- 1Department of Geosciences, Georgia State University, Atlanta, GA, United States
- 2Institute of Geography Education, University of Cologne, Cologne, Germany
- 3Department of Earth Sciences, The University of Western Ontario, London, ON, Canada
- 4Department of Earth and Planetary Sciences, Rutgers University, Piscataway, NJ, United States
- 5Department of Geosciences, University of Tübingen, Tübingen, Germany
- 6Department of Climate Geochemistry, Max Planck Institute for Chemistry, Mainz, Germany
- 7Institute of Geography, University of Cologne, Cologne, Germany
- 8Institute of Geosciences, University of Potsdam, Potsdam, Germany
- 9Department of Geography and Earth Sciences, Aberystwyth University, Aberystwyth, United Kingdom
- 10Botany Department, School of Natural Sciences, Trinity College, Dublin, Ireland
- 11School of Earth Sciences, Addis Ababa University, Addis Ababa, Ethiopia
We present new mineralogical and geochemical data from modern sediments in the Chew Bahir basin and catchment, Ethiopia. Our goal is to better understand the role of modern sedimentary processes in chemical proxy formation in the Chew Bahir paleolake, a newly investigated paleoclimatic archive, to provide environmental context for human evolution and dispersal. Modern sediment outside the currently dry playa lake floor have higher SiO2 and Al2O3 (50–70 wt.%) content compared to mudflat samples. On average, mudflat sediment samples are enriched in elements such as Mg, Ca, Ce, Nd, and Na, indicating possible enrichment during chemical weathering (e.g., clay formation). Thermodynamic modeling of evaporating water in upstream Lake Chamo is shown to produce an authigenic mineral assemblage of calcite, analcime, and Mg-enriched authigenic illitic clay minerals, consistent with the prevalence of environments of enhanced evaporative concentration in the Chew Bahir basin. A comparison with samples from the sediment cores of Chew Bahir based on whole-rock MgO/Al2O3, Ba/Sr and authigenic clay mineral δ18O values shows the following: modern sediments deposited in the saline mudflats of the Chew Bahir dried out lake bed resemble paleosediments deposited during dry periods, such as during times of the Last Glacial Maximum and Younger Dryas stadial. Sediments from modern detrital upstream sources are more similar to sediments deposited during wetter periods, such as the early Holocene African Humid Period.
Introduction
The field of sedimentary geochemistry is at the forefront of both outcrop- and core-based paleoclimate research. Aided by the introduction of X-ray fluorescence (XRF) core scanning techniques, down-core patterns of element intensities and ratios in both marine and lake sediments are routinely applied as proxies of past climate changes (e.g., Croudace et al., 2006, 2019; Lyons et al., 2015; Gebregiorgis et al., 2020b). Although the link between the chemistry of deposited weathering products and climate is complex, the distribution of mobile versus immobile elements in sediment likely varies in accordance with climate variables (e.g., temperature, precipitation; e.g., Harriss and Adams, 1966; Nesbitt, 1979; Chesworth et al., 1981; Middelburg et al., 1988). Element intensities (e.g., Zr, Ti, K) and ratios (e.g., K/Zr, K/Ti, K/Al, and K/Rb) are routinely used to trace a wide range of climate-related processes such as changes in rainfall amount (e.g., Foerster et al., 2012; Hendy et al., 2015) and intensity of weathering processes (e.g., Burnett et al., 2011; Tian et al., 2011; Clift et al., 2014) within lake catchments.
The application of XRF-generated elemental proxies in paleoclimatology, however, is not without its pitfalls. Depositional variability, hiatuses and erosion, diagenesis, and pedogenesis commonly work together to obscure or overprint primary geochemical signals preserved in sediments. Authigenic mineral formation can also control lake sediment geochemistry, particularly in saline lakes (Deocampo and Jones, 2014). Indeed, in many African lakes, clay minerals rich in Al, or zeolites rich in Ca or K, have been shown to alter in saline and alkaline lakes to produce Mg-rich authigenic clay minerals and Na-rich analcime (e.g., Surdam and Eugster, 1976; Singer and Stoffers, 1980; Trauth et al., 2001; Deocampo, 2004, 2015). As a result, large discrepancies between lake records remain unresolved, leading to difficulties in interpreting proxy records of climate (e.g., Trauth et al., 2003). These challenges are in large part due to an incomplete understanding of proxy formation and are particularly relevant in paleolimnology, where down-core variability of selected elements in the sediment may have little direct relation with the rate and intensity of weathering of the surrounding catchment, but might rather reflect post-depositional hydrochemical conditions.
The potassium (K) record of the Chew Bahir basin provides an excellent example demonstrating the complexity of interpreting highs and lows in K content in the sediment as past water balance changes in the catchment (Foerster et al., 2012; Fischer et al., 2020). Foerster et al. (2018) considered multiple hypotheses to explain the K record, concluding that K enrichment in the sediment could not entirely indicate episodes of enhanced detrital input, but rather incipient low temperature K-fixation during illitization associated with Mg uptake and clay mineral layer charge increase (Deocampo and Tactikos, 2010; Deocampo, 2015). It is worth noting that the temporal variability of the K record is remarkably consistent with half a dozen well-dated proxy records from continental Africa (e.g., Brown et al., 2007; Junginger and Trauth, 2013; Tierney and deMenocal, 2013; Otto-Bliesner et al., 2014; Shanahan et al., 2015).
The present study takes its impetus from Foerster et al. (2018) and has three main objectives. The study’s core objective is to describe the major input and alteration processes utilizing new geochemical data in modern sediments collected from the Chew Bahir basin. Second, by comparing the new geochemical data from the modern sediments with PHREEQC geochemical modeling, where the acronym PHREEQC stands for PH (pH), RE (redox), EQ (equilibrium), and C (program written in C), of local waters, the study aims to improve our understanding of relevant hydrogeochemical processes in the Chew Bahir catchment. Third, the study aims to further refine interpretations of the Chew Bahir K record using clay mineralogical and geochemical analysis of modern sediments collected from the Chew Bahir catchment. Within this framework, this study is aimed at advancing our understanding of such climate proxies, and the development of a reliable high-resolution environmental context for the newly investigated site of Chew Bahir. A preliminary attempt is made to investigate whether oxygen-isotope measurements of authigenic clay minerals covering notable warm/wet (e.g., the early-middle Holocene) and cold/dry intervals (e.g., the Last Glacial Maximum, LGM) suggest similar changes in wet and dry conditions inferred from the Chew Bahir K record.
Geomorphology of the Chew Bahir Basin
The Chew Bahir basin is a hydrographic and sedimentary system located at the southern sector of the Main Ethiopian Rift, northeast of the Omo-Turkana basin of southern Ethiopia and northern Kenya (Figures 1a–d). It forms part of a ∼250 km wide broadly rifted zone, mainly composed of Pliocene-Holocene sediment-filled basins underlying strongly uplifted Precambrian blocks (e.g., Ebinger et al., 2000). Oligocene basalts and subordinate rhyolites, trachytes, tuffs and ignimbrites cover the Precambrian basement units near the northeastern, northern and northwestern parts of the catchment. The Teltele plateau, which consists of Miocene basalts and rhyolite, trachyte and felsic tuff, is located in the eastern part of the Chew Bahir catchment (Figure 1d; see also Davidson, 1983). The northern part of the Teltele plateau extends to the Konso upland, which is drained by the Segen River, one of the major rivers draining to the Weyto River, and then to the Chew Bahir basin (Fischer et al., 2020).
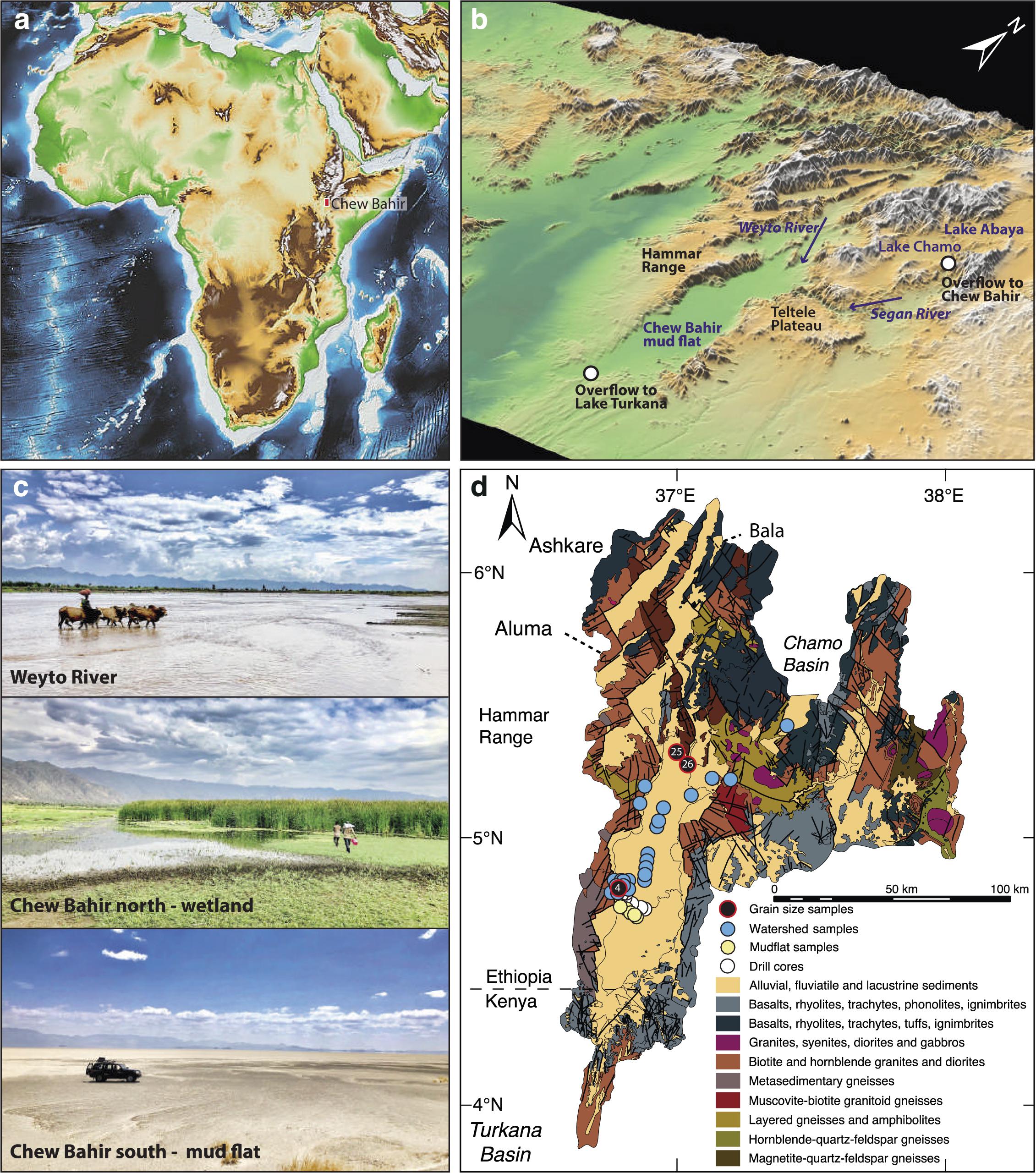
Figure 1. Elevation map of (a) eastern Africa with (b) 3D, (c) field, and (d) geologic representation of the Chew Bahir basin. Filled blue and yellow-green circles in (d) show sampling locations for modern watershed (WS) and mudflat (MF) samples, respectively. Filled black circles in (d) show locations of samples collected from the Chew Bahir catchment for grain size analysis.
The Chew Bahir basin is a deep tectonic basin, where a nearly flat, ∼30 km wide and ∼100 km long playa surface is bounded by a ∼500 m high tectonic escarpments to its west and east. Formation of shallow but wide alluvial fans, the biggest of which is a 20 km by 10 km fan located just west of the Chew Bahir coring sites, has been the major geomorphological features at the borders between the basin and the adjacent highland graben shoulders. The Weyto River flows in a north-south direction, forming a wide and shallow deltaic system before it disappears into the playa wetland (Figure 1c). The northern part of the flat basin is covered by relatively dense grassland vegetation mostly inhibiting aeolian geomorphological processes. Only on the vegetation-free, strongly desiccated southernmost (∼20 km long) part of the basin can local aeolian deflation, remobilization and sedimentation of fine sediments by “dust-devils” or dust storms be observed. In the extreme southern part of the Chew Bahir basin, small aeolian geomorphological features like “kupsten” dunes appear close to the recent “coastline.”
The Chew Bahir tectonic basin, which has an elevation of ∼500 masl, lies on the southeastern side of the western Ethiopian highlands, which are greater than ∼2,500 masl (Figures 1a,b). The deep basin is thus located on the lee of potential dust sources, especially the Sahara. Likewise, the ∼1,800 m high Teltele plateau, which gently descends to the east, blocks potential dust plumes from reaching the southeastern Ethiopian lowlands. The paleo-lake ’periodically fills with water (up to 2 m in depth) supplied primarily via the Weyto River. Modern dust input in the form of local short events of “dust-devils” occurs during remobilization and deposition of dust from the loose playa sediments on the strongly desiccated surface in the extreme southern part of the basin.
In sum, the Hammar Range to the west and the highlands to the north and northeast, consisting of Late Proterozoic granite and gneiss, are therefore the primary sediment sources to the Chew Bahir basin (Foerster et al., 2012). All surface samples were therefore collected between sub-watersheds from riverbeds or the desiccated lake floor to help improve our understanding of proxy formation in this region (see sampling locations in Figure 1d and methods). However, it should be noted that our study cannot be considered an exhaustive survey of all geomorphological processes active in the larger Chew Bahir catchment.
Hydrogeological and Climatic Features of the Chew Bahir Catchment
Today the Chew Bahir basin and catchment area has two rainy seasons, during boreal spring and autumn, owing to the North–South migration of the tropical African rain belt (Figures 2a–c). The tropical African rain belt is commonly referred to as the intertropical convergence zone (ITCZ) but the twice-yearly passing of the tropical African rain belt may not necessarily be synchronized with the annual cycle of insolation (e.g., Nicholson, 2018). Catchment wide mean annual rainfall is in the order of 900–1,000 mm, and the boreal spring rainy season (i.e., March–May) accounts for >50% of the annual total (Fischer et al., 2020).
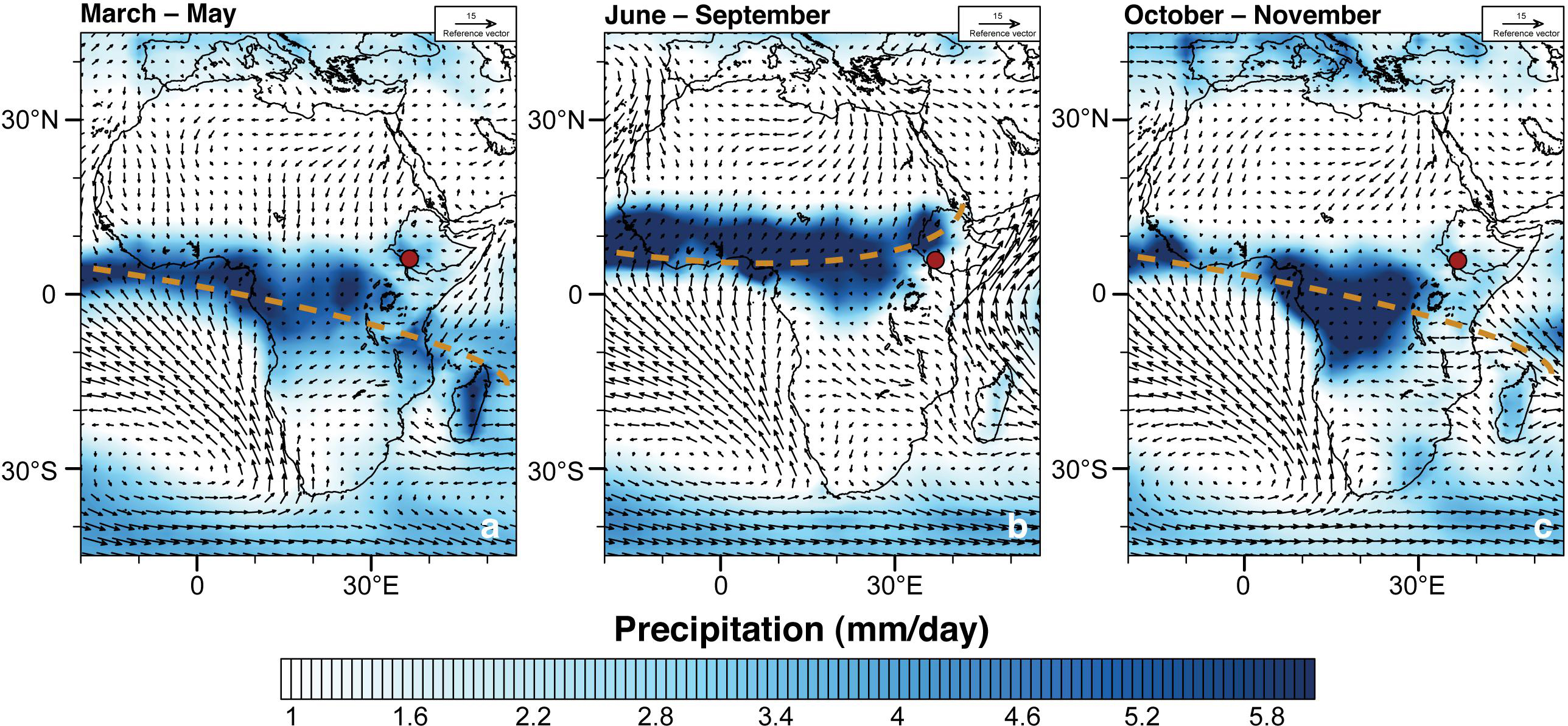
Figure 2. Seasonal precipitation (mm/day) patterns in eastern Africa during (a) March–May, (b) June–September, and (c) October–November; mean precipitation for the period 1979–2015 at 1,000 hPa geopotential height (gph) fields. Global Precipitation Climatology Centre (GPCC) monthly precipitation data is acquired from the National Oceanic and Atmospheric Administration (NOAA) and can be accessed at https://www.esrl.noaa.gov/psd/. Thick, dashed, brown lines indicate the approximate location of the tropical African rain belt. Red circle indicates the location of the drill site.
Water balance model estimates for paleo-lake Chew Bahir, based on the Surface Energy Balance Algorithm for Land, estimate an actual evaporation rate for the catchment of 847 mm/a (Fischer et al., 2020). Although mesoscale convective processes and topography also play an important role in the development of rain-bearing systems in eastern Africa (e.g., Hession and Moore, 2011), aridity in the catchment area is linked to large-scale divergence in the lower troposphere (Nicholson, 2016). The prevailing aridity in northern Kenya and southern Ethiopia is also associated with the Turkana Jet, which flows at low levels with highest wind speeds at the 850 mb pressure level; maximum aridity over the region occurs when the Turkana Jet is at minimum speed (Nicholson, 2016). There is also a negative correlation between the strength of the Turkana Jet and low level divergence, and rainfall over that region (e.g., Sun et al., 1999). On a broader scale, rainfall patterns in eastern Africa are also sensitive to sea surface temperature variability in both the Indian and Pacific Oceans (e.g., Gebregiorgis et al., 2019).
During the last African Humid Period (AHP), 20–30% more precipitation led to lake level rises of Lake Abaya-Chamo (Fischer et al., 2020). At that time, overflow spilled into the Chew Bahir basin forming paleo-lake Chew Bahir, which in turn overspilled into Lake Turkana after reaching the overflow sill at 543 masl (Fischer et al., 2020).
Materials and Methods
Sampling
Thirty-four surface samples from the main catchment areas of paleo-lake Chew Bahir were collected in October 2018 for mineralogical, and major, minor and trace element analyses (Table 1). Twenty-eight of these surface samples were collected between watersheds from riverbeds (henceforth referred to as watershed samples or WS samples). Six samples were collected from the desiccated lake floor (henceforth referred to as mudflat samples or MF samples). Twelve samples from the Chew Bahir pilot drill core CB-01 were analyzed from selected wetter and drier intervals of the late Pleistocene and Holocene based on the Chew Bahir K record (Figure 3; Foerster et al., 2012, 2018). Samples collected from wetter intervals (henceforth referred to as WI) include 6 samples from the AHP interval, ∼15–5 ka (Demenocal et al., 2000; Shanahan et al., 2015). Samples collected from drier intervals (henceforth referred as DI) include 2 samples each from the LGM (∼21 ka BP; Clark et al., 2009), Younger Dryas (YD; ∼12.9–11.7 ka BP; Roberts et al., 1993) and Late Holocene (representing the last ∼2 ka BP of the record; Figure 3). Three samples (i.e., W4, W25, and W26) were collected in November 2018 for grain size analysis (Figure 1). W25 and W26 were collected from the Weyto River catchment entering into the Chew Bahir basin. W26 was collected from a freshly accumulated riverbank, while W25 represents an alluvial fan accumulation site between Weyto town and Weyto River. W4 was collected from a deep incised river at the foot of the Hammar range in the southern part of the Chew Bahir basin (see Figure 1 for sample locations).
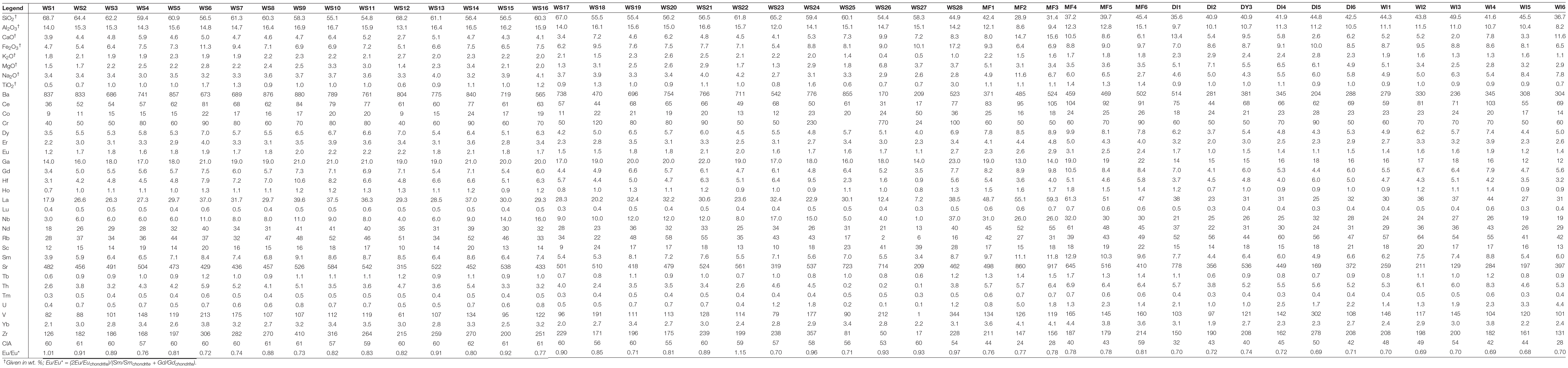
Table 1. Major/minor (wt. %) and trace (including rare earth) (ppm) element compositions of modern and down-core Chew Bahir samples.
X-Ray Diffraction, Electron Microprobe and Grain Size Analysis
The mineralogical composition of powdered bulk sediments and oriented mounts of clay particles (<2 μm) were analyzed using X–ray powder diffraction (XRD) at the Department of Geosciences, Georgia State University. The clay fractions were extracted according to Stokes’ settling velocity principle in 1,000 ml glassware. Randomly-oriented, powdered bulk samples were analyzed using Cu-Kα radiation (45 kV, 40 mA) from 5 to 70° 2θ, with a 0.02° 2θ step and 60 s per step, to identify a suite of non-clay minerals. Preferred-oriented samples of the <2 μm size fraction were analyzed in both air-dried (AD) and ethylene glycol-solvated (EG) states from 3 to 35° 2θ with a 0.02° 2θ step at 60 s per step, following the principles of Moore and Reynolds (1997).
The octahedral cation ratios [(Mg/(Al + Fe)] of the <0.1 μm size-fractions were determined using the JEOL-8200 electron microprobe at Rutgers University. The analyses were performed at 15 Kv accelerating voltage and 15 mA beam current, using a suite of in-house standards, including several hydroxyl-bearing sheet silicates, and derived from multiple sources. Data corrections were done using ZAF procedures. Since the samples are intrinsically fine-grained powders, normal polishing procedures could not be used and instead the samples had the form of compressed pellets that were sufficiently smooth to permit analysis. The presence of unquantified inter-grain voids reduces the overall density of the samples and, together with unanalyzed hydroxyl fractions, prevents the analysis totals reaching the nominal 100%.
Grain size analysis was carried out at the laboratory of the Geographic Institute, University of Cologne. Analysis was conducted in 116 channels from 0.04 to 2,000 mm with a Laser Diffraction Particle Size Analyzer (LS 13320 Beckmann CoulterTM), using the Fraunhofer optical model. Before analysis, organic and carbonate content was removed using 15% H2O2 and 10% HCL, respectively. Grain-size parameters are based on Folk and Ward (1957) and were calculated by GRADISTAT software version 8 (Blott and Pye, 2001).
Quantitative Multi-Element and Oxygen-Isotope Analysis
A total of 46 samples were analyzed for major, minor and trace elements at the Activation Laboratories Ltd. in Ontario, Canada following the analytical procedure termed “Code 4LITHO Major Elements Fusion ICP (WRA)/Trace Elements Fusion ICP/MS.” Major, minor and trace element data were acquired by ICP-OES fusion and ICP-MS, respectively. Samples were analyzed in a batch system with each batch containing a method reagent blank, certified reference material and duplicates. Samples were analyzed for major oxides and selected trace elements (Code 4B) using Perkin Elmer Sciex ELAN 6000, 6100, or 9000 ICP/MS, providing a precision of ±5% and 10% for major and minor oxides, and trace elements at 100x the detection limit, respectively. Seven USGS and CANMET certified reference materials were used for calibration and one of the 7 standards was analyzed for every group of 10 samples. Three blanks and duplicates were analyzed per group of samples and every 15 samples, respectively.
The chemical index of alteration (CIA) was calculated on the basis of major element chemistry following Nesbitt and Young (1982) as follows:
Six samples (3 each from DI and WI) were selected for clay mineral purification and subsequent oxygen-isotope analysis. Ultrafine clay size-fractions (<0.1 μm) were separated from the bulk sediments following routine centrifugation protocols (Moore and Reynolds, 1997) for XRD analysis. Samples were analyzed in both AD and EG states from 3 to 35° 2θ. Oxygen-isotope analyses were performed on the same samples at the Laboratory for Stable Isotope Science at the University of Western Ontario following Libbey et al. (2013) and references therein. The results are presented in the normal δ-notation relative to the VSMOW-SLAP calibrated scale in parts per thousand (‰). Reproducibility was better than ±0.2‰. VSMOW-SLAP calibrated standards analyzed as unknowns returned δ18O = + 11.54 ± 0.2‰ (quartz; n = 2) and +10.18 ± 0.01‰ (carbon dioxide; n = 2), which compares well with their accepted values of +11.5‰ and +10.25‰, respectively (Huggett et al., 2017).
Geochemical Modeling
Geochemist’s Workbench® (Bethke et al., 2019) was used to model the evaporative concentration of water with a dissolved solid composition equivalent to Lake Chamo, a freshwater lake that drains through the Segen River to the Chew Bahir basin (Table 2; Ayenew, 2005). Equilibrium reactions were modeled iteratively to dryness, assuming equilibrium gas exchange with the atmosphere. Equilibrium precipitation-dissolution was assumed with respect to gibbsite, and the precipitation of dolomite, talc, chrysotile, and mica were all suppressed as reaction kinetics likely do not favor these authigenic phases (Deocampo, 2010; Deocampo and Jones, 2014).
Results
Mineralogy and Grain Size Distribution
The mineralogical assemblage of the WS samples is composed primarily of sand- and silt-size particles containing a higher fraction of non-clay mineral silicate phases than the MF samples, which comprised mostly clay minerals. In addition to illite, smectite, and kaolinite, the <2 μm size-fraction of the WS samples also contain quartz and feldspar, and trace amounts of calcite and analcime (Figure 4). Analcime content increases in the MF samples that are transitional to the desiccated lake floor of the Chew Bahir basin. The <2 μm size-fraction of the MF samples contains illite, smectite and kaolinite (Figure 4).
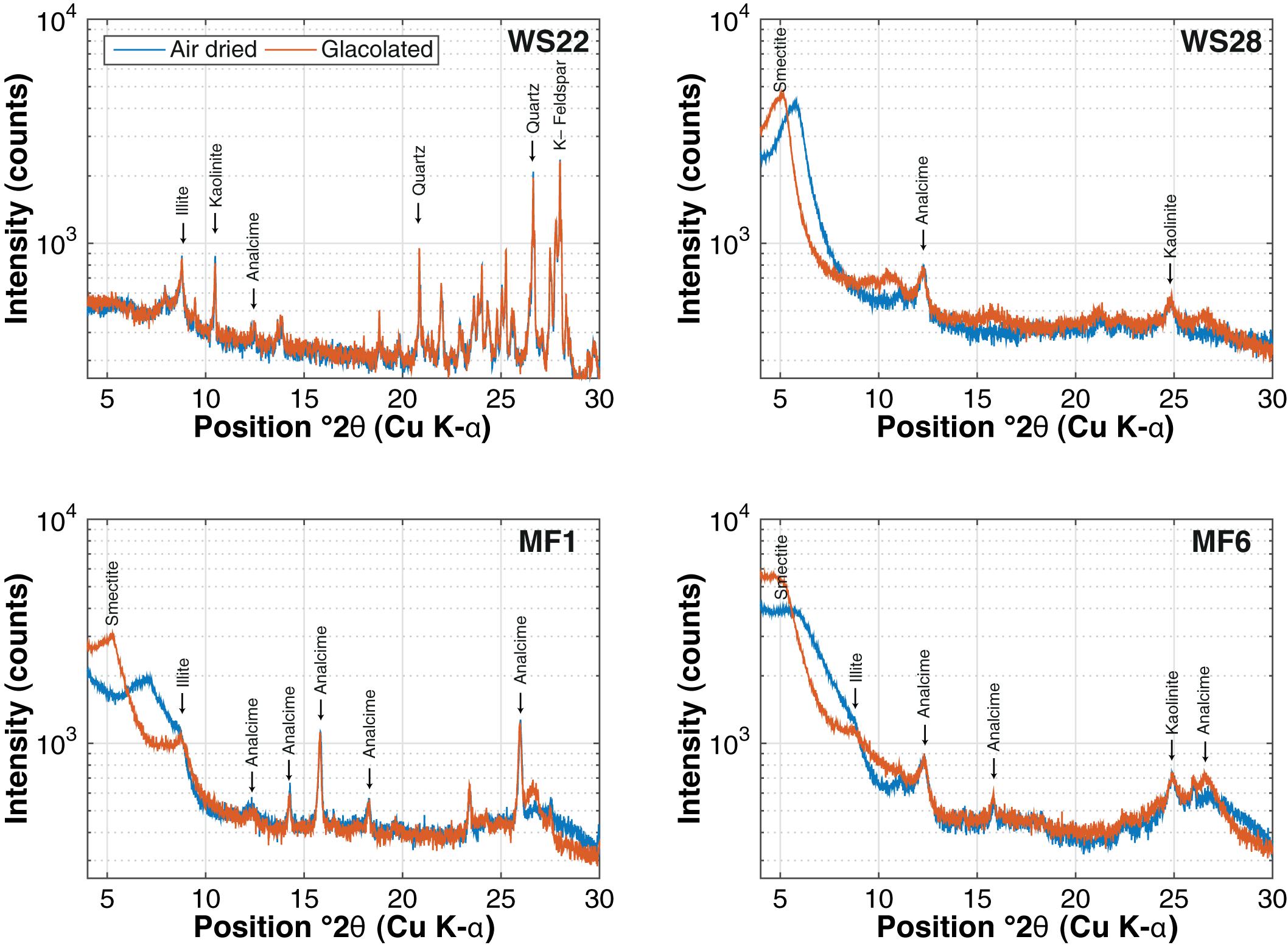
Figure 4. XRD patterns of preferred-oriented, air-dried and glycolated mounts of the <2 μm size-fraction for four (4) WS and MF samples of modern Chew Bahir sediments. WS, modern watershed; and MF, modern mudflat. XRD patterns for air-dried samples are shown in blue, and those for samples treated with ethylene glycol, red.
Grain-size data of three samples are shown in Supplementary Figure 2. Two of the three samples (i.e., W26 and W4) show unimodal features, while one (W25) shows multimodal features (Supplementary Figure 2). All samples contained relatively little material in the 2–50 μm aeolian size range (Supplementary Figure 2). The amount of material within the 2–50 μm fraction in W26 and W4, and W25 samples is ∼14% and 36.8%, respectively.
Whole-Rock and Authigenic Clay Mineral Geochemistry
Major and trace element concentrations of the different sample groups show clear intergroup differences in the enrichment and depletion of certain major elements (Table 1). WS samples have higher SiO2 (50–70 wt.%) contents compared to the MF samples (Figure 5 and Table 1). In WS samples, as SiO2 content increases, most other element abundances decrease, suggesting their association with non-quartz minerals. K2O contents, however, remain relatively constant as SiO2 varies. In MF samples, major element contents increase with increasing SiO2, except for CaO, which strongly decreases. On the A–CN–K diagram (Figure 6a), WS samples are richest in Al2O3 (∼50–70%); CaO + Na2O contents in MF samples trend toward the cluster of WS samples while their K2O contents remain at ≤10%. On the A–CNK–FM diagram (Figure 6b), the CaO + Na2O + K2O content of some MF samples increases to ∼50% approaching the cluster of WS samples. CIA values (Table 1) range between 24 and 62 with WS samples consistently yielding higher values. Mean CIA values for WS and MF samples are 59 and 40, respectively. Mean CIA values for WI and DI samples are 44 and 42, respectively.
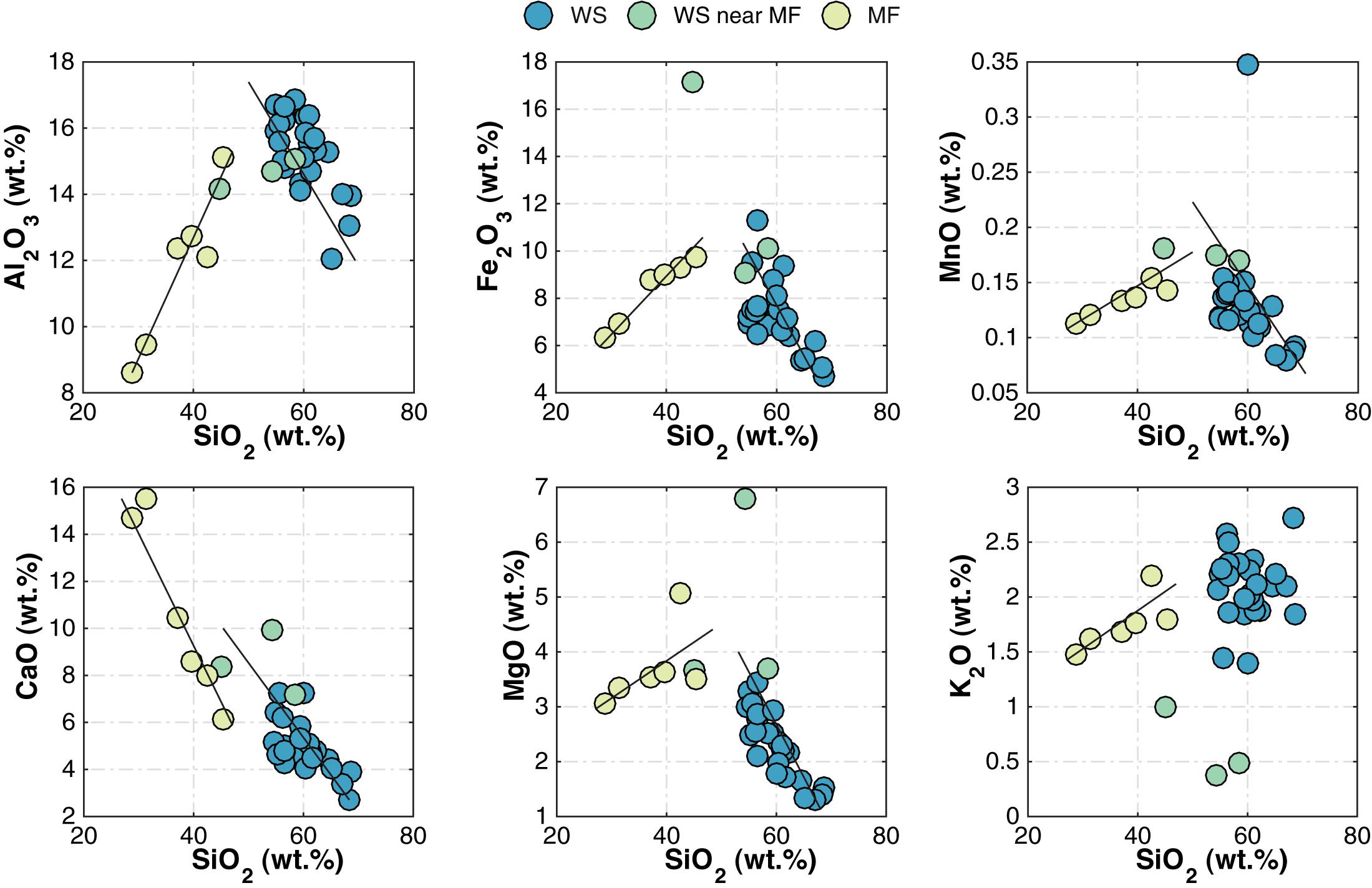
Figure 5. Relationships between major element oxide and SiO2 contents in WS and MF samples. WS, modern watershed; and MF, modern mudflat.
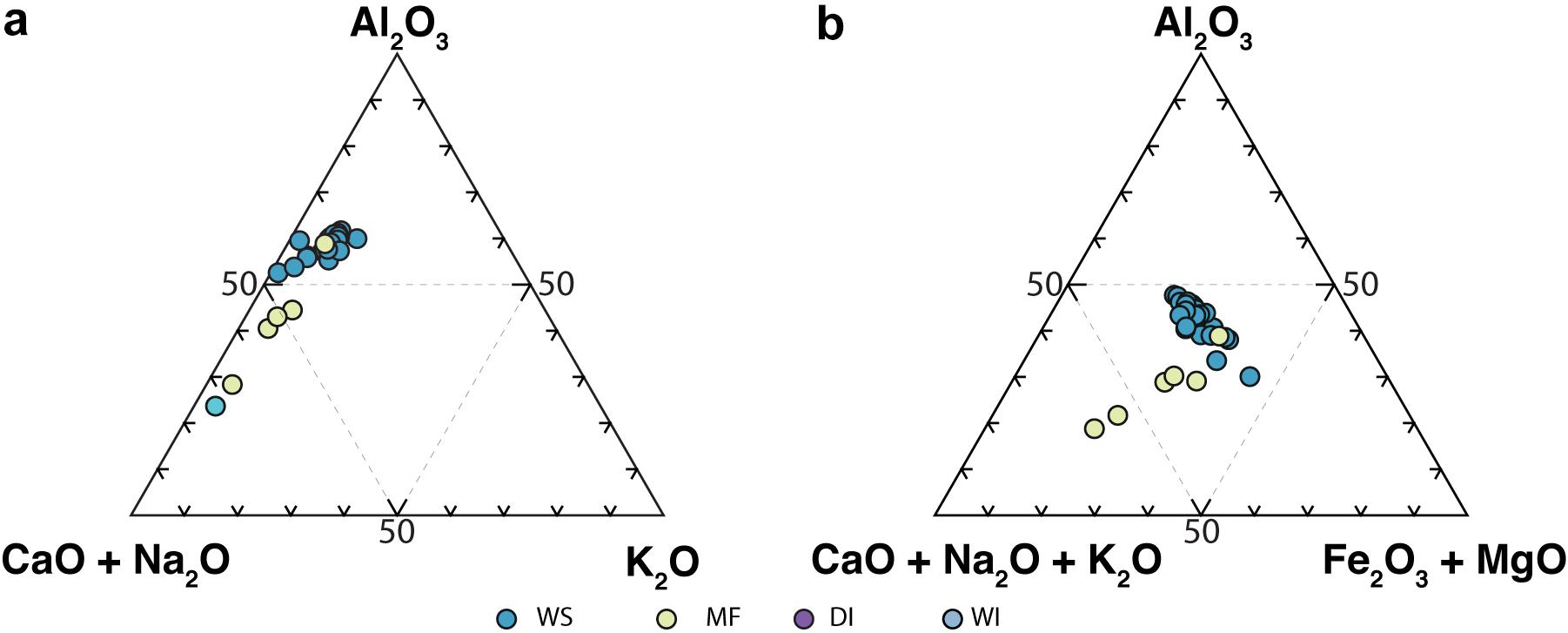
Figure 6. Ternary plots of (a) A-CN-K, and (b) A-CNK-FM illustrating the degree of alteration in WS, MF, WI, and DI samples. WS, modern watershed sample; and MF, modern mudflat sample.
Upper Continental Crust-normalized (McLennan, 2001) chemical compositions of the sediments show that MF samples, on average, are enriched in elements such as Mg, Ca, Ce, Nd, and Na, and depleted of elements such as Ba and Zr relative to WS samples (Figures 7a,b). DI samples, on average, are enriched in Mg and depleted of K relative to WS samples (Figures 7c,d). Chondrite-normalized REE patterns reveal minor differences between the WS and MF samples (Figure 8). WS samples [e.g., mean (La/Sm)N = 2.61] are slightly depleted of Light REE (LREE) compared to MF samples [e.g., mean (La/Sm)N = 3.07] (Figure 8). Similarly, no significant differences are observed between Heavy REE (HREE) in WS [e.g., average (Gd/Yb)N = 1.61] and MF [e.g., average (Gd/Yb)N = 1.86] samples. No preferential removal of LREE or HREE is observed for either DI or WI samples (Figure 8). Eu anomalies in WS (mean Eu/Eu∗ = 0.85), MF (mean Eu/Eu∗ = 0.78), DI (mean Eu/Eu∗ = of 0.71), and WI (mean Eu/Eu∗ = 0.69) samples are also virtually identical (Table 1).
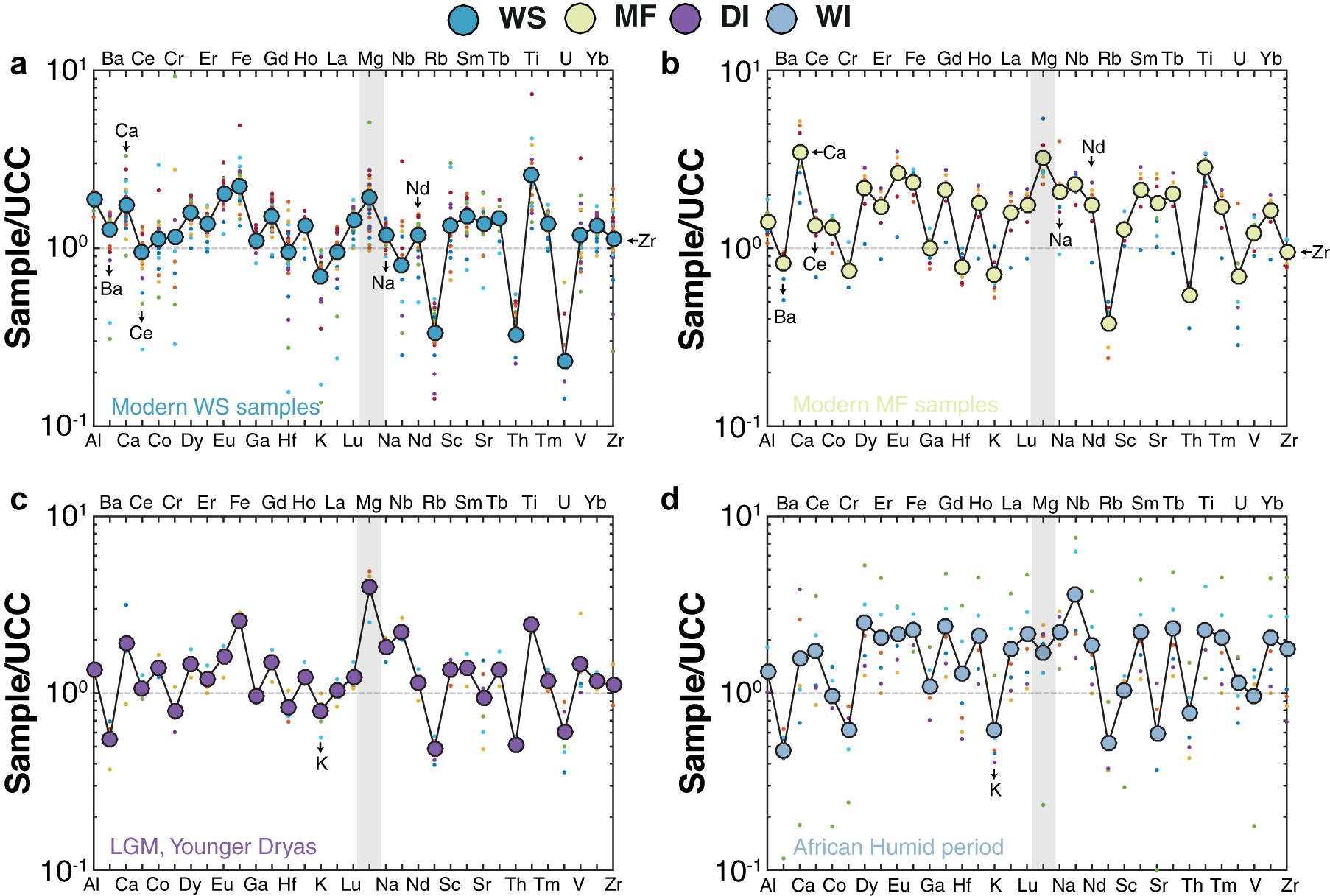
Figure 7. Upper Continental Crust (UCC)-normalized (McLennan, 2001) chemical composition in (a) WS, (b) MF, (c) DI, and (d) WI samples. Colored dots show the individual averages for each sample group. WS, modern watershed; MF, modern mudflat; MF, modern mudflat; DI, late Quaternary dry interval; and WI, late Quaternary wet interval.
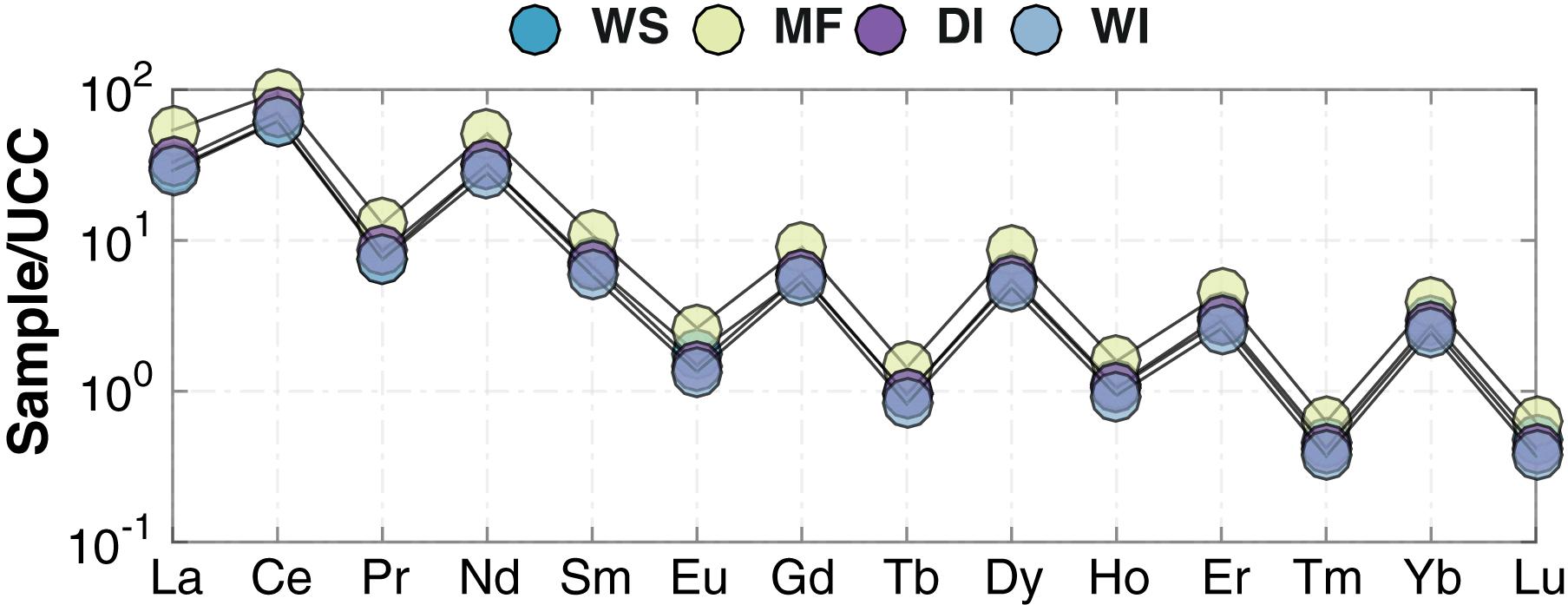
Figure 8. REE patterns normalized to chondrite (McDonough and Sun, 1995) in MF (yellow-green), DI (purple), WI (light blue), and WS (blue) samples. WS, modern watershed; MF, modern mudflat; DI, late Quaternary dry interval; and WI, late Quaternary wet interval.
Whole-rock Ba/Sr ratios, a widely used proxy for weathering intensity (e.g., Buggle et al., 2011), show WS and WI samples generally having higher Ba/Sr ratios compared to MF and DI samples (Figure 9a). On average, MF samples have higher MgO/Al2O3 ratios (mean = 0.3) than WS samples (mean = 0.15), but both DI and WI samples have higher MgO/Al2O3 ratios compared to MF samples (Figures 9a,b). MgO/Al2O3 and Ba/Sr ratios in WS and MF samples show a somewhat higher degree of correlation (R2 = 0.64) than in WI and DI samples (R2 = 0.52; Figure 9a). MgO/Al2O3 and K2O/Al2O3 ratios in DI and WI samples similarly show a high degree of correlation (R2 = 0.64; Figure 10a), and this relationship is the same for MgO and K2O.
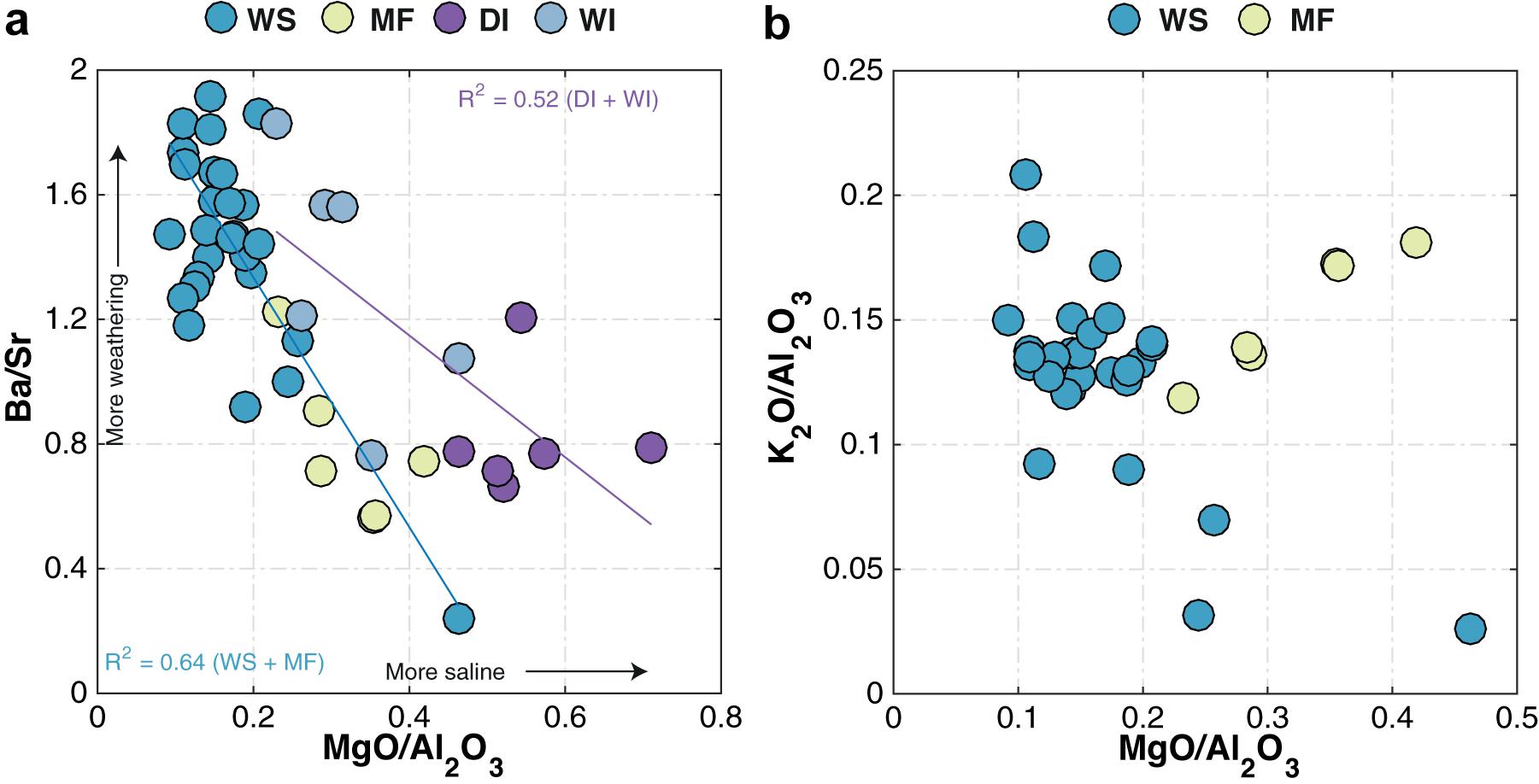
Figure 9. Comparison between (a) Ba/Sr and MgO/Al2O3 for WS, MF, DI, and WI samples and (b) K2O/Al2O3 and MgO/Al2O3 for WS and MF samples. WS, modern watershed; MF, modern mudflat; DI, late Quaternary dry interval; and WI, late Quaternary wet interval. The R2 values shown in the lower left corner of (b) are for Ba/Sr versus MgO/Al2O3 in WS and MF (blue), and WI and DI (purple) samples, respectively. Arrows in (b) show direction of increase in weathering intensity and salinity inferred from the Ba/Sr and MgO/Al2O3 ratios, respectively.
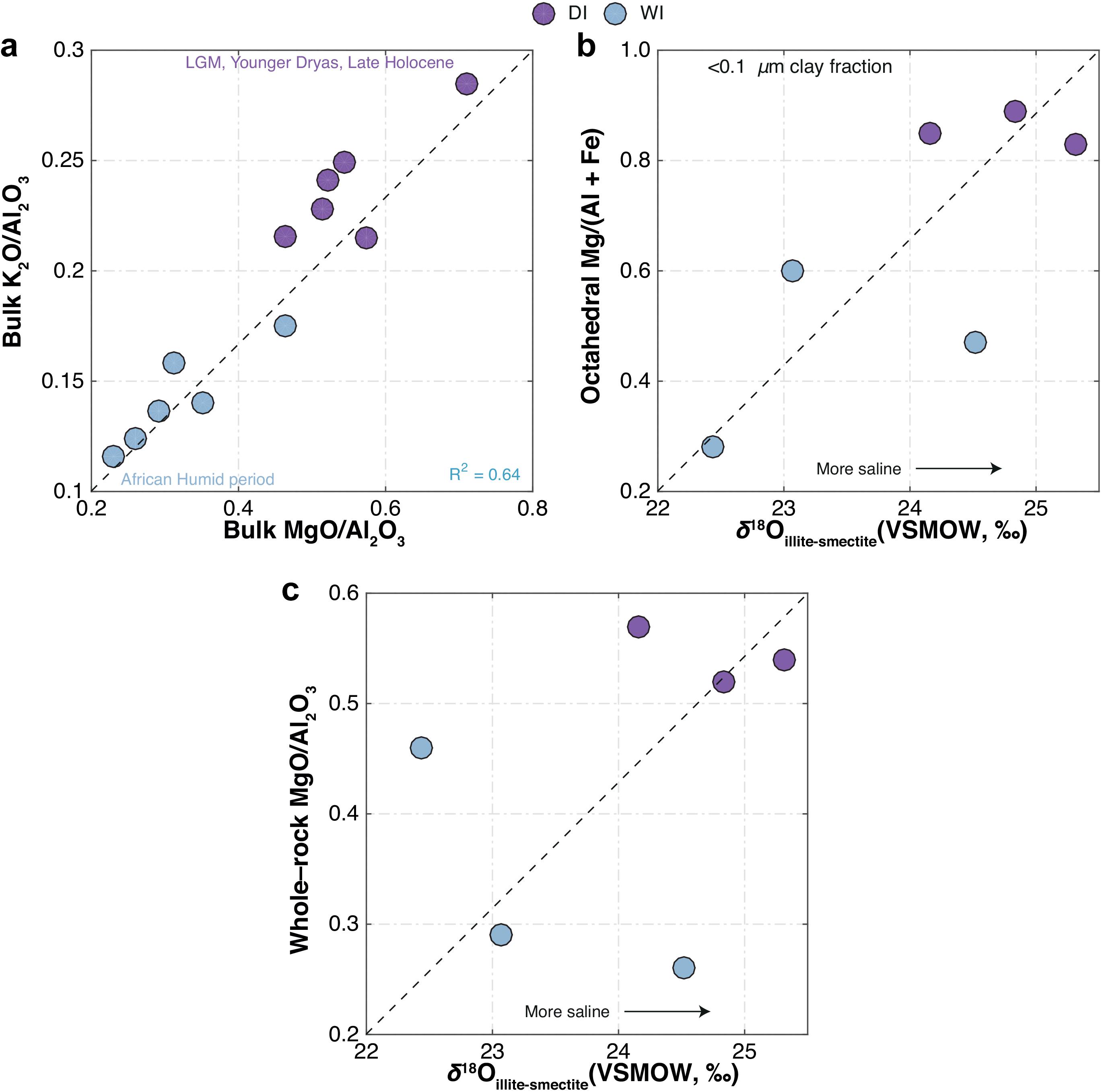
Figure 10. Comparison for DI and WI samples between (a) bulk K2O/Al2O3 and MgO/Al2O3, (b) octahedral Mg/(Al + Fe) versus δ18O of authigenic illite-smectite (<0.1 μm size-fraction), and (c) bulk MgO/Al2O3 versus δ18O of authigenic illite-smectite (<0.1 μm size-fraction). Arrows in (b,c) show direction of increase in salinity deduced from δ18Oillite–smectite.
Oxygen-isotope measurements of authigenic clay minerals (δ18Oillite–smectite = + 22.44 to + 25.32‰) show that, except for one sample, the DI samples have higher δ18Oillite–smectite compared to WI samples (Figures 10b,c and Table 3). The oxygen-isotope results for the <0.1 μm size-fraction also correlate well with the octahedral Mg/(Al + Fe) ratios determined by electron microprobe analysis (Figure 10b and Table 3).
Geochemical Modeling
Model results (Figure 11) show the immediate precipitation of calcite, reflecting the near-saturation of most surface waters in the region with respect to calcium carbonate. Evaporative concentration increases the amount of calcite precipitation and is also associated with analcime formation (Foerster et al., 2018). The next phase to reach supersaturation in the system is analcime (NaAlSi2O6∙H2O). Although solid phase analcime is stable in these surface waters, only a small mass of mineral was predicted to precipitate. The final phase to reach supersaturation is sepiolite [Mg4(Si6O15)(OH)2∙6H2O].
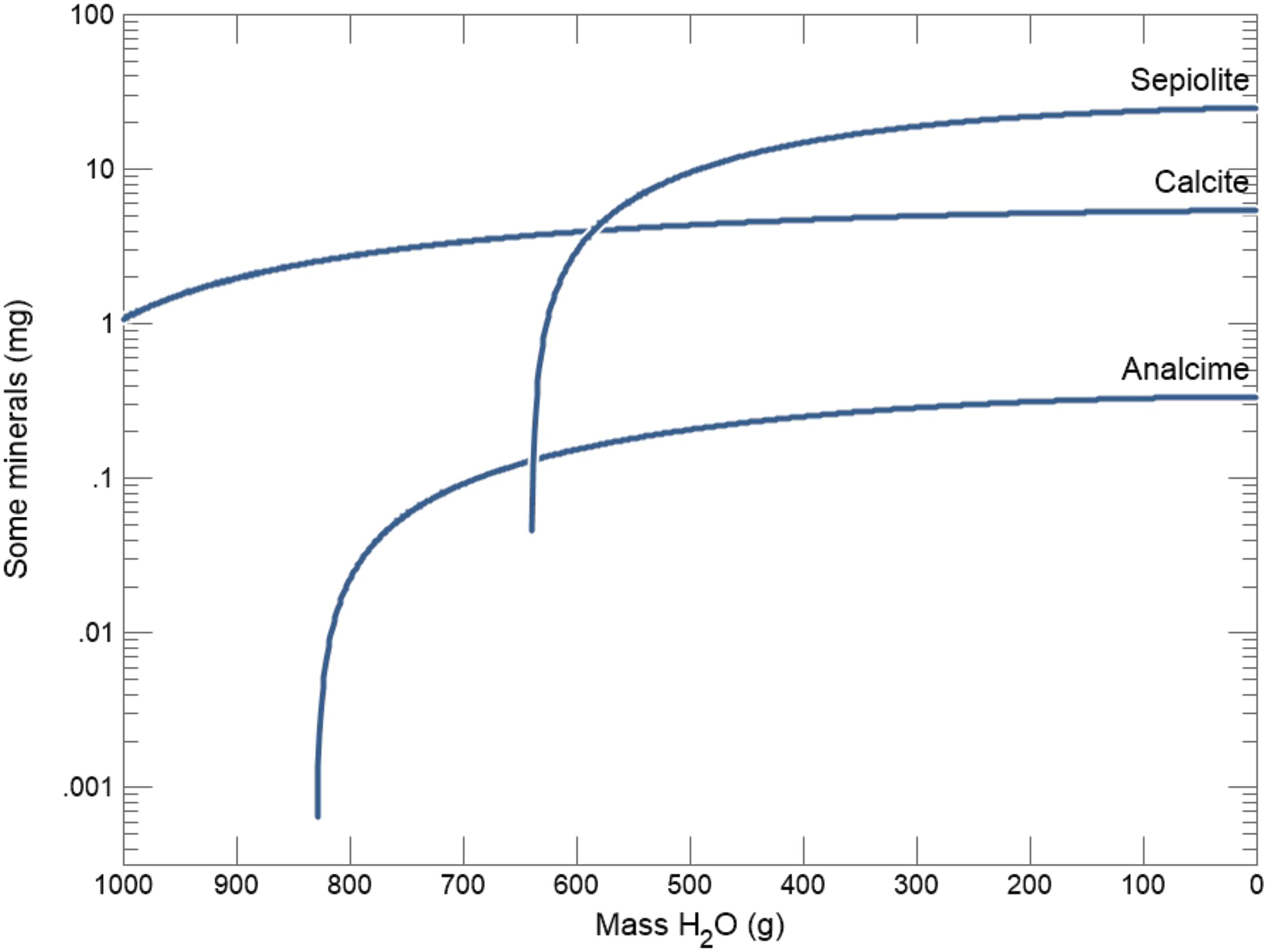
Figure 11. Modeled hydrochemical evolution and mineral reaction pathways in the Chew Bahir basin showing the immediate precipitation of calcite, and followed by analcime and sepiolite, calculated based on Lake Chamo dissolved solid composition (Table 2; Ayenew, 2005).
Discussion
Weathering, Early Diagenesis and Authigenesis in Chew Bahir Sediments
The sedimentary deposits comprised in the Chew Bahir cores are primarily derived from Precambrian metamorphic rocks of the Hammar Range and the volcanic rocks at the northern and northeastern parts of the catchment via erosion and physical weathering of the source rock, which also facilitates chemical weathering. The CIA, first introduced by Nesbitt and Young (1982), has proved useful for quantifying progressive chemical weathering of the source rock, with higher values indicating stronger weathering. In the case of WS samples, both physical weathering of the source rock material (i.e., metamorphosed granitic and gneissic rocks) and progressive chemical weathering are likely at play. The presence of authigenic carbonates, abundant in MF samples, however, complicates application of the CIA when endeavoring to capture the true extent of weathering (e.g., Cullers, 2000; Buggle et al., 2011; Garzanti et al., 2014). The Fe2O3, MnO, and K2O contents of both WS and MF samples are about the same but the CaO content is generally higher in MF samples (Figure 5). Solving Equation 1 excluding CaO provides higher CIA values for WS samples (i.e., mean CIA = 74) than MF samples (i.e., mean CIA = 60), indicating stronger weathering in WS samples than MF samples. Although the unavailability of a systematic dust record is a key limitation, the conclusion that sedimentary deposits comprised in the Chew Bahir cores are primarily derived from Precambrian metamorphic rocks of the Hammar Ranis is consistent with the inferences made from XRD and grain size data (Supplementary Figures 1, 2). The XRD data do not show evidence for windblown or distant aeolian-transported dust sources, which are commonly associated with clay minerals such as palygorskite (see, for example, Ehrmann et al., 2017 and Supplementary Figure 1). Two of the three samples (i.e., W26 and W4) collected for grain size analysis derived from freshly transported material of recent riverbeds show unimodal features and very little material in the 2–50 μm aeolian size range (Supplementary Figure 2). W25 collected from an alluvial fan accumulation is a mixture of different grain-size fractions with multimodal features, suggesting strong local fluvial reworking (Supplementary Figure 2). The amount of material in the 2–50 μm size range is also much higher in W25 (∼36. 8%) than in W26 and W4 (∼14.4%), and lends further support for the aforementioned interpretations vis-à-vis the source of Chew Bahir sediments. We concede, however, that the above interpretations need to be independently verified using dust traps.
As described in the Results section, notable differences in major and minor whole-rock geochemistry exist between MF and WS samples (Figure 5 and Table 1). Differences also include significant enrichment of MF samples compared to WS samples in some mobile elements (e.g., Na and Mg), which may have been introduced externally from saline, alkaline pore waters (Figures 7a,b). Na contents in WI and DI samples, however, are not significantly different (Figure 7c,d). The decreasing trend in Al2O3, Fe2O3, MnO, and MgO contents with increasing SiO2 in the WS samples reflects the process involved in the formation of the source granitic rocks by a simple fractional crystallization (e.g., Asrat and Barbey, 2003). However, the MF samples also show an increase in MgO, which indicates its possible enrichement during chemical weathering (e.g., clay formation; Figure 5).
The true extent of early diagenetic control on the elemental composition of Chew Bahir sediments is amplified in their clay mineralogy. The modern MF samples mostly contain illite, Mg-rich smectite and some kaolinite (Figure 4). The Mg enrichment observed in clay minerals from MF samples is similar to late Quaternary DI samples and most likely reflects authigenic clay mineral formation from the alteration of feldspathic glass (Deocampo and Jones, 2014; Deocampo, 2015), a precursor phase present in abundant quantities in the Chew Bahir basin (Foerster et al., 2018).
Watershed samples also show a slightly higher degree of LREE and HREE fractionation compared to MF samples, while all sample groups also have negative Eu anomalies with no clear differences between WS and MF samples (average Eu/Eu∗ of 0.85 versus 0.78, respectively; Table 1) or WI and DI samples (average Eu/Eu∗ of 0.71 versus 0.69, respectively; Table 1). Assuming Eu concentration is not affected by weathering, a negative Eu anomaly is an indication of strong plagioclase fractionation in the source rocks; notably, the Proterozoic granitic and gneissic rocks of the Hammar Range show strong negative Eu anomalies (Asrat and Barbey, 2003). It also suggests that the bulk sediment source rock remained very similar through time. In short, the REE data suggest that the detrital sediment load is predominantly derived from the Hammar Range.
Consistent with the CIA, intergroup comparisons between MgO/Al2O3 and K2O/Al2O3 or Ba/Sr for the bulk sediments suggest that the intensity of weathering, inferred from Ba/Sr data, is (and was) highest in late Quaternary WI and modern WS samples (Figure 9a). Although the link between weathering and geochemical proxy formation is known to be more complex, Ba/Sr ratios are routinely used as a weathering proxy. The power of this proxy is provided by the selective removal of more mobile Sr coupled with the relative immobility of Ba, which is readily adsorbed by clay minerals during weathering (e.g., Nesbitt and Markovics, 1980; Middelburg et al., 1988; Deocampo et al., 2010). By comparison, DI samples have consistently higher MgO/Al2O3, while K2O/Al2O3 in MF samples holds constant (Figure 10b). A strong positive relationship between MgO/Al2O3 and K2O/Al2O3 (also between MgO and K2O) within the mudflat samples indicates the effect of greater exposure to salinity and alkalinity on the mudflats, with octahedral Mg substitution and K-uptake due to incipient illitization (Deocampo, 2015; Figure 9b). The presence of both smectite and illite-smectite (the latter formed by low temperature illitization of smectite; e.g., Eberl et al., 1986) is reminiscent of similar differences observed between the late Quaternary WI and DI samples. The geochemistry of MF samples is most likely constrained by diagenesis and authigenic mineral formation, rather than solely representing the end product of weathering. Indeed, neoformation of clay minerals in alkaline solutions is termed “inverse weathering” as it is chemically the opposite of silicate hydrolysis, which produces authigenic alkaline silicate minerals (Deocampo and Jones, 2014; Deocampo, 2015).
The presence of analcime, a precursor zeolite formed under conditions of increasing lake water salinity and alkalinity, is noteworthy in all MF samples and WS samples collected near the desiccated lake floor (Figure 4). Analcime is also present in cores collected from the center of the Chew Bahir basin (e.g., Viehberg et al., 2018). Analcime can form under diverse physical, chemical and geological settings including marine and hydrothermal alteration, but it is much more common in saline and alkaline lake deposits (e.g., Hay and Sheppard, 2001). We have considered a range of possibilities to explain diagenetic formation of analcime in the Chew Bahir basin. In some other eastern African lakes such as Lake Magadi, analcime is thought to form by reaction of precursor zeolites, volcanic glass, hydrous sodium aluminosilicate gels or a combination of all three phases in saline alkaline solutions (e.g., Surdam and Eugster, 1976). In other eastern African rift lakes such as Lake Bogoria, authigenic analcime has been shown to form from poorly crystalline smectite clay minerals reacting with saline, alkaline pore fluids (e.g., Renaut, 1993).
Hydrogeochemical Processes in the Chew Bahir Basin
The Geochemist’s Workbench§ model results indicate that evaporative concentration increases the amount of calcite precipitation, eventually eliminating calcium from evolved waters (Deocampo and Jones, 2014). The next phase to reach supersaturation in the model system is analcime (NaAlSi2O6∙H2O; Figure 11). This reflects the high alkalinity of the waters in the presence of reactive aluminosilicates (Hay, 1978). Although analcime is stable in these modeled waters, however, only a small mass of mineral was predicted to precipitate. This suggests that the significant accumulations of analcime observed in the Chew Bahir sediments (e.g., Foerster et al., 2018) result not only from evaporative concentration, but also from alteration of locally abundant volcanic glass in alkaline waters (Hay and Sheppard, 2001).
Sepiolite [Mg4(Si6O15)(OH)2∙6H2O] is the final phase to reach supersaturation in the modeled system (Figure 11). Although sepiolite has not been observed locally and is not expected to precipitate in this setting, we can use its thermodynamics to model the behavior of Mg-silicates more generally (Deocampo, 2005, 2015). Abundant aluminous detrital clay minerals in fluvio-lacustrine environments provide reactive substrates with low kinetic barriers for precipitation of Mg-rich interstratifications rather than pure Mg-silicate such as sepiolite (Deocampo, 2015). The model prediction of sepiolite precipitation shows the potential for Mg-rich authigenic clay minerals to form, which is well known as an indicator of elevated salinity in lake deposits in the region (Deocampo et al., 2017; Foerster et al., 2018).
In sum, thermodynamic modeling of evaporating Lake Chamo water produces an authigenic mineral assemblage typical of both modern lake mudflats in the Chew Bahir basin and Late Quaternary sediments recovered from cores (Foerster et al., 2018). These data suggest that the mineral assemblage of calcite, analcime, and Mg-enriched authigenic illitic clay minerals is indicative of environments of enhanced evaporative concentration in the basin.
Refining Interpretation of the Chew Bahir K Record Using Whole-Rock and Clay Mineral Geochemistry
Foerster et al. (2018) demonstrated that K content in the Chew Bahir sediments is likely derived from enhanced K-fixation during dry high-salinity episodes. Salinity in the hydrologically closed paleo-Lake Chew Bahir was controlled by the amount of paleo-precipitation in the catchment and the rate of evaporation in the paleolake. It is therefore conceivable that the chemical composition of the paleolake significantly impacted the crystal chemistry of authigenic minerals present in the sediment, including the progress of K-fixation in clay minerals (Foerster et al., 2018). Like many other eastern African rift lakes, aridity and the prevalence of hypersaline conditions facilitate authigenic illitization of smectite (e.g., Singer and Stoffers, 1980; Deocampo et al., 2009) and consequently K-enrichment in Chew Bahir sediments. The major element geochemistry of the late Quaternary DI and WI samples establishes a clear relationship between K-enrichment in Chew Bahir sediments and hypersaline conditions in the basin (Figure 10a). It should also be noted that sediments deposited during humid episodes (e.g., during the AHP) had whole-rock MgO/Al2O3 ratios ranging from ∼0.2 to 0.4, whereas those deposited during arid episodes (e.g., at LGM and YD times) had MgO/Al2O3 ratios ranging from ∼0.5 to 0.7, indicating higher salinity (Figure 10a). These results show that ancient sediments deposited during dry periods most similarly resemble modern saline mudflats in dry-Lake Chew Bahir today and had Ba/Sr values ranging from ∼0.6 to 1.2 (Figure 9a). Conversely, sediments deposited during relatively wetter periods, such as the AHP, have sources similar to those found presently in upstream locations, e.g., WS samples.
The proposed relationship between basin aridity and K uptake in the sediment is compared with oxygen - isotope analysis of authigenic illite-smectite. The oxygen−isotope composition of authigenic clay minerals formed in lake water at isotopic equilibrium is controlled primarily by clay mineral composition, structure, and water temperature (Savin and Lee, 1988). Once formed, oxygen in the tetrahedral and octahedral sheets does not undergo isotopic exchange with pore fluids in the absence of dissolution− precipitation reactions. In the current study, the δ18Oillite–smectite has been determined by the oxygen-isotope composition of the incoming waters draining through the Weyto River (Figures 1b,c) and the extent of lake-water evaporation (e.g., Gebregiorgis et al., 2020a). Lake-water δ18O is certainly influenced by the isotopic composition of the prevailing source (i.e., Lake Chamo; see Figure 1b) during periods of rapid lake level change. This signal, however, is likely overprinted in a system like Chew Bahir, where the extent of lake-water 18O-enrichment was largely driven by evaporation.
The δ18O of authigenic illite-smectite in the late Quaternary WI and DI samples range from +22.4 to +24.5‰ (average + 23.3‰), and +24.1 to +25.3‰ (average + 24.77‰), respectively (Figures 10b,c). Notably wet (i.e., during the AHP) and dry (e.g., at LGM and YD times) periods of the late Quaternary have been identified from the Chew Bahir K record, which are now largely confirmed independently by these oxygen-isotope measurements. The authigenic illite-smectite is generally most enriched in 18O during periods of regional aridity, which also coincides with significant Mg enrichment (Figure 10b and Table 3) and low-temperature illitization suggestive of enhanced evaporative concentration in the basin (Figure 11 and Table 4). Collectively, our results confirm that, in closed-basin lake deposits not overly diluted by detrital or biogenic sediments, subtle changes in water balance can be detected using both elemental and oxygen-isotope analysis of submicron authigenic clay minerals. Our first attempt to reproduce the climate signals recorded in the Chew Bahir K record using oxygen-isotope measurements of authigenic illite-smectite from notable warm/wet (e.g., during the Mid-Holocene) and cold/dry intervals (e.g., during the LGM) has proven to be successful.
Conclusion
New mineralogical and geochemical data from modern and ancient sediments in the Chew Bahir basin shed light on the role of sedimentary processes in chemical proxy formation. The data presented here show that the geochemistry of the modern saline mudflats in dry Lake Chew Bahir today resemble ancient sediments deposited during dry periods. Conversely, the geochemistry of modern detrital upstream sources can be compared to sediments deposited during relatively wetter periods, such as the AHP. This study demonstrates that interpretation of such geochemical datasets is complex and highly site-specific, and careful evaluation of any seemingly straightforward relationship between sediment composition and climate is required. This knowledge is critical for understanding proxy formation in playa sites and sedimentary records from semi-arid regions of the world.
Data Availability Statement
All data generated or analyzed during this study are included in this published article.
Author Contributions
DG, DD, VF, and AA designed the project. DD conducted the geochemical modeling. FL provided and acquired funding for the oxygen-isotope analyses. AA, FS, HL, and MT acquired the funding, designed, and directed the Chew Bahir Drilling Project as part of the Hominin Sites and Paleolakes Drilling Project, directed by A. Cohen of the University of Arizona. FS, AA, HL, and VF did the coring at Chew Bahir. JD carried out electron probe microanalyses of samples. VF, FS, AJ, MM, and HL added modern samples to this study. SO carried out grain size analysis of samples. DG led sample collection, data analysis, and wrote the manuscript with contributions from all co-authors. All authors revised the manuscript.
Funding
This research was supported by NSF grant (NSF 1349651) to DD. The oxygen-isotope measurements were supported by a Natural Sciences and Engineering Research Council Discovery Grant of Canada and the Canada Research Chairs Program (FL). FS acknowledges further funding from the DFG through grants SCHA 472/13 and SCHA 472/18. MT acknowledges funding from the DFG through grants TR 419/8, TR 419/9, and TR 419/16. In addition, FS acknowledges funding from the CRC 806 Research Project “Our way to Europe” – Project Number 57444011. HL was funded by grant NE/K014560/1 from the UK Natural Environment Research Council. This was Western’s Laboratory for Stable Isotope Science Contribution #385.
Conflict of Interest
The authors declare that the research was conducted in the absence of any commercial or financial relationships that could be construed as a potential conflict of interest.
Supplementary Material
The Supplementary Material for this article can be found online at: https://www.frontiersin.org/articles/10.3389/feart.2021.607695/full#supplementary-material
References
Asrat, A., and Barbey, P. (2003). Petrology, geochronology and Sr–Nd isotopic geochemistry of the konso pluton, south-western ethiopia: implications for transition from convergence to extension in the mozambique belt. Int. J. Earth Sci. 92, 873–890. doi: 10.1007/s00531-003-0360-9
Ayenew, T. (2005). Major ions composition of the groundwater and surface water systems and their geological and geochemical controls in the ethiopian volcanic terrain. SINET Ethiopian J. Sci. 28, 171–188.
Bethke, C. M., Farrell, B., and Yeakel, S. (2019). GWB essentials guide. Champaign, Illinois: Aqueous Solutions, LLC.
Blott, S. J., and Pye, K. (2001). GRADISTAT: a grain size distribution and statistics package for the analysis of unconsolidated sediments. Earth Surface Processes Landforms 26, 1237–1248. doi: 10.1002/esp.261
Brown, E., Johnson, T., Scholz, C. A., Cohen, A., and King, J. (2007). Abrupt change in tropical african climate linked to the bipolar seesaw over the past 55,000 years. Geophys. Res. Lett. 34:20702. doi: 10.1029/2007GL031240
Buggle, B., Glaser, B., Hambach, U., Gerasimenko, N., and Marković, S. (2011). An evaluation of geochemical weathering indices in loess–paleosol studies. Quaternary Int. 240, 12–21. doi: 10.1016/j.quaint.2010.07.019
Burnett, A. P., Soreghan, M. J., Scholz, C. A., and Brown, E. T. (2011). Tropical east african climate change and its relation to global climate: a record from lake tanganyika, tropical east africa, over the past 90+ kyr. Palaeogeogr. Palaeoclimatol. Palaeoecol. 303, 155–167. doi: 10.1016/j.palaeo.2010.02.011
Chesworth, W., Dejou, J., and Larroque, P. (1981). The weathering of basalt and relative mobilities of the major elements at belbex, france. Geochimica et Cosmochimica Acta 45, 1235–1243. doi: 10.1016/0016-7037(81)90147-2
Clark, P. U., Dyke, A. S., Shakun, J. D., Carlson, A. E., Clark, J., Wohlfarth, B., et al. (2009). The last glacial maximum. Science 325, 710–714.
Clift, P. D., Wan, S., and Blusztajn, J. (2014). Reconstructing chemical weathering, physical erosion and monsoon intensity since 25 ma in the northern south china sea: a review of competing proxies. Earth Sci. Rev. 130, 86–102. doi: 10.1016/j.earscirev.2014.01.002
Croudace, I. W., Löwemark, L., Tjallingii, R., and Zolitschka, B. (2019). Current perspectives on the capabilities of high resolution XRF core scanners. Quaternary Int. 514, 5–15. doi: 10.1016/j.quaint.2019.04.002
Croudace, I. W., Rindby, A., and Rothwell, R. G. (2006). “ITRAX: description and evaluation of a new multi-function X-ray core scanner,” in New techniques in sediment core analysis, ed. R. G. Rothwell (London, UK: Geological Society of London), 51–63.
Cullers, R. L. (2000). The geochemistry of shales, siltstones and sandstones of pennsylvanian–permian age, colorado, USA: implications for provenance and metamorphic studies. Lithos 51, 181–203. doi: 10.1016/S0024-4937(99)00063-8
Davidson, A. (1983). The omo river project: reconnaissance geology and geochemistry of parts of Ilubabor, kefa, gemu gofa and sidamo. Ethiopian Instit. Geol. Surv. Bull. 2, 1–89.
Demenocal, P., Ortiz, J., Guilderson, T., Adkins, J., Sarnthein, M., Baker, L., et al. (2000). Abrupt onset and termination of the african humid period: rapid climate responses to gradual insolation forcing. Quaternary Sci. Rev. 19, 347–361. doi: 10.1016/S0277-3791(99)00081-5
Deocampo, D., Cuadros, J., Wing-Dudek, T., Olives, J., and Amouric, M. (2009). Saline lake diagenesis as revealed by coupled mineralogy and geochemistry of multiple ultrafine clay phases: pliocene olduvai gorge, tanzania. Am. J. Sci. 309, 834–868. doi: 10.2475/09.2009.03
Deocampo, D. M. (2004). Authigenic clays in east africa: regional trends and paleolimnology at the plio–pleistocene boundary, olduvai gorge, tanzania. J. Paleolimnol. 31, 1–9. doi: 10.1023/B:JOPL.0000013353.86120.9b
Deocampo, D. M. (2005). Evaporative evolution of surface waters and the role of aqueous CO2 in magnesium silicate precipitation: lake eyasi and ngorongoro crater, northern tanzania. South Afric. J. Geol. 108, 493–504. doi: 10.2113/108.4.493
Deocampo, D. M. (2010). The geochemistry of continental carbonates. Dev. Sedimentol. 62, 1–59. doi: 10.1016/S0070-4571(09)06201-3
Deocampo, D. M. (2015). Authigenic clay minerals in lacustrine mudstones. Geol. Soc. Am. Spl. Papers 515, SE503–SE515.
Deocampo, D. M., Behrensmeyer, A. K., and Potts, R. (2010). Ultrafine clay minerals of the pleistocene olorgesailie formation, southern kenya rift: diagenesis and paleoenvironments of early hominins. Clays Clay Minerals 58, 294–310. doi: 10.1346/CCMN.2010.0580301
Deocampo, D. M., Berry, P. A., Beverly, E. J., Ashley, G. M., and Jarrett, R. E. (2017). Whole-rock geochemistry tracks precessional control of pleistocene lake salinity at olduvai gorge, tanzania: a record of authigenic clays. Geology 45, 683–686. doi: 10.1130/G38950.1
Deocampo, D. M., and Jones, B. F. (2014). “Geochemistry of saline lakes,” in Treatise on Geochemistry, Second Edn, eds H. D. Holland and K. K. Turekian (Amsterdam: Elsevier), 437–469.
Deocampo, D. M., and Tactikos, J. C. (2010). Geochemical gradients and artifact mass densities on the lowermost Bed II eastern lake margin (~ 1.8 Ma), olduvai gorge, tanzania. Quaternary Res. 74, 411–423. doi: 10.1016/j.yqres.2010.09.004
Eberl, D. D., Środoñ, J., and Northrop, H. R. (1986). “Potassium fixation in smectite by wetting and drying,” in Geochemical Processes at Mineral Surfaces, eds J. A. Davies and K. F. Hayes (Washington, DC: American Chemical Society Symposium), 296–326.
Ebinger, C., Yemane, T., Harding, D., Tesfaye, S., Kelley, S., and Rex, D. (2000). Rift deflection, migration, and propagation: linkage of the ethiopian and eastern rifts, africa. Geol. Soc. Am. Bull. 112, 163–176. doi: 10.1130/0016-76062000112<163:RDMAPL<2.0.CO;2
Ehrmann, W., Schmiedl, G., Beuscher, S., and Krüger, S. (2017). Intensity of african humid periods estimated from saharan dust fluxes. PloS one 12:e0170989. doi: 10.1371/journal.pone.0170989
Fischer, M. L., Markowska, M., Bachofer, F., Foerster, V. E., Asrat, A., Zielhofer, C., et al. (2020). Determining the pace and magnitude of lake level changes in southern ethiopia over the last 20,000 years using lake balance modelling and SEBAL. Front. Earth Sci. 8:197. doi: 10.3389/feart.2020.00197
Foerster, V., Deocampo, D. M., Asrat, A., Günter, C., Junginger, A., Krämer, K. H., et al. (2018). Towards an understanding of climate proxy formation in the chew bahir basin, southern ethiopian rift. Palaeogeogr. Palaeoclimatol. Palaeoecol. 501, 111–123. doi: 10.1016/j.palaeo.2018.04.009
Foerster, V., Junginger, A., Langkamp, O., Gebru, T., Asrat, A., Umer, M., et al. (2012). Climatic change recorded in the sediments of the chew bahir basin, southern ethiopia, during the last 45,000 years. Quaternary Int. 274, 25–37. doi: 10.1016/j.quaint.2012.06.028
Folk, R. L., and Ward, W. C. (1957). Brazos river bar: a study in the significance of grain size parameters. J. Sediment. Petrol. 27, 3–26. doi: 10.1306/74D70646-2B21-11D7-8648000102C1865D
Garzanti, E., Padoan, M., Setti, M., López-Galindo, A., and Villa, I. M. (2014). Provenance versus weathering control on the composition of tropical river mud (southern africa). Chem. Geol. 366, 61–74. doi: 10.1016/j.chemgeo.2013.12.016
Gebregiorgis, D., Deocampo, D. M., Longstaffe, F. J., Simpson, A., Ashley, G. M., Beverly, E. J., et al. (2020a). Oxygen isotopes in authigenic clay minerals: Toward building a reliable salinity proxy. Geophys. Res. Lett. 47:e2019GL085576. doi: 10.1029/2019GL085576
Gebregiorgis, D., Giosan, L., Hathorne, E. C., Anand, P., Nilsson-Kerr, K., Plass, A., et al. (2020b). What can we learn from X-ray fluorescence core scanning data? A paleomonsoon case study. Geochem. Geophys. Geosyst. 21:e2019GC008414. doi: 10.1029/2019GC008414
Gebregiorgis, D., Rayner, D., and Linderholm, H. W. (2019). Does the IOD independently influence seasonal monsoon patterns in northern ethiopia? Atmosphere 10:432. doi: 10.3390/atmos10080432
Harriss, R. C., and Adams, J. A. (1966). Geochemical and mineralogical studies on the weathering of granitic rocks. Am. J. Sci. 264, 146–173. doi: 10.2475/ajs.264.2.146
Hay, R. L. (1978). “Geologic occurrence of zeolites,” in Natural zeolites: Occurrence, properties, use, eds L. B. Sand and F. A. Mumpton (Elmsford, NY: Pergamon Press), 135–143.
Hay, R. L., and Sheppard, R. A. (2001). Occurrence of zeolites in sedimentary rocks: an overview. Rev. Mineral. Geochem. 45, 217–234. doi: 10.2138/rmg.2001.45.6
Hendy, I. L., Napier, T. J., and Schimmelmann, A. (2015). From extreme rainfall to drought: 250 years of annually resolved sediment deposition in santa barbara basin, california. Quaternary Int. 387, 3–12. doi: 10.1016/j.quaint.2015.01.026
Hession, S. L., and Moore, N. (2011). A spatial regression analysis of the influence of topography on monthly rainfall in east africa. Int. J. Climatol. 31, 1440–1456. doi: 10.1002/joc.2174
Huggett, J., Adetunji, J., Longstaffe, F., and Wray, D. (2017). Mineralogical and geochemical characterisation of warm-water, shallow-marine glaucony from the tertiary of the london basin. Clays Clay Minerals 52, 25–50. doi: 10.1180/claymin.2017.052.1.02
Junginger, A., and Trauth, M. H. (2013). Hydrological constraints of paleo-lake suguta in the northern kenya rift during the african humid period (15–5 ka BP). Global Planetary Change 111, 174–188. doi: 10.1016/j.gloplacha.2013.09.005
Libbey, R. B., Longstaffe, F. J., and Flemming, R. L. (2013). Clay mineralogy, oxygen isotope geochemistry, and water/rock ratio estimates, Te Mihi area, Wairakei geothermal field, new zealand. Clays Clay Minerals 61, 204–217. doi: 10.1346/CCMN.2013.0610304
Lyons, R. P., Scholz, C. A., Cohen, A. S., King, J. W., Brown, E. T., Ivory, S. J., et al. (2015). Continuous 1.3-million-year record of east african hydroclimate, and implications for patterns of evolution and biodiversity. Proc. Natl. Acad. Sci. 112, 15568–15573. doi: 10.1073/pnas.1512864112
McDonough, W. F., and Sun, S. S. (1995). The composition of the earth. Chem. Geol. 120, 223–253. doi: 10.1016/0009-2541(94)00140-4
McLennan, S. M. (2001). Relationships between the trace element composition of sedimentary rocks and upper continental crust. Geochem. Geophys. Geosyst. 2:1021. doi: 10.1029/2000GC000109
Middelburg, J. J., van der Weijden, C. H., and Woittiez, J. R. (1988). Chemical processes affecting the mobility of major, minor and trace elements during weathering of granitic rocks. Chem. Geol. 68, 253–273. doi: 10.1016/0009-2541(88)90025-3
Moore, D., and Reynolds, R. (1997). X-ray Diffraction and the Identification and Analysis of Clay Minerals, Second Edn. Oxford, England: Oxford University Press.
Nesbitt, H., and Young, G. (1982). Early Proterozoic climates and plate motions inferred from major element chemistry of lutites. Nature 299, 715–717. doi: 10.1038/299715a0
Nesbitt, H. W. (1979). Mobility and fractionation of rare earth elements during weathering of a granodiorite. Nature 279, 206–210. doi: 10.1038/279206a0
Nesbitt, H. W., and Markovics, G. (1980). Chemical processes affecting alkalis and alkaline earths during continental weathering. Geochim. et Cosmochim. Acta 44, 1659–1666. doi: 10.1016/0016-7037(80)90218-5
Nicholson, S. (2016). The turkana low-level jet: mean climatology and association with regional aridity. Int. J. Climatol. 36, 2598–2614. doi: 10.1002/joc.4515
Nicholson, S. E. (2018). The ITCZ and the seasonal cycle over equatorial africa. Bull. Am. Meteorol. Soc. 99, 337–348. doi: 10.1175/BAMS-D-16-0287.1
Otto-Bliesner, B. L., Russell, J. M., Clark, P. U., Liu, Z., Overpeck, J. T., Konecky, B., et al. (2014). Coherent changes of southeastern equatorial and northern african rainfall during the last deglaciation. Science 346, 1223–1227. doi: 10.1126/science.1259531
Renaut, R. (1993). Zeolitic diagenesis of late quaternary fluviolacustrine sediments and associated calcrete formation in the lake bogoria basin, kenya rift valley. Sedimentology 40, 271–301. doi: 10.1111/j.1365-3091.1993.tb01764.x
Roberts, N., Taieb, M., Barker, P., Damnati, B., Icole, M., and Williamson, D. (1993). Timing of the younger dryas event in east africa from lake-level changes. Nature 366, 146–148. doi: 10.1038/366146a0
Savin, S., and Lee, M. (1988). Isotopic studies of phyllosilicates. Rev. Mineral. Geochem. 19, 189–223.
Shanahan, T. M., McKay, N. P., Hughen, K. A., Overpeck, J. T., Otto-Bliesner, B., Heil, C. W., et al. (2015). The time-transgressive termination of the african humid period. Nat. Geosci. 8, 140–144. doi: 10.1038/ngeo2329
Singer, A., and Stoffers, P. (1980). Clay mineral diagenesis in two east african lake sediments. Clays Clay Minerals 15, 291–307. doi: 10.1180/claymin.1980.015.3.09
Sun, L., Semazzi, F. H., Giorgi, F., and Ogallo, L. (1999). Application of the NCAR regional climate model to eastern africa: 1. Simulation of the short rains of 1988. J. Geophys. Res. Atmospheres 104, 6529–6548. doi: 10.1029/1998JD200051
Surdam, R. C., and Eugster, H. P. (1976). Mineral reactions in the sedimentary deposits of the lake magadi region, kenya. Geol. Soc. Am. Bull. 87, 1739–1752. doi: 10.1130/0016-7606197687<1739:MRITSD<2.0.CO;2
Tian, J., Xie, X., Ma, W., Jin, H., and Wang, P. (2011). X-ray fluorescence core scanning records of chemical weathering and monsoon evolution over the past 5 Myr in the southern south china sea. Paleoceanography 26
Tierney, J. E., and deMenocal, P. B. (2013). Abrupt shifts in horn of africa hydroclimate since the last glacial maximum. Science 342, 843–846. doi: 10.1126/science.1240411
Trauth, M. H., Deino, A., Bergner, A. G. N., and Strecker, M. R. (2003). East african climate change and orbital forcing during the last 175 kyr BP. Earth Planetary Sci. Lett. 206, 297–313. doi: 10.1016/S0012-821X(02)01105-6
Trauth, M. H., Deino, A., and Strecker, M. R. (2001). Response of the east african climate to orbital forcing during the last interglacial (130-117 kyr BP) and the early last glacial (117-60 kyr BP). Geology 29, 499–502. doi: 10.1130/0091-76132001029<0499:ROTEAC<2.0.CO;2
Keywords: oxygen isotopes in authegenic clay minerals, X-ray core scanning, paleoclimate proxy formation and interpretation, whole-rock and clay mineralogy, geochemical modeling, the Chew Bahir K record
Citation: Gebregiorgis D, Deocampo DM, Foerster V, Longstaffe FJ, Delaney JS, Schaebitz F, Junginger A, Markowska M, Opitz S, Trauth MH, Lamb HF and Asrat A (2021) Modern Sedimentation and Authigenic Mineral Formation in the Chew Bahir Basin, Southern Ethiopia: Implications for Interpretation of Late Quaternary Paleoclimate Records. Front. Earth Sci. 9:607695. doi: 10.3389/feart.2021.607695
Received: 17 September 2020; Accepted: 24 March 2021;
Published: 30 April 2021.
Edited by:
Gary E. Stinchcomb, Murray State University, United StatesReviewed by:
Steven L. Forman, Baylor University, United StatesDhananjay Anant Sant, Maharaja Sayajirao University of Baroda, India
Copyright © 2021 Gebregiorgis, Deocampo, Foerster, Longstaffe, Delaney, Schaebitz, Junginger, Markowska, Opitz, Trauth, Lamb and Asrat. This is an open-access article distributed under the terms of the Creative Commons Attribution License (CC BY). The use, distribution or reproduction in other forums is permitted, provided the original author(s) and the copyright owner(s) are credited and that the original publication in this journal is cited, in accordance with accepted academic practice. No use, distribution or reproduction is permitted which does not comply with these terms.
*Correspondence: Daniel Gebregiorgis, ZGdlYnJlZ2lvcmdpc0Bnc3UuZWR1