- 1Department of Earth and Environmetal Sciences, Ludwig Maximilian University of Munich, Munich, Germany
- 2National Museums of Kenya, Nairobi, Kenya
- 3Department of Geography, Ludwig Maximilian University of Munich, Munich, Germany
- 4UMR7154, Institut De Physique Du Globe De Paris (IPGP), Paris, France
Tectonically active regions are characterized by complex landscapes comprising soils with heterogeneous physicochemical properties. Spatial variability of nutrient sources enhances landscape biodiversity and creates heterogeneous habitats potentially attractive for animals and humans. In this study, we analyze the role of geological processes in the distributions of soil nutrients in the southern Kenya Rift, a key region in the interpretation of early human-landscape interactions. Our aim is to determine how spatial variations in rock chemistry, as well as topographic gradients and localized zones of rock fracturing from tectonic faulting determine the distributions of plant-available soil nutrients in soils. We hypothesize that present-day soil nutrient levels reflect the long-term chemical and geomorphological characteristics of the landscape and underlying parent material, and that regions with high nutrient availability occur along pathways correlating with locations of hominin fossil sites. Analyses of 91 topsoil samples from the main geological units show that Calcium (Ca) deficiencies predominately occur in shallow soils developed on trachytic volcanic rocks and granitic gneisses, while high Ca levels are associated with basaltic parent material and sedimentary deposits of mixed sources. XRF analysis of rock samples confirms that CaO levels in trachyte rocks are significantly lower than those in basalts, and Ca mobilization in basalt is more effective than in trachyte. Along two toposequences in densely faulted basaltic and trachytic rocks, we observed slope dependent soil nutritional gradients and a systematic increase of the concentrations of Ca, Mg and SOC in topsoils of colluvial sediments downslope of active normal faults. Known hominin sites in the region are located either along corridors of long-term Ca availability or at short-term nutrient hotspots potentially related to active CO2 degassing along active fault zones. This implies a strategic advantage of Ca-rich regions for hominin subsistence strategies, such as provision of predictable constraints on the distribution and mobility of grazing animals in complex tectonic landscapes. Our study implies that geological processes impact nutrient distributions in the southern Kenya Rift. Results of this study have further implications for understanding the role of soils in the interpretation of hominin-landscape interactions in the early stages of human evolution.
Introduction
Soils in landscapes characterized by tectonic and volcanic activity can exhibit a large variability in the distribution and availability of nutrients vital for plant growth and animal nutrition. Topographic complexity and lithological diversity leads to catchment-to-landscape-scale variations in soil nutritional characteristics. Knowledge on the long-term status of soil chemical properties can be gained by detailed and quantitative analysis of geological factors and processes at the rock-soil interface influencing the release and distribution of soil nutrients. While short-term variations in soil properties and vegetation dynamics are often associated with climatic factors, recent studies have shown that geological factors such as bedrock chemistry (Hahm et al., 2014) and topographic gradients (Weintraub et al., 2015; Chadwick and Asner, 2016; Calitri et al., 2019) play an important role in influencing the long-term nutritional fate of soils.
From a geological point of view, the morphological characteristics of a landscape, particularly hillslope steepness and related erosional and depositional processes, are important drivers in soils that can counteract the effect of nutrient depletion through chemical weathering, as enhanced erosion along steep slopes locally rejuvenates the soil parent material in downslope deposits through provision of freshly eroded rocks (Li and Lindstrom, 2001; Porder et al., 2005). This is of particular importance in high rainfall tropical and equatorial regions where long-term exposure (105–106 years) of stable land surfaces are prone to deep-seated weathering processes and the formation of nutrient-depleted lateritic soils (Tardy, 1997).
Stark contrasts between geochemical properties of neighboring rock units, e.g., the occurrence of ultramafic rocks in regions of magmatic rocks of intermediate geochemical characteristics, can lead to the formation of azonal vegetation cover and barren landscapes (Kruckeberg, 2004). But also more subtle geochemical variations can lead to noticeable changes in the soil nutritional status of a region, sometimes severe enough to impact the health of grazing animals (Maskall and Thornton, 1996).
In the East African Rift, volcanism has influenced greatly the nature of soils and the geochemistry of ground and surface waters (Maskall and Thornton, 1996; Davies, 2008). Strong heterogeneities in rock chemistry and intense tropical weathering result in variations in the availability of soil nutrients, with zones of severe nutrient deficiencies in contrast to zones of excessive nutrient levels that can both be critical for human and animal health. A general lack of rock-derived calcium in soils developed on acidic and intermediate volcanic rocks of the East African Rift restricts plant growth and leads to widespread deficiencies of this macronutrient in wildlife and livestock (Abate, 1988; Gachuiri et al., 2012). Long-term Ca-deficiencies manifest as nutritional diseases such as hypocalcemia or rickets in grazing animals (Maskall and Thornton, 1996; Davies, 2013). Deficiency of plant available phosphorus is also a widespread issue in East African soils, leading to limited plant growth and reduced reproductive rates in grazing animals (Howard, 1963; Abate, 1988; Margenot et al., 2016). The Lake Nakuru region in the central Kenya Rift Valley is located about 50 km north of our study region, and is well known for geochemically induced cattle diseases. In the ground and surface waters of this region, as well as in the Lake Baringo region, the Kerio river valley and in northern and central Tanzania, fluoride levels are up to 20 times higher than the upper levels defined by the World Health Organization (Gaciri and Davies, 1993; D'Alessandro, 2006; Ghiglieri et al., 2012; Gevera et al., 2019). As a result, dental and skeletal fluorosis are a widespread problem for humans and animals (Gaciri and Davies, 1993; Moturi et al., 2002; Davies, 2008). Also, cattle diseases related to nutrient deficiencies are reported for the Nakuru region (Maskall and Thornton, 1996). Low levels of copper and cobalt cause a cattle disease locally known as nakuruitis, that eventually leads to starvation of ruminants because their enteric bacteria need cobalt to process food (e.g., Howard, 1963). Indications of mineral deficiencies in East African pastures can indirectly be observed by the widespread phenomena of wildlife and livestock practising geophagia (eating of soils and sediments), particularly along exposed salt-rich lakebeds and volcanic ash deposits in the East African Rift (Davies, 2013).
Understanding the factors that determine variations in soil nutrient levels is important, not only for agricultural and livestock research, but also for other disciplines including ecology and paleoanthropology, which can exploit knowledge of the long-term distribution and availability of soil nutrients. This helps to better understand paleoecological conditions in regions of early human presence, and deduce regional-scale mobility patterns of animals and humans in past and present (Sturdy and Webley, 1988; McNaughton, 1990; Murray, 1995; Sturdy et al., 1997; Devès et al., 2014; Devès et al., 2015; Kübler et al., 2015; Kübler et al., 2016; Kübler et al., 2019; Eckmeier et al., 2020; Junginger and Kübler, 2020; Kübler et al., 2020).
Interactions between geological, topographic and pedological processes have rarely been considered, especially regarding the long-term soil nutritional status of soils at a regional scale. Further, the implementation of knowledge on local-to-regional scale variations on soil nutrients in archeological and paleoanthropological studies is lacking in most regions. Considering results of pedological and CRITICAL ZONE studies on the long-term influence of geological factors on soils in paleoanthropological research can improve interpretations related to how our ancestors have strategically exploited beneficial landscape elements and may further lead to new methods in discovering hominin fossil sites in other regions in the world.
The main objective in this study was to provide a geological perspective on the various drivers of soil nutrient distributions in a complex, tectonically active landscape with special focus on: 1) identifying spatial variations in soil chemical properties in the southern Kenya Rift, with emphasis on soil organic carbon (SOC), calcium (Ca), magnesium (Mg) and plant available phosphorus (P); 2) correlating soil nutrient levels with the chemistry of underlying volcanic and metamorphic rocks, and different sedimentary deposits; 3) correlating soil nutrient levels with topographic gradients and regions of erosion and deposition created by active extensional tectonics; 4) correlating potential soil nutritional corridors with the location of hominin sites in the study region.
Setting of the Study Region
Geology and Soils
The study region is located in the southern sector of the Kenya Rift, an integral part of the eastern part of the East African Rift System (Figure 1A), and extends from the Magadi trachyte plateau in the north to the southern shores of Lake Magadi in the south (Figure 1B). The region is characterized by extensive volcanism and associated tectonic activity from late Miocene to present. Pleistocene extensional tectonics have formed a complex system of grid faults in the rift center that hosts a series of tectonically controlled lake basins.
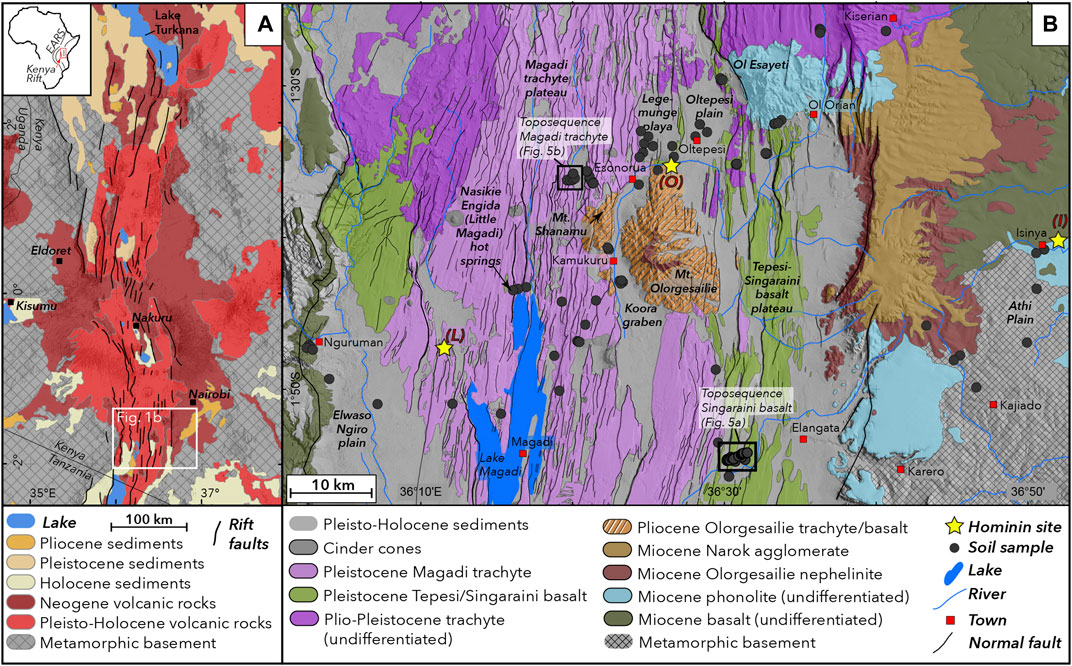
FIGURE 1. Geological setting of the study region. (A) Geological frameworkof the Kenya Rift depicting the main lithological and tectonic features. (B) Geological framework and sampling locations of the study region in the southern Kenya Rift (geological data from Guth et al., 2013). Black boxes depict locations of two toposequences shown in Figure 5; yellow stars indicate locations of hominin fossil sites: (I) Isinya, (L) Lainyamok, (O) Olorgesaile.
The oldest geological units are exposed at the uplifted rift shoulders at the eastern and western parts of the study area, comprising a series of Precambrian metamorphic basement rocks including granitic gneiss, quartzites and schists (Baker et al., 1971). Basement rocks are overlain by a series of Miocene basalt, phonolite and nephelinite (Figure 1B). The rift shoulders are characterized by the effects of long-term surface uplift and subtle tilting perpendicular from the rift axes. The Athi Plain on the eastern rift shoulder reflects long-term eastward tilt and forms a complex dendritic fluvial network oriented eastwards.
Mt. Olorgesailie, a prominent topographic feature located in the center of the rift valley, is a late Pliocene rift volcano (Baker et al., 1971) comprising trachytic, basaltic and nephelinitic lavas and pyroclastic deposits. The rift valley floor surrounding Mt Olorgesailie is extensively covered with Early to Mid Pleistocene lavas forming extensive plateaus, which resulted from widespread fissure eruptions of the Magadi trachyte (1.4–0.7 Ma BP: Baker et al. (1971); (Crossley, 1979)) in the north and west, as well as the Tepesi-Singaraini basalt plateau, comprising the Ol Tepesi (1.65–1.4 Ma BP), Ol Keju Nero (1.79–1.65) and Singaraini (2.31–2.33 Ma BP) olivine basalts in the eastern and southeastern segments of the study region (Baker and Mitchell, 1976). Due to their mineralogical similarities and for simplicity, we use in this study for the Pleistocene basalt series the combined term “Tepesi-Singaraini basalts”. The Mid Pleistocene volcanic units along with associated sedimentary deposits were subjected to complex and densely spaced extensional tectonic faulting in the Mid to Late Pleistocene (Figure 1B).
The most prominent sedimentary basins of the study area are 1) the Olorgesailie basin north of Mt. Olorgesailie comprising the alkaline Legemunge plain to the west and the Oltepesi alluvial plain to the east, 2) the Koora Graben west and south of Mt. Olorgesailie, 3) the Lake Magadi basin, and 4) the Ewaso Ngiro Plain (Figure 1B). Lake Magadi is characterized by high salinity, widespread exposures of trona deposits, and frequent water recharge by saline hot springs along the northwestern and southern lake shores. Normal faults in the Lake Magadi region are among the most active tectonic structures in the otherwise seismically relatively quiescent Kenya rift. Present seismicity in the region is characterized by high-frequency low-magnitude seismic swarms (Ibs-von Seht et al., 2001), and individual stronger events of magnitudes up to 4.2 accompanied by extensional surface fissures in the epicentral region (Atmaoui and Hollnack, 2003).
To describe soils in the study region (Supplementary Figure S1), we use the soil classification provided by the World reference base for Soil Resources (WRB, 2015). Playa deposits of the Lengemunge playa and alluvial sediments of the Oltepesi plain, are characterized by high soluble salt accumulations and are dominated by soil types haplic solonchak and associated haplic solonetz. Clay rich sediments in the Koora Graben comprise eutric cambisols and eutric fluvisols and associated chromic and pellic vertisols. Alluvial plain deposits in the Ewaso Ngiro plain and other smaller alluvial plains comprise predominantly eutric fluvisols and eutric cambisols. The densely faulted landscape of the Magadi plateau is characterized by an alternation of shallow soils comprising rhodic nitisols, and associated calcaric regosols and chromic luvisols along the fault ridges and steep slopes, and eutric cambisols on the alluvial deposits in the fault-bounded basins. The Singaraini-Tepesi basalt plateau comprises haplic luvisols and associated chromic luvisols along the fault ridges. Soils on basaltic rocks and associated alluvial deposits at the western rift shoulder predominately comprise gleyic solonchaks, eutric planosols, haplic phaeozems and associated haplic calcisols. The eastern rift shoulder is characterized by luvic phaeozem and associated vertic luvisols developed on Pliocene volcanic rocks, and eutric vertisols and chromic luvisols on metamorphic basement rocks (Touber, 1977).
Besides extensive soda ash mining in the Lake Magadi region, traditional Maasai livestock grazing is the main anthropogenic and agricultural activity in this region, which is otherwise only scarcely settled and influenced by human impact.
Topography, Climate and Vegetation
The landscape of the southern Kenya Rift is characterized by steep topographic gradients from the eastern and western rift shoulders situated above 1500 m asl to the rift center that lies at 600 m asl in the Lake Magadi basin. While the rift shoulders, particularly in the east, are dominated by wide and gently sloping plains and a generally low relief, the rift center is much more complex and characterized by a low-topography high-relief landscape as a result of densely spaced extensional faulting.
Climate in the study region can be classified between humid climate in regions above 2000 m, semi-arid to sub-humid climate in regions between 1000 and 2000 m asl, and arid climate in the low lying parts of the rift center below 1000 m asl (Köppen, 1900; Thornthwaite, 1948; Pratt et al., 1966). Major parts of the study region are characterized by aridity and seasonal large-scale rainfall patterns controlled by the East African monsoonal system and locally modified by topography (Vincens and Casanova, 1987). Lowest rainfall (<750 mm/yr) and highest mean potential evaporation (>2400 mm/yr) in the study region are observed in the Lake Magadi basin, leading to widespread exposure of evaporites and very sparse vegetation cover.
Regional scale vegetation patterns in the study area closely follow topographic and climatic gradients leading to widespread distribution of semi-desert shrubland in the low lying lake basins between 600–900 m asl, Acacia commiphora dominated woodland in widespread regions below 1500 m asl, and mosaic evergreen and semi-evergreen bushland in regions above 1500 m asl (Mworia et al., 1988). On a local scale, vegetation varies most significantly with hydrology and relief. In the densely faulted landscape of the plateau-like landscape dominated by Magadi trachyte and Tepesi-Singaraini basalt (Figure 1B), vegetation systematically varies with hillslope, and the associated distribution of colluvial and alluvial sediments. Uplifted surfaces on horst structures are dominated by open Acacia commiphora woodland, while alluvial plains next to active faults are dominated by open grassland frequently scattered with bushes of different species including Acacia melifera and Acacia tortilis. Vegetation density along colluvial deposits downslope of active fault scarps is systematically higher than on adjacent uplifted horst structures. Vegetation on colluvium comprises Acacia commiphora, densely interspersed with other shrubs and small trees including Grewia similis, Grewia bicolour, Balanicis egyptiaca, and Maerua sp. Steep fault scarps are dominated by bedrock exposure and scattered grass tussocks, as well as sporadic occurrence of Acacia melifera and Acacia tortilis (Mworia et al., 1988).
In recent years, the southern Kenya Rift is further affected by rapid invasion of Prosopis juliflora (Kyuma et al., 2016), an invasive subaerial plant species originating from Central and South America, which predominately occupies extensive regions on floodplain deposits in the Koora Graben, and along hillslopes in the Lake Magadi basin and Ewaso Ngiro plain.
Fossil Sites
The southern Kenya rift is a key region for studying Pleistocene hominin and archeological sites (Isaac, 1977; Potts, 1989; Behrensmeyer et al., 2002; Behrensmeyer et al., 2018). The most significant is Olorgesailie, located in diatomaceous lakebeds of the Legemunge Plain north of Mt. Olorgesailie. It is famous for its abundant Acheulean stone stools and fossil mammals spanning 1.2–0.5 Ma (Potts, 1989; Potts et al., 1999; Potts et al., 2004) and Middle Stone Age artifacts spanning 320 to ca. 36 ka–(Behrensmeyer et al., 2018; Deino et al., 2018). Other sites bearing evidence for Pleistocene hominins include the Isinya site (>0.98 Ma) on the eastern rift shoulder (Roche et al., 1988; Durkee and Brown, 2014), and Lainyamok (0.7–0.56 Ma) located north of Lake Magadi (Shipman et al., 1983). The latter site is interpreted as a locality of minor hominin activity (Potts et al., 1988).
Methods
We have carried out an integrated geopedological analysis in order to decipher the influence of lithology and topography on the nutrient status of soils in the southern Kenya Rift. We have used a combination of remote sensing and field-based analysis to identify rock and soil sample locations along a lithosequence comprising the main geological units exposed in the study area, which covers a 70 × 40 km large region, as well as two toposequences across the faulted Magadi trachyte and Tepesi-Singaraini basalt plateaus (Figure 1B). The main objective of field sampling and analysis was to determine the regional and local variations in physicochemical soil properties and their association with bedrock chemistry and topographic gradients created by tectonic activity. Soil transects were chosen to be located perpendicular to the strike of well-preserved normal faults vertically displacing volcanic bedrock. Fault erosion along the transects is dominated by gravity-driven slope processes forming colluvial deposits downslope of the fault exposures. We have mapped the geology and geomorphology in the vicinity of the transects and carefully chosen sample sites to avoid locations overprinted by the effects of local heterogeneities in sediment transport and nutrient redistribution due to e.g. alluvial processes, localized springs and groundwater seepage as well as local soil disturbances from burrowing animals.
Remote Sensing and Topographic Analysis
We have performed detailed topographic and optical analyses of satellite remote sensing datasets including high resolution digital elevation models of the TanDEM-X mission (Krieger et al., 2013), and multispectral Sentinel-2 datasets. We combined our observations with geological and pedological datasets of the region (Touber, 1977; Sombroek et al., 1982; Guth and Wood, 2013). The aim of the remote sensing work was to identify geological and geomorphological features that could potentially influence soil formation processes, such as tectonic fault scarps and volcanic deposits, as well as river networks, and local scale erosional features. Based on this analysis, we selected preliminary soil sampling sites on volcanic and meatamorphic bedrock, as well as on different sedimentary deposits, and the locations of two toposequences in Pleistocene volcanic rocks.
To analyze the relationship between vegetation growth and soil nutrient levels, we have produced a one-year average Normalized Differential Vegetation Index (NDVI) map of the study region from January 2017 to January 2018. To do so, we have selected one Sentinel-2 scene per month and calculated the mean NDVI using ArcGIS Raster Calculator (D’Allestro and Parente, 2015).
Rock Analysis
The objective of rock analysis was to identify weathering processes and related element mobilization in the volcanic rocks of the study region, and to compare the results with those of the analyzed soils developed on the selected rock types. We collected rock samples from several locations in the study region and along the Magadi and Tepesi-Singaraini transects and produced thin sections for polarized microscopic analysis as well as powder and glass beads for XRF analysis.
Polished thin sections were produced and analyzed by polarized light microscopy and scanning electron microscope (SEM). Microscopic pictures were taken by a Keyence Microscope (Mineralogische Staatssammlung München, SNSB), using a polarizing filter. Back scattered electron images (BSE) were taken by a Zeiss scanning electron microscope at the Department of Earth- and Environmental Sciences at the Ludwig Maximilian University Munich (LMU). WD-XRF analysis of the whole-rock composition were measured with a Philips, MagiX Pro WD-XRF spectrometer at the LMU, Department of Earth- and Environmental Sciences. Sample powders were dried at 110°C for more than 6 h and subsequently ignited at 1050°C for more than 2 h to determine the loss on ignition (LOI). Major and minor elements were measured using glass beads prepared by fusion of 1 g ignited sample powder and 9 g SPECTROMELT A12 (66% di-lithium tetraborate, 34% lithium metaborate) in a PANalytical Eagon 2 furnace fusion system. SO3 was measured by XRF analysis of powder tablets; these were prepared by mixing 8 g of the sample powder with 2 g of Merck Hoechst wax C micropowder (C38H76N2O2). Powder tablets were homogenized and pressed with p = 15 bar for >1 min. The calibration was done using international certified standards. Analytical quality was ensured by analyzing reference materials by the US Geological Survey as unknowns (Flanagan, 1969).
Soil Analysis
The main objective of soil analysis was to collect data on the variability and distribution of key soil nutrients in soils developed on the individual rock units and sedimentary deposits exposed in the study region. A sampling location usually consists of three to five soil samples. Composite topsoil samples were taken in a 5 × 5 m square from the uppermost 25 cm of a soil profile, to capture the portion of the soil profile most relevant for nutrient uptake by grassroots. In regions of deep soils additional sampling was carried out along depth profiles, and samples were taken from the individual soil horizons. Along a ∼60-km-wide lithosequence we sampled topsoil at 91 locations located on six different bedrock units and three different sedimentary units. To capture the effect of thermal activity and active CO2 degassing, three sample locations are in the vicinity of active hot springs at Nasikie Engida (Little Magadi: Figure 1B). We also sampled depth profiles at three locations on the Legemunge Plain, Oltepesi plain and a river terrace of the Ol Keju Nero river, respectively. To analyze the effect of topography and tectonics, we sampled 51 topsoils at 17 locations across a 3 km long toposequence in tectonically faulted Tepesi-Singaraini basalt, and 21 topsoils at 7 locations across a 750 m long toposequence in tectonically faulted Magadi trachyte. Originally, we intended to take a similar amount of samples along both toposequences, however, for security reasons, we had to prematurely terminate field work in the Magadi region.
Soil samples were tested for a wide range of macronutrients and trace elements. We will show here only the results for Ca, Mg, and plant available P, as previous studies carried out in the wider vicinity of the study region have documented deficiencies and related health problems for humans and animals in relation to those nutrients (McNaughton, 1990; Maskall and Thornton, 1996; Davies, 2013; Kübler et al., 2015; Kübler et al., 2019).
Soil analysis was carried out by the soil chemistry laboratories of the Kenyan National Agricultural and Livestock Research Organization (KALRO-Kabete) and the National Museums of Kenya. Before analysis, samples were air dried and sieved through a 2 mm sieve. Organic carbon was oxidized with concentrated H2SO4 and K2CrO7 and determined calorimetrically (Anderson and Ingram, 1993). Available P was determined using the method by Olsen (1954); Exchangeable cations were extracted using 1N ammonium acetate at pH 7.0, followed by flame photometry for the determination of Na, K, Mg and Ca, using an A2 flow analyser. Determinations of low, moderate and high nutrient levels follow recommendations by Shand (2007) and Hengl et al. (2017).
Results
Tectonic Geomorphology of the Study Region
The study region is characterized by the typical geomorphological features of an asymmetric continental rift system with uplifted and slightly tilted rift flanks away from the rift axes along the Athi Plains to the east and Nguruman escarpment to the west (Figure 1B) (Baker and Mitchell, 1976; Birt et al., 1997; Chorowicz, 2005). Extensional tectonics are manifested in large N-S trending border faults at the eastern and western rift shoulder, and a more complex system of N-S and NNE-SSW trending normal faults in the rift center. Mt. Olorgesailie in the rift center is subject to strong fluvial erosion and incision by the Ol Keju Nero river and its tributaries, forming the up to 6-km wide Koora Graben and separating Mt. Olorgesailie from Mt. Shanamu (Figure 1B). Fault scarps in the study region display different stages of erosion suggesting preservation of faults of different ages, as well as variations in the erosional behaviour of the fault structures due to material differences. Faults developed in trachytic volcanic rocks along the northern Magadi trachyte plateau are typically characterized by a stronger degree of erosion and fault scarp degradation than those exposed in the southern Koora Graben and Lake Magadi region, suggesting a gradual N-S shift in faulting activity over time (Owen et al., 2018b; Owen et al., 2019).
The landscapes of the Magadi trachyte and Tepesi-Singaraini basalt plateaus comprise a diverse suite of tectonic landforms created by extensional faulting such as north-south trending successions of horst and graben structures dissected by sub-vertical fault scarps in volcanic bedrock dominated by lavas and pyroclastic deposits. The uplifted fault ridges and horst surfaces are subject to frequent surface runoff and pronounced soil erosion leading to a patchwork of shallow and rocky soils and exposed volcanic bedrock. Bedrock fault scarps are well preserved as sub-vertical cliffs and commonly display strong coating by desert varnish. Downslope of the steep fault cliffs the transition from the uplifted footwall to the fault zone is expressed by an increase of fracture density in the exposed bedrock (fault core). Here, densely fractured fault breccias and clastic dykes are exposed. Fault breccias are frequently cemented with light-colored Ca and Na-rich carbonates. Colluvial deposits at the footslope of the fault cliffs are commonly densely vegetated–with the exception of the little vegetated faulted margins of Lake Magadi and Nasikie Engida–and characterized by a large range of grain sizes including meter-sized angular blocks of volcanic rocks. Basin fill in the tectonic grabens are a wide range of alluvial and lacustrine deposits. The Magadi transect exposes gravelly fluvial terraces in the center, and fine fluvial sands and playa deposits in the easternmost graben. The Tepesi-Singaraini transect exposes clay-rich gravely alluvial plain deposits at the westernmost graben, coarse sand and gravel-rich alluvial and playa deposits in the center, and a mixture of fine-grained sand-rich fluvial deposits and poorly sorted slope wash deposits in the easternmost graben.
Results of XRF and Microscopic Rock Analysis
Whole Rock Composition
The lithology in the study area consists of basaltic and trachytic volcanic rocks and subordinate granitic gneiss. In this study, five rock types were selected as representatives: the Tepesi-Sagaraini basalt, Miocene basalt, the Magadi trachyte and Plio-Pleistocene trachyte as well as a granitic gneiss. These rocks can be divided chemically into two major groups. Primitive (basic), mafic basalts with high concentrations of CaO, MgO and P2O5 and more evolved (acidic) and alkaline granitic gneiss and trachyte with low concentrations of CaO, MgO and P2O5 (Figure 2). CaO is highest in the Tepesi-Singaraini basalt with values ranging between 12.3 and 13.5 wt% and intermediate values of 3.3–7.5 wt% in the Miocene basalts. CaO concentration in Plio-Pleistocene trachyte is always <1.2 wt% and it is slightly elevated in the Magadi trachyte (1.6–2.7 wt%). MgO is also highest in the Tepesi-Singaraini basalt with values ranging between 5.6 and 6.5 wt%. In Miocene basalts, MgO concentrations are highly variable ranging between 1.4 and 6.0 wt%, while in granitic gneiss the contents are always <0.8 wt%. P2O5 concentrations are highest in Pliocene basalt at 0.6–1.1 wt%, whereas concentrations are moderate in Tepesi-Singaraini basalt at about 0.4 wt%. P2O5 concentrations in trachyte and granitic gneiss are always low, with maximal contents in Magadi trachyte (0.2 wt%).
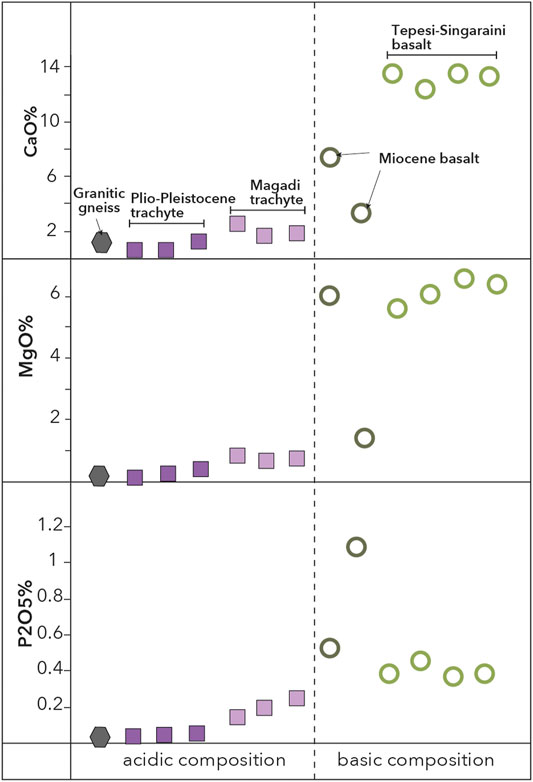
FIGURE 2. Results of XRF whole rock analysis of the representative samples comprising basaltic, trachytic and subordinate granitic lithologies. Different lithological units are represented by varying symbols and colors. Dashed vertical line depicts separation between basic and acidic geochemical compositions.
Mineralogy of the Rock Samples
The whole rock composition reflects the mineralogy of the respective rocks (Figure 3). Basalt consist mainly of Ca-rich plagioclase (50–60 vol%) and Mg-bearing olivine (20–30 vol%) and minor proportions of melt blobs containing primary (magmatic) Ca- and Mg carbonates. Trachytes consist mainly of K-rich alkali feldspar (60–70 vol%) and only subordinate Ca-containing mineral phases like carbonate and clinopyroxene.
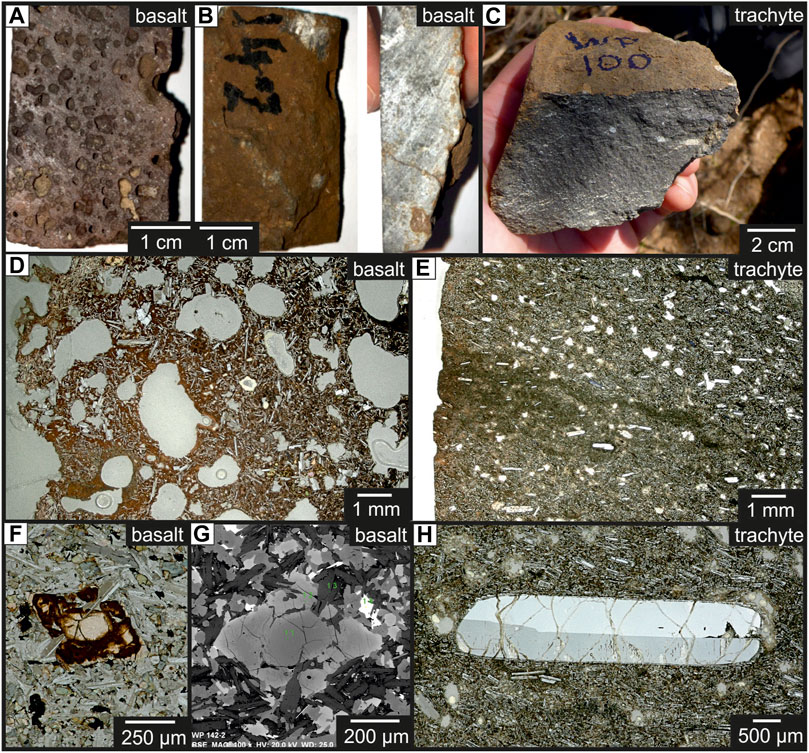
FIGURE 3. (A–C) Photos of hand samples of (A) a bubble rich, completely weathered basalt, (B) a compact, strongly weathered basalt in top view (left) and along a section (right), (C) a compact trachyte with a thin brown weathering crust. (D–H) Microscopic images of (D) a strongly weathered, bubble-rich basalt with a high proportion of white needle-shaped feldspar in a reddish-brown altered matrix, (E) trachyte containing elongated feldspar crystals in a very fine-grained groundmass, with a thin, near-surface, brown weathering crust (left) and in the middle a greenish-brown area indicating the course of a fluid path running from the left (surface of the rock) into deeper (several mm) parts of the rock. Olivine within a moderately weathered basalt, (F) showing strong alteration (dark brown areas) along the grain boundaries and along the cracks (thin section) and (G) a BSE-image of the same olivine crystal; the darker gray area represents the primary olivine with Mg-rich composition and the brighter gray parts of the crystals indicates Fe-enrichment within the altered section of the mineral. (H) One large feldspar crystals within trachyte with weak alteration specifically along the cracks.
Influence of Weathering
The basalt and trachyte rocks show significant differences in weathering behavior (Figures 3A–C). The two basalt types in this region are always fine-grained and often show different degrees of porosity, with some of the basalts being highly vesicular. As the porosity of the basalts increases, the degree of weathering generally increases. Accordingly, basalts are often coated with thick (0.5 - > 1 cm) weathering crusts (denser varieties, Figure 3B) up to completely weathered varieties (bubble-rich varieties, Figures 3A,D). In contrast, trachyte exposed along the toposequence is more compact and therefore more resistant to weathering. Weathering crusts are thin (<1 mm) and present near the surface (Figures 3C,E). Only occasionally, greenish-brown areas were identified, representing fluid pathways (Figure 3E). However, these fluid paths are always limited to a maximum of several millimeters from the surface into the rock (Figure 3E).
Fresh Tepesi-Singaraini Basalts and Magadi trachyte with the weathered samples were analyzed for rock chemistry and mineralogy to determine the consequences of the weathering process.
The weathered parts of Tepesi-Singaraini basalt are depleted in Na, Mg and Ca, which reflect the alteration and dissolution of strongly weathered olivine (releasing Mg) and in-situ crystallization of Fe-rich varieties (Figures 3F,G) and plagioclase (releasing Ca, Na). In contrast, P, Fe, Ti and K are enriched in the weathered part. The newly formed minerals consist mainly of Fe-Ti-rich mineral phases, such as Fe-Ti-oxides or clay minerals among others (Figures 3D,F,G), which dominate strongly altered rock specimens, in some cases up to complete replacement of the primary feldspathic groundmass (Figure 3D).
The comparison of the whole-rock composition of fresh and weathered Magadi trachyte showed only a slight enrichment of Mg, Ca, Na and P in the weathering crusts. Microscopic investigations showed that the primary minerals, such as feldspars, are only slightly altered along the grain boundaries and cracks (Figure 3H). Primary carbonates have been observed in both rock types and are believed to reflect carbonate melt blobs (size range 100–500 μm). Microscopic observations showed that in both rocks these carbonates are only weakly altered along the grain boundaries. Fine-grained secondary carbonates within the rocks indicate that they precipitated in situ. In general, the enrichment of Mg, Ca, Na and P in the weathered parts indicates a fixation of these elements in secondary minerals within the weathered crust of trachytes, whereas the respective elements in basalts are depleted in the weathered areas and therefore released to the soils and groundwater.
Results of Soil Analysis
Soil Organic Carbon and Normalized Differential Vegetation Index
SOC values vary significantly in the study region (Figure 4A) between 0.11% in soils developed on saline lakebeds at the Lake Magadi shore and 2.76% on the Pliocene Narok Agglomerate at higher elevations on the eastern rift shoulder. NDVI values vary between 0.12 and 0.97 in the study area, with lowest NDVI values found on soils derived from volcanic ash exposures south of Mt Suswa in the northern segment of the study region, as well as on exposed lacustrine sediments of Lake Magadi. Highest values were observed on swampy and densely vegetated fluvial deposits in the Koora Graben to the north and east of Kamukuru village (Figure 1B). NDVI strongly correlates with SOC (Figure 4B), indicating that plant biomass production plays an important role in the accumulation of carbon stocks across the entire study region. SOC and NDVI values do not show a clear correlation with particular lithologies, but correlate positively with altitude, as values are on average higher on the uplifted rift flanks (Athi Plain, Ol Eyaseti, Narok, Nguruman). In the densely faulted landscape of the rift center, SOC and NDVI values are systematically higher on the hanging wall sediments compared to lower values on uplifted fault ridges. Generally, high SOC and NDVI values occur in regions that are characterized by the presence of groundwater and/or surface water.
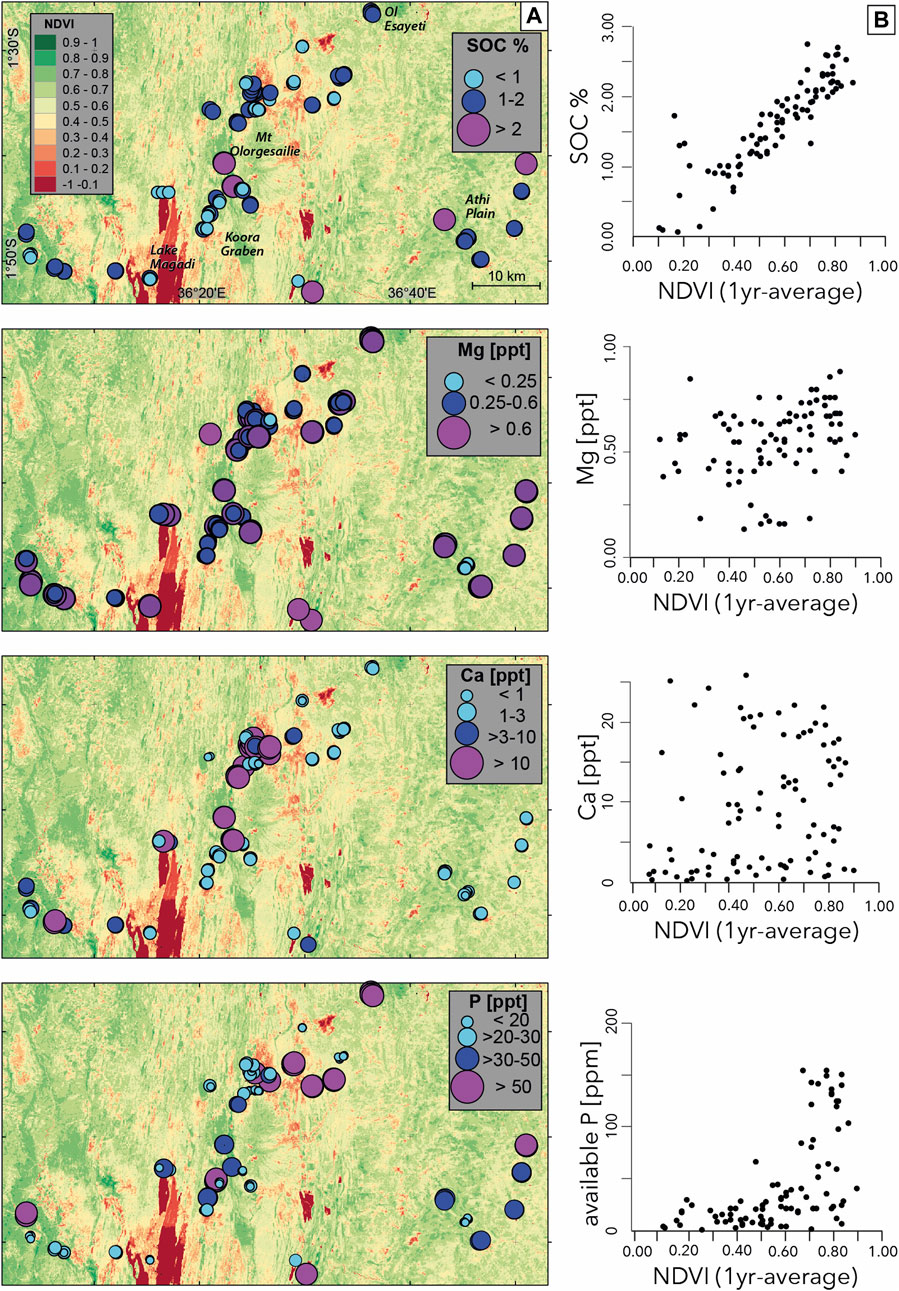
FIGURE 4. SOC, NDVI and soil nutrient distributions; (A) variations in SOC and soil nutrient levels in topsoil samples of the study area, sample locations are represented by colored circles, backround map shows distribution of healthy vegetation using a 1-year average NDVI dataset, derived from Sentinel 2 data. (B) diagrams showing the relationship between SOC and soil nutrients vs. NDVI.
Soil Nutrient Levels
Calcium
Variations in Ca concentrations were driven by both topographic location and chemistry of parent material. Ca levels vary significantly between 0.54 ppt on granitic gneiss and 24.6 ppt on fluvial sediments in the Koora Graben (Figure 4A). Highest Ca levels (>25 ppt) occur in alkaline soils developed on lacustrine sediments of the Legemunge Playa and on fluvial sediments of the Koora Graben. Soils on alluvial plains display more heterogeneous Ca levels, potentially reflecting differences in sedimentary composition or catchment sizes and lithologies; the highest Ca levels are found on soils of the Ol Tepesi plain, lowest levels are found on alluvial deposits dominated by quartzite and granitoid clasts south of Nguruman, in the western sector of the study region.
Soils developed on Limuru Trachyte, Eyasi Phonolite, and Narok Agglomerate have systematically low to very low Ca levels. Shallow soils on Magadi trachyte generally contain low to very low amounts, with the exception of soils sampled in the vicinity of the Magadi hot springs at Little Magadi Lake, which have adequate to high Ca levels. Soils on hanging wall sediments in the faulted landscape of the Magadi trachyte display slightly higher Ca levels than the shallow and rocky soils developed on top of the fault scarps. Ca levels in soils developed on the Athi plain on metamorphic basement rocks are generally low to very low, lowest values are found in shallow rocky soils developed on granitic gneiss south of Kaijado, an area characterized by widespread sheet erosion negatively affecting soil productivity (Sindiga, 1984). Shallow basaltic soils North of Mt Olorgesailie are characterized by adequate to high Ca levels, shallow soils on basalts northeast of Mt Olorgesailie display low Ca levels. Highest amounts of Ca in shallow soils are found on basaltic rocks in the western sector of the study area at Nguruman.
Soils developed on the sedimentary deposits surrounding Mt Olorgesailie contain adequate to high amounts of Ca. Highest levels are found in soils on lakebeds of the Legemunge Plain and in the Koora Graben on river sediments of Ol Keju Nero River. Ca levels in Fluvisols on river deposits in the western sector of the study area are adequate to high, whereas soils on alluvial fan deposits sourced from metamorphic basement rocks (phyllites and granites) have low to very low Ca levels. Unlike SOC, Ca contents in the study region do not show a clear correlation with NDVI values (Figure 4B).
Soils along the Tepesi-Singaraini basalt toposequence (Figure 5A) have consistently moderate to high Ca levels; highest Ca levels are found in playa sediments in the middle of the transect, lowest in alluvial soils east of the playa. Ca distribution shows a systematic correlation with hillslope location, and proximity to steep fault ridges, respectively. In colluvial deposits Ca levels in soils are systematically higher compared to those located upslope on fault ridges and plateaus. Highest Ca variations are in soils developed on alluvial plains and fluvial deposits.
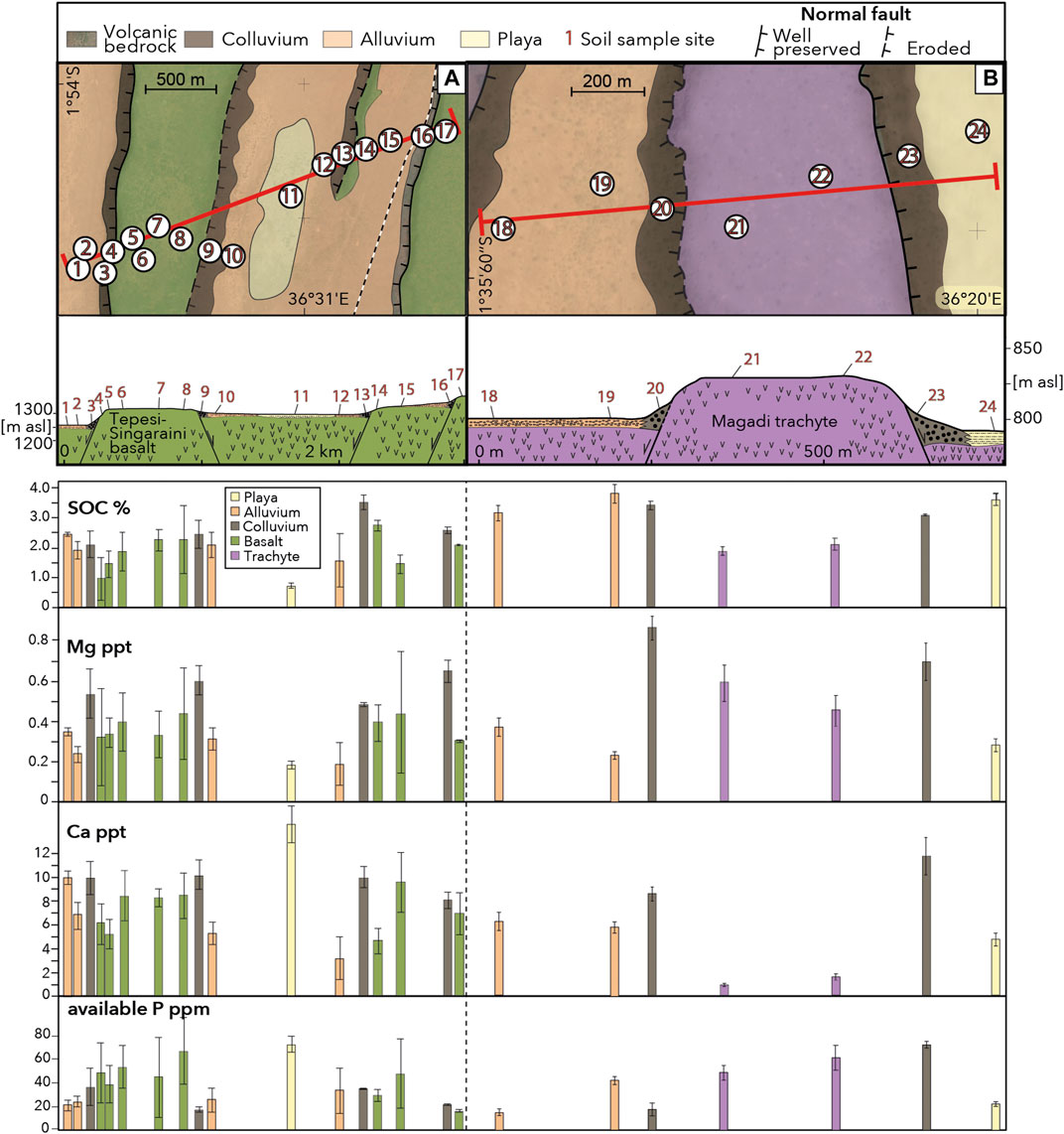
FIGURE 5. Soil nutrient and SOC variations along two toposequnces crossing (A) the Tepesi-Singaraini basalt plateau, and (B) the Magadi trachyte plateau. Red lines depict the locations of topographic profiles; numbers represent locations of a soil sampling site that usually consists of 3-5 individual soil samples; colored bars represent differences in parent material.
Compared to soils along the Tepesi-Singaraini transect, Ca levels are on average lower along the Magadi trachyte toposequence (Figure 5B). However, the 5 to 7-fold increase of Ca in soils on colluvial deposits at the base of fault scarps vs. those in shallow soils on fault ridges and the uplifted plateau is much more distinct in trachytic soils compared to that observed in basaltic soils (1.5 to 2-fold increase).
Magnesium
Mg levels in the study region vary relatively little between and only weakly correlate with the different lithologies (Figure 4A). Low levels are found in a few soils developed on granitic gneiss and at one sample location on the Legemunge playa. At all other sample locations the soils contained moderate (0.25–0.6 ppt) to high (>0.6 ppt) amounts. Mg is highest in soils developed on trachytic and phonolitic parent material and thus negatively correlates with Ca, which is lowest in these soils. Mg levels along the Tepesi-Singaraini and Magadi toposequences vary in a similar pattern to Ca (Figure 5). Soils on fault colluvium are systematically higher in Mg than those on ridges and plateaus. Mg levels on the uplifted Magadi plateau negatively correlate with Ca, a pattern we have also observed in other soils on trachytic and phonolitic parent material. Mg levels in the study region do not show a clear correlation with NDVI values (Figure 4B).
Plant-Available Phosphorus
Available P levels in the study area vary significantly between 1 ppm on granitic gneiss and 155 ppm on Narok agglomerate. Highest P levels occur in soils located on the eastern rift shoulder (Figure 4A) that has been subjected to agricultural use, which is why the use of phosphate fertilizer cannot be excluded as an explanation for high P values. Soils with high P that were not affected by cropping occur on sediments in the footwall of fault ridges on Tepesi-Singaraini basalt, as well as basaltic soils in the vicinity of Nguruman. P levels of soils in the Koora Graben and Legemunge playa vary significantly and correlate negatively with Ca levels. Variations in P across the study area show a weak positive correlation with NDVI (Figure 4B), and regions with high P (>50 ppm) are located in regions with NDVI >0.6; P levels <50 ppm are less clearly correlated with NDVI. This suggests that biochemical processes such as root-soil interactions or the presence of grazing animals may play a dominant role in the release of plant available P levels in such regions (Mathews et al., 1994; Richardson et al., 2009).
P levels along the Tepesi-Singaraini and Magadi toposequences vary strongly with proximity to hillslopes and show a trend opposite to Ca and Mg. P levels are systematically higher on flat terrain compared to steep slopes. Highest P values occur in regions subject to intensive animal grazing on playa deposits and the top of the westernmost fault plateau, which suggests additional P input from biological sources.
Discussion
Geological Factors in Soil Nutrient Distributions
The availability of soil nutrients in ecosystems and natural landscapes depends on a combination of climate, topography, parent material, biota and time, and the interplay of these factors can be very complex and our understanding of their relative significance remains limited (Mage and Porder, 2013). While climatic and biological activity can vary drastically over short time scales and may change some soil properties on time scales of a few decades, factors like the chemistry of parent material and the topographic setting of a tectonically quiescent region can potentially control soil properties for much longer time scales of 103–106 years. However, in dynamic landscapes controlled by e.g., volcanism and earthquake activity and associated hillslope processes, both topographic and lithological factors can change drastically during single events, as well as continuously over long time periods. Single events can include: 1) volcanic eruptions and related widespread deposition of volcanic ash over several days or weeks as recently and historically documented for the Serengeti plains in northern Tanzania (Murray, 1995; Quigley et al., 2017; Eckmeier et al., 2020); 2) surface rupturing by large earthquakes and related dissection and uplift of the landscape leading to drastic readjustments of the landscape such as transient river damming (e.g., King and Vita-Finzi, 1981), as well as enhanced erosion and slope failure processes along uplifted fault escarpments (e.g., Hermanns et al., 2001). Long-term processes may include drainage rearrangement by river capture and river gorge development in steadily uplifting regions (Bishop, 1995; Stokes et al., 2008). To understand how soil nutrient variations determine ecosystem processes and human-landscape interactions over long periods of time, we need to carefully consider the whole range of processes influencing the availability and distributions of soil nutrients, as well as the temporal variability of such processes in our studied region.
Rock Chemistry and Soil Properties
Base cation concentrations in soils have commonly been attributed to in-situ mineral weathering, suggesting that high levels of Ca, Mg, Na, and K can be directly attributed to the mineralogical and sedimentological composition of the parent material. Due to high solubility of these base cations in the soil solution, frequent provision of freshly weathered minerals is necessary to prevent leaching losses (Bern et al., 2005). In our study area, we observed strong variations in Ca, available P and SOC levels over the entire study area as well as across both toposequences. While Ca, P and SOC levels all vary with topography and relief, only Ca can systematically be correlated with the composition of the parent material. Low Ca levels in soils occur on trachyte, phonolite and granitic gneiss (Figure 6)–the lithologies with the lowest amounts of CaO in the study region (Figure 2).
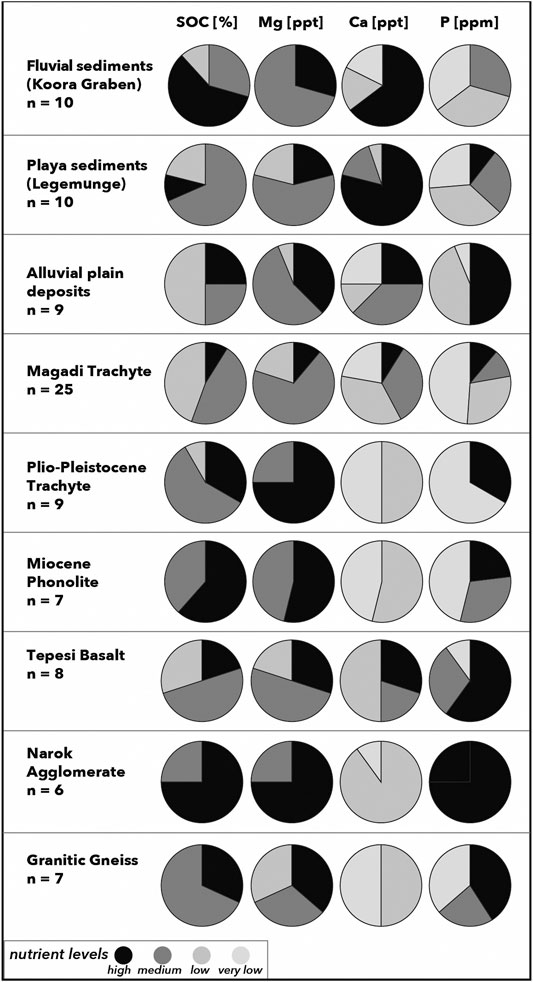
FIGURE 6. Distributions of soil nutrient levels and SOC attributed to the main lithological and sedimentological units of the study area; SOC: low <1%, moderate <2%, high >2%; Mg: low <0.25 ppt, moderate 0.25–0.6 ppt, high >0.6 ppt; Ca: very low <1 ppt, low 1–3 ppt, moderate > 3–10 ppt, high >10 ppt; available P: very low <20 ppm, low 20–30 ppm, moderate >30–50 ppm, high >50 ppm.
Excessive Ca levels (>10 ppt) are found in soils on playa and alluvial deposits characterized by high alkalinity (Figures 4, 5A), which suggests an enrichment of Ca in the form of CaCO3 as a component of calcretes, dust or lacustrine sediments. It can accumulate in the topsoil through evapotranspiration (e.g., Alonso-Zarza, 2003) or deposition of carbonate rich materials such as dust or volcanic ash. This hypothesis is supported by soil profiles analyzed on the Legemunge and Oltepesi plains. Here, Ca levels are highest in the topsoil and systematically decrease with depth, while clay rich vertisols in the Koora Graben show an increase of Ca with depth, potentially due to leaching processes in near surface horizons (Figure 7). Our observations suggest that while geological data in general can provide useful information on the long-term availability of Ca in soils, locations of high alkalinity may display drastic differences in near-surface Ca levels under wetter climates.
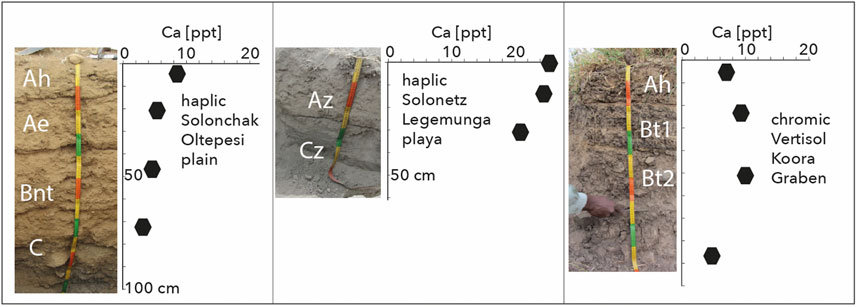
FIGURE 7. Soil profiles and Ca variations with depth; (A) haplic Solonchak from soil pit on Oltepesi plain; (B) haplic Solonetz exhibiting strong salinification features from soil pit on Legemunge playa north of Olorgesailie site; (C) chromic Vertisol from road cut in floodplain deposits of the Koora graben, SW of Mt. Olorgesailie.
In contrast to Ca, levels of available P are scattered over different lithologies (Figure 6), suggesting minor influence of mineral-bound P in the parent material on available P in soils. In alkaline soils of the Legemunge playa and Lake Magadi basin available P levels are consistently low and negatively correlated with Ca levels (Figure 8). Calcium is known to cause P fixation in high pH soils (Hemwall, 1957). We observe a threshold of Ca > 10 ppt above which available P is suppressed (Figure 8). This observation suggests that under stable climatic conditions, the studied soils in arid locations on playa deposits subjected to high evaporation rates and excess soil Ca levels are likely to be characterized by long-term deficient available P. This in turn implies that present day P levels are not necessarily a reliable proxy for the long term nutritional soil status, as P fixation might have been drastically different under wetter climatic conditions as reported for the study region in Pleistocene and early Holocene times (Trauth et al., 2005; Owen et al., 2018a; Owen et al., 2019).
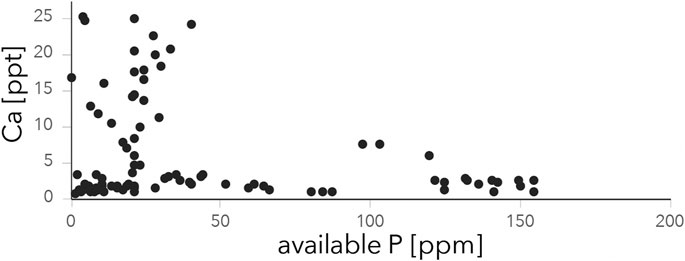
FIGURE 8. Relationship between soil Ca and plant available P in the study region. Note the ∼10 ppt threshold of Ca concentrations supressing available P in topsoil samples.
We did not observe a clear correlation between SOC and lithology (Figure 6), which suggests that parent material does not primarily influence biomass production, as reported from regions interspersed with e.g. ultramafic rocks or in limestone landscapes (Kruckeberg, 2004). However, SOC systematically follows topographic gradients and related vegetation patterns, as shown in the strong correlation between NDVI and SOC (Figure 4A).
The major rock types occurring in the study area, trachyte and basalt, show differences in weathering behavior. In comparison to the fine-grained and often highly porous (vesicular) basalts, trachyte is more resistant to weathering processes because it is more compact and therefore resist better the penetration of fluids into the rock. Macroscopic and microscopic observations showed that trachyte shows predominately near-surface alteration rims of millimeter to centimeter in size, whereas basalts are mostly characterized by deep to complete alteration (Figure 3).
Microscopic examinations revealed a specific resistivity of the minerals present in the assemblages, with olivine < plagioclase < pyroxene; this trend corresponds to the observations of Eggleton et al. (1987). Accordingly, olivine and plagioclase, the main components of basalts, show strong weathering effects and are replaced by secondary minerals, such as Fe-Ti oxides. The observed enrichment of Fe and Ti and a depletion of Mg, Ca and Na in the whole rock composition of the weathered parts of basalt compared to fresh samples means that Fe and Ti are mineralogically bound within the weathering crusts of the rocks and Mg, Ca and Na are released into the soil, and groundwater–if present. In contrast, weathering of trachyte is limited to an alteration of clinopyroxene, which induces a mobilization of Ca and Mg. However, the whole rock composition shows an accumulation of these elements in the weathered parts of the trachyte. This indicates limited mobilization and mineralogical fixation of Mg and Ca in secondary minerals, such as carbonates, within the rock. Consequently, these elements are not available for overlying soils.
Puzzling are high Ca concentrations of soils developed on colluvial deposits in both basaltic and in trachytic areas, with significant increase of Ca concentrations in soils on trachytic colluvium in comparison to the original fresh rock. Primary carbonates, which are present in trachytes and in basalts, represent carbonatitic melt blobs, which can theoretically be a source of Ca and Mg in soils. However, microscopic observations showed that these carbonatite blobs are generally only slightly altered along grain boundaries and are replaced in situ as fine-grained secondary carbonates. Therefore, the availability of Ca and Mg from these carbonates for soils is limited.
Petrological investigation of a soil-rock (basalt)-profile from Sahand volcano (NW Iran), located in an area of active CO2 degassing, has shown a preferential mobilization of Ca from the host rock and precipitation of carbonates along cracks and at the interface between rock and soil (Aßbichler et al., in preparation). Correspondingly, preliminary results of leaching experiments carried out at the geological laboratories at the Department of Earth and Environmental Sciences, LMU Munich, showed that the addition of CO2 gas generally improves the leachability of Ca from minerals and thus enhances the mobilization of divalent elements such as Ca and Mg (Aßbichler et al., in preparation). Previous studies carried out in the Lake Magadi and Lake Natron regions (Lee et al., 2016; De Cort et al., 2019) have shown that generally fault zones provide perfect pathways for mantle gases such as CO2 (diffusive soil gas).
High Ca contents in trachytic soils can not only be found on the colluvial deposits, but also at the northern shore of Nasikie Engida in the vicinity of the Little Magadi hot springs (Figures 1B, 4A), suggesting an influence of CO2 degassing on Ca mobilization. In this context, one would also expect travertine or other secondary carbonate formations at the hot springs. Thicker carbonate formations at the hot springs are, however, only fossil (Pleistocene) calcretes (>30 cm) north of Nasikie Engida (Renaut et al., 2020, in press). In addition, the recent saline spring fluids are characterized by low dissolved Ca- and Mg- concentration and precipitates are dominated by Na-(hydro)-carbonates (Renaut et al., 2020, in press), indicating that nowadays Ca mobilization by the fluids of the hot springs does not play a major role. In this context, two things have to be considered: 1) At the warm temperature of the hot springs fluids, Ca-carbonate hardly dissolves in water. Even at room temperature, Ca-bearing water immediately precipitates carbonates in the presence of CO2 (e.g. Dodson and Standing, 1944). The carbonate solubility decreases with increasing temperature, and in consequence water with low Ca (and Mg) concentrations remains. In the Magadi basin, the effect of early precipitation of calcite and Mg-calcite in subsurface flow paths has been observed by (Owen et al., 2019) for the Lake Magadi brines. 2) At the presence of water, leaching of Ca is expected to be enhanced due to the formation of carbonic acid compared to “dry” (water-free) conditions, leading to a faster leaching of Ca from the exposed trachytes. The occurrence of Pleistocene carbonate precipitates and carbonates at the bedrock interface indicates that Ca mobilization has occurred within a limited period in the past. Trachytes in the Magadi area have low Ca contents (<2.7 wt% CaO), while Na- and K- concentrations are significantly higher (e.g. >5 wt% Na2O). It is therefore reasonable, that after most of the Ca has already been leached from the exposed trachytes, the conditions shifted to more Na- (and K-) rich conditions, which are now present at the hot springs. Correspondingly, a study of samples from Laacher See volcano has shown that under CO2 rich conditions Ca is preferentially leached, followed by the leaching of Na from the primary minerals (Aßbichler, 2020).
The soil and rock samples in our study were taken from areas in which water is likely to play only a subordinate role, particularly along the Magadi toposequence (Figure 5), where no groundwater influence on soil formation has been observed. Assuming a “dry” diffusive CO2 soil degassing, in which water is only a subordinate reaction partner, leaching and dissolution reaction via CO2 is to be expected to be much slower, but continuous. This contributes to a constant, low level-mobilization of Ca from the rocks to the surface, and consequently to a continuous enrichment of Ca in the soils, and at the interface between rock and soil. We therefore assume that ascending mantle gases may be an overlooked factor in mobilizing Ca ± Mg from deeper parts of the rock profile and may contribute to the accumulation of Ca in the colluvial soil and areas close to the hot springs.
Tectonic Faulting and Soil Nutrient Redistribution
Nutrient distributions in soils are controlled by two main factors 1) release and vertical transport through in situ mineral weathering and soil profile development in residual soils 2) lateral transport through mass flux along topographic gradients or airborne accessions of dust and/or volcanic ash (Simonson, 1995; Calitri et al., 2019).
Extensional tectonics led to a horst-and-graben landscape characterized by a complex patchwork of uplifted and eroding surfaces. This promoted the formation of residual soils, and down-dropped basins constantly subjected to deposition of freshly eroded material, as well as redeposition of soil cover and sediments through slope failure and alluvial processes (Tucker and Bras, 1998; Roering et al., 2001; Pelletier and Rasmussen, 2009).
Along both toposequences, we observe a systematic increase of Ca and Mg in soils from uplifted fault ridges, to colluvial deposits downslope of active fault structures. This suggests that primary weathering of freshly eroded minerals releases sufficient amounts of Ca and Mg-bearing minerals to rejuvenate soils in these locations. Alluvial soils in the valleys are often depleted in Ca and Mg, either because of fast weathering and dissolution of Ca and Mg-bearing minerals or lateral translocation of sediments through alluvial processes. Systematic increase of Ca, as well as other nutrients including Mg and K downslope along steep convex hillslopes has already been reported in other studies (e.g., Chadwick and Asner, 2016). Our field observations and laboratory analyses suggest that Ca enrichment in downslope deposits occurs through a combination of accumulation of freshly eroded rocks in colluvial deposits and in-situ alteration of rejuvenated soils, potentially enhanced by the effect of diffusive CO2 degassing along the fault zones (Lee et al., 2016).
Levels of available P are lower in colluvial deposits than on flat surfaces, suggesting that slope and topographic position influences available P levels in a different way than Ca and Mg. While earlier studies showed that parent material and topographic position have a strong influence on the levels of total P in soils (Mage and Porder, 2013; Chadwick and Asner, 2016), the controls on plant available P appear to be different. In our study area along the Singaraini toposequence, flat terrain is frequently visited by herds of grazing animals. This suggests that the sources of plant available P might be related to other biological P sources such as animal manure. Also, the effects from burrowing animals or termites in soil redistribution and P sorption and availability, as well as the role of eolian input by dust or volcanic ash in providing extra amounts of various minerals may play a role in this context and need further attention (Chadwick et al., 1999; Seymour et al., 2014). Particularly the potential role of carbonatitic volcanic ash on the distribution of exchangeable cations could be of interest for future studies in the area, as there are three volcanic centers of in the vicinity of the study region (Pleistocene volcanos Suswa and Shombole and the currently active Ol Doinyo Lengai) exposing carbonatitic eruption phases. Studies in the Serengeti-Mara ecosystem have shown that frequent input of Na- and Ca-rich carbonatitic ash from recent eruptions of Ol Doinyo Lengai volcano has had a strong effect on the distribution of Na and Ca in the topsoils of the Serengeti Plains (Jager, 1982; Murray, 1995; Eckmeier et al., 2020).
SOC variations along both toposequences correlate with vegetation density suggesting coupling between soil carbon storage and total biomass production. This observation is consistent with SOC levels of the entire study area, which strongly correlate with average annual NDVI values (Figure 4A). In the southern Kenya Rift, stripes of dense vegetation systematically occur along colluvial sediments deposited adjacent to active normal faults, a phenomena common for tectonic landscapes worldwide as fault zones are often characterized by structurally controlled hydrology and vegetation cover. Footwall sediments adjacent to active fault ridges are likely situated in favorable hydrological conditions as fault zones promote water infiltration and storage, as well as zones of localized water discharge. Therefore, wetlands and other regions of stable vegetation cover are often associated with active fault zones (Forsberg et al., 2000; Reynolds et al., 2011). The correlation between SOC and average NDVI throughout the study area further implies that topsoils in regions of seasonally stable vegetation cover are significantly more effective in building up SOC stocks than regions dominated by dry vegetation and short green vegetation periods during the rainy seasons. Less precipitation or even drought inhibit plant growth and soil biota activity, which results in lower production of soil organic matter or SOC (e.g., McSherry and Ritchie, 2013).
Soil Nutrients and Hominin-Landscape Interactions
While the importance of soils to influence the growth and annual dynamics of vegetation is well studied in plant ecology and evolution (Kruckeberg, 2004; Rajakaruna and Boyd, 2019), the ability of soil parameters to drive other ecosystem dynamics such animal grazing patterns and human subsistence strategies is less well known. Studies on the mobility of present-day ungulate herds in the Serengeti-Mara ecosystem, in northern Tanzania, have provided first insights in the interplay between the nutrient status of soils on a regional scale and related seasonal movements (McNaughton, 1990; Murray, 1995; Eckmeier et al., 2020). Results of these studies suggest that rock-soil interactions and frequent input of weatherable minerals from volcanism, particularly carbonatitic ash from nearby Ol Doinyo Lengai volcano, as well as spatially limited tectonic activity play a role in driving and maintaining the long-term movement of large animal herds from one nutritional hot spot to another. Regions of high soil nutrient availability represent seasonally stationary grazing areas, while regions of low soil nutrient availability serve as short-term or transitional grazing (Murray, 1995). In contrast to the landscape in the Serengeti-Mara ecosystem, characterized by relatively low relief and - with the exception of the Utimbaru-Isuria fault system (Kabete et al., 2012)–little tectonic activity, the Southern Kenya Rift is highly complex and dominated by the long-term effects of extensional faulting volcanic activity. Wildlife dynamics as responses to soil nutritional gradients in a relatively flat Serengeti-type landscape lead to wide corridors of animal movements distributed over a large region. In complex tectonic landscapes such as the southern Kenya Rift, animal movements are likely channeled through narrow corridors along soil nutritional and/or topographical pathways (King et al., 1994; King and Bailey, 2006; Devès et al., 2014; Kübler et al., 2019). In our study region, during Pleistocene lake-level high stands such migration corridors would have likely lead along narrow strips of exposed lake sediments or the northern or southern footslopes of Mt. Olorgesailie (Figure 9).
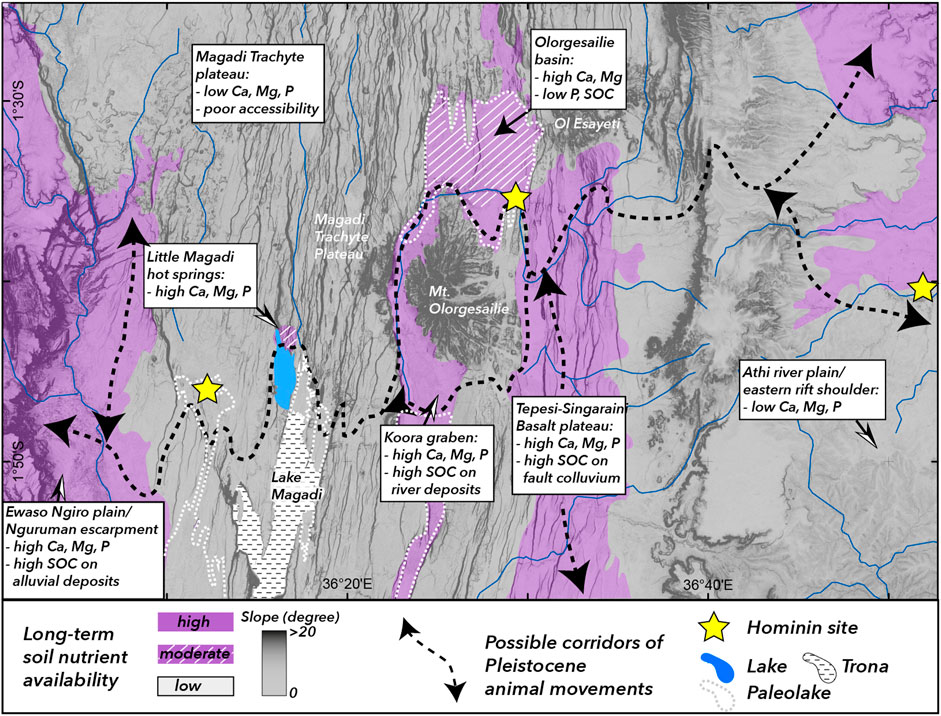
FIGURE 9. Soil nutritional corridors and Pleistocene human-animal-landscape interactions. Regions colored in gray are characterized by low long-term soil nutrient availability and/or poor accessibility due to topographic barriers such as fault scarps and complex terrain, purple regions represent possible soil nutritional corridors and paleo-migration routes for animal herds that could have been exploited by early hominins. Purple regions with white cross patterns depict regions of moderate nutrient availability (e.g., high Ca, but low P or SOC) potentially representing locations of transitional or short-term grazing. Steep slopes are potential migration barriers in regions of high landscape complexity and are indicated by dark gray shading. Yellow stars indicate locations of hominin fossil sites: (I) Isinya, (L) Lainyamok, (O) Olorgesaile. Potential paleolake extents (dashed white lines) are derived from geological and pealeoenvironmetal datasets (Guth and Wood, 2013; Muiruri, 2017; Owen et al., 2019).
In a paleoanthropological context, the study of landscape complexity and related heterogeneity on soil parameters derived from long-term geological processes can serve as proxy for long-term environmental variability–a factor that has been identified as key element in human evolution studies (Potts, 1996; Potts, 1998; Potts, 2013; Potts et al., 2018). Geologically controlled and topographically accessible corridors of reliable vegetation cover and soils high in vital nutrients thus represent potential paleo-migration corridors of grazing animal herds (Devès et al., 2014; Kübler et al., 2015; Kübler et al., 2016) and regions of importance for hominin subsistence strategies and enhanced hominin mobility over long periods of time (Figure 9).
The Olorgesailie site is located at a narrow corridor, extending from the Oltepesi plain to the southern Koora Graben, characterized by spatially consistent high Ca-levels and variable P-levels in a wider region deficient of these important macronutrients. The possibility that such conditions can be extended to paleoenvironmental settings depends on the supply of rock-derived nutrients to the Olorgesailie basin and their temporal and spatial variability. The extremely high Ca levels on Legemunge playa and Oltepesi plain at present-day likely result from superficial evapotranspiration processes under semi-arid conditions, potentially combined with periodic eolian input of Ca-rich dust and/or volcanic ash, and would have likely been less pronounced during more humid climate periods. Higher soil moisture contents in the past environment around Olorgesailie site would have had two likely consequences: first, Ca levels in topsoils would have overall been lower and likely closer to those observed at present in the Koora Graben or at higher altitudes; second, fixation of available P through excessive Ca would have been less pronounced, and P levels would have likely been higher in soils. Under moister conditions, the overall coverage of seasonally stable green vegetation would likely be higher than today. Our results on the correlation between SOC and NDVI (Figure 4) imply that regions of short green vegetation periods are not well reflected in the topsoil’s SOC stocks, we argue that the present-day distribution of topsoil SOC is a not a suitable proxy for past vegetation patterns.
To better understand the changing sources of nutrients for the Olorgesailie site and surrounding region from Mid Pleistocene to present, it is important to take a closer look at the development of the fluvial system supplying the Olorgesailie basin (Figure 1B). The Ol Keju Nero river is characterized by a widespread network of different tributaries draining through various different lithological units and therefore provides a wide range of nutrients from various mineral sources. Reconstructions of Mid Pleistocene to recent depositional cycles show that from ∼1.2–0.5 Ma BP the Olorgesailie basin was characterized by an extensive lake located north of Mt. Olorgesailie and south-westward drainage by the ancestral Ol Keju Nero river (Isaac, 1977; Behrensmeyer et al., 2018). Sediment input was dominated by transport of volcanic sediments from proximal sources, primarily consisting of Ol Tepesi Basalt and Magadi trachyte ridges surrounding the basin (Behrensmeyer et al., 2018). After 0.5 Ma, a series of tectonic and/or volcanic events (Kübler et al., 2015) led to the disappearance of the extensive lake and incision of Ol Keju Nero river in lacustrine sediments. A northern tributary draining from the northern rift center and northeastern rift shoulder into the basin was established after ∼320 ka BP and included sediments from carbonatitic lavas and ash deposits derived from Mt Suswa after ∼240 ka BP. The modern course of Ol Keju Nero river with main drainage from the east was established after ∼45 ka BP.
Despite the modifications in river networks since the Middle Pleistocene that lead to various changes of the catchment sizes and tributaries draining into Olorgesailie basin (Behrensmeyer et al., 2018), sediments were persistently characterized by a mixture of different volcanic and metamorphic rock sources likely providing a wide range of weatherable minerals to the depositional system. Thus, it is reasonable to assume that soils in the vicinity of Olorgesailie site would have had sufficient soil nutrient levels under different paleoclimatic conditions since the Mid Pleistocene. The location of Olorgesailie site proximal to the lakeshores and reliable soil nutrient levels providing attractive animal grazing grounds has likely promoted the long-term attractiveness of this location for hominin subsistence strategies.
Isinya site is the first Acheulean site excavated in the highlands on the eastern rift shoulder (Figures 1B, 9) and represents in contrast to the long-lived Olorgesailie site a location where only one time period of site inhabitance (>0.96 Ma BP) can be resolved (Durkee and Brown, 2014). The site is located in fluvio-lacustrine deposit at the transition between phonolitic rocks to the south and basaltic rocks to the north (Roche et al., 1988; Guth and Wood, 2013). Given the limited amount of artifacts and relatively short time span of site occupation, reasons for hominin presence at Isinya site is poorly understood. However, the proximity to water as well as workable stones from phonolitic and basaltic sources, and reliable nutrient provision from soils developed on basaltic parent material have likely played a role in hominin inhabitance around Isinya site.
Lainyamok site is located north of Lake Magadi in the densely faulted Magadi trachyte plateau. Unlike at the other two sites, the site likely represents a location of hominin bone deposition from proximal sources through debris flows and redistribution from carnivore activity (Potts et al., 1988). The poor soil nutrient status of the surrounding region would support the interpretation that Lainyamok represents a region promoting short-term or transitional landuse and high hominin mobility between several small-scale nutritional hot spots like the one identified at Little Magadi hot springs (Figures 1B, 4A, 9) rather than long-term occupation of one location.
In summary, our results show that integrating information from pedological and geological analysis provided additional information to interpret the long-term attractiveness for grazing animals and human subsistence of the southern Kenya Rift with regard to its soil nutritional status.
Conclusion
Soil chemical properties in the southern Kenya Rift are strongly variable and controlled by geochemical as well as topographic factors. SOC is coupled with vegetation density, which in turn is controlled by topography and tectonic activity. Stable vegetation cover in the rift center is predominately fault controlled and occurs along colluvial sediments downslope of active normal faults.
Chemical properties of the parent material represent an important constraint on the distribution of soil nutrients, particularly of Ca, in topsoils. Granitic and trachytic rocks are systematically lower in CaO compared to basaltic rocks sampled in the study region, resulting in low Ca levels in regions dominated by soils developed on felsic and intermediate parent material. Locally enhanced Ca levels in soils proximal to the Little Magadi hot springs represent a striking anomaly compared to the surrounding region possibly results from CO2 degassing, which suggests that localities of high seismicity and related thermal activity may provide sufficient nutrients for a limited amount of time. Ca levels correlate with hillslope position and are consistently high on colluvial deposits downslope of active faults, suggesting higher CO2-induced Ca mobilization from the substrate rock through enhanced weathering and/or tectonic degassing (Lee et al., 2016). The potential role of CO2 in the precipitation of Ca is a novel aspect of our analysis and requires additional systematic studies in the future. Improved knowledge on this process may have implications for other research fields such as ecosystem and agricultural sciences. In contrast to Ca, correlation between lithology, relief and plant available P is less obvious, which implies that P availability is controlled by other factors such as P fixation through Ca in alkaline soils and additional biological and/or eolian P sources.
Known hominin sites in the region are located either along corridors of long-term Ca availability (Olorgesailie, Isinya) or in proximity to short-term nutrient hotspots (Lainyamok) potentially related to active CO2 degassing along active fault zones. The correlation between locations of hominin activity and the long-term soil nutrient availability in the study region suggests that knowledge of the long-term soil nutrient availability of a region can improve paleoenvironmental and paleoanthropological interpretations related to how hominins have strategically exploited beneficial elements of complex landscapes and provide important information on the interpretation of hominin subsistence strategies. Further, integrated geopedological studies in a paleoanthropological context can become a new tool in discovering hominin fossil sites in other tectonically active regions in the world.
Data Availability Statement
The original contributions presented in the study are included in the article/Supplementary Material, further inquiries can be directed to the corresponding author.
Author Contributions
SK supervised the project and designed the field study. SK, SR, GK have carried out field sampling and mapping; DA has carried out chemical and microscopic analysis of rock samples; EE has interpreted soil data. SK wrote the manuscript with support from DA and EE. All authors provided critical feedback and helped shape the research, analysis and manuscript.
Funding
Research was carried out under a permit (NACOSTI/P/16/8491/7891) by the National Commission for Science, Technology and Innovation of Kenya, and was supported by the European Research Council through ERC Advanced Grant 269686 DISPERSE, granted to GK (IPGP) and G. Bailey (University of York). SK received financial support from a postdoctoral fellowship of the German Science Foundation (DFG grant No. KU 3512/2-1) and provision of high-resolution Satellite data (TanDEM-X mission) from the Helmholtz Alliance EDA. Additional financial support for field work and sample analysis was granted by the Carl Friedrich von Siemens Fellowship from the Alexander von Humboldt Foundation.
Conflict of Interest
The authors declare that the research was conducted in the absence of any commercial or financial relationships that could be construed as a potential conflict of interest.
Acknowledgments
We are grateful to Peter Owenga and Maurice Obunga for assistance during field work as well as soil sampling and soil laboratory work, and to Daniel Lohner and Nikolai Rauchberger (University of Munich) for thin section and XRF preparation and technical support.
Supplementary Material
The Supplementary Material for this article can be found online at: https://www.frontiersin.org/articles/10.3389/feart.2021.611687/full#supplementary-material.
References
Abate, A. (1988). Effect of pasture mineral levels on extensive cattle production in Kenya. Afr. Forage Plant Genet. Resour. Eval. Forage Germplasm Extensive Livestock Prod. Syst. 449.
Alonso-Zarza, A. M. (2003). Palaeoenvironmental significance of palustrine carbonates and calcretes in the geological record. Earth-Science Rev. 60 (3-4), 261–298. doi:10.1016/s0012-8252(02)00106-x
J. M. Anderson, and J. S. Ingram (1993). Tropical soil biology and fertility: a handbook of methods. Wallingford: CAB International.
Atmaoui, N., and Hollnack, D. (2003). Neotectonics and extension direction of the southern Kenya rift, Lake Magadi area. Tectonophysics 364 (1), 71–83. doi:10.1016/s0040-1951(03)00051-9
Baker, B. H., and Mitchell, J. G. (1976). Volcanic stratigraphy and geochronology of the Kedong-Olorgesailie area and the evolution of the South Kenya rift valley. J. Geol. Soc. 132 (5), 467–484. doi:10.1144/gsjgs.132.5.0467
Baker, B. H., Williams, L. A. J., Miller, J. A., and Fitch, F. J. (1971). Sequence and geochronology of the Kenya rift volcanics. Tectonophysics 11 (3), 191–215. doi:10.1016/0040-1951(71)90030-8
Behrensmeyer, A. K., Potts, R., Deino, A., and Ditchfield, P. (2002). Olorgesailie, Kenya: a million years in the life of a rift basin. Soc. Sediment. Geology. Spec. Publ. No. 73, 97–106. doi:10.2110/pec.02.73.0097
Behrensmeyer, A. K., Potts, R., and Deino, A. (2018). The Oltulelei Formation of the southern Kenyan Rift Valley: a chronicle of rapid landscape transformation over the last 500 ky. GSA Bull. 130 (9-10), 1474–1492. doi:10.1130/b31853.1
Bern, C. R., Townsend, A. R., and Farmer, G. L. (2005). Unexpected dominance of parent-material strontium in a tropical forest on highly weathered soils. Ecology 86 (3), 626–632. doi:10.1890/03-0766
Birt, C., Maguire, P., Khan, M., Thybo, H., Keller, G. R., and Patel, J. (1997). The influence of pre-existing structures on the evolution of the southern Kenya Rift Valley—evidence from seismic and gravity studies. Tectonophysics 278 (1-4), 211–242. doi:10.1016/s0040-1951(97)00105-4
Bishop, P. (1995). Drainage rearrangement by river capture, beheading and diversion. Prog. Phys. Geogr. Earth Environ. 19 (4), 449–473. doi:10.1177/030913339501900402
Calitri, F., Sommer, M., Norton, K., Temme, A., Brandová, D., Portes, R., et al. (2019). Tracing the temporal evolution of soil redistribution rates in an agricultural landscape using 239+ 240Pu and 10Be. Earth Surf. Process. Landforms 44 (9), 1783–1798. doi:10.1002/esp.4612
Chadwick, K. D., and Asner, G. P. (2016). Tropical soil nutrient distributions determined by biotic and hillslope processes. Biogeochemistry 127 (2-3), 273–289. doi:10.1007/s10533-015-0179-z
Chadwick, O. A., Derry, L. A., Vitousek, P. M., Huebert, B. J., and Hedin, L. O. (1999). Changing sources of nutrients during four million years of ecosystem development. Nature 397 (6719), 491–497. doi:10.1038/17276
Chorowicz, J. (2005). The east African rift system. J. Afr. Earth Sci. 43 (1-3), 379–410. doi:10.1016/j.jafrearsci.2005.07.019
Crossley, R. (1979). The Cenozoic stratigraphy and structure of the western part of the rift valley in southern Kenya. J. Geol. Soc. 136 (4), 393–405. doi:10.1144/gsjgs.136.4.0393
D'Alessandro, W. (2006). Human fluorosis related to volcanic activity: a review. Environ. Toxicol. 1, 21–30. doi:10.2495/ETOX060031
Davies, T. C. (2013). Geochemical variables as plausible aetiological cofactors in the incidence of some common environmental diseases in Africa. J. Afr. Earth Sci. 79, 24–49. doi:10.1016/j.jafrearsci.2012.11.002
Davies, T. (2008). Environmental health impacts of East African rift volcanism. Environ. Geochem. Health 30 (4), 325–338. doi:10.1007/s10653-008-9168-7
De Cort, G., Mees, F., Renaut, R. W., Sinnesael, M., Van der Meeren, T., Goderis, S., et al. (2019). Late-Holocene sedimentation and sodium carbonate deposition in hypersaline, alkaline Nasikie Engida, southern Kenya Rift Valley. J. Paleolimnol 62 (3), 279–300. doi:10.1007/s10933-019-00092-2
Deino, A. L., Behrensmeyer, A. K., Brooks, A. S., Yellen, J. E., Sharp, W. D., and Potts, R. (2018). Chronology of the acheulean to middle stone age transition in eastern africa. Science 360 (6384), 95–98. doi:10.1126/science.aao2216
Devès, M. H., Reynolds, S., King, G. C. P., Küebler, S., Sturdy, D., and Godet, N. (2015). Insights from Earth Sciences into human evolution studies: the example of prehistoric landscape use in africa and the levant. Comptes Rendus Geosci. 347 (4), 201–211. doi:10.1016/j.crte.2015.02.012
Devès, M., Sturdy, D., Godet, N., King, G. C., and Bailey, G. N. (2014). Hominin reactions to herbivore distribution in the lower palaeolithic of the southern levant. Quat. Sci. Rev.
Dodson, C., and Standing, M. (1994). "Pressure-volume-temperature and solubility relations for natural-gas-water mixtures", in Paper presented at the Drilling and production practice, New York, January 1, 1994 (American Petroleum Institute).
Durkee, H., and Brown, F. H. (2014). Correlation of volcanic ash layers between the early pleistocene acheulean sites of Isinya, kariandusi, and Olorgesailie, Kenya. J. archaeological Sci. 49, 510–517. doi:10.1016/j.jas.2014.06.006
D’Allestro, P., and Parente, C. (2015). GIS application for NDVI calculation using Landsat 8 OLI images. Int. J. Appl. Eng. Res. 10 (21), 42099–42102.
Eckmeier, E., Kübler, S., Meya, A., and Mathai Rucina, S. (2020). “The role of geology and climate in soil nutrient variability-potential drivers for large ungulate migrations in the Serengeti ecosystem (Northern Tanzania, East Africa),” in EGU General Assembly Conference Abstracts, May 4-8, 2020. 13969.
Eggleton, R. A., Foudoulis, C., and Varkevisser, D. (1987). Weathering of basalt: changes in rock chemistry and mineralogy. Clays Clay Miner. 35 (3), 161–169.
Flanagan, F. J. (1969). U.S. Geological Survey standards-II. First compilation of data for the new U.S.G.S. rocks. Geochimica et Cosmochim. Acta 33 (1), 81–120. doi:10.1016/0016-7037(69)90094-5
Forsberg, B. R., Hashimoto, Y., Rosenqvist, Å., and Pellon de Miranda, F. (2000). Tectonic fault control of wetland distributions in the Central Amazon revealed by JERS-1 radar imagery. Quat. Int. 72 (1), 61–66. doi:10.1016/s1040-6182(00)00021-5
Gachuiri, C., Lukuyu, M., Lusweti, C., and Mwendia, S. W. (2012). Feeding dairy cattle in east Africa,” in East africa dairy development project. Editors Lukuyu, B., and Gachuiri, C.
Gaciri, S. J., and Davies, T. C. (1993). The occurrence and geochemistry of fluoride in some natural waters of Kenya. J. Hydrol. 143 (3), 395–412. doi:10.1016/0022-1694(93)90201-j
Gevera, P., Mouri, H., and Maronga, G. (2019). Occurrence of fluorosis in a population living in a high-fluoride groundwater area: Nakuru area in the Central Kenyan Rift Valley. Environ. Geochem. Health 41 (2), 829–840. doi:10.1007/s10653-018-0180-2
Ghiglieri, G., Pittalis, D., Cerri, G., and Oggiano, G. (2012). Hydrogeology and hydrogeochemistry of an alkaline volcanic area: the NE Mt. Meru slope (East African Rift - northern Tanzania). Hydrol. Earth Syst. Sci. 16 (2), 529–541. doi:10.5194/hess-16-529-2012
Guth, A., and Wood, J. (2013). Geological maps of the southern Kenya rift (nairobi, Suswa, Magadi)Michigan technical university. Digital map and chart series DMCH016. Boulder, Colorado: USGS.
Hahm, W. J., Riebe, C. S., Lukens, C. E., and Araki, S. (2014). Bedrock composition regulates mountain ecosystems and landscape evolution. Proc. Natl. Acad. Sci. USA 111 (9), 3338–3343. doi:10.1073/pnas.1315667111
Hemwall, J. B. (1957). The fixation of phosphorus by soils. Adv. Agron. 9, 95–112. doi:10.1016/S0065-2113(08)60110-8
Hengl, T., Leenaars, J. G. B., Shepherd, K. D., Walsh, M. G., Heuvelink, G. B. M., Mamo, T., et al. (2017). Soil nutrient maps of Sub-Saharan Africa: assessment of soil nutrient content at 250 m spatial resolution using machine learning. Nutr. Cycl Agroecosyst 109 (1), 77–102. doi:10.1007/s10705-017-9870-x
Hermanns, R. L., Niedermann, S., Garcia, A. V., Sosa Gomez, J., and Strecker, M. R. (2001). Neotectonics and catastrophic failure of mountain fronts in the southern intra-Andean Puna Plateau, Argentina. Geol 29 (7), 619–622. doi:10.1130/0091-7613(2001)029<0619:nacfom>2.0.co;2
Howard, D. A. (1963). Notes on animal diseases XXIII - mineral deficiency diseases. East Afr. Agric. For. J.28 (April), 191–194. doi:10.1080/00128325.1963.11661872
Ibs-von Seht, M., Blumenstein, S., Wagner, R., Hollnack, D., and Wohlenberg, J. (2001). Seismicity, seismotectonics and crustal structure of the southern Kenya Rift-new data from the Lake Magadi area. Geophys. J. Int. 146 (2), 439–453. doi:10.1046/j.0956-540x.2001.01464.x
Isaac, G. L. (1977). Olorgesailie: archaeological studies of a middle Pleistocene lake basin in Kenya. Chicago: University of Chicago Press.
Junginger, A., and Kübler, S. (2020). "One million years of human-landscape interaction in the Ethiopian and Kenyan rift-system," in EGU General assembly conference abstracts, May 4-8, 2020. 9748.
Kabete, J. M., Groves, D. I., McNaughton, N. J., and Mruma, A. H. (2012). A new tectonic and temporal framework for the Tanzanian Shield: implications for gold metallogeny and undiscovered endowment. Ore Geology. Rev. 48, 88–124. doi:10.1016/j.oregeorev.2012.02.009
King, G., Bailey, G., and Sturdy, D. (1994). Active tectonics and human survival strategies. J. Geophys. Res. 99 (B10), 20063–20078. doi:10.1029/94jb00280
King, G., and Bailey, G. (2006). Tectonics and human evolution. Antiquity 80 (308), 265–286. doi:10.1017/s0003598x00093613
King, G. C. P., and Vita-Finzi, C. (1981). Active folding in the Algerian earthquake of 10 October 1980. Nature 292 (5818), 22–26. doi:10.1038/292022a0
Köppen, W. (1900). Versuch einer Klassifikation der Klimate, vorzugsweise nach ihren Beziehungen zur Pflanzenwelt. Geographische Z., 6 (11), 593–611.
Krieger, G., Zink, M., Bachmann, M., Bräutigam, B., Schulze, D., Martone, M., et al. (2013). TanDEM-X: a radar interferometer with two formation-flying satellites. Acta Astronautica 89, 83–98. doi:10.1016/j.actaastro.2013.03.008
Kruckeberg, A. R. (2004). Geology and plant life: the effects of landforms and rock types on plants. University of Washington Press.
Kübler, S., Owenga, P., Reynolds, S. C., Rucina, S. M., and King, G. C. (2015). Animal movements in the Kenya Rift and evidence for the earliest ambush hunting by hominins. Sci. Rep. 5, 14011. doi:10.1038/srep14011
Kübler, S., Rucina, S., Reynolds, S., Owenga, P., Bailey, G., and King, G. (2016). Edaphic and topographic constraints on exploitation of the Central Kenya rift by large mammals and early hominins. Open Quat. 2, 5. doi:10.5334/oq.21
Kübler, S., King, G. C., Devès, M. H., Inglis, R. H., and Bailey, G. N. (2019). “Tectonic geomorphology and soil edaphics as controls on animal migrations and human dispersal patterns,” in Geological setting, palaeoenvironment and archaeology of the red sea. Editors N. M. A. Rasul, and I. C. F. Stewart (Cham: Springer), 653–673.
Kübler, S., Bailey, G., Rucina, S., Devès, M., and King, G. C. P. (2020). Rift dynamics and archaeological sites:. Archaeopress Archaeology, 42–63. doi:10.2307/j.ctvx5w983.8
Kyuma, R. K., Wahome, R. G., Kinama, J. M., and Waonga, V. O. (2016). Temporal relationship between climate variability, Prosopis juliflora invasion and livestock numbers in the drylands of Magadi, Kenya. Afr. J. Environ. Sci. Technol. 10 (4), 129–140. doi:10.5897/ajest2015.2034
Lee, H., Muirhead, J. D., Fischer, T. P., Ebinger, C. J., Kattenhorn, S. A., Sharp, Z. D., et al. (2016). Massive and prolonged deep carbon emissions associated with continental rifting. Nat. Geosci 9 (2), 145–149. doi:10.1038/ngeo2622
Li, Y., and Lindstrom, M. J. (2001). Evaluating soil quality-soil redistribution relationship on terraces and steep hillslope. Soil Sci. Soc. Am. J. 65 (5), 1500–1508. doi:10.2136/sssaj2001.6551500x
Mage, S. M., and Porder, S. (2013). Parent material and topography determine soil phosphorus status in the Luquillo Mountains of Puerto Rico. Ecosystems 16 (2), 284–294. doi:10.1007/s10021-012-9612-5
Margenot, A. J., Singh, B. R., Rao, I. M., and Sommer, R. (2016). “Phosphorus fertilization and management in soils of Sub-Saharan Africa,” in Soil phosphorus (Boca Raton: CRC Press), 151–208.
Maskall, J., and Thornton, I. (1996). The distribution of trace and major elements in Kenyan soil profiles and implications for wildlife nutrition. Geol. Soc. Lond. Spec. Publications 113 (1), 47–62. doi:10.1144/gsl.sp.1996.113.01.05
Mathews, B. W., Sollenberger, L. E., Nair, V. D., and Staples, C. R. (1994). Impact of grazing management on soil nitrogen, phosphorus, potassium, and sulfur distribution. J. Environ. Qual. 23 (5), 1006–1013. doi:10.2134/jeq1994.00472425002300050022x
McNaughton, S. J. (1990). Mineral nutrition and seasonal movements of African migratory ungulates. Nature 345 (6276), 613–615. doi:10.1038/345613a0
McSherry, M. E., and Ritchie, M. E. (2013). Effects of grazing on grassland soil carbon: a global review. Glob. Chang Biol. 19 (5), 1347–1357. doi:10.1111/gcb.12144
Moturi, W. K., Tole, M. P., and Davies, T. C. (2002). The contribution of drinking water towards dental fluorosis: a case study of Njoro Division, Nakuru District, Kenya. Environ. Geochem. Health 24 (2), 123–130. doi:10.1023/a:1014204700612
Muiruri, V. M. (2017). Late quaternary diatom and palynomorph stratigraphies and palaeoenvironments of the Koora graben and Lake Magadi basin, Kenya rift valley. Open Access Theses and Dissertations. 461.
Murray, M. G. (1995). Specific nutrient requirements and migration of wildebeest,” in Serengeti II:dynamics, management, and conservation of an ecosystem. Editors A. R. E. Sinclair, and P. Arcese (Chicago: University of Chicago Press), 231–256.
Mworia, J., Dallmeijer, A., and Jacobs, B. (1988). Vegetation and modern pollen rain at Olorgesailie, Kenya. Utafiti 1 (1), 1–22.
Olsen, S. R. (1954). Estimation of available phosphorus in soils by extraction with sodium bicarbonate. Washington, DC: US Department of Agriculture.
Owen, R. B., Muiruri, V. M., Lowenstein, T. K., Renaut, R. W., Rabideaux, N., Luo, S., et al. (2018a). Progressive aridification in East Africa over the last half million years and implications for human evolution. Proc. Natl. Acad. Sci. USA 115 (44), 11174–11179. doi:10.1073/pnas.1801357115
Owen, R. B., Renaut, R. W., and Lowenstein, T. K. (2018b). Spatial and temporal geochemical variability in lacustrine sedimentation in the East African Rift System: evidence from the Kenya Rift and regional analyses. Sedimentology 65 (5), 1697–1730. doi:10.1111/sed.12443
Owen, R. B., Renaut, R. W., Muiruri, V. M., Rabideaux, N. M., Lowenstein, T. K., McNulty, E. P., et al. (2019). Quaternary history of the lake Magadi basin, southern Kenya rift: tectonic and climatic controls. Palaeogeogr. Palaeoclimatol. Palaeoecol. 518, 97–118.
Pelletier, J. D., and Rasmussen, C. (2009). Quantifying the climatic and tectonic controls on hillslope steepness and erosion rate. Lithosphere 1 (2), 73–80. doi:10.1130/l3.1
Porder, S., Asner, G. P., and Vitousek, P. M. (2005). Ground-based and remotely sensed nutrient availability across a tropical landscape. Proc. Natl. Acad. Sci. USA 102 (31), 10909–10912. doi:10.1073/pnas.0504929102
Potts, R., Behrensmeyer, A. K., Deino, A., Ditchfield, P., and Clark, J. (2004). Small mid-pleistocene hominin associated with East African acheulean Technology. Science 305, 75–78. doi:10.1126/science.1097661
Potts, R., Behrensmeyer, A. K., and Ditchfield, P. (1999). Paleolandscape variation and early pleistocene hominid activities: members 1 and 7, Olorgesailie formation, Kenya. J. Hum. Evol. 37, 747–788. doi:10.1006/jhev.1999.0344
Potts, R., Behrensmeyer, A. K., Faith, J. T., Tryon, C. A., Brooks, A. S., Yellen, J. E., et al. (2018). Environmental dynamics during the onset of the middle stone age in eastern africa. Science 360 (6384), 86–90. doi:10.1126/science.aao2200
Potts, R. (1989). Olorgesailie: new excavations and findings in Early and Middle Pleistocene contexts, southern Kenya rift valley. J. Hum. Evol. 18 (5), 477–484. doi:10.1016/0047-2484(89)90076-6
Potts, R. (1996). Evolution and climate variability. Science 273 (5277), 922. doi:10.1126/science.273.5277.922
Potts, R. (1998). Environmental hypotheses of hominin evolution. Am. J. Phys. Anthropol. 107 (27), 93–136. doi:10.1002/(sici)1096-8644(1998)107:27+<93::aid-ajpa5>3.0.co;2-x
Potts, R. (2013). Hominin evolution in settings of strong environmental variability. Quat. Sci. Rev. 73 (0), 1–13. doi:10.1016/j.quascirev.2013.04.003
Potts, R., Shipman, P., and Ingall, E. (1988). Taphonomy, paleoecology, and hominids of Lainyamok, Kenya. J. Hum. Evol. 17 (6), 597–614. doi:10.1016/0047-2484(88)90087-5
Pratt, D. J., Greenway, P. J., and Gwynne, M. D. (1966). A classification of East African rangeland, with an appendix on terminology. J. Appl. Ecol. 3, 369–382. doi:10.2307/2401259
Quigley, K. M., Donati, G. L., and Anderson, T. M. (2017). Variation in the soil ‘silicon landscape’explains plant silica accumulation across environmental gradients in Serengeti. Plant and Soil 410 (1-2), 217–229. doi:10.1007/s11104-016-3000-4
Rajakaruna, N., and Boyd, R. S. (2019). “Edaphic Factor,” in Reference module in earth systems and environmental sciences. Editor S. A. Elias (Oxford, United Kingdom: Elsevier).
Renaut, R. W., Owen, R. B., Lowenstein, T. K., De Cort, G., Mcnulty, E., Scott, J. J., et al. (2020). The role of hydrothermal fluids in sedimentation in saline alkaline lakes: evidence from Nasikie Engida, Kenya Rift Valley. Sedimentology 68 (1), 108–134. doi:10.1111/sed.12778
Reynolds, S. C., Bailey, G., and King, G. C. (2011). Landscapes and their relation to hominin habitats: case studies from Australopithecus sites in eastern and southern Africa. J. Hum. Evol. 60, 281–298. doi:10.1016/j.jhevol.2010.10.001
Richardson, A. E., Hocking, P. J., Simpson, R. J., and George, T. S. (2009). Plant mechanisms to optimise access to soil phosphorus. Crop Pasture Sci. 60 (2), 124–143. doi:10.1071/cp07125
Roche, H., Brugal, J.-P., Lefevre, D., Ploux, S., and Texier, P.-J. (1988). Isenya: état des recherches sur un nouveau site acheuléen d’Afrique orientale. Afr. Archaeol Rev. 6 (1), 27–55. doi:10.1007/bf01117111
Roering, J. J., Kirchner, J. W., and Dietrich, W. E. (2001). Hillslope evolution by nonlinear, slope-dependent transport: steady state morphology and equilibrium adjustment timescales. J. Geophys. Res. 106 (B8), 16499–16513. doi:10.1029/2001jb000323
Seymour, C. L., Milewski, A. V., Mills, A. J., Joseph, G. S., Cumming, G. S., Cumming, D. H. M., et al. (2014). Do the large termite mounds of Macrotermes concentrate micronutrients in addition to macronutrients in nutrient-poor African savannas?. Soil Biol. Biochem. 68, 95–105. doi:10.1016/j.soilbio.2013.09.022
Shand, C. (2007). “Plant nutrition for food security. A guide for integrated nutrient management,” in Experimental agriculture. Editors R. N. Roy, A. Finck, G. J. Blair, and H. L. S. Tandon (Rome: Food and Agriculture Organization of the United Nations 2006), 348, 43 (1), 132.
Shipman, P., Potts, R., and Pickford, M. (1983). Lainyamok, a new middle Pleistocene hominid site. Nature 306 (5941), 365–368. doi:10.1038/306365a0
Simonson, R. W. (1995). Airborne dust and its significance to soils. Geoderma 65 (1-2), 1–43. doi:10.1016/0016-7061(94)00031-5
Sindiga, I. (1984). Land and population problems in kajiado and Narok, Kenya. Afr. Stud. Rev. 27 (1), 23–39. doi:10.2307/523948
Sombroek, W. G., Braun, H., and Van der Pouw, B. (1982). Exploratory soil map and agro-climatic zone map of Kenya, 1980. Scale 1: 1,000,000. Nairobi: Kenya Soil Survey.
Stokes, M., Mather, A. E., Belfoul, A., and Farik, F. (2008). Active and passive tectonic controls for transverse drainage and river gorge development in a collisional mountain belt (Dades Gorges, High Atlas Mountains, Morocco). Geomorphology 102 (1), 2–20. doi:10.1016/j.geomorph.2007.06.015
Sturdy, D. A., and Webley, D. P. (1988). Palaeolithic geography: or where are the deer?. World Archaeology 19 (3), 262–280. doi:10.1080/00438243.1988.9980041
Sturdy, D., Webley, D., and Bailey, G. (1997). “The palaeolithic geography of Epirus,” in Klithi: palaeolithic settlement and quaternary landscapes in northwest Greece. Editor G. Bailey (Cambridge: McDonald Institute for Archaeological Research), 2, 587–614.
Tardy, Y. (1997). Petrology of laterites and tropical soils. Rotterdam, Netherlands: AA Balkema.
Thornthwaite, C. W. (1948). An approach toward a rational classification of climate. Geographical Rev. 38 (1), 55–94. doi:10.2307/210739
Touber, L. (1977). Soil map of kajiado district (rift valley province, Kenya), current land suitability for extensive cattle range. Nairobi: National Soil Maps (EUDASM), 24.
Trauth, M. H., Maslin, M. A., Deino, A., and Strecker, M. R. (2005). Late cenozoic moisture history of East Africa. Science 309 (5743), 2051–2053. doi:10.1126/science.1112964
Tucker, G. E., and Bras, R. L. (1998). Hillslope processes, drainage density, and landscape morphology. Water Resour. Res. 34 (10), 2751–2764. doi:10.1029/98wr01474
Vincens, A., and Casanova, J. (1987). Modern background of Natron-Magadi basin (Tanzania-Kenya) : physiography, climate, hydrology and vegetation. Contexte actuel du bassin Natron-Magadi (Tanzanie-Kenya): physiographie, climat, hydrologie et végétation. Sci. Geol. Bull. Mem. 40 (1), 9–21. doi:10.3406/sgeol.1987.1747
Keywords: rock-soil interface, tectonic geomorphology, east african rift system, plant-available soil nutrients, human-landscape interactions
Citation: Kübler S, Rucina S, Aßbichler D, Eckmeier E and King G (2021) Lithological and Topographic Impact on Soil Nutrient Distributions in Tectonic Landscapes: Implications for Pleistocene Human-Landscape Interactions in the Southern Kenya Rift. Front. Earth Sci. 9:611687. doi: 10.3389/feart.2021.611687
Received: 29 September 2020; Accepted: 08 February 2021;
Published: 26 March 2021.
Edited by:
Verena E. Foerster, University of Cologne, GermanyReviewed by:
Emily J. Beverly, University of Houston, Houston, United StatesFelix Bachofer, German Aerospace Center, Weßling, Germany
Copyright © 2021 Kübler, Rucina, Aßbichler, Eckmeier and King. This is an open-access article distributed under the terms of the Creative Commons Attribution License (CC BY). The use, distribution or reproduction in other forums is permitted, provided the original author(s) and the copyright owner(s) are credited and that the original publication in this journal is cited, in accordance with accepted academic practice. No use, distribution or reproduction is permitted which does not comply with these terms.
*Correspondence: S. Kübler, cy5rdWVibGVyQGxtdS5kZQ==