- 1College of Forest Resources and Environmental Sciences, Michigan Technological University, Houghton, MI, United States
- 2Northern Research Station, USDA Forest Service, Houghton, MI, United States
- 3College of Biological Science, University of Guelph, Guelph, ON, Canada
- 4Department of Biology, Ball State University, Muncie, IN, United States
- 5Institute of Arctic and Alpine Research, University of Colorado Boulder, Boulder, CO, United States
Globally important carbon (C) stores in boreal peatlands are vulnerable to altered hydrology through changes in precipitation and runoff patterns, groundwater inputs, and a changing cryosphere. These changes can affect the extent of boreal wetlands and their ability to sequester and transform C and other nutrients. Variation in precipitation patterns has also been increasing, with greater occurrences of both flooding and drought periods. Recent work has pointed to the increasing role of algal production in regulating C cycling during flooded periods in fen peatlands, but exactly how this affects the C sink-strength of these ecosystems is poorly understood. We evaluated temporal trends in algal biomass, ecosystem C uptake and respiration (using static and floating chamber techniques), and spectroscopic indicators of DOM quality (absorbance and fluorescence) in a boreal rich-fen peatland in which water table position had been experimentally manipulated for 13 years. Superimposed on the water table treatments were natural variations in hydrology, including periods of flooding, which allowed us to examine the legacy effects of flooding on C cycling dynamics. We had a particular focus on understanding the role of algae in regulating C cycling, as the relative contribution of algal production was observed to significantly increase with flooding. Ecosystem measures of gross primary production (GPP) increased with algal biomass, with higher algal biomass and GPP measured in the lowered water table treatment two years after persistent flooding. Prior to flooding the lowered treatment was the weakest C sink (as CO2), but this treatment became the strongest sink after flooding. The lower degree of humification (lower humification index, HIX) and yet lower bioavailability (higher spectral slope ratio, Sr) of DOM observed in the raised treatment prior to flooding persisted after two years of flooding. An index of free or bound proteins (tyrosine index, TI) increased with algal biomass across all plots during flooding, and was lowest in the raised treatment. As such, antecedent drainage conditions determined the sink-strength of this rich fen—which was also reflected in DOM characteristics. These findings indicate that monitoring flooding history and its effects on algal production could become important to estimates of C balance in northern wetlands.
Introduction
Northern peatlands are a globally important reservoir of carbon (C), representing a large magnitude of C vulnerable to loss in a changing climate (Goldstein et al., 2020). Rich fens are one of the most common boreal peatland types in western North America (Vitt et al., 2000), and open peatlands and mineral wetlands comprise about 85% of wetland area in Alaska (Kolka et al., 2018) — an area of land nearly twice that of all wetland area in the contiguous United States (Ford and Bedford, 1987). Moreover, the extent of fens in interior Alaska has been increasing owing to permafrost degradation and changing ground water and runoff patterns in recent decades (Jorgenson et al., 2001; Lara et al., 2016). Observed regional climate trends and modelling efforts also suggest increased precipitation with overall higher variation (anomalies) in total amounts in Alaska’s boreal region (Stewart et al., 2013; Euskirchen et al., 2016). These patterns suggest prolonged or more variable periods of inundation in the future, with yet uncertain consequences for the C storage capacity of Alaska’s vast fen ecosystem complexes.
The carbon balance of peatlands has shown varied responses to changes in the water table position occurring with periods of flooding or drought. While high water tables are generally associated with higher fluxes of methane (e.g., Turetsky et al., 2014), the balance between ecosystem respiration (ER) and gross primary production (GPP) can vary with changes in water table position (Belyea, 2009; Strack et al., 2009). For example, a decade of research in an interior Alaskan fen has shown that drought conditions generally reduced GPP, but had muted effects on ER, which switched from increased autotrophic respiration in wet years to increased heterotrophic respiration in dry years (Olefeldt et al., 2017). However with flooding, variation in respiration in this fen complex changed with the timing and sources of inundation, with persistent flooding late into the growing season resulting in lower GPP relative to ER (Euskirchen et al., 2020). Exactly how constituent autotrophic and heterotrophic respiration components are likely to change with different regimes of flooding are not yet well understood.
A key difference in the active mode of primary production during flooded conditions, which affords a photic zone within the water column, is the relative activity of algae (Goldsborough and Robinson, 1996). While little is still known about algal production in boreal fens, recent work highlights the importance of algae as a component of the photosynthetic community during flooded conditions in interior Alaskan peatlands (Wyatt and Turetsky, 2015; DeColibus et al., 2017). Moreover, antecedent drainage conditions prior to flooding have been shown to exert strong controls over algal production. Experimental drainage in an Alaskan fen peatland resulted in increased pore water nutrient concentrations which fostered algal production upon flooding due to high rainfall (Wyatt et al., 2012). However, exactly how long this “ecosystem memory” of antecedent dry conditions can affect algal production, and how this in turn affects net C balance with persistent flooding is not clear (DeColibus et al., 2017). For example, observed increases in the exudates (dissolved organic matter, DOM) concomitant with increased algal production are very bioavailable (Bertilsson and Jones, 2003; Wyatt et al., 2012; Wyatt and Rober 2020) and could increase ER with persistent flooding. As such, component fluxes of ecosystem respiration along with algal production need to be measured together during periods of persistent flooding to ascertain the net consequences of flooding history on C balance in fens.
We investigated trace gas production, algal biomass, and DOM quality in an experimental manipulation of water table positions that simulate both drought (lowered water table treatment) and flooding (raised water table treatment) conditions relative to a control treatment without manipulation in an Alaskan boreal rich fen. We conducted our measurements prior to flooding across all experimental treatments and after two years of consecutive flooding. Our over-arching hypothesis was that hydrologic variation affects the balance between GPP and ER (Figure 1). With flooding, we expected algal production to offset carbon losses through ER, increasing the C sink-strength of the ecosystem. As such, we hypothesized the lowered water table treatment would be a stronger sink of C when reflooded owing to greater algal biomass associated with elevated nutrient availability, whereas the previously raised water table treatment would be a weaker sink of C when flooded, owing to reduced GPP from vascular plants. On the other hand, increased water-soluble organic carbon, algae-derived DOM and nutrients from the lowered water table treatment could increase heterotrophic respiration, and hence drive higher ER relative to GPP in the flooded environment. However, exactly how antecedent changes in DOM quality persist with prolonged flooding periods are not well understood. Therefore, we specifically ask: 1) How does the legacy of flooding history influence peatland C balance? 2) What is the role of DOM quality in driving ecosystem C fluxes and how does algal biomass relate to DOM quality?
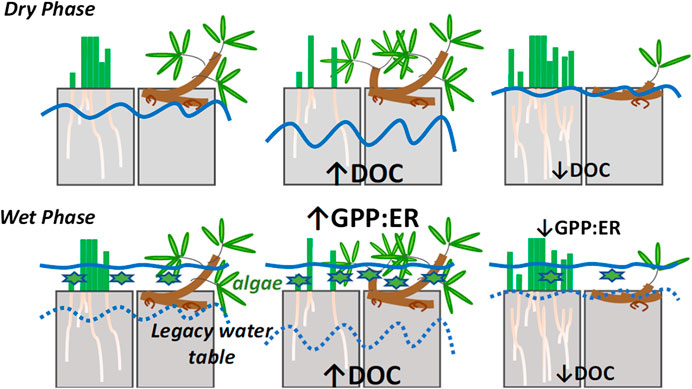
FIGURE 1. Conceptual model of how our experimental water table treatments (control, lowered and raised) have affected the pool size of nutrients, dissolved organic carbon [DOC] and its quality, and how these factors have affected algal production during flooding (wet phase). We hypothesize that these factors affect the balance between gross primary production and ecosystem respiration (GPP:ER). The solid blue lines indicate water table position by treatment, and the dashed lines indicate legacy water table position during flooding. The green stars indicate the relative abundance of algae. The plants indicate relative abundances of sedges and shrubs (see Rupp et al., 2019).
Materials and Methods
Study Site and Experimental Design
The rich fen (surface pH ∼6) is in the boreal peatland lowlands of the Tanana Flats of interior Alaska (cf. Hopkins et al., 1955) approximately 30 km southeast of Fairbanks, Alaska United States. The vegetation and climate characteristics of this site have been previously described in detail (Churchill et al., 2015; Olefeldt et al., 2017; Euskirchen et al., 2020), but briefly, emergent vascular species (Equisetum, Carex, and Comarum (Potentilla) genera) and some brown mosses and Sphagnum dominate the site. The fen complex lacks permafrost but is within the region of discontinuous permafrost in Alaska’s interior. There is little microtopography at the rich fen, which has accumulated peat to a depth of approximately 1–1.5 m. The site is associated with the Bonanza Creek Long Term Ecological Research Program (lter.uaf.edu).
The Alaska Peatland Experiment (APEX) was established in 2005 to investigate the effects of altered hydrologic regimes on C cycling processes in a controlled experiment. Three plots (approximately 20 × 20 m) were assigned each to one of three water table (WT) treatments (raised, lowered, and a control reference plot, each approximately 25 m apart), as previously described in detail (see Turetsky et al., 2008 and Chivers et al., 2009 for experiment details). Briefly, in the winter of 2005 channels were dug to facilitate water drainage from the lowered water table plot. This water in turn was pumped into the raised water table treatment with solar-powered bilge pumps, throughout the growing season (approximately June-September). There were no significant differences in water table position or vegetation composition among plots prior to treatment initiation. The goal of the experiment was to maintain lowered (drained) and raised water table positions relative to the control while allowing for the natural seasonal variability in water table position at this site. However, it was impossible to experimentally manipulate the water table position during periods of natural flooding, including years when flooding (water table > 40 cm above peat surface) persisted for the entire growing season (2014, 2017 and 2018; see Euskirchen et al., 2020 for hydrograph data). In this current study, we measured trace gas flux, algal biomass, and DOM quality within the APEX plots prior to a natural flooding event in 2016, which persisted through 2017 and 2018. We remeasured the experimental plots after continuous flooding during the growing season of 2018 to test our hypotheses regarding persistent flooding effects on C cycle dynamics.
Gas Flux Measurements
We used the static chamber technique for gas flux measurements during non-flooded years as previously described in detail (Chivers et al., 2009). Briefly, six collars (60 × 60 cm) were inserted to a depth of 10 cm in 2005 within each WT treatment. A clear lexan chamber (0.227 m3) with closed-cell foam sealed the chamber with the collar. Two computer CPU fans mixed the air within the chamber during measurements. The change in chamber CO2 concentrations was measured for approximately 4 min using a portable infrared gas analyzer (IRGA; PP Systems EGM 4, Amesbury, Massachusetts, United States). ER was measured by shading chambers with an opaque shroud and net ecosystem exchange (NEE) was measured without a shroud. Temperature, relative humidity, and photosynthetically active radiation (PAR) were logged continuously within the chamber during each flux measurement with a PP Systems TRP-1 sensor. Measurements occurred approximately bi-weekly.
A floating chamber was employed for gas flux measurements in flooded conditions during the growing season of 2018. This static chamber was constructed from a clear polycarbonate plastic bucket (18.9 L) with Styrofoam floatation around the base and one CPU fan inside for air circulation. This design allows for the measure of CO2 flux in the airspace above the water. The floating chamber was equipped with an airlock to equilibrate pressure. The clear chamber was covered with an opaque shroud during ER measurements and NEE was measured without a shroud. Temperature, relative humidity, and PAR were logged continuously with a PP Systems TRP-1 sensor mounted within the clear floating chamber. The change in chamber CO2 concentrations was measured with an IRGA as previously described. Floating chamber measurements were conducted within water table manipulation plots (within plot n = 3) from a small inflatable boat and by wading along the established boardwalk network.
Algal Biomass
Algal material was collected biweekly during the 2018 growing season for estimates of algal biomass measured as chlorophyll a (mg cm−2). Owing to shallow water with a well-lit photic zone, algae within this fen complex grow primarily affixed to submersed substrata (DeColibus et al., 2017). Attached algal material was collected from four senesced Carex utriculata stems (10 cm length) cut below the water surface within each of three randomly selected 1 m2 areas per water table treatment using methods modified from DeColibus et al. (2017). There was no floating or loosely attached algae (i.e. metaphyton) observed during the study. Each composite sample was placed into a clean 15 ml polyethylene tube and kept on ice until returning to the lab where samples were frozen at −20°C until subsequent processing. Algal chlorophyll a was extracted with 90% ethanol in the dark for 24 h and the resulting pigment extracts were analyzed with an Agilent Cary 60 UV-Vis spectrophotometer (Agilent Technologies, Santa Clara, CA, United States) at 665 and 750 nm after acidification to correct for phaeophytin (APHA, 1998).
Dissolved Organic Matter Quality
When the water table was below the peat surface in 2016, a stainless steel “sipper” (0.52 cm diameter tubing with 2 cm slotted region at the end) was carefully inserted into the peat to a depth of 20 cm. Pore water was drawn up through the sipper into a syringe that was rinsed first with deionized (DI) water, then with sample prior to collection. When conditions were flooded in 2018, surface waters from the photic zone were collected in addition to pore water. Specifically, a clean, sample rinsed 60 ml syringe was used to sample the water column 10 cm below the surface of the water. After collection, the sample was injected into a 125 ml dinitrogen gas flushed and evacuated Wheaton glass vial capped with a butyl rubber septa via a 0.45 μm Whatman syringe filter and needle and then refrigerated prior to analysis, following Rupp et al. (2019). Samples were collected at three locations, spaced approximately 3 m apart, in each WT treatment throughout the growing seasons of 2016 (prior to flooding) and 2018 (during sustained flooding). Dissolved organic carbon (DOC) and total dissolved nitrogen (TDN) were determined on samples within 10 days on an TOCV Analyzer with TDN module (Shimadzu Scientific Instruments, Columbia, MD, United States).
Pore water was extracted from each of the Wheaton vials and run on a fluorometer (Horiba–Jobin–Yvon Aqualog C; Horiba Co., Edison, NJ) to simultaneously collect UV–Vis absorbance and florescence spectra. Run parameters were excitation: 240–600 nm in 3 nm increments; emission: 212–608 nm by 3 nm bandpass; integration time = 0.25 s. Samples with absorbance greater than 0.6 at λ = 254 were diluted to satisfy the assumption of detector linearity required by modeling (Stedmon and Bro 2008; Lawaetz and Stedmon 2009). Data post-processing and correction for inner filter effects were as described in detail by Veverica et al. (2016), following Stedmon and Bro (2008) and Lawaetz and Stedmon (2009).
Fluorescence and absorbance spectroscopic indices were calculated to characterize dissolved organic matter in all samples as previously described (Veverica et al., 2016; Rupp et al., 2019). Fluorometric indices considered in this study included an index of DOM humification (humification index; HIX; Zsolnay et al., 1999; Ohno, 2002; cf. Zsolnay, 2002). Higher HIX values can generally be considered as more biologically recalcitrant or aromatic in boreal systems (Chen et al., 2011; Frey et al., 2016; Herzsprung et al., 2017). We calculated an index of free or bound proteins (tyrosine or tryptophan index; TI; Fellman et al., 2010; cf. Chen et al., 2003). Higher TI values are considered relatively labile in comparison to the humified products associated with HIX (cf. Cory and Kaplan, 2012). We also calculated the Spectral slope ratio (Sr), an absorbance index of photodegradation, molecular weight (Helms et al., 2008) and source (Chen et al., 2011). The Sr is also an inverse indicator of relatively labile carbon compounds, with lower values associated with more bioavailable DOM (Frey et al., 2016).
Statistics and Analyses
We examined WT treatment effects, flooding, and their interaction (WT treatment x flooded or not flooded state) on CO2 flux data (ER, GPP, NEE), algal biomass, and DOM composition variables (HIX, Sr, TI). We collected these data throughout the growing seasons of 2016 (pre-flood; four campaigns) and 2018 (after continuous flooding; five campaigns). We used a generalized linear mixed model approach to examine differences in CO2 flux and DOM composition data prior to flooding and in the flooded state; this approach enables statistical models to be fit to data where the response is not necessarily normally distributed (PROC GLIMMIX; SAS version 9.4, SAS Institute, Cary, North Carolina, United States). Sampling campaign was treated as a random effect to account for repeated measures. The distributions of response variables were evaluated in Kolmogorov-Smirnov tests using the UNIVARIATE procedure, and were assigned as gaussian or lognormal as appropriate. Differences in DOC and TDN concentrations were explored using a mixed effects model via the “nlme” package in R statistical software version 3.4.2 (R Core Development Team, 2017). The WT treatment was used as a fixed effect, while sampling date was a random effect to account for repeated measures. The TDN values were transformed using the power-based Tukey Ladder of Powers transformation via the “rcompanion” package (Mangiafico, 2018; TDN 10 cm below water surface lambda = 2.2; TDN 20 cm below peat surface lambda = 1.5). We explored relationships with algal biomass (chlorophyll a) and ER, Sr, and TI with linear regression. Type-III tests of fixed effects and post hoc comparisons of least squared means tests across treatments were considered significant at α = 0.05. All data used in these analyses are available in the public domain via the Bonanza Creek Data Catalog (https://www.lter.uaf.edu/data/data-catalog).
Results
Change in Carbon Balance
Across all treatments, there were changes in ER and GPP in response to flooding that resulted in interactions between drainage history and flooding for net C balance (Table 1). Prior to flooding, the raised water table (WT) treatment had marginally lower ER (2.79 ± 0.14 CO2 μmol m−2 s−1) than the lowered (3.25 ± 0.30 CO2 μmol m−2 s−1) or control (4.10 ± 0.54 CO2 μmol m−2 s−1) treatments (F = 3.31, p = 0.08), though there were no differences in ER among treatments after flooding (mean of 3.28 ± 0.63 CO2 μmol m−2 s−1; F = 0.75, p = 0.60; cf. Figure 2). In contrast, GPP was reduced (less uptake, less negative) following flooding in both the control (−8.22 ± 0.65 vs. −5.33 ± 0.99 CO2 μmol m−2 s−1) and raised (−7.19 ± 0.33 vs. −4.18 ± 0.99 CO2 μmol m−2 s−1) treatments, whereas in the lowered treatment GPP did not decrease with flooding (−6.36 ± 0.82 vs. −6.07 ± 0.34 CO2 μmol m−2 s−1), resulting in an interaction between WT treatment and flooding (Table 1; F = 4.28, p = 0.045). As a result of these processes the site was a stronger sink of C prior to flooding (mean NEE −3.88 ± 0.67 CO2 μmol m−2 s−1) than after flooding (−1.18 ± 0.87 CO2 μmol m−2 s−1), with the lowered treatment becoming a stronger sink, and the raised treatment being a source of C on one measurement day with flooded conditions (Figure 2B). The change in the C sink-strength with flooding in the raised treatment contributed to a weak interaction between water table treatment and flooding in explaining variation in NEE (Table 1; F = 4.09, p = 0.05).
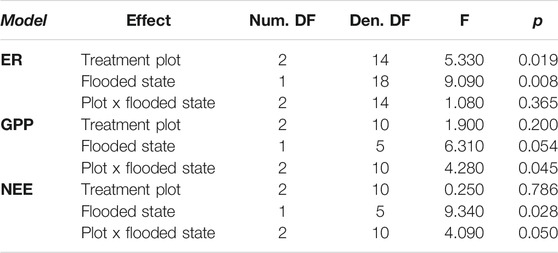
TABLE 1. Results of mixed effects models testing the effects of experimental water table treatment, flooding, and their interaction on ecosystem respiration (ER), gross primary production (GPP) and net ecosystem exchange (NEE). Model numerator (Num.) and Denominator (Den.) degrees of freedom (DF) are provided.
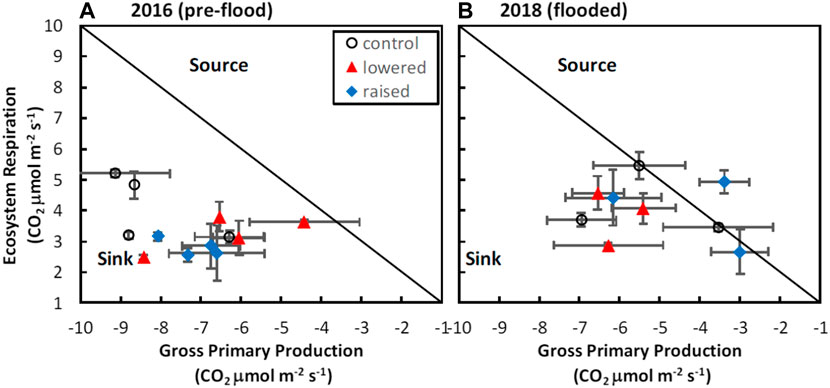
FIGURE 2. Changes in ecosystem carbon balance prior to flooding, and after two years of continuous flooding. Depicted are means for a given campaign by water table treatment plot. Error bars represent standard errors of the mean values.
In 2018, mean [DOC] obtained from 10 cm beneath the surface of the water table was significantly lower in the raised WT treatment (32 ± 1.4 mg L−1) than in both the lowered (36 ± 0.4 mg L−1) or control (39 ± 0.5 mg L−1) treatments (F = 16.53, p < 0.001). Mean [DOC] obtained from 20 cm beneath the surface of the peat was also lower in the raised WT treatment (56 ± 3 mg L−1) than in both the lowered (93 ± 7 mg L−1) or control (80 ± 2 mg L−1) treatments (F = 29.88, p < 0.001). There were no WT treatment effects on mean [TDN] obtained from 10 cm beneath the surface of the water table (0.5 ± 0.1 mg L−1; F = 0.73, p = 0.49). Mean [TDN] obtained from 20 cm beneath the surface of the peat was lower in the raised WT treatment (1.1 ± 0.1 mg L−1) than in both the lowered (2.8 ± 0.2 mg L−1) or control (2.1 ± 0.2 mg L−1) treatments (F = 20.61, p < 0.001).
Algal Biomass and Carbon Quality
Algal biomass (chlorophyll a) was significantly higher in the control (mean ± standard error; 2.61 mg m−2 ± 0.46) and lowered (2.57 mg m−2 ± 0.38) treatment plots compared with the raised (1.55 mg m−2 ± 0.22) treatment (F = 4.24, p = 0.04). Ecosystem respiration and the index of free or bound-in proteins (TI) increased with algal biomass, while the degree of DOM photodegradation or molecular weight (Sr, or inversely related to humification, or HIX) declined with algal biomass (Figure 3). After accounting for changes in chlorophyll a content in mixed models (Type III tests of fixed effects), WT treatment also had significant effects on Sr (F = 6.50, p = 0.03), and marginally significant treatment effects on ER and TI (F = 3.12, p = 0.09 and F = 3.50, p = 0.08, respectively).
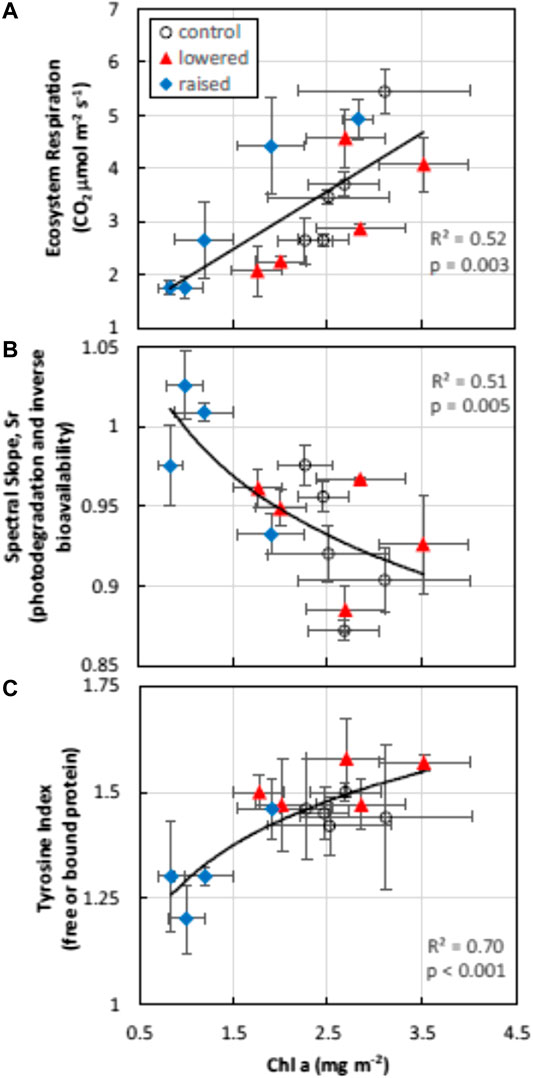
FIGURE 3. Algal biomass (chlorophyll a content) was strongly related to changes in ecosystem respiration (top pane) measured with floating chambers during flooding, the bioavailability of DOM (inversely related to spectral slope ratio, Sr, middle pane) and the content of free or bound protein (TI, bottom pane). Depicted are daily means by water table treatment plot in the flooded year (2018). Error bars represent standard errors of the mean values.
DOM Quality Before and After Flooding
Indices of DOM quality changed as a function of WT treatment and flooding. Prior to flooding (2016), DOM humification (HIX) at 20 cm in the peat was greater in the lowered water table treatment than in the raised WT treatment (F = 8.09, p = 0.004; Figure 4A). Following flooding, humification was much more variable at the lowered WT treatment, and was only significantly different (lower value) in the raised treatment (Figure 4B; p < 0.001). Overall, the degree of humification was higher, in the flooded year, in the control and lowered plots (year x WT treatment interaction, F = 9.84, p < 0.001). In contrast, Sr, an indicator of photodegradation and an inverse indicator of molecular weight and bioavailability, was higher in the raised WT treatment than in the other treatments (Figures 4C, D), and there was no difference between the flooded and non-flooded years (p = 0.70). The index of free or bound-in proteins (TI) did not vary by WT treatment prior to flooding (Figure 4E; p = 0.17), but was significantly greater in the lowered WT treatment, and less in the raised treatment following flooding (Figure 4F; p < 0.001). Moreover, TI was greater overall in the flooded year than prior to flooding (F = 17.67, p < 0.001) and there was a marginal year x WT treatment interaction (F = 2.92, p = 0.06). These changes in DOM quality measured at 20 cm within the peat pore water were maintained in DOM collected within the water column two years after persistent flooding (10 cm from water table surface), with the raised plot being different from the other treatments (Figure 5). With flooding, DOM in the water column of the raised WT treatment exhibited less bioavailability or increased photodegradation (Sr; F = 6.17, p = 0.005), a lower degree of humification (HIX; F = 11.29, p < 0.001) and less proteinaceous compounds (TI; F = 8.12, p = 0.001).
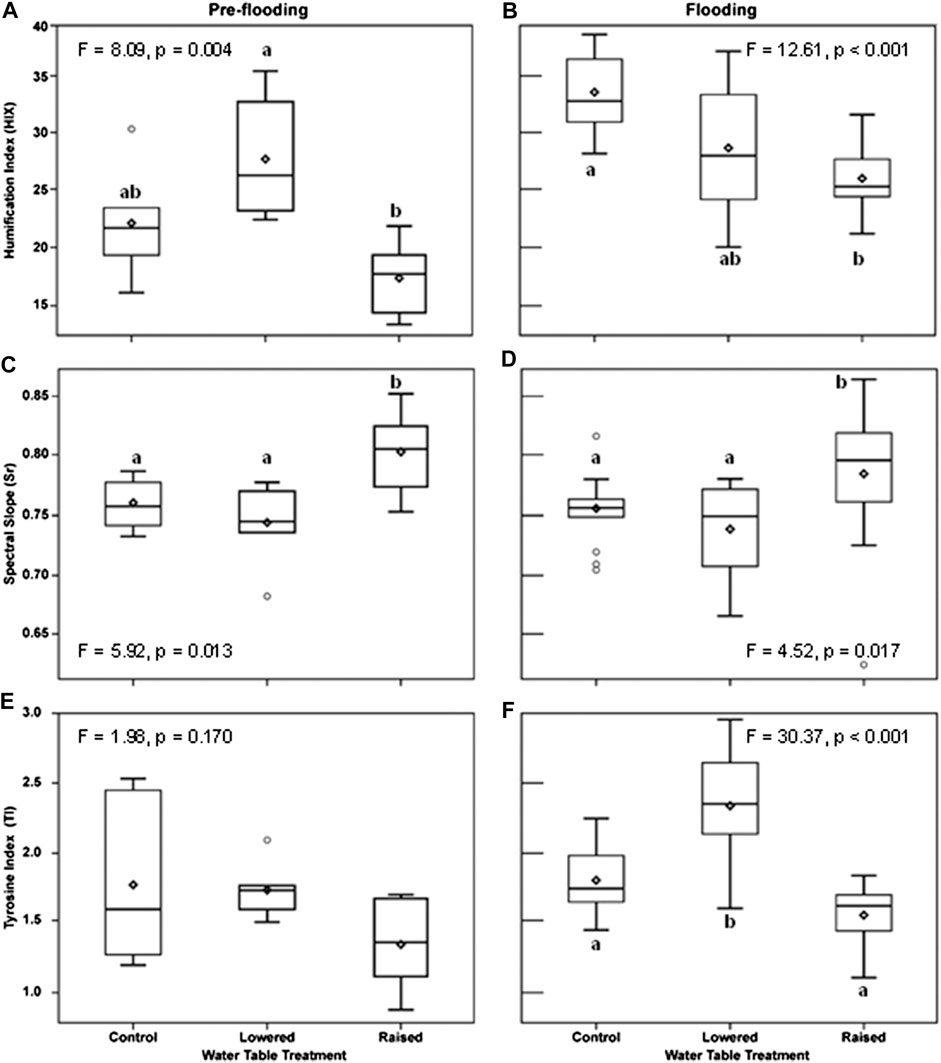
FIGURE 4. Indexes of peat pore water (20 cm into the peat) character before flooding in 2016 (A,C,E) and after continuous flooding, measured in 2018 (B,D,F). Depicted are seasonal means by water table treatment plot. An index of DOM humification (HIX) was higher in the flooded year (b, F = 44.09, p < 0.001), and there was a year × treatment interaction (F = 8.84, p < 0.001). An inverse index of DOM bioavailabilty and molecular size (Spectral Slope Ratio, Sr) did not vary by year (F = 0.15, p = 0.70), but was higher in the raised treatment (C,D). An index of peat pore water free or bound protein (TI) was higher in the flooded year (F = 17.67, p < 0.001), and the lowered treatment was higher than the raised treatment (F). Letters indicate significant differences in post-hoc comparisons of means within a treatment year (flood state).
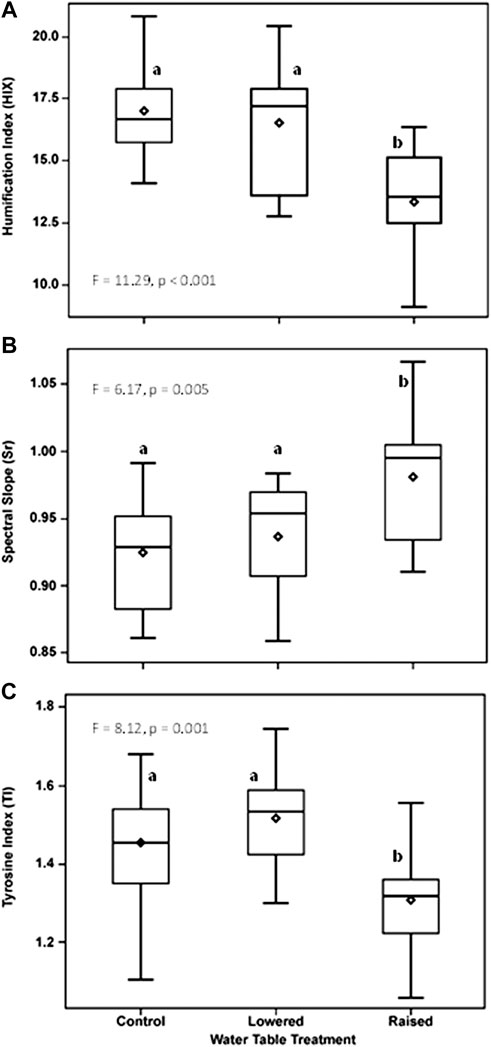
FIGURE 5. Water table treatment differences in an index of humification (HIX, (A), an index of photodegradation or an inverse index of bioavailability (Sr, (B), and an index of free or bound protein (TI, (C) of water taken 10 cm below the surface of the water during flooding throughout the 2018 growing season. Depicted are seasonal means by water table treatment plot. Letters indicate significant differences in post-hoc comparisons of means.
Discussion
Carbon Balance With Drainage History and Flooding
In conditions of variable hydrology, lowering the water table generally promotes an increase in both ER and GPP, which has made it difficult to ascribe single mechanisms for the sign of net C balance with hydrologic change (Weltzin et al., 2003; Strack and Waddington, 2007; Ballantyne et al., 2014). A major difference between previous studies and this current work, and fens in general, was the prolonged period of saturation where water table remained above the peat surface. Prior work at this site had shown no significant WT treatment effects on ER (Chivers et al., 2009; Olefeldt et al., 2017), but was not able to investigate WT treatment effects on C cycling when the site was flooded. More recent work has shown that the site as a whole exhibited higher ER in years when flooding occurred from the spring season onward (Euskirchen et al., 2020). This work showed that with persistent flooding, the previously raised WT treatment exhibited higher ER relative to GPP, with the most parsimonious explanation being limitations to the production of algal biomass (cf. Figure 3). While prior work had also shown the ability of algae to capitalize on the nutrients released from increased decomposition in the lowered WT treatments when flooded (DeColibus et al., 2017), this current work demonstrated that algae also affected ecosystem C balance. Interestingly, the increase in labile C supply in response to algal production (e.g., higher TI) did not result in a significant increase in the relative amount of heterotrophic respiration (cf. Wyatt et al., 2019), which certainly warrants further investigation as to the limiting factors of ER when the ecosystem is flooded. For example, the protein-like fluorescence in this study may not be reflecting the pool of bioavailable DOM (Maie et al., 2007). These findings refine our prior hypothesis (Olefeldt et al., 2017) that the lack of a response in ER to WT treatments reflects a shift in the component flux contributions to ER, from autotrophic respiration in wet years to heterotrophic respiration in dry years, and demonstrate that an algal-mediated increase in GPP in the lowered WT treatments can attenuate net C losses during flooding.
Insights Gained From DOM Composition
The DOM absorbance and fluorescence spectroscopy provided insights into C cycle processes within the peat prior to and after two years of flooding. Prior to flooding, pore water was more humified in the lowered WT treatment, which is consistent with the accumulation of water-soluble products of increased decomposition in a broader, more aerobic region above the water table, or acrotelm (Strack et al., 2008; Tfaily et al., 2018). HIX remained higher (and yet more variable) in the lowered WT treatment upon being flooded, which is consistent with prior work demonstrating that a sustained HIX upon rewetting drained peatland soil represents an increased amount of oxygen-rich and relatively unsaturated (O/C > 0.5; H/C < 1.0) DOM (Herzsprung et al., 2017). The lower HIX values in the raised WT treatment, regardless of flooding history, suggest less accumulation of the products of peat mineralization. Lower HIX is consistent with the increased Sr (Chen et al., 2011) observed at the raised WT treatment, and could also be reflecting increased photodegradation of the DOM as opposed to biological degradation of the DOM (Helms et al., 2008; Wilske et al., 2020). Increased photodegradation would be facilitated by the water table being at or slightly above the peat surface in the raised WT treatment. The potential for DOM photodegradation to CO2 could be contributing to the lower C sink strength in the raised WT treatment. In turn, DOM with relatively high Sr exhibits low bioavailability (Frey et al., 2016). In contrast, TI—an index of free or bound proteins, is generally thought to be highly bioavailable (Cory and Kaplan, 2012). There were no WT treatment effects on soil TI values prior to flooding, but significant increases in the lowered WT treatment and decreases in the raised WT treatments were measured when flooded (Figure 4E, F; Figure 5). Taken together, these indices indicate that the raised WT treatment had less bioavailable DOM within the peat (higher Sr, low TI) than the lowered WT treatment, even though the lowered WT treatment also had a fraction of more humified DOM (higher HIX). A parsimonious explanation for increased HIX and decreased Sr with increasing TI is the increase in algal production and algal exudation in response to the increased nutrients released in the lowered WT treatment relative to the raised WT treatment (Wyatt et al., 2012), while also having more water-soluble products from increased decomposition in the lowered WT treatment prior to flooding (Kane et al., 2010). Moreover, it could be that the protein-like fluorescence in this case reflects higher molecular weight lignin polyphenols (Herns et al., 2009; Stubbins et al., 2014), and more detailed analysis of the presence of aromatic amino acids in this system is certainly warranted.
These results are consistent with prior work demonstrating the ability of algae to capitalize on the “legacy effect” of prior drainage and nutrient release (Wyatt et al., 2012; DeColibus et al., 2017), and further demonstrate that the elevated nutrient legacy effects on algal production can persist after two consecutive years of flooding. While vascular plants are a significant component of NEE (Rupp et al., 2019), photosynthetic capacity (NDVI) has been shown to be lower in the lowered WT treatment during flooded conditions (McPartland et al., 2019), which further suggests a different mode of primary production to be driving C uptake when flooded. The duration of flooding is an important consideration for C cycling and algal production. For example, relatively short-term (<40 days) wet events did not generate lag effects in C cycling, and the lowered WT treatment in wet years exhibited similar GPP and NEE to that of the raised WT treatment in dry years (Olefeldt et al., 2017). After persistent flooding, however, the lowered WT treatment became the strongest C sink, whereas the raised WT treatment became a source (e.g., Table 1; Figure 2). However, it is not clear how long the “legacy effect of drought” can continue to fuel increased algal production. For example, nutrient co-limitations or competition with vascular plants may override any nutrient legacy effect on algal production over the span of decades (Lougheed et al., 2011; Lougheed et al., 2015). Flooding events at this rich fen site have been increasing over time, commensurate with the study region becoming a greater source of C to the atmosphere (Euskirchen et al., 2020). If periods of inundation increase in duration (i.e., become more lake-like), as has been observed in other regions of interior Alaska (Jorgenson et al., 2001), these data suggest rich fens could become a weaker sink for C, with reduced algal production likely being a contributing factor. These findings indicate that incorporating an understanding of flooding history and its effects on algal production are important in assessments of C storage in boreal fen peatlands.
Data Availability Statement
The datasets presented in this study can be found in online repositories. The names of the repository/repositories and accession number can be found below: All data used in these analyses are available in the public domain via the Bonanza Creek Data Catalog (https://www.lter.uaf.edu/data/data-catalog).
Author Contributions
All authors contributed to the conception of this study. EK lead the analyses and writing, with input from all authors. CD lead field activities during flooding (2018), with input from all authors. KW and AR lead algal biomass methods and analyses.
Funding
This project was funded by National Science Foundation grants DEB LTREB-1354370 (to EK and MT) and DEB-1651195 (to KW and AR). The APEX site has been supported by National Science Foundation Grants DEB-0425328, DEB-0724514 and DEB-0830997 (to MT). The Bonanza Creek Long-Term Ecological Research program was funded jointly by NSF Grant DEB-0620579 and an USDA Forest Service Pacific Northwest Research Grant PNW01-JV11261952-231. The project received in-kind support from the U.S.D.A. Forest Service, Northern Research Station (Houghton, MI, United States). Jamie Hollingsworth and the Bonanza Creek LTER site provided tremendous support for this project.
Conflict of Interest
The authors declare that the research was conducted in the absence of any commercial or financial relationships that could be construed as a potential conflict of interest.
Acknowledgments
We thank Morgan Brown, Evan Schijns, Mara McPartland, Lucas Albano, and Natalie Zwanenburg for their help in the field and Karl Meingast for his expertise in spectroscopy.
References
APHA (1998). Standard methods for the examination of water and wastewater. 20th edition. Washington, DC: American Public Health Association.
Ballantyne, D. M., Hribljan, J. A., Pypker, T. G., and Chimner, R. A. (2014). Long-term water table manipulations alter peatland gaseous carbon fluxes in Northern Michigan. Wetl. Ecol. Manag. 22 (1), 35–47. doi:10.1007/s11273-013-9320-8
Belyea, L. R. (2009). “Nonlinear dynamics of peatlands and potential feedbacks on the climate system,” in Carbon cycling in northern peatlands. Editors A. J. Baird, L. R. Belyea, X. Comas, A. S. Reeve, and L. D. Slater (Washington, DC: American Geophysical Union Geophysical Monograph), 184, 5. doi:10.1029/2008GM000829
Bertilsson, S., and Jones, J. B. (2003). “Supply of dissolved organic matter to aquatic ecosystems: autochthonous sources,” in Aquatic ecosystems: interactivity of dissolved organic matter. Editors S. E. G. Findlay, and R. L. Sinsabaugh (San Diego, CA: Academic Press), 3–25.
Chen, H., Zheng, B., Song, Y., and Qin, Y. (2011). Correlation between molecular absorption spectral slope ratios and fluorescence humification indices in characterizing CDOM. Aquat. Sci. 73, 103–112. doi:10.1007/s00027-010-0164-5
Chen, W., Westerhoff, P., Leenheer, J. A., and Booksh, K. (2003). Fluorescence excitation-emission matrix regional integration to quantify spectra for dissolved organic matter. Environ. Sci. Technol. 37, 5701–5710. doi:10.1021/es034354c
Chivers, M. R., Turetsky, M. R., Waddington, J. M., Harden, J. W., and McGuire, A. D. (2009). Effects of experimental water table and temperature manipulations on ecosystem CO2 fluxes in an Alaskan rich fen. Ecosystems 12, 1329–1342. doi:10.1007/s10021-009-9292-y
Churchill, A. C., Turetsky, M. R., McGuire, A. D., and Hollingsworth, T. N. (2015). Response of plant community structure and primary productivity to experimental drought and flooding in an Alaskan fen. Can. J. For. Res. 45, 185–193. doi:10.1139/cjfr-2014-0100
Cory, R. M., and Kaplan, L. A. (2012). Biological lability of streamwater fluorescent dissolved organic matter. Limnol. Oceanogr. 57 (5), 1347–1360. doi:10.4319/lo.2012.57.5.1347
DeColibus, D. T., Rober, A. R., Sampson, A. M., Shurzinske, A., Walls, J. T., Turetsky, M. R., et al. (2017). Legacy effects of drought alters the aquatic food web of a northern boreal peatland. Freshw. Biol. 62, 1377–1388. doi:10.1111/fwb.12950
Euskirchen, E. S., Bennett, A., Breen, A. L., Genet, H., Lindgren, M., Kurkowski, T., et al. (2016). Consequences of changes in vegetation and snow cover for climate feedbacks in Alaska and northwest Canada. Environ. Res. Lett. 11 (10, 105003. doi:10.1088/1748-9326/11/10/105003
Euskirchen, E. S., Kane, E. S., Edgar, C. W., and Turetsky, M. R. (2020). When the source of flooding matters: divergent responses in carbon fluxes in an alaskan rich fen to two types of inundation. Ecosystems 23, 1138–1153. doi:10.1007/s10021-019-00460-z
Fellman, J. B., Hood, E., and Spencer, R. G. M. (2010). Fluorescence spectroscopy opens new windows into dissolved organic matter dynamics in freshwater ecosystems: a review. Limnol. Oceanogr. 55 (6), 2452–2462. doi:10.4319/lo.2010.55.6.2452
Ford, J., and Bedford, B. L. (1987). The hydrology of Alaskan wetlands, United States: a review. Arctic Antarct. Alpine Res. 19 (3), 209–229. doi:10.1080/00040851.1987.12002596
Frey, K. E., Sobczak, W. V., Mann, P. J., and Holmes, R. M. (2016). Optical properties and bioavailability of dissolved organic matter along a flow-path continuum from soil pore waters to the Kolyma River mainstem, East Siberia. Biogeosciences 13, 2279–2290. doi:10.5194/bg-13-2279-2016
Goldsborough, L. G., and Robinson, G. G. C. (1996). “Pattern in wetlands,” in Algal Ecology: freshwater benthic ecosystems. Editors R. J. Stevenson, M. L. Bothwell, and R. L. Lowe (New York, NY: Academic Press), 77–117.
Goldstein, A., Turner, W. R., Spawn, S. A., Anderson-Teixeira, K. J., Cook-Patton, S., Fargione, J., et al. (2020). Protecting irrecoverable carbon in Earth’s ecosystems, Nat. Cli. Change 10, 287–295. doi:10.1038/s41558-020-0738-8
Helms, J. R., Stubbins, A., Ritchie, J. D., Minor, E. C., Kieber, D. J., and Mopper, K. (2008). Absorption spectral slopes and slope ratios as indicators of molecular weight, source, and photobleaching of chromophoric dissolved organic matter. Limnol. Oceanogr. 53 (3), 955–969. doi:10.4319/lo.2008.53.3.0955
Herns, P. J., Bergamaschi, B. A., Eckard, R. S., and Spencer, R. G. M. (2009). Fluorescence-based proxies for lignin in freshwater dissolved organic matter. J. Geophys. Res. 114, G00F03. doi:10.1029/2009JG000938
Herzsprung, P., Osterloh, K., von Tümpling, W., Harir, M., Hertkorn, N., Schmitt-Kopplin, P., et al. (2017). Differences in DOM of rewetted and natural peatlands—Results from high-field FT-ICR-MS and bulk optical parameters. Sci. Total Environ. 586, 770–781. doi:10.1016/j.scitotenv.2017.02.054
Hopkins, D. M., Karlstrom, T. N. V., Black, R. F., Williams, J. R., Pewe, T. L., Fernold, A. T., et al. (1955). Permafrost and ground water in Alaska. U. S. Geol. Surv. Prof. Pap. 264‐F, 113–130.
Jorgenson, M. T., Racine, C. H., Walters, J. C., and Osterkamp, T. E. (2001). Permafrost degradation and ecological changes associated with a warming climate in central Alaska. Climatic Change 48, 551–579. doi:10.1023/A:1005667424292
Kane, E. S., Turetsky, M. R., Harden, J. W., McGuire, A. D., and Waddington, J. M. (2010). Seasonal ice and hydrologic controls on dissolved organic carbon and nitrogen concentrations in a boreal‐rich fen. J. Geophys. Res. 115, G04012. doi:10.1029/2010JG001366
Kolka, R., Trettin, C., Tang, W., Krauss, K., Bansal, S., Drexler, J., et al. (2018). “Chapter 13: Terrestrial wetlands,” in Second state of the carbon cycle report (SOCCR2): a sustained assessment report. Editors N. Cavallaro, G. Shrestha, R. Birdsey, M. A. Mayes, R. G. Najjar, S. C. Reedet al. (Washington, DC: Z. U.S. Global Change Research Program), 507–567. doi:10.7930/SOCCR2.2018.Ch13
Lara, M. J., Genet, H., McGuire, A. D., Euskirchen, E. S., Zhang, Y., Brown, D., et al. (2016). Thermokarst rates intensify due to climate change and forest fragmentation in an Alaskan boreal forest lowland. Global Change Biol. 22, 816–829. doi:10.1111/gcb.13124
Lawaetz, A. J., and Stedmon, C. (2009). Fluorescence intensity calibration using the Raman scatter peak of water. Appl. Spectrosc. 63 (8), 936–940. doi:10.1366/000370209788964548
Lougheed, V. L., Butler, M. G., McEwen, D. C., and Hobbie, J. E. (2011). Changes in Tundra pond limnology: re-sampling Alaskan ponds after 40 years. Ambio 40 (6), 589–599. doi:10.1007/s13280-011-0165-1
Lougheed, V. L., Hernandez, C., Andresen, C. G., Miller, N. A., Alexander, V., and Prentki, R. (2015). Contrasting responses of phytoplankton and benthic algae to recent nutrient enrichment in Arctic tundra ponds. Freshw. Biol. 60, 2169–2186. doi:10.1111/fwb.12644
Maie, N., Scully, N. M., Pisani, O., and Jaffé, R. (2007). Composition of a protein-like fluorophore of dissolved organic matter in coastal wetland and estuarine ecosystems. Water Res. 41, 563–570. doi:10.1016/j.watres.2006.11.006
Mangiafico, S. (2018). Package “rcompanion”: functions to support extension education program evaluation. CRAN R Project. Available at: https://cran.r-project.org/web/packages/rcompanion/rcompanion.pdf
McPartland, M. Y., Kane, E. S., Falkowski, M. J., Kolka, R., Turetsky, M. R., Palik, B., et al. (2019). The response of boreal peatland community composition and NDVI to hydrologic change, warming, and elevated carbon dioxide. Global Change Biol. 25, 93–107. doi:10.1111/gcb.14465
Ohno, T. (2002). Comment on “Fluorescence inner-filtering correction for determining the humification index of dissolved organic matter”. Environ. Sci. Technol. 36 (4), 4195–4196. doi:10.1021/es0155276
Olefeldt, D., Euskirchen, E. S., Harden, J., Kane, E., McGuire, A. D., Waldrop, M., et al. (2017). Greenhouse gas fluxes and their cumulative response to inter-annual variability and experimental manipulation of the water table position in a boreal fen. Global Change Biol. 23, 2428–2440. doi:10.1111/gcb.13612
R Core Development Team (2017). R: a language and environment for statistical computing. Vienna, Austria: R Foundation for Statistical Computing.
Rupp, D. R., Kane, E. S., Dieleman, C., Keller, J. K., and Turetsky, M. R. (2019). Plant functional group effects on peat carbon cycling in a boreal rich fen. Biogeochemistry 144, 305–327. doi:10.1007/s10533-019-00590-5
Stedmon, C. A., and Bro, R. (2008). Characterizing dissolved organic matter fluorescence with parallel factor analysis: a tutorial. Limnol. Oceanogr. 6 (11), 572–579. doi:10.4319/lom.2008.6.572
Stewart, B. C., Kunkel, K. E., Stevens, L. E., Sun, L., and Walsch, J. E. (2013). NOAA technical report NESDIS 142-4. Regional climate trends and scenarios for the United States national climate assessment. Available at: www.nesdis.noaa.gov/content/technical-reports (Accessed January, 2021).
Strack, M., Waddington, J. M., Bourbonniere, R. A., Buckton, E. L., Shaw, K., Whittington, P., et al. (2008). Effect of water table drawdown on peatland dissolved organic carbon export and dynamics. Hydrol. Process. 22, 3373–3385. doi:10.1002/hyp.6931
Strack, M., Waddington, J. M., Lucchese, M. C., and Cagampan, J. P. (2009). Moisture controls on CO2 exchange in a Sphagnum-dominated peatland: results from an extreme drought field Experiment. Ecohydrology 2, 454–461. doi:10.1002/eco.68
Strack, M., and Waddington, J. M. (2007). Response of peatland carbon dioxide and methane fluxes to a water table drawdown experiment. Global Biogeochem. Cycles 21, GB1007. doi:10.1029/2006GB002715
Stubbins, A., Lapierre, J. F., Berggren, M., Prairie, Y. T., Dittmar, T., and del Giorgio, P. A. (2014). What’s in an EEM? Molecular signatures associated with dissolved organic fluorescence in boreal Canada. Environ. Sci. Technol. 48, 10598–10606. doi:10.1021/es502086e
Tfaily, M. M., Wilson, R. M., Cooper, W. T., Kostka, J. E., Hanson, P., and Chanton, J. P. (2018). Vertical stratification of peat pore water dissolved organic matter composition in a peat bog in northern Minnesota. J. Geophys. Res.: Biogeosciences 123, 479–494. doi:10.1002/2017JG004007
Turetsky, M. R., Kotowska, A., Bubier, J., Dise, N. B., Crill, P., Hornibrook, E. R., et al. (2014). A synthesis of methane emissions from 71 northern, temperate, and subtropical wetlands. Global Change Biol. 20 (7), 2183–2197. doi:10.1111/gcb.12580
Turetsky, M. R., Treat, C. C., Waldrop, M. P., Waddington, J. M., Harden, J. W., and McGuire, A. D. (2008). Short-term response of methane fluxes and methanogen activity to water table and soil warming manipulations in an Alaskan peatland. J. Geophys. Res. 113, G00A10. doi:10.1029/2007jg000496
Veverica, T. J., Kane, E. S., Marcarelli, A. M., and Green, S. A. (2016). Ionic liquid extraction unveils previously occluded humic-bound iron in peat soil pore water. Soil Sci. Soc. Am. J. 80 (3), 771–782. doi:10.2136/sssaj2015.10.0377
Vitt, D. H., Halsey, L. A., Bauer, I. E., and Campbell, C. (2000). Spatial and temporal trends of carbon sequestration in peatlands of continental western Canada through the Holocene, Can. J. Earth Sci. 37, 683–693. doi:10.1139/cjes-37-5-683
Weltzin, J., Bridgham, S., Pastor, J., Chen, J. M., and Harth, C. (2003). Potential effects of warming and drying on peatland plant community composition. Global Change Biol. 9 (2), 141–151. doi:10.1046/j.1365-2486.2003.00571.x
Wilske, C., Herzsprung, P., Lechtenfeld, O. J., Kamjunke, N., and von Tumpling, W. (2020). Photochemically induced changes of dissolved organic matter in a humic-rich and forested stream. Water 12 (2), 331. doi:10.3390/w12020331
Wyatt, K. H., Turetsky, M. R., Rober, A. R., Giroldo, D., Kane, E. S., and Stevenson, R. J. (2012). Contributions of algae to GPP and DOC production in an Alaskan fen: Effects of historical water table manipulations on ecosystem responses to a natural flood. Oecologia 169 (3), 821–832. doi:10.1007/s00442-011-2233-4
Wyatt, K. H., and Rober, A. R. (2020). Warming enhances the stimulatory effect of algal exudates on dissolved organic carbon decomposition. Freshw. Biol. 65, 1288–1297. doi:10.1111/fwb.13390
Wyatt, K. H., Seballos, R. C., Shoemaker, M. N., Brown, S. P., Chandra, S., Kuehn, K. A., et al. (2019). Resource constraints highlight complex microbial interactions during lake biofilm development. J. Ecol. 107, 2737–2746. doi:10.1111/1365-2745.13223
Wyatt, K. H., and Turetsky, M. R. (2015). Algae alleviate carbon limitation of heterotrophic bacteria in a boreal peatland. J. Ecol. 103, 1165–1171. doi:10.1111/1365-2745.12455
Zsolnay, A., Baigar, E., Jimenez, M., Steinweg, B., and Saccomandi, F. (1999). Differentiating with fluorescence spectroscopy the sources of dissolved organic matter in soils subjected to drying. Chemosphere 38, 45–50. doi:10.1016/s0045-6535(98)00166-0
Keywords: boreal, peat, climate change, carbon dioxide, dissolved organic matter, DOM, spectroscopy, algae
Citation: Kane ES, Dieleman CM, Rupp D, Wyatt KH, Rober AR and Turetsky MR (2021) Consequences of Increased Variation in Peatland Hydrology for Carbon Storage: Legacy Effects of Drought and Flood in a Boreal Fen Ecosystem. Front. Earth Sci. 8:577746. doi: 10.3389/feart.2020.577746
Received: 29 June 2020; Accepted: 07 December 2020;
Published: 03 February 2021.
Edited by:
Jianghua Wu, Memorial University of Newfoundland, CanadaReviewed by:
Jason Fellman, University of Alaska Southeast, United StatesBenjamin N. Sulman, Oak Ridge National Laboratory (DOE), United States
Copyright © 2021 Kane, Dieleman, Rupp, Wyatt, Rober and Turetsky. This is an open-access article distributed under the terms of the Creative Commons Attribution License (CC BY). The use, distribution or reproduction in other forums is permitted, provided the original author(s) and the copyright owner(s) are credited and that the original publication in this journal is cited, in accordance with accepted academic practice. No use, distribution or reproduction is permitted which does not comply with these terms.
*Correspondence: Evan S. Kane, ZXNrYW5lQG10dS5lZHU=; Catherine M. Dieleman, Y2RpZWxlbWFAdW9ndWVscGguY2E=
†These authors contributed equally to this manuscript