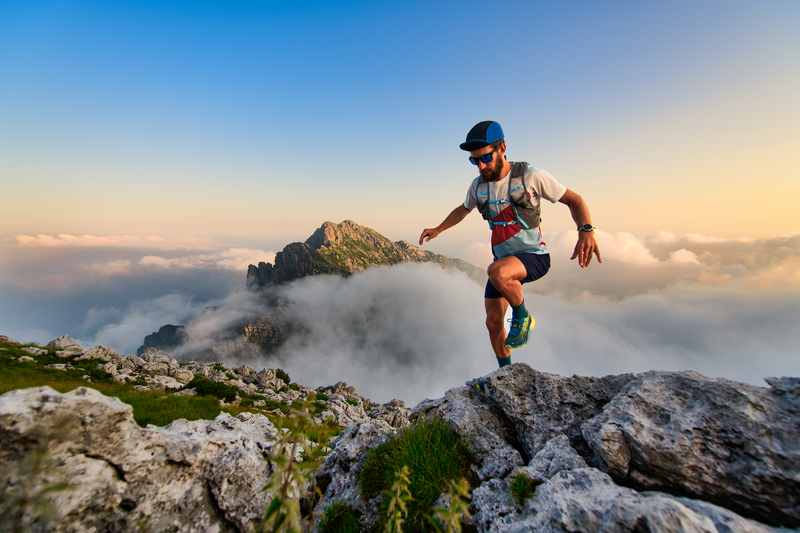
95% of researchers rate our articles as excellent or good
Learn more about the work of our research integrity team to safeguard the quality of each article we publish.
Find out more
ORIGINAL RESEARCH article
Front. Earth Sci. , 02 March 2021
Sec. Paleontology
Volume 9 - 2021 | https://doi.org/10.3389/feart.2021.615370
This article is part of the Research Topic Permian Extinctions View all 18 articles
Throughout their 420-Ma-long history, Lycopodiopsida have played a subordinate role at the landscape level with very few exceptions. One being the arborescent Lepidodendrales that dominated Pennsylvanian peat swamps in equatorial regions. Another is the enigmatic world-wide proliferation of sub-arborescent Isoëtales during, and in the aftermath of the Permo-Triassic terrestrial biosphere crisis that extended deep into the Triassic. Palynological as well as megafossil data shows that in a great proportion of locations around the globe that produced a fossil record, the provincial floras characteristic for the latest Permian were replaced by communities dominated by Isoëtales such as Pleuromeia and its allies. Our analysis of the isoëtalean biology, especially of the genus Pleuromeia, reveals an unusual suite of physiological and life-history traits, all indicating that it was an excellent stress-tolerator, but also a slow-growing weak competitor. This enabled Pleuromeia to thrive during environmental crises and occupy diverse habitats following the decline of other plants groups. Given their unusual biology, Isoëtales’ repeated ubiquity throughout the Early Triassic implies prolonged and repeated environmental stress in localities worldwide. Additionally, it demonstrates that the cosmopolitan isoëtalean-dominated systems produced a low-productivity, low-diversity terrestrial trophic base of the food web that no longer provided the same level of ecological and evolutionary goods and services (energy source, niche construction, ecosystem engineering, etc.) as the communities they replaced.
Traditionally, paleobotanists study plant fossil morphology to retrieve information regarding the fossils’ botanical affinity, to build more comprehensive phylogenies, to record changes in their communities, or use plant remains as biostratigraphic markers. In the last few decades, also the value of plant fossils as a proxy for palaeoenvironmental conditions has become widely recognized (e.g., Royer et al., 2005; Lomax et al., 2008; Peppe et al., 2018). Few studies, however, utilize the plant fossil record as a source of information for the functioning and dynamics of entire biotic systems, and to understand the macroecological and macroevolutionary force they represent. This is despite the fact that plants form the trophic basis of all terrestrial ecosystems, are prominent niche constructors, and generally comprise critical ecosystem engineers (Lawton, 1994). Understanding the processes and ultimate causes of periods of mass extinction is such a matter of macroevolutionary and macroecological importance and of great interest to the scientific community at large and beyond. To achieve this understanding, patterns of extinction—as recorded in the fossil record—are studied to give us clues about the actual nature and sequence of such events. However, many of the lineages that are disappearing are victims of secondary extinction as a result of collapsing ecosystem structure and functioning. It seems safe to assume that, the greater the dependence of a species on layer upon layer of lower trophic levels, the smaller the change that its proximal cause of extinction is relatable to the ultimate cause(s) of the crisis. For this reason alone, autotrophs—which per definition lack the same level of trophic dependence on other ecosystem members—constitute a much better model for the exploration of terrestrial crises and mechanisms for heightened extinction rates than other biota.
Terrestrial ecosystems were profoundly altered by the end-Permian biotic crisis (e.g., Grauvogel-Stamm and Ash, 2005; Roopnarine et al., 2019; Mays et al., 2020). Other than the rapid mass extinction in the marine realm, the destabilization of plant communities was less detrimental in terms of taxonomic loss (Nowak et al., 2019), and extended well into the Triassic (for overview, see: Schneebeli-Hermann et al., 2015). However, because of the temporal, spatial, geographical and environmental incompleteness of the terrestrial fossil records, most aspects of the environmental, ecological and phylogenetic dimensions and the dynamics during these times of terrestrial turmoil remain unclear. Consequently, consensus of scientific interpretations seems far beyond the horizon, and is mired in contention and controversy. This leads to seemingly diametrically opposite conclusions, for example illustrated by the following two publication titles—recently published in the same issue of Nature Communications: “No mass extinction for land plants at the Permian–Triassic transition” (Nowak et al., 2019) and “Age and pattern of the southern high-latitude continental end-Permian extinction constrained by multiproxy analysis” (Fielding et al., 2019). Such paradoxes often go beyond the semantics of what exactly constitutes a “mass extinction” or the possibility that described extinctions may be merely regional extirpations in a world with latitudinally shifting biomes. Nevertheless, many of the existing, seemingly contradictory interpretations may hold true, as they speak of slightly different or non-overlapping aspects of the events, or incongruently apply to different temporal or spatial scales.
One of confounding factors is that there are many ways to measure or express the impact of diversity decline during times of biosphere crisis, e.g., as illustrated in Erwin's metrics for the loss of evolutionary history (Erwin, 2008). When it comes to the dieback of land plants during the terrestrial Permo-Triassic crisis, it may be true that taxonomic and phylogenetic diversity decline was less severe compared to that of the marine invertebrates (Nowak et al., 2019). However, as we hope to show here, the loss of functional diversity (niches) and architectural diversity (ecospace) must have been severe. This becomes evident when we zoom out for a minute, and forget about all the precise temporal, taxonomic, or geographic details. In all floral provinces around the globe (Utting and Piasecki, 1995) (sub)dominant clades of autogenic ecosystem engineers—which formed the very structure and the trophic base of their biomes—went extinct sometime around or after the Permo-Triassic transition. In equatorial Euramerica, evidence for walchian conifers was last recorded in late Permian sediments (e.g., Poort et al., 1997). In Cathaysia, the seed fern order Gigantopteridales and the arborescent lycophytes (Lepidodendrales) had their last fossil appearances (Zhang et al., 2016; Feng et al., 2020). In the mid to high northern latitudes of (Sub)Angara, the coniferophyte Cordaitales went extinct (e.g., Dobruskina, 1994). And finally, the ecologically most successful Gondwanan seedfern order Glossopteridales, which dominated large parts of the mid-to-high latitudes in the southern hemisphere, disappeared (e.g., Retallack, 1997; Michaelsen, 2002; for an overview of these plant groups, see: Taylor et al., 2009). From this alone it is clear that—irrespective of extinction semantics and details of when exactly what transpired—the impact on the terrestrial biosphere must have been great.
Remarkably, across the planet micro- and megafossil records show that during pulses of environmental perturbation, when dominance of the taxa mentioned above declined, the isoëtalean lycophytes flourished (e.g., Ouyang and Utting, 1990; Wang, 1996; Looy et al., 2001; Lindström and McLoughlin, 2007; Grauvogel-Stamm and Ash, 2005; McLoughlin et al., 2015; Hermann et al., 2012). One of the best-known regions with early Mesozoic’s isoëtalean-dominated floras is the European Zechstein Basin. There, many Early Triassic macrofossil floras were swamped by a single species: Pleuromeia sternbergii (Münster) Corda. P. sternbergii was a sub-arborescent cormose lycophyte with an elongated unbranched stem (up to 2-m long) with helically arranged leaves, and a terminal heterosporous strobilus (Figure 1; Grauvogel-Stamm, 1999). In the last two centuries, many paleobotanical studies have featured morphological aspects of P. sternbergii fossils from the European Buntsandstein (e.g., Spieker, 1854; Solms-Laubach, 1899; Potonié, 1904; Mader, 1990; Grauvogel-Stamm, 1993; Grauvogel-Stamm, 1999; Fuchs et al., 1991, Grauvogel-Stamm and Lugardon, 2001; Grauvogel-Stamm and Lugardon, 2004). In an attempt to characterize the ecological success of this genus, Pleuromeia has previously been characterized as having a “weedy” lifestyle (e.g., Retallack, 1997; Erwin, 1998). However, this seems inconsistent with the very inconspicuous role that isoëtalean lycophytes played before the crisis or ever since. It is also inconsistent with the biology of extant isoëtalean lycophytes, which may share some life-history aspects with opportunists, but the rather informal characterization “weedy” offers little insight into the mechanics of their success. Here we will use P. sternbergii as a case study to better understand the significance of the world-wide presence of isoëtaleans. We tried to achieve this goal by aiming to answer the following consecutive questions: 1) Is the prevalence of isoëtaleans real? 2) What can we infer about the biology and ecology of these isoëtaleans and 3) what does this mean for the locations that documented such prevalence? Finally, 4) what clues does this provide for what may have happened during the terrestrial Permo-Triassic biosphere crisis?
FIGURE 1. Stem and leaf fossils of Pleuromeia sternbergii. Scale bars are 10 cm in (A), and 1 cm in (B–H). (A): Typical sandstone cast of a stem segment with the position of leaf traces preserved as depressions. (B,C): Compression fossil (part and counterpart) of the lowest portion of a strobilus with obovate sporophylls. (D): Sandstone cast of rhizomorph and stem revealing rare external leaf scar features. Visible as double vertical lines in each leaf scar are pairs of sclerenchymatous lamellae that accompany the vascular bundle inside the cortex. The white square highlights the part of the stem shown in (E,F). (E): Polymer cast of the same individual as in (D) but based on its fossil counterpart; PA is parichnos/aerenchyma, LC is leaf cushion. (F): Shape of the leaf base, as revealed by the leaf scars in (E); the position of the vascular bundle and accompanying sclerenchymatous lamellae (VB; visible in the specimen in (D)) is shown as overlay. (G): Leaf compression fossil with a clear rhomboid abscission surface. (H): Leaf fragment in which the position of collapsed air channels is visible as vertical furrows on either side of the leaf axis. See Material and Methods for museum specimen numbers.
In 2016, the Pteridophyte Phylogeny Group (PPG I, 2016) placed all extant lycophytes into the class Lycopodiopsida, which includes the orders Lycopodiales, Isoëtales and Selaginellales. This replaced classification schemes employing three classes (Lycopodiopsida, Isoëtopsida and Selaginellopsida) or two classes (Lycopodiopsida and Isoëtopsida—the latter including both Isoëtales and Selaginellales). The new single-class classification removed the necessity for a higher ranked taxon to contain the old classes. Extrapolating the new classification to the late Paleozoic, and in accordance with Pigg's classification of isoëtaleans (Pigg, 1992), we consider Pleuromeia to be a genus within the order Isoëtales in the class Lycopodiopsida, rather than a lineage in an order of its own, the Pleuromeiales, within the class Isoëtopsida. We interpret the Isoëtales sensu PPG I to contain the families Isoëtaceae (e.g., including the genera Lepacyclotes (=Annalepis), Isoëtes, Isoetites, Skilliostrobus, Tomiostrobus), the Pleuromeiaceae (holding the genera Cylomeia, Cylostrobus, Lycomeia, Lycostrobus, Mesenteriophyllum, Pleurocaulus, Pleuromeia and Takhtajanodoxa—among others) and the basal, sub-arborescent Chaloneriaceae (such as Chaloneria, Polysporia and Sporangiostrobus). Further support for this interpretation is lent by recently described finds of a new, well-preserved Pleuromeia species from Northeast China (P. shaolinii, Zhang et al., 2020) that confirmed the close relation of the genera Pleuromeia and Isoëtes earlier inferred by Pigg (1992).
The taxonomic division of Early Triassic isoëtaleans into genera is based on variations in stem shape and length, strobilus characteristics, and morphology of sporophylls, leaves, microspores and megaspores. Their size ranged from very small herbaceous species to subarborescent ones (e.g., Retallack, 1997; Grauvogel-Stamm and Lugardon, 2001; Kustatscher et al., 2015; Feng et al., 2020). What all isoëtaleans have in common is their spirally arranged succulent leaves, sporophylls, and roots on a corm or elongated trunk.
In the supplementary material of their publication on the quantitative analysis of the micro- and megafossil floras across the Permo-Triassic boundary, Nowak et al. (2019) included their extensive compilation of published fossil occurrences. We further explored these data sets in this study. First, in the microfossil data set, all known non-embryophytic palynomorphs (e.g., acritarchs, algal and fungal remains, foraminiferal linings, etc.) were removed. Next, for a detailed chronological analysis, we filtered both micro- and megafossil data sets for occurrences for which geochronological ages could be assigned (Wuchiapingian–Norian). For an epoch-level, paleogeographic comparison, we filtered the data for records, assignable to the Lopingian or Early Triassic, and binned data entries according to their paleogeographic floral province of origin (sensuUtting and Piasecki, 1995). In both analyses, we counted occurrences of isoëtaleans as a fraction of all recorded taxa for each stage or epoch×province.
To single out Isoëtales in the megafossil data compilation we filtered based on the following identifiers of isoëtalean taxa: Annalepis (= Lepacyclotes, see Kustatscher et al., 2015), Araucarias tomiensis (Meyen, 1982), Cidarophyton, Cyclodendron (when marked as Pleuromeiaceae), Cylomeia/Cyclomeia (invalidly published, see Cantrill and Webb, 1998), Cylostrobus (potentially misspelled as Cyclostrobus), Duckworthia, Helicorhiza, Isoëtes, Isoetites, Lepacyclotes (potentially misspelled as Lepacyclothes), Lepidanthium (when marked as Pleuromeiaceae), Leptocyclotes americana (= Lepacyclotes circularis, see Retallack, 1997), Lycomeia, Lycostrobus, Mesenteriophyllum (originally thought to be a gymnosperm leaf; see Bomfleur et al., 2011), Pleurocaulis, Pleuromeia, Skilliostrobus, Takhtajanodoxa, Tomiostrobus, Pleuromeiales and Isoëtales (for overview: Pigg, 1992; Skog and Hill, 1992; Retallack, 1997; Grauvogel-Stamm and Lugardon, 2001).
Micro- and megaspores filtered from the microfossil dataset are taxa that either have been found in situ in the sporangia of Pleuromeia (e.g., see Lugardon et al., 1999; Lugardon et al., 2000; Grauvogel-Stamm and Lugardon, 2004), other isoëtaleans, or have a wall ultrastructure that is comparable to those produced by isoëtalean or other rhizomorphic taxa. Selected microspores include Aratrisporites, Endosporites, Densoisporites, Lundbladispora, Uvaesporites and “cavate forms”, “trilete spores (cavate)”, and “spore tetrads” (Wang, 1991; Retallack, 1997; Cantrill and Webb, 1998; Lugardon et al., 1999; Grauvogel-Stamm and Lugardon, 2001, 2004; Naugolnykh and Zavialova, 2004; Looy et al., 2005; Kustatscher et al., 2010; Naugolnykh, 2012). Selected megaspores are Banksisporites, Dijkstraisporites, Horstisporites, Maiturisporites, Biharisporites, Cristatisporites, Nathorstisporites, Tenellisporites, Trileites and Zonalasporites (Wang, 1991; Skog and Hill, 1992; Balme, 1995; Retallack, 1997; Cantrill and Webb, 1998; Lugardon et al., 2000; Grauvogel-Stamm and Lugardon, 2004; Kustatscher et al., 2010; Kustatscher et al., 2015).
The studied and imaged material comprised a large collection sandstone casts of Pleuromeia sternbergii stems, compression fossils of strobili, sporangia and leaves (e.g., the specimens used for Figure 1 and Supplementary Figure S1). Most specimens are housed at the Museum für Naturkunde, Humboldt-University, Berlin, and several stem casts are housed at the Naturalis Biodiversity Center, Leiden. The specimens from Thuringia and Saxony-Anhalt in Germany originate from Middle Buntsandstein localities at Singen and Gösselborn, and Upper Buntsandstein localities at Bernburg an der Saale. Museum specimens were photographed using a Canon EOS 20D digital camera and a Canon EOS 28–70 2.8L lens. Images were stored as JPEG and RAW files. The Pleuromeia specimens imaged in Figure 1 are from the paleobotanical collections of the Museum für Naturkunde, Berlin (specimen numbers: A: MB. Pb. 1980/426; B: MB. Pb. 1980/429; C: MB. Pb. 1980/428; D-F: MB. Pb. 1984/307; G: MB. Pb. 2013/1849; H: MB. Pb. 2013/1763). The taxon P. sternbergii is conceptually reconstructed sensuBateman et al. (1992) on the basis of the descriptions of the studied specimens and published photographs (Mägdefrau, 1931; Mader, 1990; Fuchs et al., 1991; Grauvogel-Stamm, 1993; Grauvogel-Stamm, 1999). The internal structure is based on descriptions by Karl Mägdefrau (1931) and anatomically preserved stems of the Pleuromeia segregate Pleurocaulis rewanense Cantrill & Webb from the Bowen Basin, Australia (Cantrill and Webb, 1998).
Of the specimens in the museum collections and photographs in published Pleuromeia sternbergii studies, thirteen sandstone stem casts and two strobilus compressions were selected for growth rate analysis because they had clearly distinguishable sporophylls or leaf scars/-traces along large segments of the stem surface. The stems fossilized during various stages of decortication, resulting in a variation of parastichy surface patterns associated with the leaf scars and traces on the casts. Images of these specimens were used to count the cumulative number of leaf-/sporophyll scars and leaf traces vs. their height along the vertical axis of the stem segments. Since these scars and traces are often only visible on one side of the specimens, we counted one side and doubled their numbers as an approximation for the phyllotaxis of the full circumference. The ratio of the length of stem height intervals over the number of leaves in that interval (see Supplementary Figure S1) is a measure for vertical growth rate over that interval, expressed in mm growth per plastochron. A plastochron (Thomas, 1975) is the amount of time between successive moments of leaf or sporophyll initiation, thus the total number of plastochrons represents the functional age of a plant, based on morphological criteria. This works well in Pleuromeia, since it had a monojugate phyllotaxis—so all leaves and sporophylls were produced successively by the apical meristem in one generative spiral with an angle between successive leaves in apical view close to (3–√5)π (i.e., the golden angle).
Fifteen P. sternbergii specimens used for the line tracings and measurements shown in Supplementary Figure S1 (identified by lower case letters a–o) are based on published plates (a: Grauvogel-Stamm, 1999, Abb. 9; d: Mägdefrau, 1931, Tafel III-4; g: Mägdefrau, 1931, Tafel VI-4; m: Fuchs et al., 1991, Plate 3–4), specimens housed at the Museum für Naturkunde, Berlin (the museum specimen identifiers are as follows: b: MB. Pb. 1980/426; c: MB. Pb. 1980/428 and 429; e: MB. Pb. 2013/2,730; f: MB. Pb. 2014/0,477; h: MB. Pb. 2014/0,478; i: MB. Pb. 2002/1,064; k: MB. Pb. 2003/792; l: MB. Pb. 2013/2,728; n: MB. Pb. 1984/307; o: MB. Pb. 1983/429), and the Naturalis Biodiversity Center in Leiden (j: Naturalis RGM 13742).
Moving away from anecdotal evidence for the increased presence of isoëtaleans, we set out to quantify the proportion of isoëtalean taxonomic occurrences at stage level, based on fossil identifications in the literature as compiled by Nowak et al. (2019). They published two extensive literature based data compilations of published taxonomic occurrences that respectively included palynological records and plant megafossil records from around the world. Within the subset of all counted taxonomic occurrences in these data matrices (i.e., individual rows) that were also associated with stage-level chronostratigraphic information (∼10,000 megafloral taxonomic identifications and ∼45,000 plant palynomorph occurrences), we counted how many likely represented isoëtaleans (see Supplementary Table S1). Despite a likely over-representation of isoëtalean taxa in the window of preservation (see Section 4.5), their prevalence in the last two stages of the Paleozoic (Figure 2) was low (respectively 0.0% and 0.4% for the stage-level megafloral data; 0.8% and 2.5% in the microfloral record). As expected, isoëtalean taxonomic contribution was highest in the first two of the Mesozoic stages (respectively 16.5% and 12.5% for the megafloral data; 22.1 and 24.0% for the microfossil data). At the stage level, the frequency of isoëtaleans stayed relatively high throughout the Triassic in the microfossil data, but declined rapidly in the case of the megafossil record, in which they were no longer detected by the Norian. The Late Triassic observations come with the caveat that the paleofloral coverage with age-level dates in the younger part of the data sets is much lower. It drops to a total of 102 Norian and 0 Rhaetian embryophytic palynomorphs, and 116 Norian and 181 Rhaetian megafossil occurrences, compared with 4,090–13,599 microfossil and 607–2,892 megafossil occurrences for Early–Middle Triassic ages (Nowak et al., 2019).
FIGURE 2. Prevalence (in black) of isoëtalean taxa in micro- and megafossil records (from Lopingian and Triassic Stages until the Rhaetian) in the fossil data compilation by Nowak et al. (2019). The occurrence per Stage of isoëtalean taxa was counted among ∼45,000 published identifications of palynomorph in the data set (brown cubes) and in ∼10,000 recorded megafossil taxa (green cubes).
As can be suspected from merely glancing the Permo-Triassic plant literature, the signal described above—isoëtaleans occurring at inconspicuous back-ground levels in plant fossil records of later Permian, then rising to become important constituents in the Triassic—was a global phenomenon (Figure 3, Supplementary Table S2). The pattern holds whether we compare northern high latitudes with equatorial regions and the southern high latitude, whether we look at the disparate late Paleozoic phytogeographic floral provinces (as recognized by many authors, e.g., Utting and Piasecki, 1995; Cleal, 2016), or whether we compare the micro- and megafossil records. Moreover, Figure 3 confirms that—albeit at a coarse geographic scale—the Nowak et al. (2019) data compilation covers the various regions of Pangea quite evenly in the Lopingian and Early Triassic.
FIGURE 3. Paleogeographic view of isoëtalean prevalence among identified plant fossil taxa in the data set compiled by Nowak et al. (2019). Shown here is the data from the final epoch of the Paleozoic (Lopingian) and the first of the Mesozoic (Early Triassic) for the plant megafossil record (A) and the palynological microfossil record (B), divided in the four main floral provinces (Sub)Angara, Euramerica, Cathaysia and Gondwana (see map at the bottom; modified after the floral provinces in Utting and Piasecki (1995); paleogeography after Scotese (1997)).
In an attempt to better understand pleuromeiacean life-history, ecophysiology and ecology (see Section 4.2–4.5), we quantified aspects of their (functional) morphology, ontogeny and ecology based on European fossil occurrences, in addition to various traits already described in the literature.
Pleuromeia sternbergii is a medium-sized cormose lycopod (see our reconstruction in Figure 4A; details in Figure 5, Supplementary Figure S2) with an elongated unbranched stem and a terminal bisporangiate (heterosporous) strobilus (Figures 4A,B). The stem of P. sternbergii could reach a height of up to 2 m, but it is generally shorter. The thickness of stems is variable (Figure 4B); observed are specimens with a diameter of the basal zone of 22 cm (Mägdefrau, 1931). In specimens from the Museum für Naturkunde in Berlin, we observed the stems of Pleuromeia and its allies (e.g., Pleurocaulis; Cantrill and Webb, 1998) have a central exarch ribbed protostele in which the primary xylem is surrounded by a ring of metaxylem. Secondary xylem has not been observed, but it is possible that small amounts were deposited on the inside of the vascular cambium that surrounds the stele. A thick three-layered cortex surrounded the stele and cambium (Cantrill and Webb, 1998). It consists of an inner narrow parenchymatous layer (inner cortex), an aerenchymatous middle cortex and more dense parenchymatous outer cortex. In the vegetative region, single vascular bundles originated from the protoxylem, passed through the cortex zones—initially at a high angle—to extend into the leaves (Mägdefrau, 1931). Each vascular bundle is surrounded by parenchymatous cells, and supported by radially orientated aerenchymatous parichnos strands. The outer cortex was surrounded by a second meristematic layer; the cork cambium. This meristematic layer is the main one responsible for secondary growth of Pleuromeia stems by the production of “secondary” cortex (a somewhat imprecize term since ‘cortex’ is a zone, not a tissue type) on the inside, and cork cells on the outside.
FIGURE 4. Pleuromeia growth habit. (A): Whole plant reconstruction of an adult Pleuromeia sternbergii with terminal strobilus (by Ivo Duijnstee and Hannah Bonner). (B): Distribution of leaf scars and traces (lower-case letters correspond to curves in Supplementary Figure S1; see Material and Methods for plate numbers in published material and specimen numbers for fossils in museum collections). (C): Frequency distribution of estimated inverse growth rates (i.e. the relative time it takes for a certain amount of vertical growth) for basal, intermediate and apical stem segments as labeled in (B). Data is based on the vertical distance represented by 10 consecutive leaves along the stem (i.e., 10 plastochrons; the amount of time between successive leaf initiation moments; shown as green rectangles shown in Supplementary Figure S1. Numbers in parentheses indicate the number of 10-plastochron intervals used in this frequency data summary). Minimum and maximum values are shown; the boxes indicate the median and interquartile range and the green circle give the position of the mean. Intermediate segments show a wide range of growth rates, reflecting growth spurts in some segments.
FIGURE 5. Comparison of phyllotaxis in the lower basal (left) and intermediate to apical (right) part of our Pleuromeia sternbergii whole plant reconstruction in Figure 4A.
The young stem surface was covered with broad helically arranged rhomboidal leaf cushions (Figures 1D–F), the density of which varied greatly along the stem (Figure 5). Succulent leaves that were triangular at the base, and became more linear distally (Figures 1G,H), clasped the stem (Figures 1E,F). Depressions visible on both sides of, and parallel to the central vascular bundle (Figures 1G,H), represent the interaxial parichnos strands. When abscized, the elongated rhomboid leaf scars had long wavy lateral extensions, where the “wings” of the delta-shaped base of the leaf were attached to the stem (Figure 1F) and that interlocked in the densely foliated regions of the basal part of the stem (Figures 1F, 5). Well-preserved fossil specimens may have leaf scars that show scars of the centrally located vascular bundle and ligule groove, flanked by large scars of the two parichnos channels (Figures 1E,F). Pleuromeia did not have a leaf base retention mechanism. When the stem diameter increased due to extensive periderm production, the outermost corky layer ruptured and sloughed off the stem (decortication). Stems of larger specimens show leaf abscission zones with variations in leaf scar densities, and stem thickness (Figure 4B; e.g., Mader, 1990).
At the reproductive stage, a bisporangiate cone was borne terminally on the unbranched stem; oval to obovate sporophylls with small, narrow bases, were helically arranged around the axis (Figures 1B,C). Pleuromeia sternbergii’s megaspores and microspores have been found in situ and can be assigned to the dispersed micro- and megaspore taxa Densoisporites nejburgii and Trileites polonicus, respectively (Grauvogel-Stamm and Lugardon, 2004). The sporoderm ultrastructure of these in situ spores strongly resembles those of extant Isoëtes.
Since Pleuromeia sternbergii had a monojugate phyllotaxis, the apical meristem produced one leaf-/sporophyll primordium at a time. As such, the consecutive leaf scars on stem fossils can be read as a developmental clock, with plastochrons as the unit of time—being the time between development of successive leaf primordia (Thomas, 1975). Reconstructing the leaf density in different vertical intervals along the stem (illustrated in Figure 5), allowed us to measure relative vertical growth rates, expressed as height increase per plastochron, or—inversely—how much time a specimen spent in various life stages. Although the variation of growth rates varies between specimens (Figures 4B,C), typically P. sternbergii individuals spent a long time as a short, foliated corm. On average in our set of fossil specimens, the lower basal part (the first 10 cm of growth) lasted just over 108 plastochrons (Figure 4C); however, at the lowest recorded growth rate a mere cm of growth took as much as 35 plastochrons. After this stage, growth accelerated and was highest as P. sternbergii approached the reproductive life stage, after which it slowed down once again during formation of the terminal strobilus. The specimens we studied demonstrated capacity for short bursts of rapid pre-reproductive growth—in its extremest form gaining more than 20 cm within 10 plastochrons (specimen ‘e’ in Figure 4B and Supplementary Figure S1).
In a compilation of 65 megafossil floras from the Early Triassic part (∼252–247 million years ago; see Bourquin et al., 2006) of the Middle Buntsandstein in Germany made by Mader (1990), almost two-thirds of the floras contained remains of Pleuromeia sternbergii (from 93% of the floras in the lowest Lower Triassic unit, gradually declining to 27% in the upper unit; Figure 6A). Of the Pleuromeia-bearing floras, 78% consisted of Pleuromeia remains only (Figure 6C). Furthermore, while most of the common taxa in these floras within the Buntsandstein data set occurred in assemblages with an average of 2-5 accompanying taxa (on average 3.2), the mean number of associates for Pleuromeia was about 0.6 (Figure 6B).
FIGURE 6. Composition of 65 Early Triassic megafossil floras from the Buntsandstein (mottled sandstones) of Western Europe (data compiled by Mader (1990)). (A): Presence of four major plant groups in floras in four consecutive middle Buntsandstein units (B1-B4, from older to younger), covering the Early Triassic (∼252–247 million years ago; see Bourquin et al., 2006). (B): Mean number of associates of each of the nine most prominent Buntsandstein taxa (i.e., the average of species diversities of each megafossil assemblages that contained the indicated species minus one). The numbers inside the bars indicate the number of localities in the data set in which the species was present. (C): The percentage of all fossil assemblages with the indicated species in the data set that were monotypic floras.
Latest Permian and Early Triassic palynological assemblages from around the world record the pulsed collapse of gymnosperm-dominated plant communities and concomitant increases of isoëtalean spores (e.g., Looy et al., 2001; Lindström and McLoughlin, 2007; Hermann et al., 2012; Schneebeli-Hermann et al., 2015). These palynological records indicate that in many places in the Early-Middle Triassic lycophyte-dominated communities existed, sometimes lasting for millions of years. They ranged from (sub)tropical Euramerica (Europe; e.g., Orłowska-Zwolińska, 1984; Looy et al., 1999) and Cathaysia (southern Tibet, Liu et al., 2020), and northern high-latitude Subangara (Barents Sea; Hochuli and Vigran, 2010), to southern mid-latitude Gondwana (Pakistan, Hermann et al., 2011; India, Tewari et al., 2015; Mishra et al., 2018), to high latitude, southernmost Gondwana (Prince Charles Mountains, Antarctica; Lindström and McLoughlin, 2007; East Australia, Mays et al., 2020). In the lower temporal resolution typical for the discontinuous megafossil record, a megafloral analysis by Grauvogel-Stamm and Ash (2005) revealed a similar pattern. Early-Middle Triassic macrofossil assemblages include the sub-arborescent Pleuromeia (Figure 2A) and its segregates, and the more Isoëtes-like, short-statured Tomiostrobus, Lepacyclotes (=Annalepis), Skilliostrobus and Isoetites (e.g., Retallack, 1997; Grauvogel-Stamm and Ash, 2005; Vajda and McLoughlin, 2007; Kustatcher et al., 2010; Hermann et al., 2011; Feng et al., 2020; Zhang et al., 2020). The partial, sometimes temporary, replacement of dominant flora province endemics with more cosmopolitan taxa resulted in a loss of floral provinciality—at the very least in the range of environments that is preserved in the fossil record. The long reign of isoëtalean lycophytes such as Pleuromeia (sensu lato) is remarkable, if only for the modest role they played in pre-crisis plant communities (Figures 2, 3).
The fossils of Pleuromeia and segregates such as Pleurocaulis and Mesenteriophyllum, as well as the more Isoëtes-like Isoëtales such as Tomiostrobus and Lepacyclotes, reveal a particular syndrome of morphological traits associated with well-studied (semi-)aquatic extant plants with a so-called isoetid growth form (Den Hartog and Segal, 1964; Raven et al., 1988). “Isoetids” are slow-growing perennial plants with thick leaves or sporophylls (generally with low epidermal gas permeability), arranged in a rosette around a reduced stem or corm, and with comparatively large root systems. They have an interconnected network of gas-filled channels and/or aerenchymatous tissues throughout their leaves, stem/corm and root system (for an overview, see Keeley, 1998; Madsen et al., 2002). Stem, root and leaf fossils of the Early Triassic Pleuromeiaceae and Isoëtaceae, as well as other Paleozoic and Mesozoic Isoëtales, shared these characteristics with modern isoetids (Grauvogel-Stamm, 1993; Keeley, 1998; McLoughlin et al., 2015).
The isoetid growth form arose homoplastically across many plant lineages, including lycophytes (e.g., Isoëtes spp.), monocot (e.g., Eriocaulon aquaticum) and dicot angiosperms (e.g., Littorella uniflora, Lobelia dortmanna) (e.g., see Madsen et al., 2002). The unusual suite of morphological traits is so consistent across such distantly related clades because it is the functional morphological manifestation of a particular ecophysiological strategy that enables plants to live potentially submerged, and in nutrient- and/or carbon-limited wetland environments. When submerged (and in quite a few cases even when growing terrestrially), they rely on hydrosoil CO2 taken up by roots for primary production, as well as internally recycled CO2 (Nygaard, 1958; Richardson et al., 1984; Raven et al., 1988; Madsen et al., 2002; Smolders et al., 2002; Green, 2010). Conversely, O2 produced by their photosynthetic organs diffuses downward and leaves the plant via the root system, where it helps to oxidize and detoxify the rhizospheric sediment, and remineralize organic matter.
Isoetid plant species can (in many cases facultatively) sequester and concentrate inorganic carbon—taken up by leaves and/or roots to be used in daytime photosynthesis—by temporarily converting it into organic acids and store it in large vacuoles. This metabolic pathway is the aquatic version of the carbon-concentrating mechanism known as Crassulacean Acid Metabolism (a.k.a. aquatic CAM, or aquatic acid metabolism (AAM); for overview, e.g., see: Raven et al., 1988; Raven et al., 2008; Keeley, 1998). Just like the isoetid growth form, CAM has evolved independently in many tracheophyte clades. Much of the isoetid functional morphology (e.g., succulent, aerenchymatous leaves) has evolved in interdependent conjunction with the CAM capability. In general, when mapping CAM in plants beyond isoetids alone, we see that it is especially prevalent among succulent, xerophytic plants (opening stomata only at night to take in, and store carbon—thus limiting daytime water loss) and in submerged aquatic plants (where overnight carbon sequestration helps with carbon starvation due to low diffusion rates in water). CAM thus enables plants to grow in stressful (i.e., biomass-formation constraining; Grime, 1979) habitats such as carbon-starved, flooded, or extremely dry environments. The flipside, however, is that employing CAM is energetically costly and severely curtails maximum photosynthetic rates—irrespective of the already lower productivity caused by the environmental stress (and thus even when environmental stressors are removed). As such, utilization of CAM implies poor competitiveness. This is illustrated by extant aquatic CAM plants in oligotrophic lakes, whose combined above-ground standing biomass is on average three orders of magnitude smaller than that of non-CAM aquatics in eutrophic lakes (Sculthorpe, 1967; Wetzel, 1975; see; Keeley, 1998).
Thinking of inorganic carbon budgets in arborescent lycophytes, Green (2010) proposed to differentiate between the contributions of carbon stored as organic acids in CAM, and gaseous CO2 accumulated in their extended internal lacunal systems. The latter he dubbed Lycopod Photosynthetic Pathway, or LPP. Although one may wonder to what extent LPP contributed to the biomass of giant arborescent lycophytes in Carboniferous peat swamps, LPP can be a significant contributor to the growth of small, slow-growing plant taxa. We would argue that specifically the few extant isoetid species in which CAM has not been demonstrated, may rely on LPP for daytime photosynthesis, utilizing recycled and hydrosoil CO2 taken up by roots 24 h a day.
Supporting evidence that—just as in their extant counterparts—the isoetid morphological syndrome of Mesozoic isoëtaleans was associated with root-uptake of CO2 and CAM and/or LPP physiology, came from exceptionally well-preserved pleuromeian leaves, Mesenteriophyllum, from the Upper Triassic of the Transantarctic Mountains (Bomfleur et al., 2011), and the Middle to Upper Triassic Madygen Lagerstätte from Kyrgyzstan in central Asia (Moisan and Voigt, 2013). It is quite common for isoetid macrophytes using CAM to lack functional stomata when completely submerged (e.g., Rascio, 2002). This is generally not the case when they live terrestrially, though: then they tend to change from CAM to C3 photosynthesis and develop leaves with normal, functioning stomata. However, in some cases—notably all within Isoëtes—plant species rarely produce stomata, even under aerial conditions (e.g., Isoëtes palmeri, I. lechleri and I. karsteni; Keeley, 1998), or have never shown to produce stomata at all (e.g., I. triquetra and I. andina; Keeley et al., 1984; Raven and Spicer, 1996). All of these taxa commonly live in submerged habitats, so one may argue that the absent capability of stomata production is merely a lack of adaptation to emergence. However, the fully terrestrial Isoëtes andicola also lacks stomata, irrespective of environmental conditions (Keeley et al., 1984). Not surprisingly, physiological experiments with terrestrial I. andicola specimens have shown that more than 95% of inorganic carbon used in photosynthesis was taken up via the roots (Keeley et al., 1984).
In the only two instances that we are aware of that Mesenteriophyllum leaves were preserved with epidermal features intact (M. serratum, Bomfleur et al., 2011 and M. kotschnevii, Moisan and Voigt, 2013), both lacked stomata altogether, just like Isoëtes andicola. This demonstrates that at least some of the terrestrial, or at best littoral Mesenteriophyllum-bearing Pleuromeiaceae, effectively lacked direct atmospheric gas exchange. These plants therefore must have relied on internal CO2 for photosynthesis, taken up from the groundwater by their rootlets—just as suggested by their isoetid-like functional morphology.
Given the low diffusion rates of dissolved inorganic carbon in groundwater compared to CO2 from the atmosphere, the lack of stomata and below-ground carbon use confirms low rates of primary productivity, while it does not necessarily indicate that the leaves were formed under water. Just as in modern isoetids, this suite of productivity-curtailing ecophysiological adaptations comes at the price—again—of abominably low competitiveness. This may sound like a doomed strategy, but it is actually an exaptive, stress-tolerating superpower when facing conditions that don’t allow for high primary productivity in the first place.
Besides from the Middle-Upper Triassic, Mesenteriophyllum is also known from the Lower Triassic of Taimyr in north-central Russia (Sadovnikov, 2008). There the specimens are found in association with other fossil lycophyte organs (i.e., Pleuromeia and Tundrodenron), but unfortunately epidermal features were not observed. In addition to the observed astomatousness of pleuromeians, this unusual trait was also found in the leaves of closely related isoëtalean fossils, e.g., Paurodendron (McLoughlin et al., 2015), Isoetites madygensis and Isoetites sixteliae (Moisan and Voigt, 2013), although in these short-statured Isoëtes-like taxa a fully aquatic lifestyle cannot be ruled out.
Stems of (semi-)adult Pleuromeia sternbergii specimens show variations in thickness and leaf scar density (Figure 4B). Their roots were durable and firmly rooted in the substrate, as shown by in situ findings (Fuchs et al., 1991; Grauvogel-Stamm, 1993). Evidently, these plants were able to withstand severe environmental changes. The robust construction and signs of resilience, as well as their considerable size while being slow growing, are evidence of their longevity. It is hard to estimate an average reproductive age for P. sternbergii, but it is clear the plants were perennials and, given their inferred low productivity (see Section 4.2), their longevity may have been quite considerable, and the duration of an average plastochron much longer than in most other embryophytes. Their terminal strobilus is indicative of semelparity: one single reproductive event, followed by death of the plant.
Earlier we concluded that pleuromeian isoëtaleans were slow growing plants. Yet, our leaf scar analysis (Figure 4C and Supplementary Figure S1, specimen ‘e’) indicated a capability of bursts of (relatively) rapid growth close to the end of their life span. This paradox is solved in the light of a facultative “bolt and reproduce” strategy in the last stage of Pleuromeia’s life cycle. After a long life of limited growth, slowly saved-up resources are expended in one well-timed reproductive effort. This strategy exists in several extant plant taxa, for instance in the silversword Argyroxiphium sandwicense, a specialist of habitats that rival those of Pleuromeia in terms of extreme stress and lack of competition (Robichaux et al., 1990). A. sandwicense is endemic to the barren alpine deserts of the Haleakalā and Mauna Kea volcanoes, on the islands of Maui and Hawai'i respectively. Rosettes of these monocarpic plants are generally long-lived (up to 50 years), and, prior to reproduction, they grow slowly (Walker and Powell, 1999) up to a diameter of 75 cm (U.S. Fish and Wildlife Service, 1993). At some point, within just a few weeks, A. sandwicense is capable of producing a sometimes meters-long inflorescence for reproduction, after which the plant dies. Pleuromeia also seems to have had the capability to modify its vertical growth rate prior to reproduction. This suggests some degree of control (i.e., postponement or expedition) over the moment of reproduction. This life-history strategy would have given Pleuromeia an edge over many other Paleozoic plants (particularly large arborescent ones that must survive through programmed adolescence), especially in pulsed stress scenarios that may have contributed to the ecological disasters starting at the end of the Permian.
Spores can be divided into those with, and those without chloroplasts (Dyer, 1994; Gabriel y Galán and Prada, 2010). Chlorophyllous spores have a high water content, are not capable of anabiosis, and lose their viability within a short period of time (e.g., for Equisetum within weeks; Lloyd and Klekowski, 1970; Dyer, 1994; Lebkuecher, 1997). Non-chlorophyllous spores tend to have a low water content, are rich in carbohydrates and lipids and are photodormant (Gabriel y Galán and Prada, 2010). Spores of lycophytes belong to the last group. It is quite normal for Lycopodium spores, for instance, to not germinate within the first 6 or 7 years of shedding (Whittier, 1998). Spores of extant isoëtaleans have been shown to stay viable for at least 100 years in lake beds (J. G. M. Roelofs, pers. comm.). A consequence of these persistent spore banks is that long-dead plants can still produce offspring (Dyer, 1994). Given that all three extant orders in the class Lycopodiopsida have spores capable of long lasting dormancy (Whittier, 1998; Oh et al., 2013), it is highly probable that early Triassic Pleuromeia and other coeval Isoëtales also formed persistent spore banks. Moreover, a strong resemblance between micro- and macrospore ultrastructure of Pleuromeia and extant Isoëtes confirms the taxonomic kinship between the two genera (Lugardon et al., 1999, 2000; Grauvogel-Stamm and Lugardon, 2004; Looy et al., 2005). If Pleuromeia and its allies indeed would have lacked the necessity to continuously go through the sporophyte or gametophyte stages, this would have lent their populations a profoundly increased chance of survival during periods with very stressful conditions, even if these periods lasted for many consecutive years or even decades.
In addition to our inferences based on plant functional morphology and comparative ecophysiology, the depositional environments in which Pleuromeia species are preserved (eg, Mader, 1990; Grauvogel-Stamm and Ash, 2005)—along with observations from the range of habitats of their living relatives (mostly seasonal pools; inorganic-carbon-poor, oligotrophic, softwater lakes at high latitudes or, at high elevation in lower latitudes; infertile acidic tropical lakes; e.g., Keeley, 1998)—all indicates that these Isoëtales had poor competitive abilities, but a high stress-tolerance capacity. It has been noted that other isoëtalean lycophytes with growth habits similar to Pleuromeia, such as the Carboniferous Chaloneria, also lived in habitats characterized by abiotic stress, such as oligotrophy, acidity and periodic floodings (Pigg, 1992). Occupation of such niches probably dates back to the Upper Devonian (Chitaley and Pigg, 1996).
Based on field studies and experiments, also modern isoetids such as Isoëtes are thought to be restricted to mostly oligotrophic environments because of competitive displacement, and are outcompeted and disappear during eutrophication (Keeley, 1998). In the marginalized environments they occupy, they may reach very high densities, but always with low standing biomass and low productivity rates (Madsen et al., 2002; see Keeley, 1998 for overview). In accordance with this competition-avoiding, stress-tolerating lifestyle, as shown in autochthonous fossil assemblages and in situ preservations, Pleuromeia and its allies often formed monotypic or monogeneric stands (e.g., Pleuromeia rossica (Naugolnykh, 2013); Tomiostrobus sinensis (Feng et al., 2020); P. jiaochengensis (Wang, 1996); monogeneric association of three Pleuromeia species, Meng, 1996; monogeneric association of three Annalepis (=Lepacyclotes) species, Meng, 1996; Figure 6), or were only accompanied by one or two other taxa (e.g., Pleuromeia sternbergii, Fuchs et al., 1991; Moisan and Voigt, 2013 and P. epicharis (Wang, 1996)). Extant Isoëtes may also cover three-fourth or more of shallow lake-bottoms (Keeley, 1998).
Paleoecological, sedimentological and marine biogeochemical lines of evidence show strong evidence for widespread and increased soil erosion following the end-Permian biotic crisis (Newell et al., 1999; Sephton et al., 2005). The floral turn-over may have been partly responsible for this change in sedimentary regime. Based on the sedimentary setting their fossils were found in, and based on homology with extant Isoëtes species, Pleuromeia and its allies were capable of growing in nutrient-poor, largely inorganic sediments. This would have facilitated colonization of erosional landscapes, while the lack of soils likely slowed down recolonization by more competitive taxa with greater nutrient demands.
The environmental stress(es) that triggered the end-Permian biotic crisis is frequently hypothesized to have had a significant atmospheric component (Visscher et al., 2004; Black et al., 2012). Hence, the capability of above-ground parts to be isolated from the atmosphere may have played a role in elevating the chance of survival of end-Permian and Early Triassic isoëtalean vegetation. Moreover, as we discussed earlier, it is likely that they used CAM and/or LPP just as their modern relatives do. This means that, in contrast with ‘normal’ C3 plants, their primary productivity would not have suffered from temperature-induced elevation of photorespiration (Pedersen et al., 2011) due to the global warming that started at the end of the Permian (Cui and Kump, 2015).
Interestingly, some adaptations of the isoetid growth form to cope with flooding may have doubled as exaptations for Isoëtales to survive severe water stress during periods of drought (i.e., virtually no transpiration due to a lack of stomata, CAM/LPP, extensive root system). In fact, many cases of the homoplastic occurrence of CAM in the plant world emerged in xeric plant lineages, most notably its namesake the Crassulaceae. Provided that there is little competition, Pleuromeia and allies might thus have been exapted for habitat crossing-over to the other end of the hydrologic spectrum (see Figures 7E,F). This was also suggested, for instance, by Pleuromeia remains in China that are believed to have grown in xeric instead of semi-aquatic localities (Wang and Wang, 1982; Wang, 1996). The reverse is known to have happened in some members of the succulent crassulacean plant lineage. Crassula aquatica, for example, utilizes its capacity for CAM to lead a newly adopted submerged aquatic lifestyle, instead of the xerophytic lifestyle in drought-prone habitats in which most of its close relatives live (Keeley and Morton, 1982).
FIGURE 7. Hypothetical niche space partitioning among vascular plants before and after abiotic and biotic environmental change (inspired by Jackson and Overpeck (2000)) and how it may be represented in the fossil record. (A–C): Gray shaded area is the theoretical environmental space—the hyperspace of all potential interactions between environmental factors, here simplified to two dimensions, defined by gradients along two environmental axes. Dotted lines indicate the fundamental niches of isoëtalean and other vascular plant groups (mostly euphyllophytes) within the full potential environmental space. The dark-grey-outlined rectangles are the subset of environmental combinations that are present in a region at a certain moment (i.e., the realized environmental space). Within the realized environmental space, we see the blue and green shaded areas that indicate the realized niches of isoëtaleans and other tracheophytes, respectively. Note that, because of their abysmal competitive abilities, in this model isoëtaleans do not occur where other plants flourish. Environments where fossilization occurs (mostly wet areas with active sedimentation; here the black-outlined rectangles) offer a geographically, environmentally and taxonomically very biased view of what must have been present. Due to their common association with wetlands, isoëtaleans are likely to be over-represented in the fossil record. (B): Abiotic perturbation: environmental change has altered the realized environmental space, and hence also the extent of the realized niches. The change in this example caused isoëtaleans to become more common on the landscape. Moreover, since areas of fossil preservation are to some extent geographically locked in place (e.g., in basinal areas), environmental conditions in the window of preservation have changed as well (which means this window moved to a different position in the environmental space, compared to its position in (A)), and therefore the fossil record is sampling a different set of plant individuals. (C): Biotic change: extirpation or shrinking populations of other tracheophytes opens up new realized niche space for isoëtaleans. As a result, they expand on the landscape, which is recorded as an increase of isoëtalean fossils by the window of preservation. Because of the feedback between the biosphere and abiotic environment, a combination of scenarios such as in (B,C) is unavoidable. (D): zooming in on the changing contribution of both plant groups to the fossil record under the different scenarios in (A–C). Besides competitive marginalization of isoëtaleans to opposite corners of the realized environmental space (see the isoëtalean realized niche in (A)), due to their peculiar functional morphology and physiology (e.g., isoetid growth form and CAM) they may also have been exapted for an existence in diametrically opposite environments (e.g., flooding-prone and drought-prone realized niches of Triassic isoëtaleans). Accordingly, the shape of their fundamental niche in environmental (hyper)space may well look (hyper)dumbbell-shaped (F), rather than the ellipsoid (E), predicted by uni-modal optimum curves of plant abundances along environmental gradients.
The physiological and morphological adaptations of the isoetid growth form facilitate a life in stressed environments (e.g., being periodically submerged; lacking nutrients and/or inorganic carbon) where the competitive pressure is low. As a result of this rather specialized lifestyle, their growth rates are very low (e.g., Smolders et al., 2002), or, as Boston et al. (1989) conclude: “The stress-tolerant growth strategy of the plants in these carbon-poor, nutrient-poor habitats dictates low annual production”. At the same time, the reverse is probably also true. Isoetids can live in stressful conditions (i.e., that constrain biomass production—which is not much of a problem if your natural rate of primary productivity is low to begin with), but consequently they are poor competitors in less stressed environments (sensuGrime, 1979). The morphology of Pleuromeia and its allies is consistent with the isoetid adaptations of slow growing stress-tolerating competition-avoiders. Combining substantial dormancy capabilities to survive ephemeral bad times (Section 4.3), with uniquely being able to afford a lifestyle of very low productivity for prolonged periods (Section 4.2)—lasting many generations without a severe drop in population fitness—may be the main secret to isoëtalean success during the Permo-Triassic. This produces the somewhat paradoxical situation that some of the traits that make isoëtaleans poor competitors and that condemns them to marginalized habitats are also the very traits that help explain their success during environmental crises, and even lent them the qualification by some of being “weedy”. Perhaps confusingly, the different contexts may introduce seemingly contradictory terminologies for essentially the same traits: e.g., “poor growth rates” may be a “major disadvantage” in times of plenty when competing taxa thrive, while at the same time a “capability for slowed or postponed growth” may be an “advantageous adaptation” to survive environmental stress—a capability that the competition may lack.
In the previous sections we have highlighted the stress-tolerating abilities of Pleuromeia and its allies. In the wake of the end-Permian mass extinction, the prolonged phases of worldwide success of these isoëtalean stress tolerators suggest that the same high level of global stress persisted throughout these phases of isoëtalean fossil proliferation. However, palynological records across the Permo-Triassic transition show a more differentiated picture. The pulsed terrestrial ecosystem collapse was characterized by dominance of isoëtalean microspores (i.e., Densoisporites and Lundbladispora; e.g., see Looy et al., 2001; Lindström and McLoughlin, 2007; Liu et al., 2020), but in many cases the observed peaks in lycophyte spore abundance were unusual in that the spores predominantly occurred as unseparated tetrads instead of single spores. These tetrads are interpreted as deviations in sporogenesis, triggered by high levels of environmental stress (Visscher et al., 2004; Looy et al., 2005), and are thus considered a proxy for the peak-levels of environmental stress which caused the pulsed collapse of terrestrial ecosystems. Since the duration of the spore-tetrad events is much shorter than the prolonged successive phases of isoëtalen proliferation, we think that global environmental stress levels throughout much of the Triassic may have been substantially less radical than during confined tetrad peak events. Nevertheless, they may have been high enough to frustrate recovery and re-radiation of more competitive plant clades into the environments in which the Isoëtales thrived.
Adopting a life-history strategy as stress tolerator by no means indicates that a species prefers or even requires stressful conditions. In fact, ecological experiments have shown that many stress tolerators under-perform in their natural habitat and would thrive in stress-free environments if they were not excluded from those habitats because of biotic interactions—most notably competition (Figures 7A,C). As a result, stress tolerators’ realized niches are confined to sub-optimal, yet available margins of their fundamental niche (Figure 7A; Jackson and Overpeck, 2000). We attribute the ubiquitousness of isoëtaleans in the Early Triassic mainly to the expansion of their realized niche, made possible by the prolonged absence of stronger competitors (Figure 7C), as well as the new global abiotic reality (Figure 7B), rather than to adaptive radiation of the clade, as previously suggested (e.g., Retallack, 1997). Their invasion into new environments without obvious new adaptations—including expansion into habitats with a higher productivity potential—thus provides indirect evidence of the absence of competitors. We conclude that the dominance of isoëtaleans such as Pleuromeia reflects a widespread and lasting lack in the recovery potential of more competitive plant clades that were dominant before the crisis. The associated lack of plant diversity in the many isoëtalean-dominated landscapes, combined with the inherent low isoëtalen productivity must have had serious ecological implications up the food chain (see Section 4.7).
For a meaningful interpretation of the results in our study, we must address some prominent taphonomic biases, as they shape the data that were used in our analyses. Fossilization of plant parts predominantly takes place in perhumid to wet sub-humid climates (sensuCecil, 2003) in depositional environments such as lakes, rivers, shallow marine basins, or soils with high water tables such as peats or flood plains (Figure 7A; Gastaldo and Demko, 2011). Fossil plant assemblages will only be conserved if the sediments they are part of are not eroded away over time. Because of this, the chance of retrieving fossil evidence from other environments than lowland deposits is much smaller. As such, plants that grow in, upstream from, or close to these basinal environments, have a much greater chance of being preserved in the sedimentary record (Burnham et al., 1992; Figure 7D). As a result, we have a good idea how plant communities changed over time in the wetter parts of the late Paleozoic and early Mesozoic landscape—and by proxy the environmental conditions they represent—but less information from the local drier habitats, and very little information on the communities of plants and their evolution in the extra-basinal environments, although, unfortunately, that is where many major evolutionary innovations took place (Looy et al., 2014). In addition to habitat filtering, the chance of plant structures being incorporated in the fossil record is also largely dependent on life-history aspects of the plant as well as morphological and chemical aspects of the various plant parts. Larger plants that produce and shed copious amounts of biomass, e.g., in the form of leaves and spores, or that produce decay-resistant structures, stand a better chance of being preserved in high enough numbers to eventually become detected and recorded by paleobotanists (e.g., Spicer, 1989; Greenwood, 1991).
Changes in taphonomic regimes in the Early Triassic may well have preferentially aided the preservation of isoëtaleans in the megafossil record. Although this might be true, in itself this cannot explain the unusual worldwide ecological occurrence of isoëtaleans, which is a prerequisite for their global fossil prevalence. In other words, before isoëtaleans can be preserved (preferentially) in sediments around the world, they must first have occurred in such environments—and therefore also the exceptional ecological conditions that allow for their abundant presence. Furthermore, the fact that we observe the same pattern in different biomes worldwide and in both megafossil and microfossil records, lends confidence to the conclusion that the isoëtalean prevalence, although potentially taphonomically amplified, in itself is not a preservational artifact and that the associated inferred biotic crisis extended well outside the window of megafossil preservation.
Epoch-level data compilations, such as presented in Figure 3, convincingly demonstrate worldwide isoëtalean proliferation in the Early Triassic (at the very least within the environmental window of fossil preservation). However, the observed 18.2% isoëtaleans among all recorded megafossil taxa—25.1% even for microfossil record—still only offers a watered-down, time-averaged signal of the heydays of global isoëtalean success. The reason is that the Early Triassic lasted just over 4.7 Ma, while we know from detailed palynological data from stratigraphic (composite) sections, that the greatest lycophyte proliferation occurred during much shorter intervals (e.g., Schneebeli-Hermann et al., 2015). Therefore, the ecological importance conveyed by the isoëtalean dominance is far greater than the time-averaged percentages in Figures 2, 3 suggest.
Over the last few decades, a body of literature has been published that addresses possible causes of the end-Permian terrestrial biotic crisis (for overview, see e.g., Erwin, 2006). In their search for mechanisms behind severe biosphere upheavals, paleoscientists all too often put emphasis on “kill mechanisms” in the most literal sense: causing mass-mortality among organisms (e.g., see Van de Schootbrugge and Wignall, 2016). However, mass extinctions do not concern themselves with longevity of individuals, but with the termination of evolutionary lineages at rates much higher than they originate. And although killing the majority of individuals in populations of many species could in theory be a mechanism of achieving anomalously high extinction rates, we would argue that in practice it never really is. Such scale dissonance (in the case of the example individual-level causes of lineage-level problems) is a pervasive problem in deep-time paleosciences. The temporal and spatial scales at which we observe the results of Earth system phenomena in the fossil record, tend to be out of tune with the unknown processes that drive them. As a result, it is difficult for us to find the right scale at which to study what explains our observations. Similarly, because the basis for extinction-type biotic crises is inherently ecological, we aim to study their intricacies at the ecosystem level. However, the outcome we might call a mass extinction is an evolutionary biological crisis, so instead of just studying disruption of ecological interactions using methods from neo-ecology, we need research tools that additionally fit the longer, multiple-generation timescales.
A way of looking at the isoëtalean prevalence (and what it may imply for the state of the terrestrial biosphere) that goes beyond neo-ecological scales, is through the concept of Biological Common Goods. This concept, borrowed from economic theory, was adapted by Erwin and collaborators (Erwin and Valentine, 2013; Erwin, 2015; McInerney and Erwin, 2017) to explain economic principles in paleobiology; e.g., of ecological services such as niche construction and ecosystem engineering, which affect rate and scale of evolutionary paleobiological events. This conceptual model ordinates different kinds of “goods” along two axes that determine the availability of goods: one axis of increasing excludability of the goods, and the other of increasing rivalry in the competition for the goods (see Figure 8A for examples). Public goods are those that are both non-excludable and non-rivalrous, and therefore always available to all. Analogous to economic public goods, McInerney and Erwin (2017) argue that public biological goods “are widespread in biology, including genes that are widely shared among ‘prokaryotes’, oxygen generated by oxygenic photosynthesis, transposable elements and the provision of ecological services via ecosystem engineering and niche construction”, and that public biological goods are the currency for the interactions between environmental, ecologic and genetic change, critical in the generation of major evolutionary transitions (Erwin, 2015).
FIGURE 8. The concept of economic goods applied to the Permo-Triassic terrestrial biosphere crisis. (A) different types of goods that play different roles in a system can be ordered based on their level of excludability and rivalrousness (examples are given of public goods, club goods and private goods). In economic systems, the role different goods play in a system, how much can be used and by whom changes depending on these two different availability modifiers. Similarly, in evolutionary biological systems, differently accessible types of biological goods and services shape major evolutionary transitions (see Erwin, 2015). (B) Land plants provide a host biological goods in evolving terrestrial biological systems (the center of gravity of this range in two contrasting scenarios is illustrated by the two white dots). Due to the Permo-Triassic biotic crisis and associated floristic change (which is the topic of this paper) the landscape of accessible biological goods for the evolving terrestrial biosphere changed drastically, especially the reduction of public biological goods (driving the center of gravity of goods toward private goods). See Section 4.7 for further discussion.
The importance of biological goods that vascular plants provide for the terrestrial ecosystems can hardly be overstated. Their primary productivity exclusively provides the energy that fuels all terrestrial life. As autogenic ecosystem engineers, their structural, physical and chemical alterations of the environment provide many services and creates, maintains or changes a myriad of habitats, occupied by other terrestrial organisms—from rhizosphere and soils to canopy, and from carbon sequestration to altered precipitation patterns.
In many latest Permian ecosystems, high, pre-crisis plant diversity would have allowed for a relatively high non-excludability of crucial goods such as niche construction, ecosystem engineering and organic energy sources—distributed directly or via many herbivorous and decompositional pathways. At the same time, the level of pre-crisis primary productivity and its spatial extent of vegetated landscapes, would have produced intermediate levels of rivalry for the services plants provide. In other words, most of the time the many evolutionary and ecosystem services that plants provide, are almost available like public goods, punctuated by the occasional Tragedy of the Commons (see in this context also Roopnarine and Angielczyk (2012) on the role of Tragedy of the Commons in explaining the rise and fall of species in their dynamic trophic model of various Permo-Triassic South African vertebrate communities). As argued earlier, the global proliferation of isoëtaleans implies both low primary productivity and a drop in a- and ß-diversity (at least within the window of preservation), and, as such, indicates a crash in the range and volume of biological goods, as well as a shift in availability. Lower diversity means fewer constructed niches, less ecosystem engineering and a narrowed trophic base—accessible to a smaller group of heterotrophs—resulting in increased excludability for the remaining set of goods (Figure 8B). Concomitantly, lowered primary productivity must have limited the supply of goods, thus increasing the rivalry for the few remaining services.
Based on the part of the terrestrial biosphere that can be observed in the plant fossil record, ecosystems at the end of the Permian and the beginning of the Triassic were characterized by a drastic shortage of biological goods, and especially public goods. As Erwin (2015) makes his conceptual case that abundant public biological goods is the driver of major evolutionary transitions, we would argue that the reverse is also true: a lack of public biological goods caused the major terrestrial evolutionary regression we observe at the beginning of the Mesozoic.
In general, the plant fossil record is poorly suited for analysis of the Permo-Triassic crisis in the way mass extinctions are commonly studied since the advance of quantitative paleontology, i.e., estimating global taxonomic diversity change and the diverging origination and extinction rates that cause the change. The main caveats preventing this approach include:
(1) The coverage of the plant megafossil record, and to a lesser extent the microfossil record, is spatially and temporally sparse, discontinuous and very biased—both environmentally and taxonomically—thus presenting a poor proxy for global plant diversity.
(2) Taxa disappearing from such an imperfect fossil record may merely represent local or regional extirpation (especially during times of global environmental change, which results in latitudinal biome shifts).
(3) Permanent disappearance of larger plant clades from the fossil record does likely represent actual extinctions, but because of the quality of the fossil record, the exact timing and location of extinction is usually impossible to ascertain.
In addition to the severe limitations of the plant fossil record listed above, there are other reasons why the biotic crisis on land may benefit from a different analytical approach than commonly used in quantitative paleontology covering the marine invertebrate record:
(4) As Al Traverse (1988) pointed out more than three decades ago: plant evolution dances to a different beat. Two important aspects of plant biology make Paleozoic plant taxa quite resilient to extinction and thus the termination of higher taxonomic groups generally more significant than that of animal clades. First, the lack of a dependence on ecosystem services compared to, e.g., animals (i.e., no dependence on food web integrity or architecture; little dependence (yet) on animal vectors for pollen, spore or seed dispersal). Second, the existence of common long- and short-term dormancy capability at multiple life cycle stages (e.g., persistent spore- or seed banks, retreat in corms and rhizomes, deciduousness) and capacity for regeneration and regrowth.
(5) The structure and composition of the entire web of heterotrophic life on land is completely dependent on plants as ecosystem engineers and energy providers. In the marine realm there is no equivalent of one taxonomic group with such a profound impact on all other lifeforms.
We hope to have demonstrated that trying to compare or equate the end-Permian marine invertebrate mass extinction in terms of taxonomic diversity dynamics with the Permo-Triassic plant biotic crisis is not feasible and ignores the profound differences between marine heterotrophs and terrestrial autotrophs. In contrast, a wealth of ecological information on various aspects of biosphere perturbation can be gleaned from detailed analyses of the available records, as biased and limited as they may be in geographic or taxonomic scope, for instance:
(6) As said, the plant fossil record generally does not allow to pinpoint the exact timing or location of extinction. Nevertheless, the permanent disappearance of major ecosystem engineers (e.g., glossopterids) and the ecosystems they shaped and fueled, cannot be denied its obvious and vast ecological significance.
(7) Despite the skewed representation of the global terrestrial environments (which is biased toward wetlands and riparian habitats with active sedimentation), understanding the ecological significance of the worldwide prevalence of isoëtaleans reveals a global pattern of ecological deterioration. This global signal cannot be simply explained by environmental disruption as a result of rapid latitudinal shifts of climate zones, causing latitudinal migration and thus local extirpations.
The raw data supporting the conclusions of this article will be made available by the authors, without undue reservation.
CL conceived and conceptualized the project, imaged fossil specimens, was involved in data acquisition, data analysis, figure preparation and whole plant reconstruction, and shared responsibility for data interpretation and manuscript drafting. HK was involved in the data acquisition and interpretation, literature scanning and critical revision of the manuscript. ID imaged fossil specimens, led the data acquisition, data analyses, figure preparation and whole-plant reconstruction, and shared responsibility for data interpretation and manuscript drafting.
This study was supported by a Naturalis Biodiversity Center Temminck Fellowship to CL, and a University of California Museum of Paleontology travel grant to CL and ID.
The authors declare that the research was conducted in the absence of any commercial or financial relationships that could be construed as a potential conflict of interest.
We thank Henk Visscher, Lea Grauvogel-Stamm, Jeff Benca, Charles Marshall and Swee Peck Quek for fruitful discussions. Barbara Mohr provided access to Berlin’s fossil collections. We also thank two reviewers for their helpful feedback and constructive reviews of this manuscript. This is UCMP Contribution No. 3010.
The Supplementary Material for this article can be found online at: https://www.frontiersin.org/articles/10.3389/feart.2021.615370/full#supplementary-material.
Balme, B. E. (1995). Fossil in situ spores and pollen grains: an annotated catalogue. Rev. Palaeobot. Palynol. 87, 81–323. doi:10.1016/0034-6667(95)93235-x
Bateman, R. M., DiMichele, W. A., and Willard, D. A. (1992). Experimental Cladistic analysis of anatomically preserved arborescent lycopsids from the Carboniferous of Euramerica: an essay on paleobotanical phylogenetics. Ann. Mo. Bot. Gard. 79, 500–556.
Black, B. A., Elkins-Tanton, L. T., Rowe, M. C., and Peate, I. U. (2012). Magnitude and consequences of volatile release from the Siberian Traps. Earth Planet Sci. Lett. 317-318, 363–373. doi:10.1016/j.epsl.2011.12.001
Bomfleur, B., Krings, M., Taylor, E. L., and Taylor, T. N. (2011). Macrofossil evidence for pleuromeialean lycophytes from the Triassic of Antarctica. Acta Palaeontol. Pol. 56, 195–203. doi:10.4202/app.2010.0022
Boston, H. L., Adams, M. S., and Madsen, J. D. (1989). Photosynthetic strategies and productivity in aquatic systems. Aquat. Bot. 34, 25–57. doi:10.1016/0304-3770(89)90049-1
Bourquin, S., Peron, S., and Durand, M. (2006). Lower Triassic sequence stratigraphy of the western part of the Germanic Basin (west of Black Forest): fluvial system evolution through time and space. Sediment. Geol. 186, 187–211. doi:10.1016/j.sedgeo.2005.11.018
Burnham, R. J., Wing, S. L., and Parker, G. G. (1992). The reflection of deciduous forest communities in leaf litter: implications for autochthonous litter assemblages from the fossil record. Paleobiology. 18, 30–49. doi:10.1017/s0094837300012203
Cantrill, D. J., and Webb, J. A. (1998). Permineralized pleuromeid lycopod remains from the early Triassic Arcadia formation, Queensland, Australia. Rev. Palaeobot. Palynol. 102, 189–211. doi:10.1016/s0034-6667(98)80005-5
Cecil, C. B. (2003). “Concepts, models, and examples of climatic controls on sedimentation: introduction,” in Climate controls on stratigraphy. Editors C. B. Cecil, and N. Terrence, Tulsa, OK: SEPM Vol. 77, 13–20.
Chitaley, S., and Pigg, K. B. (1996). Clevelandodendron ohioensis, gen.et sp. nov., a slender upright lycopsid from the late Devonian Cleveland Shale of Ohio. Am. J. Bot. 83, 781–789. doi:10.1002/j.1537-2197.1996.tb12767.x
Cleal, C. J. (2016). A global review of Permian macrofloral biostratigraphical schemes. Geol. Soc. London Spec. Publ. 450, 349–364. doi:10.1144/sp450.4
Cui, Y., and Kump, L. R. (2015). Global warming and the end-Permian extinction event: proxy and modeling perspectives. Earth Sci. Rev. 149, 5–22. doi:10.1016/j.earscirev.2014.04.007
Den Hartog, C., and Segal, S. (1964). A new classification of the water-plant communities. Acta Bot. Neerl. 13, 367–393. doi:10.1111/j.1438-8677.1964.tb00163.x
Dyer, A. F. (1994). Natural soil spore banks–can they be used to retrieve lost ferns?. Biodivers. Conserv. 3, 160–175. doi:10.1007/bf02291886
Erwin, D. H. (1998). The end and the beginning: recoveries from mass extinctions. Trends Ecol. Evol. 13, 344–349. doi:10.1016/s0169-5347(98)01436-0
Erwin, D. H. (2008). Colloquium paper: extinction as the loss of evolutionary history. Proc. Natl. Acad. Sci. U.S.A. 105, 11520–11527. doi:10.1073/pnas.0801913105
Erwin, D. H. (2006). Extinction: how life nearly ended 250 million years ago. Princeton, NJ: Princeton University Press.
Erwin, D. H. (2015). A public goods approach to major evolutionary innovations. Geobiology. 13, 308–315. doi:10.1111/gbi.12137
Erwin, D. H., and Valentine, J. W. (2013). The Cambrian Explosion: the construction of animal biodiversity. Greenwood, CO: Roberts & Co.
Feng, Z., Wei, H. B., Guo, Y., He, X. Y., Sui, Q., Zhou, Y., et al. (2020). From rainforest to herbland: new insights into land plant responses to the end-Permian mass extinction. Earth Sci. Rev. 204, 103153. doi:10.1016/j.earscirev.2020.103153
Fielding, C. R., Frank, T. D., McLoughlin, S., Vajda, V., Mays, C., Tevyaw, A. P., et al. (2019). Age and pattern of the southern high-latitude continental end-Permian extinction constrained by multiproxy analysis. Nat. Commun. 10, 385–412. doi:10.1038/s41467-018-07934-z
Fuchs, G., Grauvogel-Stamm, L., and Mader, D. (1991). Une remarquable flore à Pleuromeia et Anomopteris in situ du Buntsandstein moyen (Trias inférieur) de l’Eifel (R. F. Allemagne). Morphologie, paléoécologie et paléogéographie. Palaeontograph. Abteilung B. 222, 89–120.
Gabriel y Galán, J. M., and Prada, C. (2010). “Pteridophyte spores viability,” in Working with ferns: issues and applications. Editors A. Kumar, and H. Hernández (New York, NY: Springer), 193–206.
Gastaldo, R. A., and Demko, T. M. (2011). “The relationship between continental landscape evolution and the plant-fossil record: long term hydrologic controls on preservation,” in Taphonomy: Process and bias through time. Editors P. A. Allison, and D. J. Bottjer (Dordrecht: Springer), 249–285.
Grauvogel-Stamm, L., and Ash, S. R. (2005). Recovery of the Triassic land flora from the end-Permian life crisis. Comptes Rendus Palevol. 4, 593–608. doi:10.1016/j.crpv.2005.07.002
Grauvogel-Stamm, L., and Lugardon, B. (2001). The Triassic lycopsids Pleuromeia and Annalepis: relationships, evolution, and origin. Am. Fern J. 91, 115–149. doi:10.1640/0002-8444(2001)091[0115:ttlpaa]2.0.co;2
Grauvogel-Stamm, L., and Lugardon, B. (2004). The spores of the Triassic lycopod Pleuromeia sternbergii (Münster) Corda: morphology, ultrastructure, phylogenetic implications and chronostratigraphic inferences. Int. J. Plant Sci. 165, 631–650. doi:10.1086/386562
Grauvogel-Stamm, L. (1993). Pleuromeia sternbergii (Münster) Corda from the Lower Triassic of Germany-further observations and comparative morphology of its rooting organ. Rev. Palaeobot. Palynol. 77, 185–212. doi:10.1016/0034-6667(93)90004-e
Grauvogel-Stamm, L. (1999). “Pleuromeia sternbergii (Münster) Corda, eine charakteristische Pflanze des Deutschen Buntsandsteins,” in Trias - eine ganz andere Welt. Editors N. Hauschke, and V. Wilde (München: Verlag Dr. Friedrich Pfeil), 271–282.
Green, W. A. (2010). The function of the aerenchyma in arborescent lycopods: evidence of an unfamiliar metabolic strategy. Proc. Biol. Sci. 277, 2257–2267. doi:10.1098/rspb.2010.0224
Greenwood, D. R. (1991). “The taphonomy of plant macrofossils,” in The processes of fossilization. Editor S. K. Donovan (New York, NY: Columbia University Press), 141–169.
Hermann, E., Hochuli, P. A., Bucher, H., Brühwiler, T., Hautmann, M., Ware, D., et al. (2011). Terrestrial ecosystems on North Gondwana following the end-Permian mass extinction. Gondwana Res. 20, 630–637. doi:10.1016/j.gr.2011.01.008
Hermann, E., Hochuli, P. A., Bucher, H., and Roohi, G. (2012). Uppermost Permian to Middle Triassic palynology of the Salt Range and Surghar Range, Pakistan. Rev. Palaeobot. Palynol. 169, 61–95. doi:10.1016/j.revpalbo.2011.10.004
Hochuli, P. A., and Vigran, J. O. (2010). Climate variations in the boreal Triassic - inferred from palynological records from the Barents Sea. Palaeogeogr. Palaeoclimatol. Palaeoecol. 290, 20–42. doi:10.1016/j.palaeo.2009.08.013
Jackson, S. T., and Overpeck, J. T. (2000). Responses of plant populations and communities to environmental changes of the late Quaternary. Paleobiology. 26, 194–220. doi:10.1017/s0094837300026932
Keeley, J. E. (1998). CAM photosynthesis in submerged aquatic plants. Bot. Rev. 64, 121–175. doi:10.1007/bf02856581
Keeley, J. E., and Morton, B. A. (1982). Distribution of diurnal acid metabolism in submerged aquatic plants outside the genus lsoëtes. Photosynthetica. 16, 546–553.
Keeley, J. E., Osmond, C. B., and Raven, J. A. (1984). Stylites, a vascular land plant without stomata absorbs CO2 via its roots. Nature. 310, 694–695. doi:10.1038/310694a0
Kustatscher, E., Wachtler, M., and Van Konijnenburg-van Cittert, J. H. A. (2010). Lycophytes from the Middle Triassic (Anisian) locality Kühwiesenkopf (Monte Prà della Vacca) in the Dolomites (northern Italy). Palaeontology. 53, 595–626. doi:10.1111/j.1475-4983.2010.00948.x
Kustatscher, E., Donà, H., and Krings, M. (2015). Sporophyll organization in the Triassic isoetalean lycopod Lepacyclotes (formerly Annalepis) zeilleri from Germany. Paläontol. Z. 89, 303–311. doi:10.1007/s12542-014-0246-0
Lebkuecher, J. G. (1997). Desiccation-time limits of photosynthetic recovery in Equisetum hyemale (Equisetaceae) spores. Am. J. Bot. 84, 792–797. doi:10.2307/2445815
Lindström, S., and McLoughlin, S. (2007). Synchronous palynofloristic extinction and recovery after the end-Permian event in the Prince Charles Mountains, Antarctica: implications for palynofloristic turnover across Gondwana. Rev. Palaeobot. Palynol. 145, 89–122. doi:10.1016/j.revpalbo.2006.09.002
Liu, F., Peng, H., Bomfleur, B., Kerp, H., Zhu, H., and Shen, S. (2020). Palynology and vegetation dynamics across the Permian–Triassic boundary in southern Tibet. Earth Sci. Rev. 209, 103278. doi:10.1016/j.earscirev.2020.103278
Lloyd, R. M., and Klekowski, E. J. (1970). Spore germination and viability in Pteridophyta: evolutionary significance of chlorophyllous spores. Biotropica. 2, 129–137. doi:10.2307/2989770
Lomax, B. H., Fraser, W. T., Sephton, M. A., Callaghan, T. V., Self, S., Harfoot, M., et al. (2008). Plant spore walls as a record of long-term changes in ultraviolet-B radiation. Nat. Geosci. 1, 592–596. doi:10.1038/ngeo278
Looy, C. V., Brugman, W. A., Dilcher, D. L., and Visscher, H. (1999). The delayed resurgence of equatorial forests after the Permian-Triassic ecologic crisis. Proc. Natl. Acad. Sci. U.S.A. 96, 13857–13862. doi:10.1073/pnas.96.24.13857
Looy, C. V., Twitchett, R. J., Dilcher, D. L., Van Konijnenburg-van Cittert, J. H., and Visscher, H. (2001). Life in the end-Permian dead zone. Proc. Natl. Acad. Sci. U.S.A. 98, 7879–7883. doi:10.1073/pnas.131218098
Looy, C. V., Collinson, M. E., Cittert, J. H. A. V. K. V., Visscher, H., and Brain, A. P. R. (2005). The Ultrastructure and botanical affinity of end‐Permian spore tetrads. Int. J. Plant Sci. 166, 875–887. doi:10.1086/431802
Looy, C. V., Kerp, H., Duijnstee, I. A. P., and DiMichele, W. A. (2014). The late Paleozoic ecological-evolutionary laboratory, a land-plant fossil record perspective. Sed. Record. 12 (4), 4–10. doi:10.2110/sedred.2014.4.4
Lugardon, B., Grauvogel-Stamm, L., and Dobruskina, I. (2000). Comparative Ultrastructure of the megaspores of the Triassic lycopod Pleuromeia rossica Neuburg. Comptes Rendus Acad. Sci. Ser. IIA Earth Planet. Sci. 330, 501–508. doi:10.1016/s1251-8050(00)00145-2
Lugardon, B., Grauvogel-Stamm, L., and Dobruskina, I. (1999). The microspores of Pleuromeia rossica Neuburg (Lycosidae; Triassic): comparative ultrastructure and phylogenetic implications. Comptes Rendus Acad. Sci. Ser. IIA Earth Planet. Sci. 329, 435–442. doi:10.1016/s1251-8050(00)80068-3
Mader, D. (1990). Palaeoecology of the flora in Buntsandstein and Keuper in the Triassic of middle Europe, volume 1 Buntsandstein. Stuttgart: Gustav Fischer Verlag.
Madsen, T. V., Olesen, B., and Bagger, J. (2002). Carbon acquisition and carbon dynamics by aquatic isoetids. Aquat. Bot. 73, 351–371. doi:10.1016/s0304-3770(02)00030-x
Mägdefrau, K. (1931). Zur Morphologie und phylogenetischen Bedeutung der fossilen Pflanzengattung Pleuromeia. Beih. Bot. Centralbl. 48, 119–145.
Mays, C., Vajda, V., Frank, T. D., Fielding, C. R., Nicoll, R. S., Tevyaw, A. P., et al. (2020). Refined Permian–Triassic floristic timeline reveals early collapse and delayed recovery of south polar terrestrial ecosystems. GSA Bull. 132 (7-8), 1489–1513. doi:10.1130/B35355.1
McInerney, J. O., and Erwin, D. H. (2017). The role of public goods in planetary evolution. Philos. Trans. A Math. Phys. Eng. Sci. 375, 20160359. doi:10.1098/rsta.2016.0359
McLoughlin, S., Drinnan, A. N., Slater, B. J., and Hilton, J. (2015). Picrodendron stellatum: a new Permian permineralized herbaceous lycopod from the Prince Charles Mountains, Antarctica. Rev. Palaeobot. Palynol. 220, 1–15. doi:10.1016/j.revpalbo.2015.04.004
Meng, F. (1996). Middle Triassic lycopods flora of South China and its palaeoecological significance. Palaeobotanist. 45, 334–343.
Meyen, S. V. (1982). Some true and alleged Permotriassic conifers of Siberia and Russian Platform and their alliance. Palaeobotanist. 28/29, 161–176.
Michaelsen, P. (2002). Mass extinction of peat-forming plants and the effect on fluvial styles across the Permian-Triassic boundary, northern Bowen Basin, Australia. Palaeogeogr. Palaeoclimatol. Palaeoecol. 179, 173–188. doi:10.1016/s0031-0182(01)00413-8
Mishra, S., Aggarwal, N., and Jha, N. (2018). Palaeoenvironmental change across the Permian-Triassic boundary inferred from palynomorph assemblages (Godavari Graben, south India). Palaeobiodivers. Palaeoenviron. 98, 177–204. doi:10.1007/s12549-017-0302-3
Moisan, P., and Voigt, S. (2013). Lycopods from the Madygen Lagerstätte (Middle to Late Triassic, Kyrgyzstan, Central Asia). Rev. Palaeobot. Palynol. 192, 42–64. doi:10.1016/j.revpalbo.2012.12.003
Naugolnykh, S. V. (2012). Sporophyll morphology and reconstruction of the heterosporous lycopod Tomiostrobus radiatus Neuburg emend. from the Lower Triassic of Siberia. Palaeobotanist. 61, 387–405.
Naugolnykh, S. V. (2013). The heterosporous lycopodiophyte Pleuromeia rossica Neuburg, 1960 from the Lower Triassic of the Volga River basin (Russia): organography and reconstruction according to the “whole-plant” concept. Wulfenia. 20, 1–16.
Naugolnykh, S. V., and Zavialova, N. E. (2004). Densoisporites polaznaensis sp. nov.: with comments on its relation to Viatcheslavia vorcutensis Zalessky. Palaeobotanist. 53, 21–33.
Newell, A. J., Tverdokhlebov, V. P., and Benton, M. J. (1999). Interplay of tectonics and climate on a transverse fluvial system, Upper Permian, Southern Uralian Foreland Basin, Russia. Sediment. Geol. 127, 11–29. doi:10.1016/s0037-0738(99)00009-3
Nowak, H., Schneebeli-Hermann, E., and Kustatscher, E. (2019). No mass extinction for land plants at the Permian-Triassic transition. Nat. Commun. 10 (1), 384–388. doi:10.1038/s41467-018-07945-w
Nygaard, G. (1958). On the productivity of the bottom vegetation in Lake Grane Langsø. SIL Proc. 13, 144–155. doi:10.1080/03680770.1956.11895394
Oh, M. J., Kim, C., Na, H. R., Shin, H., Liu, J. R., Choi, H.-K., et al. (2013). High frequency sporophytes regeneration from the spore culture of the endangered aquatic fern Isoëtes coreana. Am. J. Plant Sci. 4, 14–20. doi:10.4236/ajps.2013.46a003
Orłowska-Zwolińska, T. (1984). Palynostratigraphy of the Buntsandstein in sections of western Poland. Acta Palaeontol. Pol. 29, 161–194.
Ouyang, S., and Utting, J. (1990). Palynology of Upper Permian and Lower Triassic rocks, Meishan, Changxing County, Zhejiang Province, China. Rev. Palaeobot. Palynol. 66, 65–103.
Pedersen, O., Rich, S. M., Pulido, C., Cawthray, G. R., and Colmer, T. D. (2011). Crassulacean acid metabolism enhances underwater photosynthesis and diminishes photorespiration in the aquatic plant Isoetes australis. New Phytol. 190, 332–339. doi:10.1111/j.1469-8137.2010.03522.x
Peppe, D. J., Baumgartner, A., Flynn, A., and Blonder, B. (2018). “Reconstructing paleoclimatic and paleoecology using fossil leaves,” in Methods in paleoecology. Editors D. A. Croft, D. F. Su, and S. W. Simpson (Cham: Springer Nature), 289–317.
Pigg, K. B. (1992). Evolution of isoetalean lycopods, Ann. Mo. Bot. Gard. 79.(3) 589–612. doi:10.2307/2399754
Poort, R. J., Clement-Westerhof, J. A., Looy, C. V., and Visscher, H. (1997). Aspects of Permian paleobotany and palynology. XVII. Conifer extinction in Europe at the Permian-Triassic junction: morphology, ultrastructure and geographic/stratigraphic distribution of Nuskoisporites dulhuntyi (prepollen of Ortiseia, Walchiaceae). Rev. Palaeobot. Palynol. 97, 9–39. doi:10.1016/s0034-6667(96)00072-3
Potonié, H. (1904). Abbildungen und Beschreibungen fossiler Pflanzen-Reste der paleozoischen und mesozoischen Formationen. König. Preuss. Geol. Landesanst. Bergakad. Berlin. 38-39, 1–15.
PPG I (2016). A community‐derived classification for extant lycophytes and ferns. J. Systemat. Evol. 54, 563–603. doi:10.1111/jse.12229
Rascio, N. (2002). The underwater life of secondarily aquatic plants: some problems and solutions. Crit. Rev. Plant Sci. 21, 401–427. doi:10.1080/0735-260291044296
Raven, J. A., Handley, L. L., MacFarlane, J. J., McInroy, S., McKenzie, L., Richards, J. H., et al. (1988). The role of CO2 uptake by roots and CAM in the acquisition of inorganic C by plants of the isoetid life-form: a review, with new data on Eriocaulon decangulare L. New Phytol. 108, 125–148. doi:10.1111/j.1469-8137.1988.tb03690.x
Raven, J. A., Cockell, C. S., and De La Rocha, C. L. (2008). The evolution of inorganic carbon concentrating mechanisms in photosynthesis. Phil. Trans. R. Soc. B. 363, 2641–2650. doi:10.1098/rstb.2008.0020
Raven, J. A., and Spicer, R. A. (1996). “The evolution of crassulacean acid metabolism,” in Crassulacean acid metabolism. Editors K. Winter, and J. A. C. Smith (Berlin: Springer), 360–385.
Retallack, G. J. (1997). Earliest Triassic origin of Isoetes and quilwort evolutionary radiation. J. Paleontol. 71, 500–521. doi:10.1017/s0022336000039524
Richardson, K., Griffiths, H., Reed, M. L., Raven, J. A., and Griffiths, N. M. (1984). Inorganic carbon assimilation in the isoetids, Isoetes lacustris L. and Lobelia dortmanna L. Oecologia. 61, 115–121. doi:10.1007/BF00379096
Robichaux, R. H., Carr, G. D., Liebman, M., and Pearcy, R. W. (1990). Adaptive radiation of the Hawaiian silversword alliance (Compositae-Madiinae): ecological, morphological, and physiological diversity. Ann. Mo. Bot. Gard. 77, 64–72. doi:10.2307/2399626
Roopnarine, P. D., and Angielczyk, K. D. (2012). The evolutionary palaeoecology of species and the tragedy of the commons. Biol. Lett. 8, 147–150. doi:10.1098/rsbl.2011.0662
Roopnarine, P. D., Angielczyk, K. D., Weik, A., and Dineen, A. (2019). Ecological persistence, incumbency and reorganization in the karoo basin during the Permian–Triassic transition. Earth Sci. Rev. 189, 244–263. doi:10.1016/j.earscirev.2018.10.014
Royer, D. L., Wilf, P., Janesko, D. A., Kowalski, E. A., and Dilcher, D. L. (2005). Correlations of climate and plant ecology to leaf size and shape: potential proxies for the fossil record. Am. J. Bot. 92, 1141–1151. doi:10.3732/ajb.92.7.1141
Sadovnikov, G. N. (2008). On the global stratotype section and point of the Triassic base. Stratigr. Geol. Correl. 16, 31–46.
Schneebeli-Hermann, E., Kürschner, W. M., Kerp, H., Bomfleur, B., Hochuli, P. A., Bucher, H., et al. (2015). Vegetation history across the Permian–Triassic boundary in Pakistan (Amb section, Salt Range). Gondwana Res. 27, 911–924. doi:10.1016/j.gr.2013.11.007
Sephton, M. A., Looy, C. V., Brinkhuis, H., Wignall, P. B., De Leeuw, J. W., and Visscher, H. (2005). Catastrophic soil erosion during the end-Permian biotic crisis. Geology. 33, 941–944. doi:10.1130/g21784.1
Skog, J. E., and Hill, C. R. (1992). The Mesozoic herbaceous lycopods. Ann. Mo. Bot. Gard. 79, 648–675. doi:10.2307/2399758
Smolders, A. J. P., Lucassen, E. C. H. E. T., and Roelofs, J. G. M. (2002). The isoetid environment: biogeochemistry and threats. Aquat. Bot. 73, 325–350. doi:10.1016/s0304-3770(02)00029-3
Spicer, R. A. (1989). The formation and interpretation of plant fossil assemblages. Adv. Bot. Res. 16, 95–191. doi:10.1016/s0065-2296(08)60240-2
Spieker, T. (1854). Pleuromoia, eine neue fossile Pflanzengattung und ihre Arten, gebildet aus der Sigillaria sternbergi Münst. des bunten Sandsteins zu Bernburg. Z. Gesamte Naturwissenschaft. 3, 177–191.
Taylor, E. L., Taylor, T. N., and Krings, M. (2009). Paleobotany: the biology and evolution of fossil plants. Amsterdam: Academic Press.
Tewari, R., Awater, R., Pandita, S. K., McLoughlin, S., Agnihotri, D., Pillai, S. S. K., et al. (2015). The Permian–Triassic palynological transition in the Guryul Ravine section, Kashmir, India: implications for Tethyan–Gondwanan correlations. Earth Sci. Rev. 149, 53–66. doi:10.1016/j.earscirev.2014.08.018
Thomas, R. L. (1975). Orthostichy, parastichy and plastochrone ratio in a central theory of phyllotaxis. Ann. Bot. 39, 455–489. doi:10.1093/oxfordjournals.aob.a084960
Traverse, A. (1988). Plant evolution dances to a different beat. Plant and animal evolutionary mechanisms compared. Hist. Biol. 1, 277–356. doi:10.1080/08912968809386480
U.S. Fish and Wildlife Service (1993). Recovery plan for the Mauna Kea silversword (Argyroxiphium sandwiscence ssp. sandwicence). Portland, ER: U.S. Fish and Wildlife Service.
Utting, J., and Piasecki, S. (1995). “Palynology of the Permian of northern continents: a review,” in The Permian of northern Pangea. Editors P. A. Scholle, T. M. Peryt, and D. S. Ulmer-Scholle (Berlin: Springer), 236–261.
Vajda, V., and McLoughlin, S. (2007). Extinction and recovery patterns of the vegetation across the Cretaceous–Palaeogene boundary—a tool for unravelling the causes of the end-Permian mass-extinction. Rev. Palaeobot. Palynol. 144, 99–112. doi:10.1016/j.revpalbo.2005.09.007
Van de Schootbrugge, B., and Wignall, P. B. (2016). A tale of two extinctions: converging end-Permian and end-Triassic scenarios. Geol. Mag. 153, 332–354. doi:10.1017/s0016756815000643
Visscher, H., Looy, C. V., Collinson, M. E., Brinkhuis, H., Van Konijnenburg-van Cittert, J. H. A., Kürschner, W. M., et al. (2004). Environmental mutagenesis during the end-Permian ecological crisis. Proc. Natl. Acad. Sci. U.S.A. 101, 12952–12956. doi:10.1073/pnas.0404472101
Walker, L. R., and Powell, E. A. (1999). Regeneration of the Mauna Kea silversword Argyroxiphium sandwicense (Asteraceae) in Hawaii. Biol. Conserv. 89, 61–70. doi:10.1016/s0006-3207(98)00132-3
Wang, Z. (1991). Advances on the Permo-Triassic lycopods in North China I. An Isoetes from the mid-Triassic in Northern Shaanxi Province. Palaeontograph. Abteilung B. 222, 1–30.
Wang, Z. (1996). Recovery of vegetation from the terminal Permian mass extinction in North China. Rev. Palaeobot. Palynol. 9, 121–142.
Wang, Z., and Wang, L. (1982). A new species of the lycopod Pleuromeia from the Early Triassic of Shanxi, China, and its ecology. Paleontology. 25, 215–225.
Whittier, D. P. (1998). Germination of spores of the Lycopodiaceae in axenic culture. Am. Fern J. 88, 106–113. doi:10.2307/1547683
Zhang, H., Cao, C. Q., Liu, X. L., Mu, L., Zheng, Q. F., Liu, F., et al. (2016). The terrestrial end-Permian mass extinction in South China. Palaeogeogr. Palaeoclimatol. Palaeoecol. 448, 108–124. doi:10.1016/j.palaeo.2015.07.002
Keywords: Permo-Triassic, Isoëtales, Pleuromeia, life history, stress tolerator, ecology, mass extinction, ecophysiology
Citation: Looy CV, van Konijnenburg-van Cittert JHA and Duijnstee IAP (2021) Proliferation of Isoëtalean Lycophytes During the Permo-Triassic Biotic Crises: A Proxy for the State of the Terrestrial Biosphere. Front. Earth Sci. 9:615370. doi: 10.3389/feart.2021.615370
Received: 08 October 2020; Accepted: 21 January 2021;
Published: 02 March 2021.
Edited by:
Yukio Isozaki, The University of Tokyo, JapanReviewed by:
Graciela Helena Piñeiro, Universidad De La República, UruguayCopyright © 2021 Looy, van Konijnenburg-van Cittert and Duijnstee. This is an open-access article distributed under the terms of the Creative Commons Attribution License (CC BY). The use, distribution or reproduction in other forums is permitted, provided the original author(s) and the copyright owner(s) are credited and that the original publication in this journal is cited, in accordance with accepted academic practice. No use, distribution or reproduction is permitted which does not comply with these terms.
*Correspondence: Cindy V. Looy, bG9veUBiZXJrZWxleS5lZHU=
Disclaimer: All claims expressed in this article are solely those of the authors and do not necessarily represent those of their affiliated organizations, or those of the publisher, the editors and the reviewers. Any product that may be evaluated in this article or claim that may be made by its manufacturer is not guaranteed or endorsed by the publisher.
Research integrity at Frontiers
Learn more about the work of our research integrity team to safeguard the quality of each article we publish.