damage, and the conditional probability of systemic impact given a dysfunction (Figure 3).
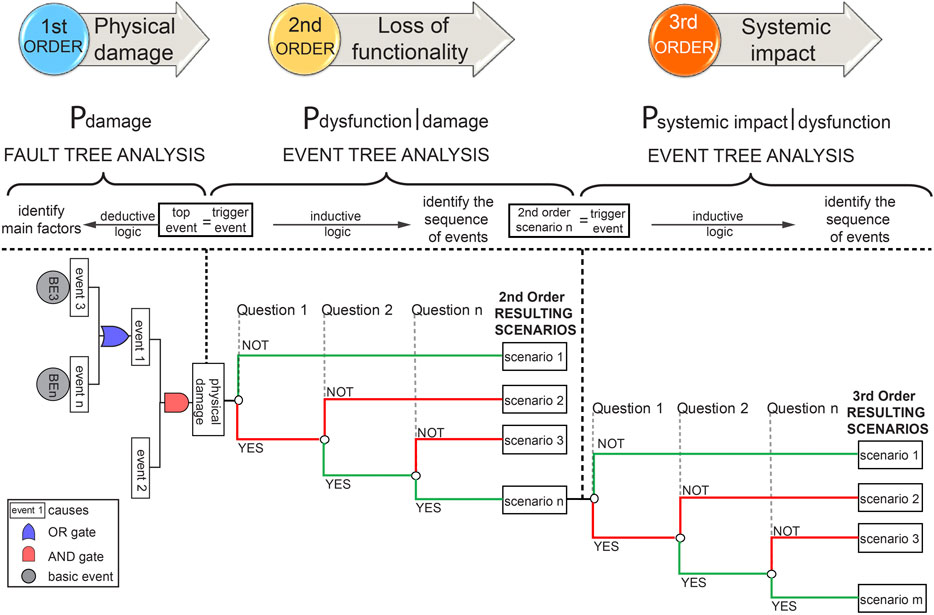
FIGURE 3. Theoretical bow-tie structure of this framework connecting first, second and third order of impacts. First order of impact is described by a fault tree whose top event serves as trigger event of an event tree describing the second order of impact. Each of the resulting scenarios of this event tree represents the trigger event of subsequent event trees describing the third order of impact.
2.1.4 Step IV–Identify Critical Points
The final goal of this comprehensive impact assessment framework is to identify the critical points where mitigation measures should be implemented in order to reduce vulnerability, and to establish response priorities in case of future volcanic events. The first attempt to decrease the vulnerability is often associated with actions on the physical damage causes; however, systemic relations between CI usually cause major consequences on the cascading sequence. The path of these interactions is often not linear. Therefore, a clear and structured impact analysis allows to detect the role of each risk component (i.e., hazard type and intensity, type of vulnerability, degree of response) on the induced impact and, in this way, develop effective strategies to reduce both physical and systemic vulnerabilities, and increase the resilience of CI.
2.2 Data Acquisition, Source and Treatment
Two field surveys in Argentina were conducted in February and December 2016 in order to gather all the relevant data concerning impact on CI and their relation with CS, CF, economic and social sectors (Table 2) in the three localities (i.e., VLA, SCB, IJ, Figure 1).
Qualitative and quantitative data were gathered from various sources (Table 3). Three main techniques of data acquisition were applied in this study: text-analysis (e.g., newspapers, technical reports, and bulletins), semi-structured in-person interviews and direct field observations (Table 3). Since this study is focused on critical infrastructures, the interviews were undertaken with several members of the Emergency Operations Centre (known in Argentina as COE), such as Civil Protection members of the 3 localities, municipalities practitioners (e.g., the mayor of IJ, urban planning engineers of IJ and SCB), CI, CS, and CF managers (e.g., director and specific engineers and technicians of the Cooperative of Electricity of Bariloche and Companies of Electricity of VLA and IJ), firemen and cooperative members (e.g., livestock cooperative of IJ). Indeed, cooperatives are key organisations in the social economy of Argentina, where people associate with their own resources to organize and provide services (e.g., electricity supply, livestock trade) (Rofman, 2010). In particular, cooperatives were fundamental in the coordination of actions in the affected communities during and after the CC volcanic crisis (Murgida et al., 2016). The complete lists of all impact data resources and interviewees are available in the Supplementary Tables S1,S2.
In-person interviews, that lasted between 20 min and 2 h, were recorded and transcribed. The questions covered five main sections as described below:
• General aspects of the system including the physical description before the eruption, as well as the relations with other sectors and country-structure dependencies (e.g., Federal system of Argentina where functions, tasks and protocols are strongly dependent on the municipality, province, or national policies).
• Description and location of impacts including the geographic location and/or the parts of the system affected (e.g., total or partial), the type of impact (i.e., physical damage or disruptions due to external factors) and all the possible causes (i.e., directly related to contact with tephra or due to dependencies on other CI or other factors).
• Technical aspects including the duration and frequency of disruption (intermittent or continuous), the number of disruptions, the number of people affected, the number of components, and/or elements affected.
• Response including all the factors relevant to the actions taken during the crisis, the strategies adopted to mitigate the impacts and the prevention measures (closure or disruptions due to cleaning, prevention, or maintenance).
• Current design of the system describing the modifications or improvements implemented since the eruption.
In order to analyze in a comprehensive way all the relevant aspects involved in the direct impacts and the cascading effects, data was classified in 11 themes including type of impact, impact intensity, starting and end time of impact, duration, number of users affected and/or area affected, causes, cascading effects, hazard intensity, response, and the corresponding references. This inventory includes the impacts occurred immediately after the beginning of the eruption (4 June 2011) as well as the chronology of impacts until the end of the eruption (December 2012) (Wilson et al., 2013; Craig et al., 2016a; Elissondo et al., 2016) and during the subsequent years when wind remobilisation of ash caused disruptions (Forte et al., 2018). Representative data from this inventory is described in the following section, and the complete associated dataset is available at https://doi.org/10.26037/yareta:xohh6yvjwbg5rkwb5m7mq6ll5u.
3 Results
3.1 Preliminary Assessment of Impacts due to Tephra Fallout and Ash Remobilisation by Wind
The analysis of the impacts and associated cascading effects of the long-lasting CC eruption highlighted some important aspects. First, the long-lasting consequences, associated with secondary hazards such as the remobilisation of ash by wind exacerbate the impacts associated with the primary hazard (e.g., tephra fallout), to unpredictable timescales and to vast areas, much larger than the areas affected by primary deposition. Second, the impacts were mostly disruptions related to the loss of functionality of the various CIs and CFs, involving physical but mainly systemic vulnerabilities. In fact, it was observed that major disruptions were intrinsic to intra- and inter-dependencies after the occurrence of a physical failure of a given component. As also observed by (Craig et al., 2016a), most systems could recover thanks to the removal of ash or to immediate interventions (e.g., transfer of functions, rapid repairing), avoiding long-term or permanent disruptions. Impacts affected all sectors (Table 2) including CI (e.g., power outages, closure of roads), CS and CF (e.g., disruption of civil protection services, loss of functionality of hospitals, and schools), economic sector (e.g., agriculture and commercial losses), and social sectors (e.g., residents’ isolation).
Figure 4 shows a summary of reported impacts from 2011 to 2013 on CI (i.e., road disruption, power and water supply) and CF (i.e., school and hospital closure) for the three target localities, VLA, SCB, and IJ. Whilst impacts reported in IJ occurred from June 2011 to December 2013, in VLA, they occurred from June 2011 to March 2012, and only during June 2011 in SCB. Although it is difficult to recognise whether impacts during the first 6–7 months after the beginning of the eruption are due to primary tephra fallout or remobilised ash by wind or both, we can conclude that impacts after January 2013 are exclusively associated with ash-remobilisation events since the end of the eruption occurred in December 2012 (OVDAS, 2012). In fact, from July 2011 to April 2012, only sporadic and small plumes produced small volumes of tephra that mostly sedimented in the proximal areas of the volcano (i.e., within the Chile-Argentina border) (Pistolesi et al., 2015; Elissondo et al., 2016). Even though IJ received less tephra in comparison with VLA (primary tephra deposit thickness of 5–7 and 15–17 cm, respectively, Pistolesi et al. (2015)), the fine ash, that was mostly produced during the weak plumes that followed the climactic phase, combined with arid conditions and strong winds on the Patagonia steppe, generated significant remobilisation events for years after the beginning of the eruption (up to the time of writing, 2020). From the interviews, we concluded that most impacts in IJ are strongly related to wind remobilisation events during and after the climactic phase of the eruption. These impacts were related to the closure of roads due to the low visibility, which in turn provoked disruptions in the main activities (e.g., schools’ closure) because of the difficulty to access the sites (Figure 4). It is important to notice that the Patagonia is indeed a very isolated region with long unpaved roads and tertiary paths where a good visibility is required. Another significant source of impacts during the years after the eruption have been recorded in the power supply system of IJ, mainly due to the accumulation of fine ash in the switches producing either a high conductivity (in contact with water) or abrasion of the electrodes reducing their normal contact. However, despite being frequently mentioned in the interviews, the impacts from 2014 have not been systematically recorded and hence cannot be shown in the Figure 4. In the case of SCB, impacts are concentrated in the period of June–July 2011 associated with a thinner deposit than the other two localities (2 cm of coarse ash; Pistolesi et al. (2015)). In fact, the associated volcanic plumes were highly dynamic due to fluctuating and strong winds as well as changes on the eruptive behaviour; and, as a consequence, SCB only received intermittent events of sedimentation of small volume of ash (Bonadonna et al., 2015). Conversely, VLA was characterized by a relatively thick tephra-fallout deposit (15–17 cm). Water supply disruptions were very significant in VLA, since the main source of potable water is the lake Nahuel Huapi, which was covered by lapilli and coarse ash. Subaerial water pumps were clogged and the accessibility was complicated due to the amount of tephra in the lake. The road system was strongly affected due to the large amount of tephra accumulated together with snow, as the eruption occurred in the middle of the austral winter. Concerning the power supply disruptions, the three localities were largely affected due to the contamination of ash on various components of the electrical system (e.g., insulators, transformers, switches).
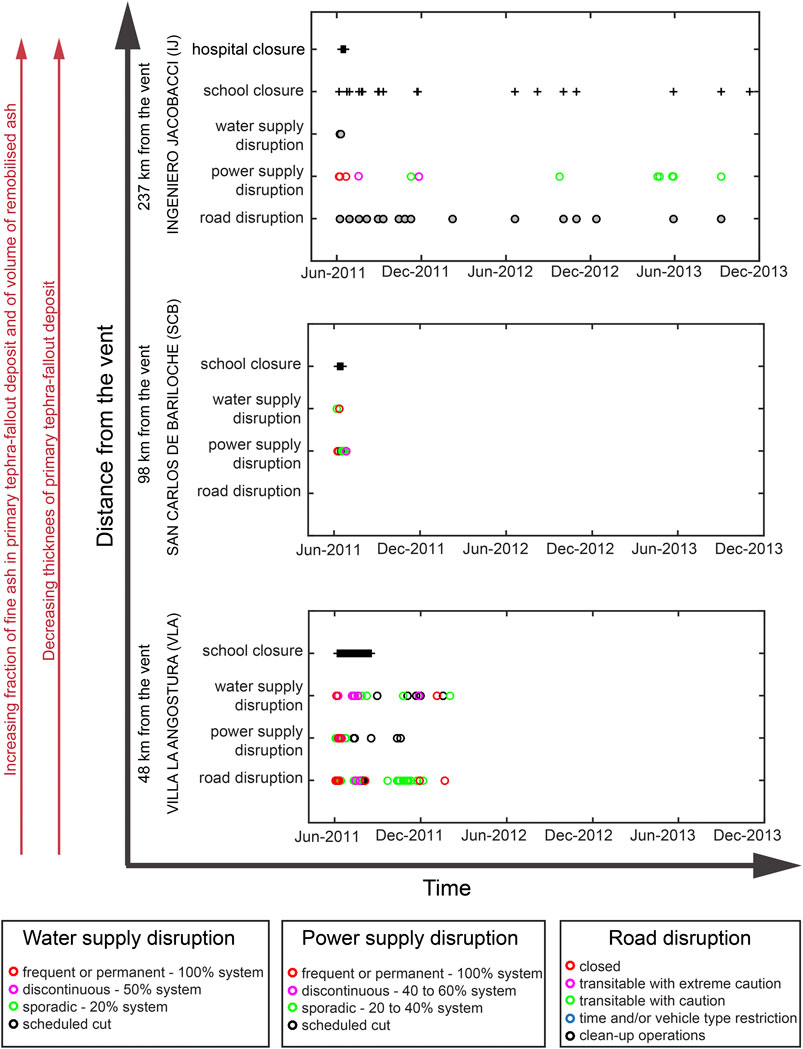
FIGURE 4. Detailed impact analysis for the three target localities analyzed in this study, VLA, SCB, and IJ. Coloured scales associated with the severity of the impact in terms of frequency and percentage of affected area (water and power supply disruption); and in terms of restrictions (road disruption). Grey circles and black crosses indicate report of disruption but no information about the duration, frequency or area affected by the impact. No data about road disruption in SCB was available. Space and time axis are indicative and not to scale.
Although not systematically, impacts on CI and CF, particularly related with the power and water supply disruptions, are reported in technical reports both in terms of frequency (e.g., frequent or highly frequent disruptions; discontinuous or short disruptions; and sporadic referring to rare disruptions), and in terms of area affected (e.g., a neighbourhood, part or the whole locality). For roads disruption, terms such as “transitable with caution” and “restrictions on time or type of vehicles”, are reported (Figure 4). Since impacts on CI and CF involve a complex interaction of various factors including systemic relations related to the dependency and connectivity of systems, transferability of functions, degree and quality of response and secondary hazards, we still need to better understand and structure the impacts in such a way that their causes as well as the possible mitigation measures can be identified. The next section is, therefore, dedicated to the application of the post-event impact assessment framework on the CC case study with particular emphasis in the power system, following the 4-steps methodology proposed in the Preliminary Assessment of Impacts due to Tephra Fallout and Ash Remobilisation by Wind (Table 1, Figures 2, 3).
3.2 Integrative Impact Assessment of the Power System at Local Scale
3.2.1 Step I: Identifying Connections and Dependencies
Based on a comprehensive analysis of the information gathered from the interviews, technical reports and scientific papers, a more in-depth analysis has been conducted for all the analyzed sectors of SCB and is represented as a dependency matrix (Figure 5). A simplified qualitative dependency index based on four degrees of dependency has been defined. This index is associated with two colour scales in order to distinguish the intra-dependencies between the same system (in blue); and the inter-dependencies between different systems (in green). Four colour tones for both green and blue scales are associated with the four degrees of dependency, and a score was assigned based on an uniform distribution, as follows:
• none – no dependency – pale colour – score value 0.
• low dependency – not relevant issues if missing – light colour – score value 0.33.
• medium dependency – dependent but can continue functioning – bright colour – score value 0.66.
• high dependency – totally dependent and cannot continue functioning – dark colour – score value 1.
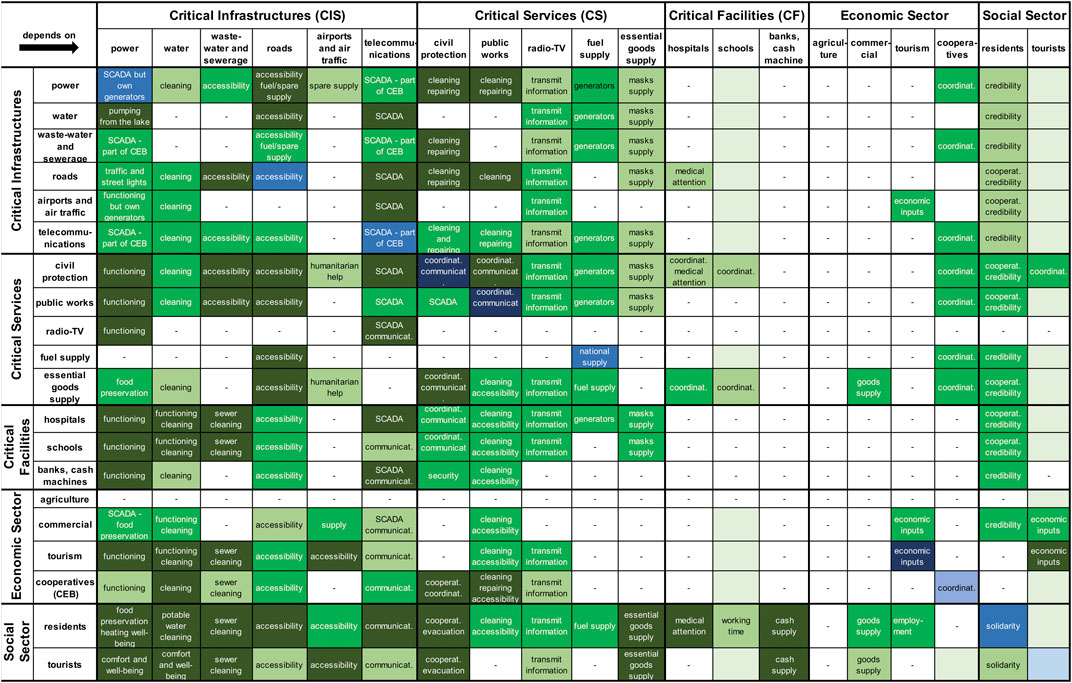
FIGURE 5. Matrix showing the relations of dependency among different sectors in SCB. Colour scales indicate the dependency index where intra-dependencies beetween the same system (blue scale) and inter-dependencies beetween different systems (green scale) have been analysed. Each of these colour scales consists of four degrees of dependency from none–no direct or indirect dependencies (pale color), through low dependency–not relevant issues if missing (light color), medium dependency–dependent but can continue functioning (bright color), to high dependency–totally dependent and cannot continue functioning (dark color). White cells with hyphen indicate no available data. Cooperatives refer only to the Cooperative of Electricity of Bariloche (CEB). SCADA refers to the Supervisory Control And Data Acquisition. (For a better readibility, these colour scales are available in the Supplementary Figure S2).
The most relevant aspects resulting from this analysis in SCB involved the role of cooperatives, the importance of accessibility to the damaged components, the nature of water source, the preparedness of the community and the importance of being informed. Cooperatives are crucial socio-economic organisations in Argentina that provide services to the communities with solidarity principles (Rofman, 2010; Murgida et al., 2016). In the case of SCB, the Cooperative of Electricity of Bariloche manages the power supply, telecommunications, wastewater treatment and sewerage system. Consequently, even when there is a high dependency, the centralised coordination and communication among these systems were much more effective than in other localities, allowing these systems to continue working during most of disruptions (Figure 5). However, the power system was fully dependent on the coordination with public works and Civil Protection for the rapid cleaning of insulators and system components as well as the opening of roads to get access to the whole system for repairing and also to get fuel and spare parts supply (Figure 5). SCB has indeed an elongated urban fabric enclosed between the mountains (pre-Andes) and the lake Nahuel Huapi with a grid-type road system where the accessibility in normal times (not crisis) is quite complicated (Figure 1C). During the first month of the CC eruption, accessibility was exacerbated due to the amount of tephra accumulated on the roads and sidewalks. In addition, the sewer lines and storm water drains, which are parallel to the roads, get clogged due to the illegal connections to the sewerage system (Craig et al., 2016a), and this caused major accumulations on the roads, highlighting the inter-dependency between the two systems (Figure 5). On the other hand, the source of potable water of SCB comes from the lake Nahuel Huapi, some springs and streams (Craig et al., 2016a). One of the main priorities of the power system was to maintain the energy or reduce the duration of outages in the water intakes and plant treatment because pumping components are fully dependent on the power system. Conversely, a large amount of water was necessary for cleaning power components.
Preparedness is a key aspect for coordinated response. Despite of certain levels of awareness already existing at the time of the CC eruption, the different stakeholders of SCB had no protocol to manage a volcanic crisis (Dominguez et al., 2020a). The long-lasting CC eruption highlighted the high dependency of all sectors on the Civil Protection and, moreover, on an efficient coordination among all systems (Figure 5). In addition, SCB is the largest city of the Argentinian Andes and it is well modernized compared to its neighbours (e.g., VLA, towns on the steppe region of Rio Negro). Most information to the population goes through social networks and internet. Consequently, communities were fully dependent on the power and telecommunications supply, and, since these systems were significantly disrupted, communication through the local radio channels played a major role. In fact, for some isolated communities, a battery-operated radio was the only means of communication to receive instructions.
The dependency matrix of Figure 5 represents the complex relations across sectors in SCB. These matrices are very useful to identify the “most” critical system (or the system that is more necessary), and the “most” dependent system by assigning the scores based on a uniform distribution from 0 to 1 to each cell of the Figure 5. The sum of resulting scores of the matrix columns provide a priority list of the systems that are most needed or indispensable; and the scores in the rows provide a sorted list from the most to least dependent systems (the complementary matrix showing the computed scores is available in the Supplementary Figure S3). Resulting scores, summarized in the Table 4, show that the power system, roads, telecommunications, Civil Protection, water and public works are the most indispensable systems in descending order; and residents, Civil Protection, tourists, public works and hospitals are the most dependent systems. It is very important to notice here that there are systems considerably less known than others because of the lack of information and this can lead to biased interpretations. Nevertheless, combined results from the analysis of Figures 4, 5 suggest that major impacts, especially in proximal areas to the volcano (i.e., VLA and SCB) were associated with the power system not only because its intrinsic impacts but mainly because of the large effects on all sectors due to the multiple connections with other systems. As an example, a simplified relational diagram showing an overview of these connections is available in the Supplementary Figure S4.
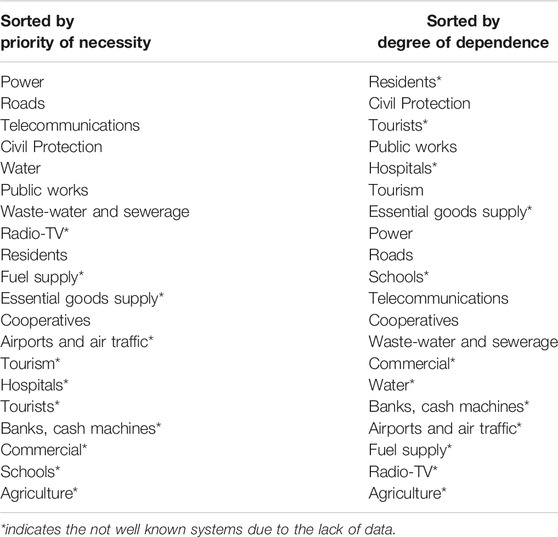
TABLE 4. Degree of dependence and necessity across systems. Lists sorted from most to least critical (left column) and dependent (right column).
3.2.2 Step II: Finding Evidence of Impact on the Power System
The national electric system of Argentina is constituted by a common network that distributes the energy across the country, which is still under development (InfoLEG, 2015). As a result, many localities, mostly in the south of the country, are not yet connected to the national system; this is the case of VLA for instance, which has its own thermal plant based on fuel generators. In contrary, SCB and IJ are connected to the national system through the hydroelectric plant of Alicura (Figure 1A). During the first days of the eruption, the sedimentation of tephra combined with rain generated damages to various components of the electrical system, mostly in VLA and SCB. Failures were associated with flashover of insulators, fuses or damages in other system elements due to the high conductivity of the tephra in wet conditions. Conversely, there was no rain in IJ during these days, and strong winds rather contributed to clean the insulators, considerably diminishing this type of damage in this area. The major issue in IJ was related to the clogging of switches with very fine ash that obstructed the contact of electrodes or, due to the contact of ash with low humidity, became conductive and burned the switches. These impacts were still ongoing in 2020 in the area of Ingeniero Jacobacci in Patagonia. Another type of damage, recorded in the three localities, was the clogging of air intakes and filters as well as abrasion of all components. Disruptions of the power supply triggered in turn cascading effects, or aggravated the impacts on other CI, such as in the road system due to the low visibility or on the water plumbing system due to the lack of energy to make the pumps work, among others (see Figure 5 and Supplementary Figure S4 for more details).
Based on the power outages recorded by the electricity companies of SCB and IJ, the summary of outages is presented in Figure 6. For the case of IJ, the data log included weather conditions (i.e., good, rainy, cloudy, stormy, windy), even before the eruption, since this is an area continuously affected by extreme meteorological conditions. During the eruption, the factor “ash” was then incorporated in the outage data log by the technicians. However, only the major events affecting the whole system, or the events due to severe and long ash storms, were recorded as “ash-related” causes (red crosses in the Figure 6A). These damages are mainly caused by the flashover of elements (insulators or fuses) due to high conductivity of wet ash, whose detection was quite difficult and long due to the lack of accessibility to the affected site because of the almost null visibility in the roads (ash suspension); or by a large failure of the power plant that generated a blackout both in IJ and SCB (June 7, 2011). From this analysis, it was found that failures mostly due to the flashover of elements often occur during the winter, most likely associated with wet ash (red crosses, Figure 6A); and, conversely, failures due to clogging of switches occur in summer (purple triangles, Figure 6A). In fact, from interviews, it was found that most of failures in IJ were associated with damages of switches due to ash contamination, therefore, although not specified in the data log, many of the events catalogued as “damages of switches” (purple triangles in Figure 6A) might be associated with ash-related causes (personal communication technicians of the Company of Electricity of Río Negro - EDERSA). As a comparison, failures before the eruption are also plotted to show that the frequency of damages of switches considerably increased after the eruption (Figure 6A). Outages associated with this type of failure have a duration between 4 min and 2.5 h, and usually affect only one or few users. Based on laser diffraction analysis at the University of Geneva, we found that the ash embedded in the switches has a mean grainsize of 49 μm (range between
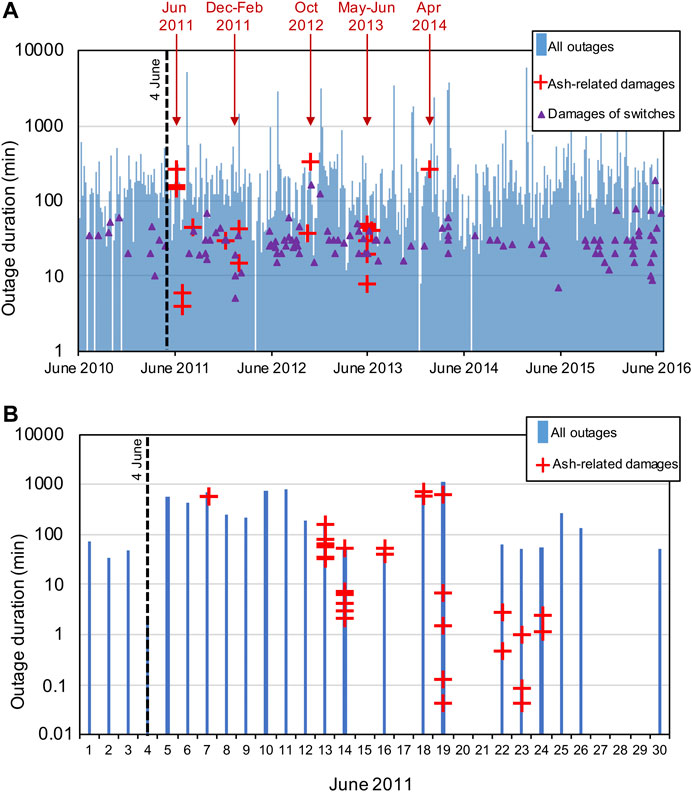
FIGURE 6. Power outages record for (A) IJ from 2010 to 2016 (courtesy: Company of Electricity of Río Negro–EDERSA), and for (B) SCB for June 2011 (courtesy: Cooperative of Electricity of Bariloche). Dashed black lines indicate the beginning of the CC eruption. Blue bars correspond to all the damages reported from which: red crosses correspond to damages unambiguously reported as related to ash, and purple triangles correspond to damages related to switches. However, according to the interviews, most of the switches damages are likely to be associated with ash.
For the case of SCB, no failure in the data log is associated with tephra after the 25 of June, 2011 (Figure 6B). Moreover, the analyzed data log does not include observations about the possible causes of failure. Therefore, impact reconstruction is based on a comparative analysis of this data log, technical reports and data gathered from the interviews. The outages identified as “ash-related” have a duration between ∼2 s and ∼13 h, where events over 1 h occurred the 7, 13, 14, 16, 18, and June 19, 2011 (red crosses, Figure 6B). From interviews, it was found that most frequent failure was associated with the flashover of insulators and other system elements due to the high conductivity of the wet tephra. Damage of the transformers of medium and high voltages were also recorded. Other impacts were associated with the abrasion of different system components (e.g., power plant roof, clogging of air intakes and switches, Craig et al., 2016a). This analysis highlighted that only major events were described in detail and that these events were related to the duration but also to the area affected, as only the outages affecting a large part or the entire locality of SCB were described in technical terms.
With this analysis, it was found that the severity of the impact can be described associated with both the duration and the area affected. The number of users affected, which is generally reported in the outage data log, can be used as a proxy of the area affected by normalizing it by the total number of users as follows,
Figure 7 shows the severity of power outage impacts for the three localities; notice that VLA points correspond to the major events that have been recorded by the Civil Protection because of their affected area (
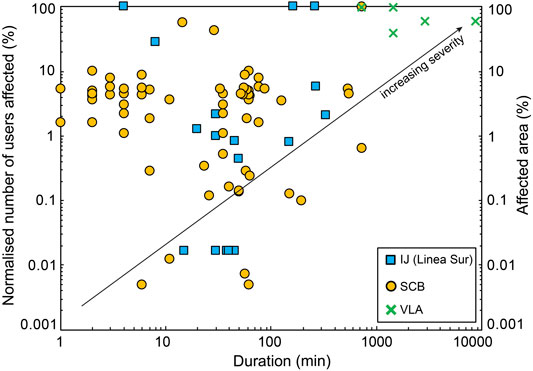
FIGURE 7. Severity of power outage impacts in terms of duration vs. normalized number of users (for SCB and IJ) or affected area (for VLA). Source of data: IJ (Company of Electricity of Río Negro–EDERSA data log), SCB (Cooperative of Electricity of Bariloche data log), VLA (Civil Protection bulletins).
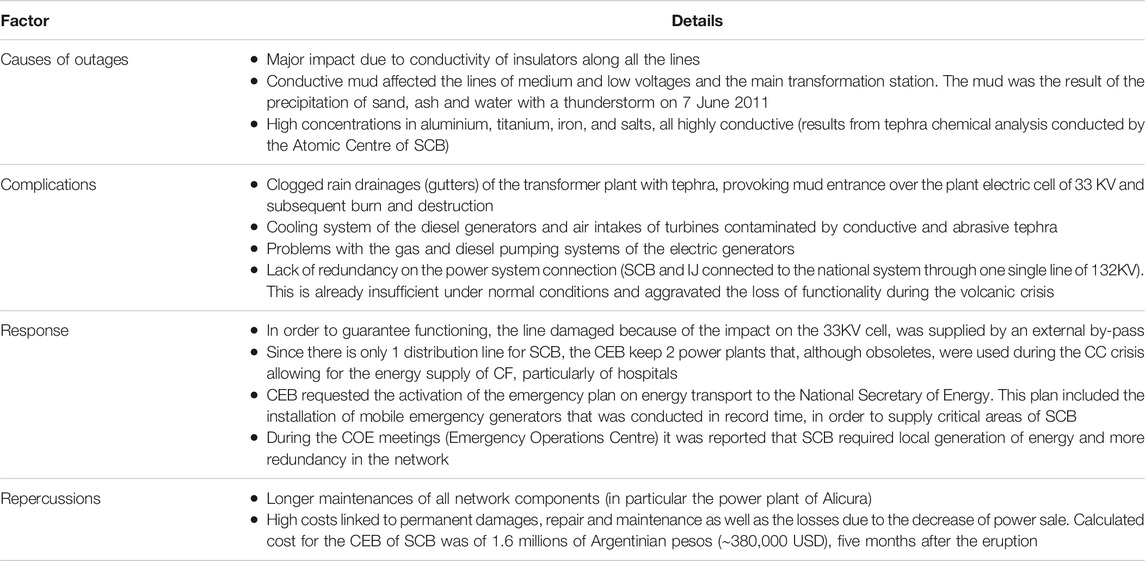
TABLE 5. Integrative impact assessment–Step II: finding evidences of impacts and aggravating factors on the power system of SCB. Data compiled from interviews and technical reports of the CEB.
3.2.3 Step III: Structuring Impact Data
Previous analysis shows that one of the most common impacts of the CC eruption on the power system was associated with the flashover of the electrical insulators caused by ash accumulation, as also stated by Wilson et al. (2013) and (Craig et al., 2016b). These components are present all along the electrical system, i.e., generation, transmission and distribution grids. Once an insulator fails, part of the system loses its functionality generating a power supply disruption that can affect from one user to the whole locality. Disruptions of the power supply will generate a sort of cascading effects in all the CI and CF. Following the methodology and structure of causal order proposed in this study (Table 1 and Figure 3), this sequence of impacts has been classified and structured here as follows: i) first order: physical damage of insulators, described by a fault tree; ii) second order: loss of functionality of the power supply, described by an event tree; and iii) third order: systemic impact on the road system, described by an event tree.
3.2.3.1. First Order–Physical Damage of Insulators
In order to develop a fault tree, a good knowledge of the power system and all possible causes that lead to the failure of insulators are required. The root causes have been analyzed based on our results combined with previous studies on the physical damage due to flashover of electrical insulators (Wardman et al., 2010, 2012, 2014; Lopez Chachalo et al., 2016; Lopez Chachalo, 2017). From the interviews, it was found that the salt content and the amount of humidity present in the tephra are key factors to initiate the conductivity around the insulator. Immediate clean-up of insulators significantly reduced the probability of flashover. However, for the three localities, the accessibility to the damaged components was not always easy and the coordination with public works was crucial. In addition, in VLA it was found that manual isolation (by covering the insulator with PVC protectors) was also useful to avoid the accumulation of tephra. As a preliminary step, a fishbone diagram was compiled to visualize and classify all the possible factors associated with hazard (i.e., tephra features, meteorological conditions), vulnerability (i.e., material type and design, insulator design), and response (i.e., intervention measures) (Supplementary Figure S5).
The fault tree presented in the Figure 8 shows the logical connections between the root causes leading to the insulator flashover. The failure occurs when the electrical current leakage builds up for a given duration of time. The leakage occurs if the ash becomes conductive and adheres to the surface of the insulator. Indeed, the electrical conductivity is produced as a result of the salt content in the tephra that dissolves in contact with water (i.e., favourable wet conditions, given by the intensity of rain, snow, fog or dew, Figure 8B). The free flow of electrons produces a leakage of current that builds up until the heat in the tephra layer is enough to induce a rate of evaporation larger than the rate of moisture accumulation (Wardman et al., 2012, Wardman et al., 2014). The adherence would be determined by the tephra grain size and the design of the insulator (Figure 8A). From experiments, it was found that flashover is favoured by adherence of fine material on the surface (Lopez Chachalo et al., 2016), and vertical orientation of the insulators (Wardman et al., 2014; Lopez Chachalo et al., 2016; Lopez Chachalo, 2017). In addition, experiments of Wardman et al. (2014) showed that the fog-type and ceramic suspension insulators are the most performant to avoid the tephra adherence. Currently, there is no knowledge of the precise thresholds for each cause of the fault tree. It was noticed in SCB that if the tephra dries up before 200 milliseconds, only a fluctuation of electrical current will happen. Wardman et al. (2014) found that more than 1 mm of tephra thickness, or more than 40% of surface coated by tephra in the interior of the insulator are critical, and can lead to failure. The solubility of salts might be favoured by a light rain, snow or fog with a sufficient intensity and duration to wet the tephra but not to wash it. In the case of the CC eruption, flashovers were reported in both proximal and distal areas (i.e., coarse and fine ash); however, the intensity of rain and wind played a major role for washing the insulators (case of IJ) instead of inducing conductivity (case of SCB).
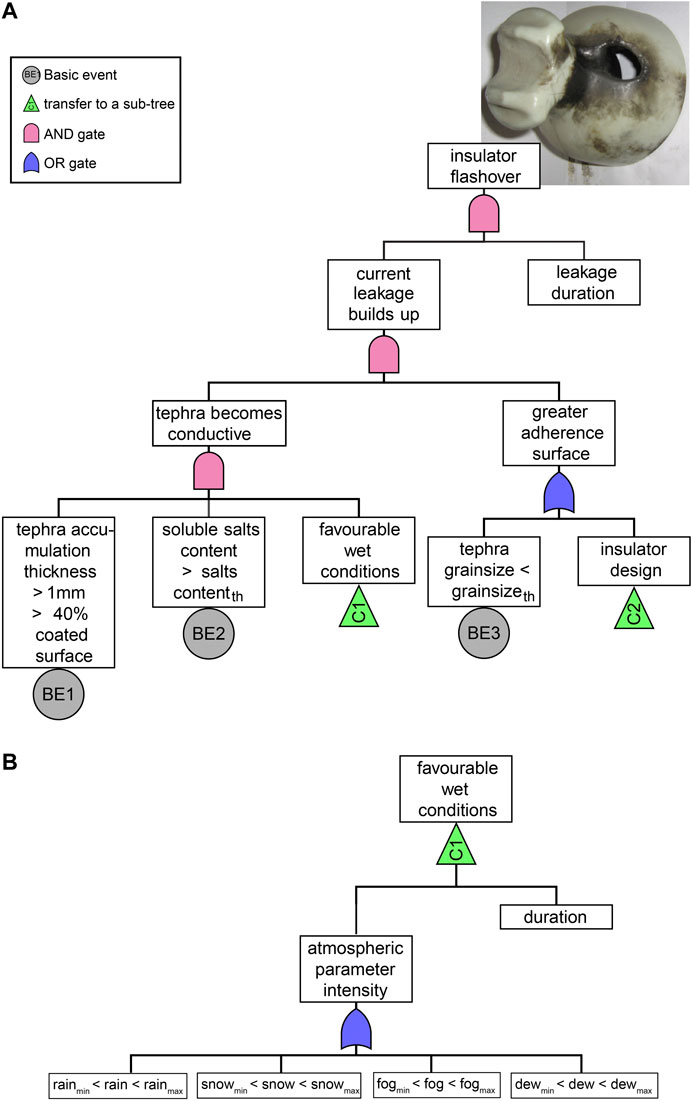
FIGURE 8. First order of impact: electrical insulator flashover described by a main fault tree (A) including subtrees (green triangles). The subtree C1 describing the atmospheric favourable conditions is shown in (B). Contentth and grainsizeth indicate threshold values that still need to be explored. Photo courtesy: Cooperative of Electricity of Bariloche.
Second Order - Loss of Functionality of Power Supply
If the short-circuit is high enough to shoot the circuit breaker, the disruption of service will occur. An event tree constituted by a series of logical questions that encompasses the main factors leading to different scenarios of power supply outages is presented in the Figure 9A. These questions are the result of the previous comprehensive analysis, which highlighted that the duration of each outage was directly related to the facility to detect and access the location of the failure, as well as the criticality of the insulator (e.g., its position in the system) and the capacity to guarantee the power supply thanks to external generators. The resulting scenarios are classified in terms of duration from very-short to very-long term outage (Figure 9A). These scenarios must be combined with the physical location (i.e., georeferenced users affected) in order to evaluate the severity of each impact in terms of duration and area affected (Figure 7).
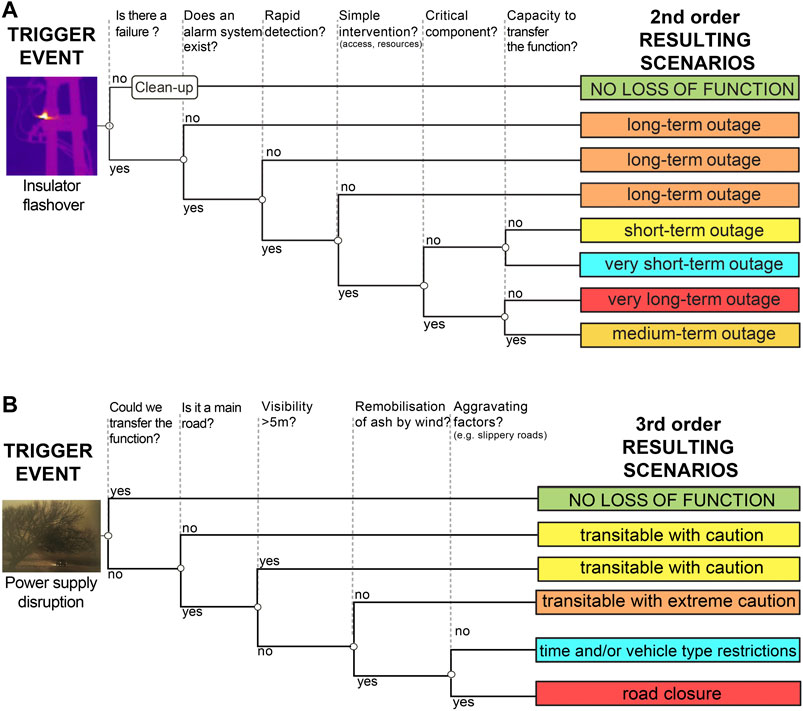
FIGURE 9. (A) Second order of impact: loss of functionality of the power system due to the flashover of insulators described by an event tree. Color scale from blue to red indicates the duration of loss of functionality from very-short term to very-long term outage. (B) Third order of impact: loss of functionality of the road system due to the power supply disruption described by an event tree. Color scale from blue to red indicates the consequences of impact in terms of degree of caution or restrictions on the road network. Photo courtesy: Cooperative of Electricity of Bariloche (insulator flashover) and Chino Leiva (power supply disruption).
3.2.3.3. Third Order - Systemic Impact on CI
Once the power system loses its functionality due to a physical damage, all the CI that depend on the power system will be affected. Figure 9B shows the event tree describing the systemic impact on the road system due to the power supply disruption, and the subsequent resulting scenarios. Logical questions concerning the type of road, the visibility and aggravating factors (e.g., slippery roads) that led to different scenarios of roads closure. Functionality scenarios used by the National Direction of the Road Network of Argentina for the province of Neuquén are applied on Figure 9B. It means that for a third order of impact, the severity is expressed in terms of the duration and area affected of the 2nd order (i.e., outage of power supply) combined with an established scale of functionality that is inherent to each CI. In the case of the road system in Argentina for example, the system can continue operating with some restrictions (i.e., caution and/or vehicle type), scenarios that are considered in the event tree of the Figure 9B.
3.2.4 Step IV: Identifying Critical Points
The final goal of the proposed framework is to detect key vulnerability factors and identify the response actions that mitigated the effects or might mitigate a potential future impact. The versatility of the logical tools (i.e., fault and event trees) allows to discretize each part of the complex cascading impacts on CI and to re-connect in such a way that key factors for each risk component are clearly identified and hotspots of vulnerability are emphasized. Figure 10 represents the bow-tie structure of the causal orders of impacts induced by a physical damage on a component of the electrical system. In this case, the severity of impacts associated with the power system can be reduced by avoiding the physical failure of insulators (i.e., 1st order impact); reducing the duration of outages (i.e., 2nd order impact); and reducing or avoiding aggravating factors (i.e., 3rd order impact) (Figure 10). It has been observed that a direct intervention on the insulators was crucial to diminish or to stop flashovers. Protecting (covering) or cleaning the insulators were effective, rapid response strategies in the three studied communities, a strategy that has been also used in other volcanic contexts (Magill et al., 2013; Jenkins et al., 2015a). However, acting directly on the design of the power system could be a more efficient mitigation measure in the long term. For instance, increasing the number of insulators in the critical lines (personal communication, Juan Ramirez), modifying the orientation of insulators (i.e., horizontal insulators are more performant than vertical ones, Wardman et al. (2014), or developing more resistant materials that can be used in volcanic regions, represent possible mitigation measures for the future. Nonetheless, this requires empirical and experimental data to better constrain the underlying processes and their thresholds.
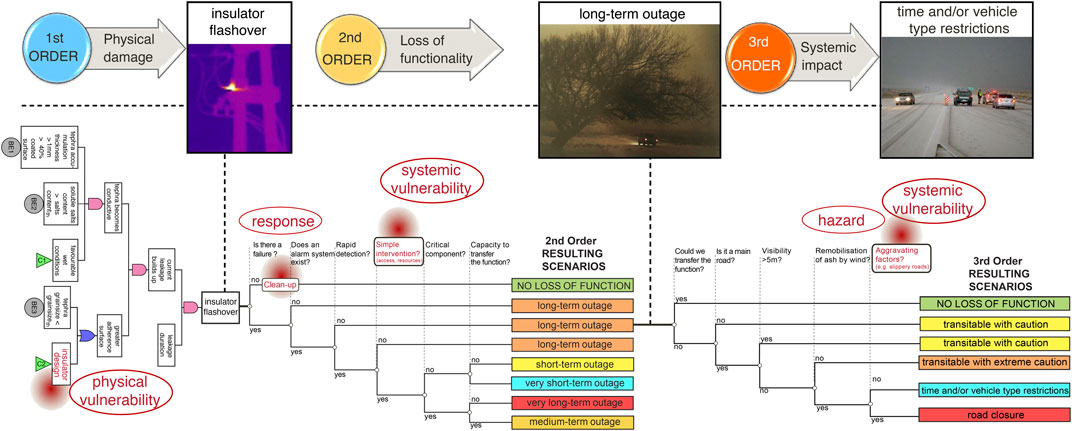
FIGURE 10. Integrative impact assessment–Step IV: Identifying critical points. The bow-tie structure corresponds to the connection of the logical tools shown in Figures 8, 9A,B associated with the cascading impacts on the power system. As an example, the identified risk components (i.e., hazard, physical and systemic vulnerabilty and capacity of response) are shown. Red circles indicate vulnerability hotspots that most likely increase the severity of impacts. Photo courtesy: Cooperative of Electricity of Bariloche (insulator flashover), Chino Leiva (power supply disruption), Diario de Río Negro, http://www.rionegro.com.ar/diario/ (road system disruption).
A critical issue to reduce the duration of outages is the coordination of technicians of the power system, Civil Protection and waste management works. In fact, clean-up processes must be very efficient, and road accessibility is fundamental not only for cleaning the insulators, but also to access the lines when a failure already happened to ensure a rapid detection and intervention, decreasing in this way the duration of the outage. All this coordination is complex and requires a good understanding of the sequence of impacts, the dependencies among systems and the existent policies in order to design and standardize protocols of actions for the future.
4 Discussion
The proposed framework attempts to build on the system-of-systems perspective of risk assessments (UNDRR, 2019) by emphasizing the roles of each risk component, in particular, the role of systemic vulnerability on triggering complex cascading effects in regions affected by volcanic eruptions. The wide forensic perspective including various risk techniques such as narrative reconstruction, relational and dependency analysis and logical tools provides a comprehensive methodology in which a holistic view of impacts (Step I) is combined with a more detailed and integrative post-event impact assessment by applying a causal order (Steps II to IV).
Following a holistic approach, the dependency index used in the matrix of Figure 5 represents a useful technique to map the most critical and the most dependent systems, and in this manner, to prioritize which systems require detailed analysis. Our results suggest that the power, road and telecommunications are the most necessary CI systems in SCB since all the other systems depend on them to continue functioning. On the other hand, the residents, Civil Protection and tourists are the sectors more dependent (in particular Civil Protection activities are highly dependent on the power supply and the roads accessibility as well as on a good coordination with all the CS, CF and social sectors) (Figure 5, Table 4). It is important to consider that this holistic approach could be biased towards the major impacts (e.g., general blackouts, general closure of roads, total isolation of people), as it is mostly based on interviews where the interviewees tended to focus on the bigger impacts often disregarding important aspects and trends of small events that could be more frequent. Besides, this analysis depends on the degree of knowledge of each sector, which is often inhomogeneous (Table 4) and this can lead to misinterpretation. A good transition towards a semi-quantitative or quantitative dependency index could be also developed if a deeper knowledge of each system is achieved and network dependency criteria can be established (Setola and Theocharidou, 2016).
The integrative impact assessment proposed in this study attempts to encompass the complexities associated with the different risk components (hazard, exposure, multiple dimensions of vulnerability and capacity) by discretizing the impacts according to a causal order (Table 1). It has been observed that direct impacts on CI due to tephra sedimentation and wind remobilisation of ash start with a physical damage (first order) in various components of a given system, which combined together, can trigger significant disruptions on the functionality of the system (second order). In modern societies, the high dependency across all sectors at all levels implies that the loss of functionality of CI triggers systemic impacts (third order) on other CI, CS and CF, as well as consequences on the economic and social sectors (higher order). The discretization proposed with this framework is possible thanks to the versatility of the bow-tie approach by connecting logical tools such as fault trees to describe physical failure of components (by applying a deductive analysis of all possible causes), and event trees to describe the possible scenarios (by applying an inductive analysis of the consequences) (Figures 3, 10). The different logical tools can be applied separately or be interconnected in different ways. In this study, a fault tree has been developed for the most common and known physical damage caused by tephra on the power system (i.e., flashover of insulators, Figure 8), which has been connected to the event trees developed to describe the loss of functionality of the power and road systems (Figure 9). However, for an exhaustive analysis, the different physical damages could be described by different fault trees that could be then connected to the already developed event tree describing the loss of functionality of the power system. Indeed, since root-cause techniques have been widely used in reliability (Schultz et al., 2010), and industrial incident analysis (Stamatelatos and Vesely, 2002; IAEA, 2015), the use of fault and event trees is highly compatible with most of the failure analysis and incident organigrams that many CI companies have already established. If existent, we only need to incorporate the factors that can be associated with tephra fallout and wind-remobilisation of volcanic ash. This versatility is tremendously interesting within the multi-character of volcanic eruptions (i.e., multi-hazard, multi-temporal, multi-spatial) that generate complex cascading paths that are difficult to disentangle. Logical tools have been widely used to map the cascading effects on CI in many other hazards (e.g., Pitilakis et al., 2011; Pitilakis et al., 2014; Garcia-Aristizabal et al., 2015; Garcia-Aristizabal et al., 2017; Garcia-Aristizabal et al., 2019), and it is proposed here for the first time in a volcanic context.
CI are complex interconnected and interdependent systems that require specific conditions to guarantee functioning especially in the case of long-lasting volcanic eruptions. The CC eruption demonstrated that primary tephra fallout and subsequent wind remobilisation of ash can be very disruptive and could generate long-lasting chains of effects. This sequence of impacts is related to several hazard conditions acting at different space and time scales, but predominantly, to the multiple dimensions of vulnerability of the systems exposed and their capacity to respond. Our results show that systemic vulnerability amplifies the effects of physical failure in a way that the functionality of other CI can be affected with the same hazard conditions (type, intensity, duration). As an example, with similar volume and grainsize of tephra fallout, the loss of functionality of the power system in SCB, mostly associated with electric insulators flashover, lasted from 2 to 120 min. This contrasting duration was mainly related to the time to detect and access the damaged insulator, which in turn depended not only on the power system resources but also on the adequate functioning of roads, rather than the hazard intensity. This difference in power outages duration implied different consequences depending on the area (e.g., neighbourhood) and/or system (e.g., hospital, water) that was subsequently affected by the power outage. Previous studies developing Disruption and Damage States scales on CI due to tephra fallout have been conducted by Wilson et al. (2014) and Jenkins et al. (2015b). Based on a single hazard parameter (i.e., tephra thickness), these Disruption and Damage States are a discrete scale, from no damage, through cleaning required, repair required, and right up to replacement or financially expensive repair, with increasing tephra thickness. Other studies, based on specific scenarios of impact for Auckland (DEVORA project, New Zealand, 2008–2015) have also proposed different metrics for the loss of functionality such as full service - rolling outages - no electricity service (Hayes et al., 2016; Blake et al., 2017; Deligne et al., 2017). The classification of damage states is a first attempt to classify the impacts of CI based on qualitative descriptors of damage. However, these Disruption and Damage State scales disregard key contributions of systemic vulnerability (e.g., interdependencies), system design and capacity to respond, that are fundamental in CI, as well as the effects of secondary hazards, that are often associated with tephra fallout, but underestimated or ignored.
The main purpose of an impact assessment and the Disruption and Damage States scales is to forecast the expected impact in the case of a future eruption and the expected scenarios, for which the severity of impacts might be well defined. For the case of the power system, the severity of outages has been associated with the duration and affected area (Figure 7). From the framework perspective, the impact scenarios resulting from the event tree analysis (Figure 9A), classified as very-short, short, medium, long, very-long term outages must be combined with the geographical location of impacts (ideally in a GIS-based system) in order to identify the potentially affected areas. Each of these scenarios represents, in turn, the basis of a third order of impact in which the exposure of the other CI or CF affected can be determined (Figure 9B). The definition of these scenarios is subject to each particular context. In our case, for example, the scenarios of loss of functionality for the road system have been defined based on the reports of the National Direction of Road Network of Argentina (Figure 9B); but this classification can certainly be adapted to another context. What is relevant here is how to define the boundaries of each severity level. For instance, an outage of 5 min for one user will not have the same cascading effects than a total blackout of a city during the same 5 min. In this sense, the thresholds associated with the maximum time that one user can stand without energy will depend on the type of user (e.g., residents vs critical infrastructure), hazard characteristics (e.g., intense tephra sedimentation and humidity conditions), the quality and duration of response actions, and the multiple vulnerability conditions that are still not investigated in volcanic impacts assessments. For this reason, the coloured scales of severity used in the scenarios of the Figures 9A,B are only indicative and accurate thresholds need to be addressed. Another important question is how to reduce the duration and/or area affected. In the case of power outages, it has been identified that acting directly on the design of the insulator or improving the coordination of stakeholders during the crisis are fundamental aspects that have to be considered in order to reduce the frequency and duration of outages. However, more empirical data is necessary for the sake to understand the different drivers that lead to long cascading impacts on CI due to tephra.
With this framework, the discretization of impacts and consequences within a general perspective of the context is important to understand what we need to assess during the next volcanic event, and with which metrics (i.e., narrative or technical description, qualitative or quantitative metrics). The resolution and accuracy of the analysis will be related to the purpose of the study and the quantity and quality of data available. In fact, the main limitation during this study has been the heterogeneity of the available data (i.e., qualitative and quantitative), involving several sources (e.g., semi-structured interviews, technical reports, newspapers, scientific papers) and different level of detail (e.g., outages logs vs narrative descriptions of punctual impacts). The limited amount of quantitative data did not allow the full use of the logical tools (i.e., quantitative estimations and probabilistic analysis of the fault and event trees). Therefore, comprehensive post-disaster impact reporting should be developed to provide the level of detail necessary to feed the mentioned tools and to build on robust statistical post-event impact assessments. Recent international initiatives have attempted to improve the current fragmentation and gaps in data information (see Walia et al. (in press) for a review). Notably, significant advances in data collection methodologies have been proposed by the European Joint Research Center of the Institute for the Protection and Security of the Citizen (De Groeve et al., 2013, 2014, 2015); and the joint effort of the UN Development Group, the World Bank and the European Union that resulted in the design of the Post-Disaster Needs Assessment methodology. As a common aim, these methodologies count on the engagement of actors at local level, who can establish impact databases for operational use that ultimately might support and feed forensic investigations of disasters. Since forensic analysis requires sufficient detailed impact information, it represents a unique approach not only to understand what type of data and with which metrics need to be collected, but also to design local databases in order to feed in the future the developed logical tools. As a lesson learned from our analysis, a detailed damage collection template for the power system has been designed based on the CC case study (Supplementary Figure S6). This form can serve as a basis for future impact assessments being adapted to local needs, according to the outage’s logs of each CI company. However, some of the recommended factors in this template can already reflect the required parameters in order to fulfill the fault and event trees, and to construct an impact data catalogue in the future.
The significant limitations in impact data collection also highlight a fundamental aspect of any post-event impact assessment framework: regardless of the tools and methodologies used, the complexity and the plural-component character of impacts due to volcanic eruptions require a synergy among different stakeholders. For this reason, within the frame of this study, the data analysis of technical reports and outage data logs has been conducted in cooperation with the technicians of the local power supply companies of SCB and IJ. In addition, within the frame of this study, we also promoted a dedicated workshop to meet local stakeholders and practitioners from various sectors (most of them actively participated since the beginning of the project), to discuss and refine the main findings and resulting outcomes of this framework. Outcomes of this workshop are available in Dominguez et al. (2020a). An efficient collaborative work beyond the interviews along the study period (e.g., workshops, join work strategies) is hence fundamental to i) co-design and co-produce future data collection guidelines; ii) understand the role of each risk component in the expected impacts; iii) improve integrative impact assessments; and iv) co-design mitigation measures and policies for future volcanic events.
5 Conclusion
Based on the forensic investigation of disasters and root cause analysis, the integrative impact assessment framework proposed in this study is applied to analyze the impacts associated with tephra fallout and wind remobilisation of volcanic ash of the 2011–2012 CC eruption as a case study.
The main outcomes of the proposed post-event impact assessment framework include:
• A strategy to collect, structure and quantify impact data for CI and CF that involves complex and multi-dimensional cascading patterns (Figures 2, 3). This approach promotes i) a comprehensive analysis of dependencies within (intra-dependencies) and among (inter-dependencies) systems; ii) the assessment of impact causes and consequences (scenarios) in a level of detail that is directly proportional to the purpose of the study and the data quality; iii) the discretization of impacts according to a causal order that ultimately provides the key factors to identify critical vulnerability hotspots where actions must be taken to reduce risk; and iv) the synergy among stakeholders that is definitely required when assessing impacts on CI, CF, and CS.
• The identification of logical tools to help elucidate and quantify the root causes and consequences of cascading impacts on CI; in particular, the bow-tie approach that allows for the quantification of complex cascading patterns. The development of the fault and event trees represents the most time- and resource-consuming step of the framework. However, many CI companies have already implemented failure and incident analysis in their organigrams; therefore, if available, only the factors related to tephra fallout and wind remobilisation of volcanic ash need to be integrated. Once the fault and event trees are developed, they can be validated, modified and/or improved based on new impact data and/or experimental advances.
• The discretization of impacts according to a causal order (Table 1) where a detailed multi-dimensional analysis including different scenarios is possible (Figure 3). This analysis provides not only a better understanding of the critical aspects for each risk component, but also the basis to develop statistically robust impact data catalogues, fragility/functionality curves and/or Damage and Disruption States scales.
• The development of a damage collection template for the power system to optimize the collection of impact data associated with future volcanic eruptions (Supplementary Figure S6).
• The crucial role of the communication, coordination and synergy among all the stakeholders (e.g., Civil Protection, CI practitioners, scientists) to advance in volcanic risk reduction.
The main conclusions associated with the application of the proposed framework to the 2011–2012 CC eruption as a case study include:
• The importance of long-lasting consequences even of small to moderate size eruptions. Impacts of the 2011–2012 CC eruption were associated with various hazardous phenomena that acted at multi-temporal and multi-spatial scales where tephra fallout and subsequent wind remobilisation caused significant consequences on the exposed communities, continuing even today (2021).
• The major role of the systemic vulnerability on the loss of functionality of CI, CS, and CF. The 2011–2012 CC eruption highlighted that major impacts are related to the dependency among systems and the transferability of functions. In particular, the dependency matrix developed for the case of SCB provided not only significant insights into the complex connections among systems, but also an objective reasoning to prioritise a more detailed analysis of impacts focused on the power system.
• The classification and structure of the cascading effects due to the impacts on the power system. The application of the proposed framework helped to establish a causal order of impacts, from the physical damage of electrical insulators (first order) that provoked power outages (second order) which in turn provoked or aggravated the loss of functionality of the road system (third order). The severity of the resulting scenarios has been associated with the duration and area affected (in the case of the second order) and a scale of functionality previously established by the CI system (in the case of the third order).
• The identification of vulnerability hotspots where mitigation measures could be implemented. Three key vulnerability aspects to reduce or avoid cascading effects on the power system are: i) avoid the physical failure of electrical insulators; ii) reduce the duration of outages; and iii) reduce or avoid aggravating factors associated with the connections among CI, CF, and CS.
Data Availability Statement
The dataset generated for this study can be found at https://doi.org/10.26037/yareta:xohh6yvjwbg5rkwb5m7mq6ll5u.
Author Contributions
All the authors were involved in the conceptualization, design and development of the framework. LD, CF, and CB conducted the field work. LD and AG developed the logical tools. LD elaborated the manuscript. All authors were involved in data analysis and interpretation as well as the edition of this manuscript.
Funding
This study was supported by the Swiss National Science Foundation (200021–163152).
Conflict of Interest
The authors declare that the research was conducted in the absence of any commercial or financial relationships that could be construed as a potential conflict of interest.
Acknowledgments
We thank Donaldo Bran and the National Institute of National Agricultural Technology of San Carlos de Bariloche (Argentina) for their support both in the field and the organization of the workshop. We thank Jazmin Miguel and Virginia Velasco for their valuable help organizing the interviews and support in the field. We thank Juan David Ramirez, Eduardo Broglio and Gonzalo Pazos for their insightful discussions and support with data treatment. Special thanks to all stakeholders from critical infrastructures and critical facilities as well as the communities of Villa La Angostura, San Carlos de Bariloche and Ingeniero Jacobacci (Argentina). We also thank two anonymous reviewers for constructive comments and corrections.
Supplementary Material
The Supplementary Material for this article can be found online at: https://www.frontiersin.org/articles/10.3389/feart.2021.645945/full#supplementary-material
Abbreviations
CC, Cordón Caulle volcano; CI, critical infrastructures; CS, critical services; CF, critical facilities; FORIN, FORensic INvestigation of Disasters program; IJ, Ingeniero Jacobacci; PERC, Post-Event Review Capability; SCB, San Carlos de Bariloche; STREVA project, Strengthening resilience in volcanic areas, 2012–2019, https://streva.ac.uk; SYNER-G project, Systemic Seismic Vulnerability and Risk Analysis for Buildings, Lifeline Networks and Infrastructures Safety Gain; VLA, Villa La Angostura.
References
Andersen, B., and Fagerhaug, T. (2006). Root Cause Analysis: Simplified Tools and Techniques. 2nd Edn. Milwaukee: William A. Tony
Armijos, M. T., and Few, R. (2017). Surviving Disaster: Resettlement, Recovery and Ongoing Risk at Nevado del Ruiz, ColombiaTech. rep (Norwich: University of East Anglia)
Armijos, M. T., Phillips, J., Wilkinson, E., Barclay, J., Hicks, A., Palacios, P., et al. (2017). Adapting to Changes in Volcanic Behaviour: Formal and Informal Interactions for Enhanced Risk Management at Tungurahua Volcano, Ecuador. Glob. Environ. Change 45, 217–226. doi:10.1016/j.gloenvcha.2017.06.002
Barclay, J., Wilkinson, E., White, C. S., Shelton, C., Forster, J., Few, R., et al. (2019). Historical Trajectories of Disaster Risk in Dominica. Int. J. Disaster Risk Sci. 10, 149–165. doi:10.1007/s13753-019-0215-z
Blake, D. M., Deligne, N. I., Wilson, T. M., Lindsay, J. M., and Woods, R. (2017). Investigating the Consequences of Urban Volcanism Using a Scenario Approach Ii: Insights into Transportation Network Damage and Functionality. J. Volcanology Geothermal. Res. 340, 92–116. doi:10.1016/j.jvolgeores.2017.04.010
Bonadonna, C., Biass, S., Manzella, I., Frischknecht, C., Gregg, C., Galderisi, A., et al. (2009). ENSURE Framework. WP 5: Application of an Integrated Vulnerability Conceptual approach. Del. 5.3.3: Development of the Integrated Approach on the Vulcano Case Study. Tech. rep., Eur. Comm. 155.
Bonadonna, C., Cioni, R., Pistolesi, M., Elissondo, M., and Baumann, V. (2015). Sedimentation of Long-Lasting Wind-Affected Volcanic Plumes: the Example of the 2011 Rhyolitic Cordón Caulle Eruption, Chile. Bull. Volcanology 77, 1–13. doi:10.1007/s00445-015-0900-8
Burton, I. (2010). Forensic Disaster Investigations in Depth: A New Case Study Model. Environ. Sci. Pol. Sustainable Development 52, 36–41. doi:10.1080/00139157.2010.507144
Craig, H. M., Wilson, T., Stewart, C., Outes, V., Villarosa, G., and Baxter, P. (2016a). Impacts to Agriculture and Critical Infrastructure in Argentina after Ashfall from the 2011 Eruption of the Cordón Caulle Volcanic Complex: an Assessment of Published Damage and Function Thresholds. J. Appl. Volcanology 5, 1–31. doi:10.1186/s13617-016-0046-1
Craig, H. M., Wilson, T., Stewart, C., Villarosa, G., Outes, V., Cronin, S., et al. (2016b). Agricultural Impact Assessment and Management after Three Widespread Tephra Falls in Patagonia, South America. Nat. Hazards 82, 1167–1229. doi:10.1007/s11069-016-2240-1
De Groeve, T., Corbane, C., and Ehlrich, D. (2015). Guidance for Recording and Sharing Disaster Damage and Loss Data. Tech. rep., Luxembourg: European Commission Joint Research Centre (EU-JRC) Report EUR 27192.
De Groeve, T., Poljansek, K., Ehlrich, D., and Corbane, C. (2014). Current Status and Best Practices for Disaster Loss Data Recording in EU Member States: A Comprehensive Overview of Current Practice in the EU Member States. Tech. rep. Luxembourg: European Commission Joint Research Centre (EU-JRC) Report EUR 26879.
De Groeve, T., Poljansek, K., and Ehlrich, D. (2013). Recording Disaster Losses: Recommendations for a European Approach. Tech. rep., Luxembourg: European Commission Joint Research Centre (EU-JRC). Report EUR 26111.
Deligne, N. I., Fitzgerald, R. H., Blake, D. M., Davies, A. J., Hayes, J. L., Stewart, C., et al. (2017). Investigating the Consequences of Urban Volcanism Using a Scenario Approach I: Development and Application of a Hypothetical Eruption in the Auckland Volcanic Field, New Zealand. J. Volcanology Geothermal Res. 336, 192–208. doi:10.1016/j.jvolgeores.2017.02.023
Dominguez, L., Bonadonna, C., and Bran, D. (2020a). Workshop on the Impacts Associated with the Primary Fallout of Volcanic Ash and Subsequent Aeolian Remobilisation, Consensual Document. Tech. rep., Geneva: University of Geneva (UNIGE) Available at: https://vhub.org/resources/4611 (Accessed September 10, 2020).
Dominguez, L., Bonadonna, C., Forte, P., Jarvis, P. A., Cioni, R., Mingari, L., et al. (2020b). Aeolian Remobilisation of the 2011-Cordón Caulle Tephra-Fallout Deposit : Example of an Important Process in the Life Cycle of Volcanic Ash. Front. Earth Sci. 7, 1–20. doi:10.3389/feart.2019.00343
Dudenhoeffer, D. D., Permann, M. R., and Manic, M. (2006). Cims: A Framework for Infrastructure Interdependency Modeling and Analysis. In Proceedings of the 2006 Winter Simulation Conference, 478–485.
Elissondo, M., Baumann, V., Bonadonna, C., Pistolesi, M., Cioni, R., Bertagnini, A., et al. (2016). Chronology and Impact of the 2011 Cordón Caulle Eruption, Chile. Nat. Hazards Earth Syst. Sci. 16, 675–704. doi:10.5194/nhess-16-675-2016
Eusgeld, I., Nan, C., and Dietz, S. (2011). "System-of-systems" Approach for Interdependent Critical Infrastructures. Reliability Eng. Syst. Saf. 96, 679–686. doi:10.1016/j.ress.2010.12.010
Few, R., Armijos, M. T., and Barclay, J. (2017). Living with Volcan Tungurahua: The Dynamics of Vulnerability during Prolonged Volcanic Activity. Geoforum. 80, 72–81. doi:10.1016/j.geoforum.2017.01.006
Forte, P., Domínguez, L., Bonadonna, C., Gregg, C. E., Bran, D., Bird, D., et al. (2018). Ash Resuspension Related to the 2011-2012 Cordón Caulle Eruption, Chile, in a Rural Community of Patagonia, Argentina. J. Volcanology Geothermal. Res. 350, 18–32. doi:10.1016/j.jvolgeores.2017.11.021
Garcia-Aristizabal, A., Capuano, P., Russo, R., and Gasparini, P. (2017). Multi-hazard Risk Pathway Scenarios Associated with Unconventional Gas Development: Identification and Challenges for Their Assessment. Energ. Proced. 125, 116–125. doi:10.1016/j.egypro.2017.08.087
Garcia-Aristizabal, A., Kocot, J., Russo, R., and Gasparini, P. (2019). A Probabilistic Tool for Multi-Hazard Risk Analysis Using a Bow-Tie Approach: Application to Environmental Risk Assessments for Geo-Resource Development Projects. Acta Geophys. 67, 385–410. doi:10.1007/s11600-018-0201-7
Garcia-Aristizabal, A., Polese, M., Zuccaro, G., Almeida, M., and Aubrecht, C. (2015). Improving Emergency Preparedness with Simulation of Cascading Events Scenarios. In 12th International Conference on Information Systems for Crisis Response and Management, 1–9. Kristiansand: ISCRAM 2015
Gasparini, P., and Garcia-Aristizabal, A. (2014). Seismic Risk Assessment, Cascading Effects. in Encyclopedia of Earthquake Engineering. Springer, 1–20. doi:10.1007/978-3-642-36197-5_260-1
Hayes, J. L., Blake, D. M., Davies, A. J., Fitzgerald, R. H., Wilson, G., Wilson, T. M., et al. (2016). A Multi-Disciplinary Approach to Developing a Volcanic Eruption Scenario and Temporal Evolution of Impacts to Critical Infrastructure. In 1st International Conference on Natural Hazards and Infrastructure, 1–10
Hicks, A., and Few, R. (2015). Trajectories of Social Vulnerability during the Soufriere Hills Volcanic Crisis. J. Appl. Volcanology 4, 1–15. doi:10.1186/s13617-015-0029-7
IAEA (2015). Root Cause Analysis Following an Event at a Nuclear Installation: Reference Manual. Tech. Rep. TECDOC-1756. Vienna: International Atomic Energy Agency (IAEA).
InfoLEG (2015). Ministerio de Planificación Federal, Inversión Pública y Servicios. Tech. rep., Ministerio de Planificación Federal, Inversión Pública y Servicios - Secretaría de Energía (Argentina). Available at: http://servicios.infoleg.gob.ar/infolegInternet/anexos/245000-249999/248465/norma.htm (Accessed 10 May, 2020)
IRDR (2011). Forensic Investigations of Disasters: The FORIN Project. Tech. rep., Beijing: Integrated Research on Disaster Risk (IRDR)Available at: http://www.irdrinternational.org/projects/forin/about-forin/ (Accessed November 15, 2018).
Jenkins, S. F., Phillips, J. C., Price, R., Feloy, K., Baxter, P. J., Hadmoko, D. S., et al. (2015a). Developing Building-Damage Scales for Lahars: Application to Merapi Volcano, Indonesia. Bull. Volcanology 77, 75. doi:10.1007/s00445-015-0961-8
Jenkins, S. F., Wilson, T. M., Magill, C., Miller, V., Stewart, C., Marzocchi, W., et al. (2015b). Volcanic Ash Fall Hazard and Risk. In Global Volcanic Hazards and Risk. Cambridge University Press, 173–221.
Livingston, A. D., and Jackson, G. (2001). Root Causes Analysis : Literature Review. 1st Edn. Warrington: WS Atkins Consultants Ltd.
Lopez Chachalo, A. J. (2017). Evaluación del impacto de ceniza volcánica en la rigidez dieléctrica de aisladores de suspensión en líneas de alto voltaje mediante análisis del factor de pérdida dieléctrica. PhD thesis. Quito: Escuela Politécnica Nacional.
Lopez Chachalo, A., Ramírez, J., Vásconez, F., Bernard, B., Fausto, V., Hidalgo, S., et al. (2016). Volcanic Ash Impacts on the Dielectric Strength of HVAC Outdoor Suspension Insulators: the Case of Cotopaxi and Tungurahua Volcanoes (Ecuador). Cities on Volcanoes 9. In Understanding Volcanoes and Society: The Key for 2507 Risk Mitigation City (Mitigation City: Puerto Varas).
Magill, C., Wilson, T., and Okada, T. (2013). Observations of Tephra Fall Impacts from the 2011 Shinmoedake Eruption, Japan. Earth Planet. Sp. 65, 677–698. doi:10.5047/eps.2013.05.010
Marzocchi, W., Garcia-Aristizabal, A., Gasparini, P., Mastellone, L., and Di Ruocco, A. (1995). Basic Principles of Multi-Risk Assessment: a Case Study in Italy. Nat. Hazards 100, 551–573.
Mendoza, M. T. (2019). Forensic Investigation of Post-Flood Damage Data to Support Spatial Planning. PhD thesis. Milan: Politecnico di Milano.
Menoni, S., Bonadonna, C., García, M., and Schawarze, R. (2017). “Recording Disaster Losses for Improving Risk Modelling Capacities,” in Science for Disaster Risk Management 2017: Knowing Better and Losing Less. Editors K. Poljanšek, M. Marín Ferrer, T. De Groeve, and I. Clark, chap. 2.4, 85–130.
Menoni, S., Molinari, D., Parker, D., Ballio, F., and Tapsell, S. (2012). Assessing Multifaceted Vulnerability and Resilience in Order to Design Risk-Mitigation Strategies. Nat. Hazards 64, 2057–2082. doi:10.1007/s11069-012-0134-4
Murgida, A. M., Laham, F. M., Chiappe, C. J. P., and Kazimierski, M. A. (2016). Desarrollo territorial bajo sequía y cenizas. Iluminuras 17, 11–29. doi:10.22456/1984-1191.64556
OVDAS (2012). Reportes de Actividad Volcánica, Región Los Ríos - Los Lagos, 2010 to 2012. Tech. rep., Temuco: Volcano Observatory of the Southern Andes - Servicio Nacional de Geología y Minería (OVDAS-SERNAGEOMIN)Available at: https://www.sernageomin.cl/complejo-volcanico-puyehue-cordon-caulle/ (Accessed January 31, 2020).
Oxford Economics(2010). The Economic Impacts of Air Travel Restrictions Due to Volcanic Ash. A Report Prepared for Airbus (Oxford), 1–12. Available at: https://www.oxfordeconomics.com/my-oxford/projects/129051 (Accessed December 10, 2019)
Pescaroli, G., and Alexander, D. (2015). A Definition of Cascading Disasters and Cascading Effects : Going beyond the “toppling dominos” metaphor, in Planet@Risk (Davos: Global Risk Forum GRF Davos), Vol. 2, 58–67.
Pistolesi, M., Cioni, R., Bonadonna, C., Elissondo, M., Baumann, V., Bertagnini, A., et al. (2015). Complex Dynamics of Small-Moderate Volcanic Events: the Example of the 2011 Rhyolitic Cordón Caulle Eruption, Chile. Bull. Volcanology 77, 1–24. doi:10.1007/s00445-014-0898-3
Pitilakis, K., Crowley, H., and Kaynia, A. M. (2014). SYNER-G : Typology Definition and Fragility Functions for Physical Elements at Seismic Risk. Tech. rep., Eur. Comm. New York: Springer, 27, 420. doi:10.1007/978-94-007-7872-6
Pitilakis, K., Franchin, P., Cavalieri, F., Pinto, P., Lupoi, A., Vanzi, I., et al. (2011). General Methodology for Systemic Vulnerability Assessment. Tech. rep., Eur. Comm.
Pyle, D. M., Barclay, J., and Armijos, M. T. (2018). The 1902-3 Eruptions of the Soufrière, St Vincent: Impacts, Relief and Response. J. Volcanology Geothermal Res. 356, 183–199. doi:10.1016/j.jvolgeores.2018.03.005
Rinaldi, S. (2004). Modeling and Simulating Critical Infrastructures and Their Interdependencies. 37th Annual Hawaii International Conference On System Sciences. Proc. of the 2004, 1–8.
Rinaldi, S. M., Peerenboom, J. P., and Kelly, T. K. (2001). Identifying, Understanding, and Analyzing Critical Infrastructure Interdependencies. IEEE Control. Syst. Mag. 21, 11–25. doi:10.1109/37.969131
Rofman, A. (2010). La Economía Solidaria Y Los Desafíos Actuales. Revista de Ciencias Sociales, segunda época 2, 159–175.
Rose, A. (2004). “Economic Principles, Issues, and Research Priorities in Hazard Loss EstimationModeling Spatial and Economic Impacts of Disasters,” in Advances in Spatial Science Editors Y. Okuyama, and S. Chang (Springer), chap. 2, 13–36. doi:10.1007/978-3-540-24787-6_2
Ruijter, A., and Guldenmund, F. (2015). The Bowtie Method: A Review. Saf. Sci. 88, 211–218. doi:10.1016/j.ssci.2016.03.001
Sapountzaki, K., Wassenhoven, L., Melissourgos, Y., Menoni, S., Kundak, S., Desramaut, N., et al. (2009). ENSURE Framework. WP 2: Integration and Connection of Vulnerabilities. Del. 2.1.2: Relation between Systemic and Physical Vulnerability and Relation between Systemic, Social, Economic, Institutional and Territorial Vulnerability. Tech. rep., Eur. Comm. 93.
Scaini, C., Biass, S., Galderisi, A., Bonadonna, C., Folch, A., Smith, K., et al. (2014). A Multi-Scale Risk Assessment for Tephra Fallout and Airborne Concentration from Multiple Icelandic Volcanoes - Part 2: Vulnerability and Impact. Nat. Hazards Earth Syst. Sci. 14, 2289–2312. doi:10.5194/nhess-14-2289-2014
Schultz, M. T., Gouldby, B. P., Simm, J. D., and Wibowo, J. L. (2010). Beyond the Factor of Safety : Developing Fragility Curves to Characterize System Reliability-US Army Corps of Engineers. Tech. Rep. Washington: US Army Corps of Engineers. doi:10.21236/ada525580
Setola, R., and Theocharidou, M. (2016). “Modelling Dependencies between Critical Infrastructures,”. Managing the Complexity of Critical Infrastructures. Editors R. Setola, V. Rosatto, E. Kyriakides, and E. Rome (Cham, Switzerland: Springer Nature), 90 chap. 2, 19–41. doi:10.1007/978-3-319-51043-9_2
Stamatelatos, M., and Vesely, W. (2002). Fault Tree Handbook with Aerospace Applications. Washington: Tech. Rep. 8, National Aeronautics and Space Administration (NASA)
Sword-Daniels, V. L., Rossetto, T., Wilson, T. M., and Sargeant, S. (2015). Interdependence and Dynamics of Essential Services in an Extensive Risk Context: a Case Study in Montserrat, West Indies. Nat. Hazards Earth Syst. Sci. 15, 947–961. doi:10.5194/nhess-15-947-2015
UNDRR (2019). Global Assessment Report on Disaster Risk Reduction. Tech. Rep. Geneva: United Nations Office for Disaster Risk Reduction (UNDRR)
Venkateswaran, K., MacClune, K., Keating, A., and Szönyi, M. (2015). The PERC Manual. Learning from Disasters to Build Resilience: a Simple Guide to Conducting a Post Event Review. Tech. rep., Zurich Insurance. Available at: https://floodresilience.net/resources/item/the-perc-manual (Accessed December 5, 2018)
Walia, A., Menoni, S., Farah Dell’Aringa, M., Bakon, M., Rovnak, M., Milenov, K., et al. (in press). Methodologies for Disaster Impact Assessmen. In EU JRC Science for DRM 2020: Acting Today, Protecting Tomorrow, chap. 3.1 (Luxembourg: European Commission Joint Research Centre, EU-JRC).
Wantim, M. N., Bonadonna, C., Gregg, C. E., Menoni, S., Frischknecht, C., Kervyn, M., et al. (2018). Forensic Assessment of the 1999 Mount Cameroon Eruption, West-Central Africa. J. Volcanology Geothermal Res. 358, 13–30. doi:10.1016/j.jvolgeores.2018.06.007
Wardman, J. B., Wilson, T. M., Bodger, P. S., Cole, J. W., and Stewart, C. (2012). Potential Impacts from Tephra Fall to Electric Power Systems: A Review and Mitigation Strategies. Bull. Volcanol. 74, 2221–2241. doi:10.1007/s00445-012-0664-3
Wardman, J. B., Wilson, T. M., Cole, J. W., Bodger, P. S., and Johnston, D. M. (2010). Quantifying the Vulnerability of High Voltage Power Transmission Systems to Volcanic Ashfall Hazards. In EEA Conference And Exhibition, 2010 (Christchurch), 17–18.
Wardman, J., Wilson, T., Hardie, S., and Bodger, P. (2014). Influence of Volcanic Ash Contamination on the Flashover Voltage of HVAC Outdoor Suspension Insulators. IEEE Trans. Dielect. Electr. Insul. 21, 1189–1197. doi:10.1109/tdei.2014.6832265
Wild, A. J., Wilson, T. M., Bebbington, M. S., Cole, J. W., and Craig, H. M. (2019). Probabilistic Volcanic Impact Assessment and Cost-Benefit Analysis on Network Infrastructure for Secondary Evacuation of Farm Livestock: A Case Study from the Dairy Industry, Taranaki, New Zealand. J. Volcanology Geothermal Res. 387, 1–20. doi:10.1016/j.jvolgeores.2019.106670
Wilson, G., Wilson, T. M., Deligne, N. I., and Cole, J. W. (2014). Volcanic Hazard Impacts to Critical Infrastructure: A Review. J. Volcanology Geothermal Res. 286, 148–182. doi:10.1016/j.jvolgeores.2014.08.030
Wilson, T., Stewart, C., Bickerton, H., Baxter, P., Outes, V., Villarosa, G., et al. (2013). Impacts of the June 2011 Puyehue-Cordón Caulle Volcanic Complex Eruption on Urban Infrastructure, Agriculture and Public Health. Tech. Rep. GNS Science Report 2012/20, 88.
Keywords: impact assessment, volcanic eruptions, forensic analysis, systemic vulnerability, cascading effects, bow-tie approach
Citation: Dominguez L, Bonadonna C, Frischknecht C, Menoni S and Garcia A (2021) Integrative Post-event Impact Assessment Framework for Volcanic Eruptions: A Disaster Forensic Investigation of the 2011–2012 Eruption of the Cordón Caulle Volcano (Chile). Front. Earth Sci. 9:645945. doi: 10.3389/feart.2021.645945
Received: 24 December 2020; Accepted: 04 May 2021;
Published: 17 June 2021.
Edited by:
Carmine Galasso, University College London, United KingdomReviewed by:
Christopher Kilburn, University College London, United KingdomPaul William Taylor, Independent Researcher, East Maitland, Australia
Copyright © 2021 Dominguez, Bonadonna, Frischknecht, Menoni and Garcia. This is an open-access article distributed under the terms of the Creative Commons Attribution License (CC BY). The use, distribution or reproduction in other forums is permitted, provided the original author(s) and the copyright owner(s) are credited and that the original publication in this journal is cited, in accordance with accepted academic practice. No use, distribution or reproduction is permitted which does not comply with these terms.
*Correspondence: Lucia Dominguez, bHVjaWEuZG9taW5ndWV6QHVuaWdlLmNo