- 1Department of Physical and Geological Sciences, Faculty of Science, Universiti Brunei Darussalam, Brunei, Darussalam
- 2Department of Geological Engineering, Faculty of Engineering, Universitas Islam Riau, Pekanbaru, Indonesia
- 3Southeast Asia Disaster Prevention Research Initiative, Universiti Kebangsaan Malaysia, Bandar Baru Bangi, Malaysia
- 4Indian Institute of Technology, Kanpur, India
The thrust top intermontane basins that are located to the east of the Hazara-Kashmir syntaxis (HKS) in NW Himalaya are ∼NW–SE oriented and run parallel to the strike of the major Himalayan thrusts, which is in contrast to the similar basins that lie at the west of HKS (e.g., Peshawar basin). Although these basins are similar in age yet their structural orientation differs, which has not been previously investigated in much details. Therefore, here we investigate the structural details using the 30m shuttle radar topography, seismicity, earthquake centroid moment tensors solutions, previously published works, and geological fieldwork at Anantnag, Kashmir, where bedrock geology and Plio-Pleistocene to Holocene sediments are mapped. The three tectonic model for the formation of Kashmir basin are investigated and new data are produced to refine the existing knowledge on the formation of Kashmir and Peshawar basins. Our results establish that Kashmir basin is definitely neither a pull-apart basin nor a rift basin, and we reinforce that the basin can be formed in a piggyback structural style. We demonstrate that intermontane basins on either side of the HKS have rotated during their evolutionary journey. The estimated >45° clockwise rotation of Kashmir basin is in contract to the <45° anticlockwise rotation of the Peshawar basin, and this rotation coincides with the emergence of the >120 km long left-lateral strike-slip fault, the Jhelum fault (JF), which has dominantly left-lateral strike-slip movement at north, and oblique in south. We show that the JF largely controls the formation rotation and the present configuration of regions on either side of the HKS. The published paleomagnetic data supports our results and show that basin formation, lateral extent, geometry, and rotation are controlled by faulting where the emergence of the main boundary thrust fault system seems to have largely controlled the formation and lateral extent of the Kashmir basin, while as the JF has contributed toward the translation, rotation and present structural configuration of the intermontane basins in the region.
Introduction
Syntaxes are some of the most eye catching topographic feature in the Tethyan collision system because these regions mark the abrupt curling of orogenic trends, which are observable on regional maps and includes turning of structures like sutures, faults, folds, etc. (Wadia, 1931; Butler, 2019). Such distinctive topographic and structural features are well preserved in many orogens, and it forms a very peculiar feature of the >2,400 km long Himalayan arc (Figure 1), which has evolved since the initiation of the suturing, and collision between India and Eurasia (Yin and Harrison, 2000). One such example is the Hazara-Kashmir syntaxis (HKS), which is located in Pakistan and defines a strongly folded pattern of the main boundary thrust (MBT, Figure 1). The equally striking observation is the location and orientation of the intermontane basins on either side of the structure, which includes the Quaternary sediment filled Peshawar and Kashmir intermontane basins (Figure 1). These two basins preserve distinctive and tectonically controlled developmental history (Burbank and Johnson, 1982; Burbank and Reynolds, 1984; Bossart et al., 1990). The relatively older Kashmir basin is ∼NW–SE oriented, which is in contract to the NE–SW trend of the relatively younger Peshawar basin, and this change coincides with the existence of the Jhelum fault (JF) at the HKS. The sedimentary history of the basin formation suggests deposition in fluvial, glacial and lacustrine conditions during the last stage of the India–Asia collision, which indicate that both basins share a similar but distinctive sedimentary and tectonic history (Burbank and Johnson, 1983; Burbank and Reynolds, 1984). However, the details on the formation of HKS remains elusive (Bossart et al., 1988; Bossart et al., 1990; Butler, 2019), and the lateral extent, evolution, and apparent rotation of the basins on its either side also remain unresolved (e.g., Burbank and Johnson, 1982; Burbank and Reynolds, 1984; Agrawal et al., 1989; Burbank et al., 1996; Shah, 2013; Shah and Malik, 2017; Shah et al., 2018). Therefore, here we produce a new synthesis to fill the gap. This has been achieved on three major fronts: firstly, we have reviewed all of the major works that have produced data and tectonic models on the formation of the HKS and the Kashmir and Peshawar basins (Bhatt, 1975; Burbank and Johnson, 1982; Burbank and Johnson, 1983; Burbank and Reynolds, 1984; Nakata, 1989; Burbank et al., 1996; Malik and Nakata, 2003; Taylor and Yin, 2009; Shah, 2013; Alam et al., 2015; Alam et al., 2016; Shah, 2016a; Alam et al., 2017; Shah and Malik, 2017; Shah et al., 2017; Shah, 2018; Shellnutt, 2018). Secondly, we have used shutter radar topography to map the structural evidence of faulting in the Jhelum region (Figure 1). The field data are acquired in part of south Kashmir (Figure 1) to map the evidence of faulting and the geology of basement rocks onto which the Neogene-Holocene sediments have accumulated since ∼4 Ma (Burbank and Reynolds, 1984). The instrumented seismological data are used to understand the type of earthquakes that have occurred in the region, and these were examined and compared with the previous and newly mapped faults. Finally, the paleomagnetic data from Bossart et al. (1988, 1990) are used to supplement our data. The results show that JF is a major oblique fault with left-lateral strike-slip movement along with thrusting, and the formation of HKS is largely controlled by the JF. We also show that regions on either side of the HKS have rotated, and the rotation corresponds with the emergence of the JF, and therefore we demonstrate that JF is the major structural ramp that has played a key role in the formation of HKS and it also controls the lateral extend, and rotation of the Peshawar and Kashmir basins.
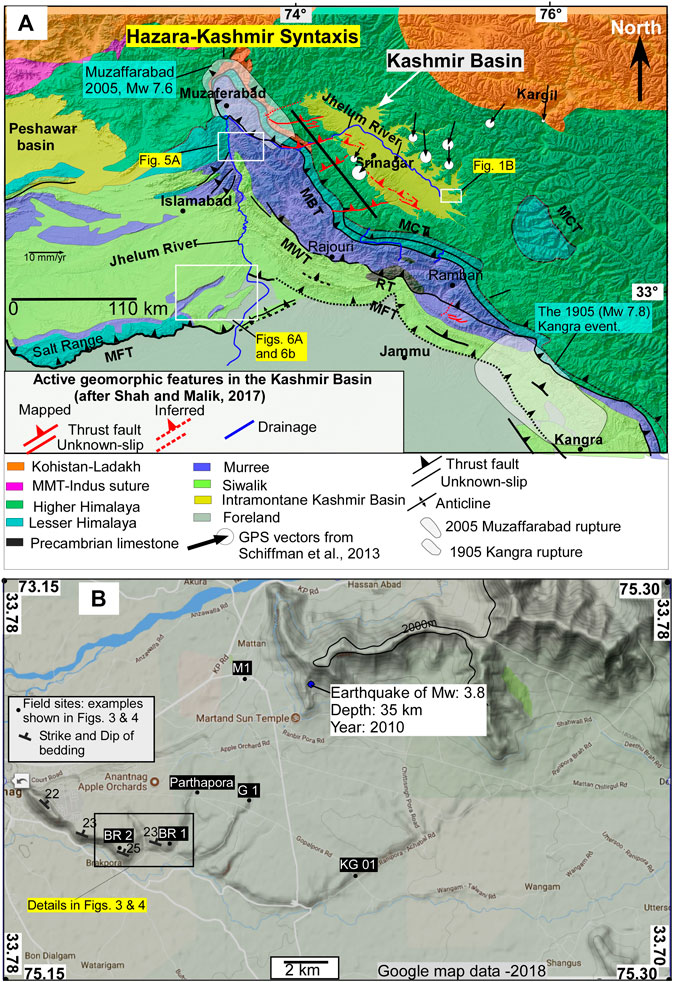
FIGURE 1. Location map of the Kashmir and Peshawar basins (A) with traces of major geological structures and rock units in NW Himalaya. The GPS velocity vectors are also plotted on the map to show the active plate convergence in the Kashmir region (after Vassallo et al., 2015; Shah and Malik, 2017). (B) The field sites are shown on the Google terrain map along with the information on strike and dip of geological units that are exposed at Anantnag, Kashmir. The details of two sites (BR-1 and BR-2) are shown in Figures 3,4. MBT, main boundary thrust; MCT, main central thrust; MFT, main frontal thrust; MWT, Medlicott –Wadia thrust; RF, Riasi fault.
Geological Setting
The HKS (Figure 1A) has attracted attention of many researchers because it typifies a distinct and quite remarkable topographic expression that cannot be missed by a keen observer. It is the place that folds the significant portion of the fold and thrust belt of Indian-Eurasia collision zone, and thus represents the changes in the tectonic vergence of major structures with a notable looping of the MBT Zone (Butler, 2019). The most detailed structural and stratigraphic data in the region are from Bossart et al. (1988), Bossart et al. (1990), Greco and Spencer (1993), and Critelli and Garzanti (1994). The new data are missing, and that is, primarily because the region is the de facto border between India and Pakistan, which has recently remained in political turmoil, and that is, why it is difficult to access the region (Shah et al., 2018; Butler, 2019). The region is tectonically active (Figure 2) and recently one of the major earthquake disasters occurred in the region because the level of unpreparedness to tackle medium to large magnitude earthquake is large (Shah et al., 2018; Bilham, 2019).
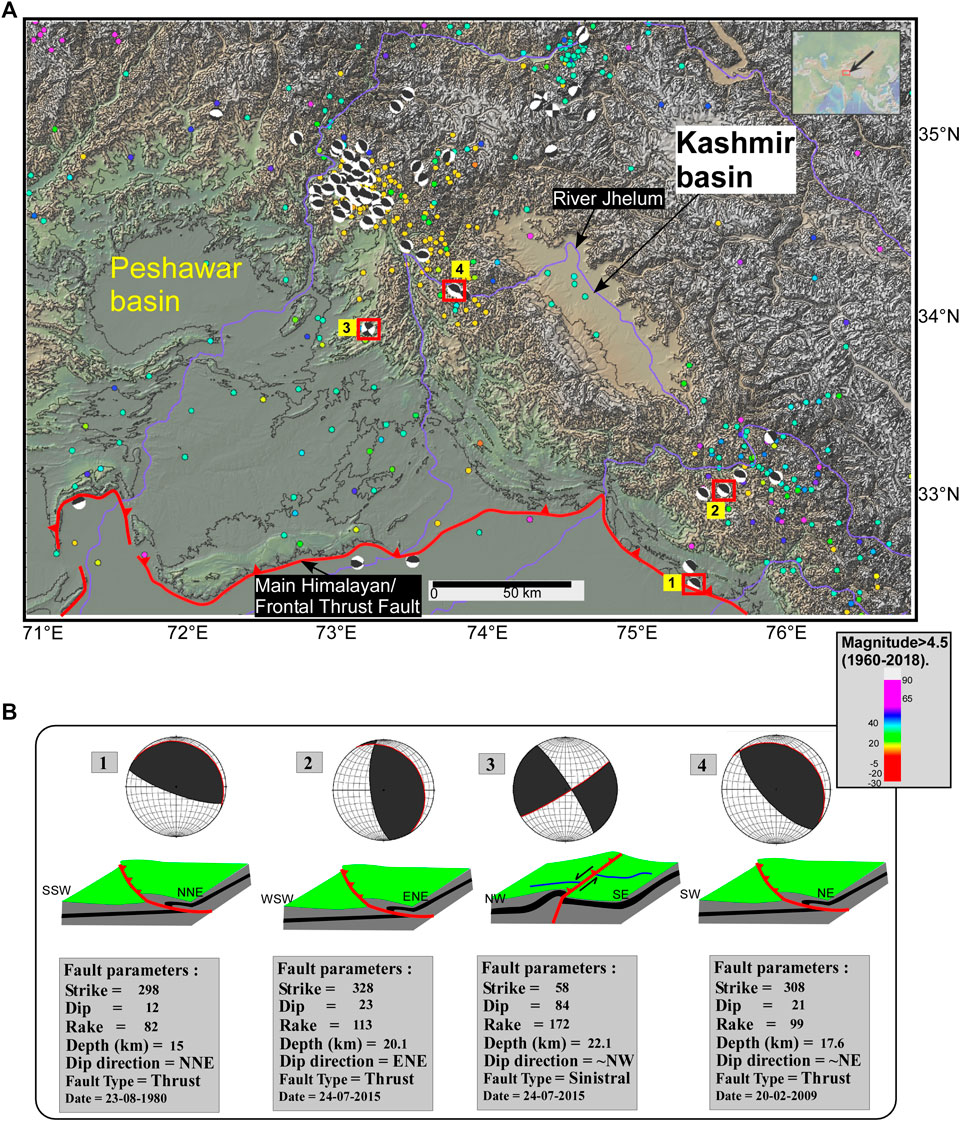
FIGURE 2. (A) Regional topographic map with contours shows the intermontane basins (Kashmir and Peshawar) in NW Himalaya. Note the relatively higher elevation of Kashmir basin relative to Peshawar basin. The trace of the Himalayan megathrust fault (the Main Himalayan/Frontal Thrust) is shown in red line with teeth toward the hanging wall block. The colored dots are earthquake epicenters with a magnitude of >4.5 (data are from 1960 to 2018). The earthquake moment tensor solutions, shown as beach balls, are also plotted, and these are clustered around the two corners of the Kashmir basin, which are mainly related to the 2005 Kashmir (in NW) and 1905 Kangra (in SE) events. (B) Shows the selected events that are used and correlated with the active faults in the region. Figure was made with data from GeoMapApp (www.geomapapp.org)/CC BY/CC BY (Ryan, 2009).
The previous understanding on the structural configuration of the HKS suggests an overall asymmetrical antiformal geometry with a series of overlapping thrust sheets that contain Precambrian, Paleozoic, and Mesozoic formations over Tertiary Murree formation (Bossart et al., 1988; Butler, 2019). A number of intermontane basins are located on either side of the HKS, which includes the Kashmir and Peshawar basins, which are some of the most striking geomorphic features in the NW Himalayan. The oval-shaped Kashmir valley is a large ∼NW–SE trending intermontane basin in NW Himalayas. It is bounded by the Pir Panjal volcanic in southwest and Tethys Oceanic rocks in the northeast (Figure 1). The Pir Panjal rocks, known as Panjal Traps, are of Early Permian (290 Ma) and are the largest outcropping of volcanic (basaltic, andesitic, and silicic) in the Himalaya. These rocks have formed much earlier than the Himalayan orogeny, and are usually associated with the Late Palaeozoic break-up of Gondwana land (Shellnutt, 2018). The Higher Himalayan rock sequence, that is, exposed in much of the Kashmir region is mainly composed of Mesozoic limestone units (Figure 1A). The basement rock is subsequently folded and faulted during the collision of India with Eurasia (Yin and Harrison, 2000). These rocks are mostly composed of Triassic limestone units, which have also been intruded by younger episodes of Panjal volcanic. At Guryal Ravine and Chandanwadi, Kashmir, the Triassic carbonates ride over the Panjal Traps and the contact is marked by a thrust fault (Shellnutt, 2018). The presence of pillow basalt (near Awantipora) clearly suggests that some portions of Panjal Trap volcanics erupted underwater. The basement rocks are now overlain by a thick blanket of Plio-Pleistocene to Holocene sediments that have formed in fluvial, glacial, and lacustrine environments (Bhatt, 1976; Burbank and Johnson, 1982). The geochemistry of these sediments provides evidence that the source lies in the nearby mountains, which are mainly composed of Panjal Traps, and Mesozoic basement (carbonates).
The Kashmir basin is ∼0–4 Ma old (Burbank and Johnson, 1983), which means it has formed very recently during the later stages of tectonic collision (Yin and Harrison, 2000). The formation of fault systems greatly contributed to the development of the basin (Shah, 2013; Shah, 2016b; Shah, 2016c), and presently the main Himalayan thrust (MHT) fault is tectonically the most active fault and absorbs ∼half of the ongoing convergence between India and Tibet (Schiffman et al., 2013). The latest instrumental earthquake records show that a major earthquake occurred in 2005 at the western Kashmir (Azad Kashmir, Pakistan) and resulted in liquefaction at Jammu, in the Indian portion of the Kashmir basin (Malik et al., 2007). The quake ruptured a portion of the previously mapped Balakot–Bagh fault (Pathier et al., 2006) that runs parallel to the strike of the Kashmir basin (Figure 1A) (Shah, 2013; Shah and Malik, 2017).
The MHT fault system runs under the Kashmir basin, and a series of major Himalayan fault ramps root from it (Figure 1). Kashmir basin rides on one of these ramps, and the slip-on these faults should have caused the formation of Kashmir basin (Burbank and Johnson, 1982). Subsequently, the basin received sediments from the rising mountains that surround it. The presence of a young fault system, the Kashmir basin fault/Balapor fault, which pierces through the Kashmir basin sediments, and tilts it further toward SE (Shah, 2013; Shah, 2016a) suggests a typical out-of-sequence thrusting in NW Himalaya, and its continuous evolution and development. The nearby Peshawar basin is strikingly similar but oriented differently with a relatively younger geological history (Burbank and Johnson, 1983; Burbank and Reynolds, 1984), and much thinner sediment accumulation (∼300 m) as compared to Kashmir (∼1,300 m).
Methodology
Field Data
We have acquired new field data from Anantnag, Kashmir (Figures 3, 4), however because of the extreme political uncertainty in Jammu and Kashmir, the Kashmir valley was mostly turbulent during our field session in June 2018. Therefore, our fieldwork in and around Kashmir basin was totally hampered, and limited to a few nearby places in the Anantnag town (Figure 4). However, it should be noted that fieldwork is not an essential part of our thesis objectives. The standard field techniques were used to collect geological evidence, and subsequently, the structural details of geological outcrops were recorded (Figures 3, 4). It involves looking for evidence of active faulting in the Karewa sediment-filled areas. We visited a total of nine sites but the recording of structural details was only possible at four locations (Figure 2B) because these sites expose basement outcrops. The other sites only expose Quaternary sediments and we examined the evidence of primary sedimentary structures, soft-sediment deformation, and fossils. The sites that expose basement rocks are used to map the structural parameters, which includes lithological contacts, dip and dip direction of beds and evidence for faulting.
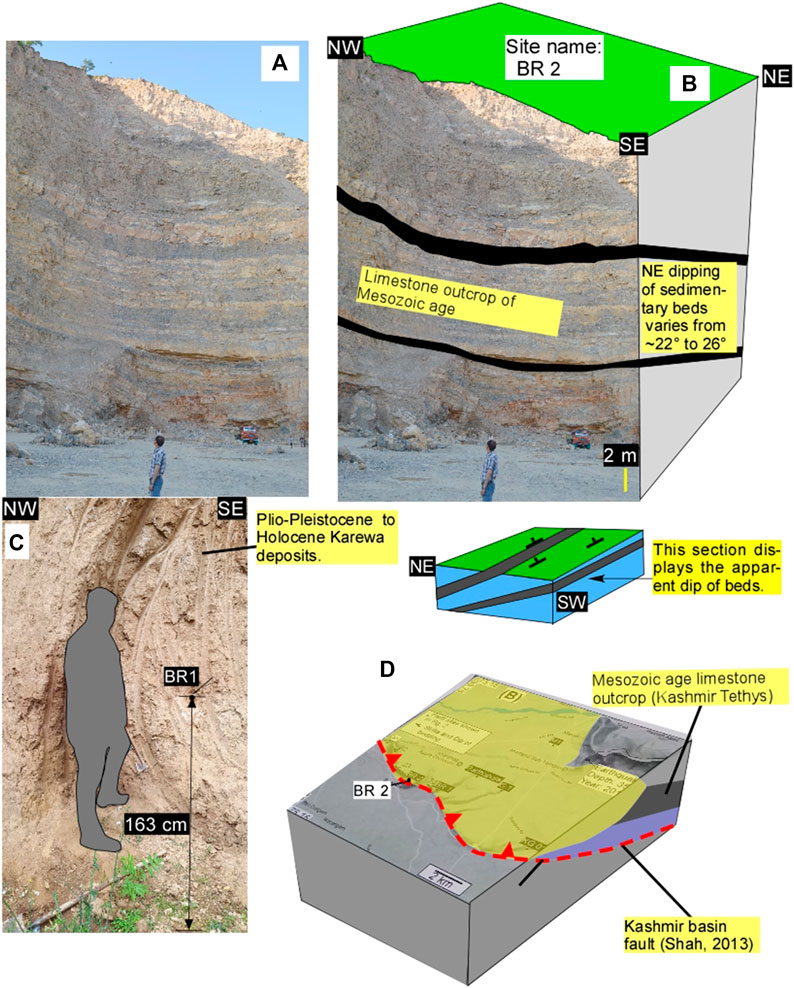
FIGURE 3. The field evidence of the undeformed Mesozoic carbonate basement rocks that are exposed at a quarry section in Anantnag, Kashmir (location in Figure 1) These rocks belong to Kashmir Tethys. The un-interpreted (A) and interpreted (B) field photograph shows the undeformed but tilted Mesozoic carbonate rocks that form the basement on which most of the Kashmir basin resides. The basin is filled with Plio-Pleistocene Karewa deposits (C and D). The exposure of the basement rocks at this location is related to the active faulting (Shah, 2013). The quarry section exposes the apparent dip of the rock units.
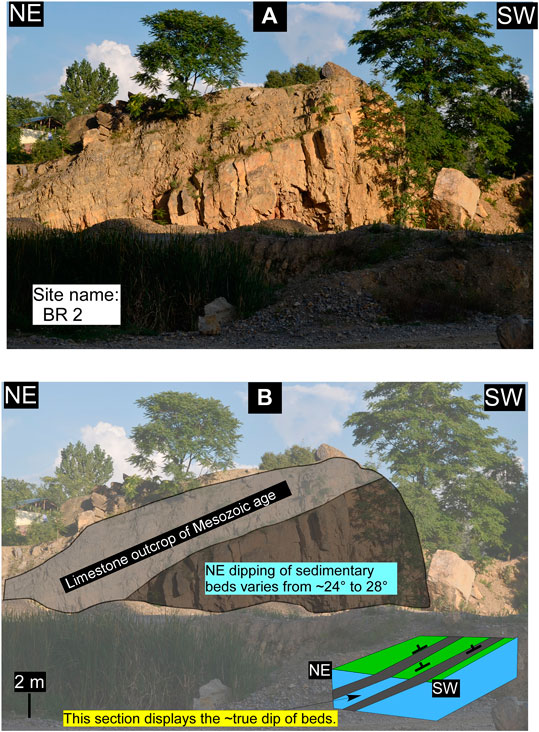
FIGURE 4. The field evidence shows the Kashmir Tethys (Mesozoic carbonate) basement rocks that are exposed at a quarry section in Anantnag, Kashmir (location in Figure 1). The un-interpreted (A) and interpreted (B) field photographs show a vertical section that exposes the true dip of the tilted basement rocks.
Structural Mapping Using Satellite Data
Satellite derived topographic maps are the most powerful tools to map the large-scale geological structures and have been used extensively since 1977 (Tapponnier and Molnar, 1977). The shuttle radar topography provides a useful and freely available topographic data that are captured at 30 m horizontal resolution (Avouac et al., 2006; Pathier et al., 2006; Kaneda et al., 2008) are particularly suitable in areas that are difficult to access because of complex terrain, or political conflicts (Shah et al., 2019). We used standard mapping techniques to map the first order geomorphic features such as displaced fluvial/glacial terraces, topographic breaks, displaced river channels, triangular facets, bedding, and folded and faulted Holocene sedimentary deposits (Figures 5, 6). The tectonic geomorphological mapping was achieved by manually looking for changes in drainage, topography, geology, and geomorphology. The ridge crests (Shah, 2013; Shah and Malik, 2017) are useful indicators to delineate structural breaks, and often such breaks are related to active faulting. Similarly, the triangular facets, which are erosional features associated with river cutting, are usually indicative of active landforms, and it also helps in mapping of the bedding dip direction (Shah and Malik, 2017). The dip direction of bedding was routinely measured by the use of rule of “V,” which a competent structural technique (Shah et al., 2018). The cross-cutting relationship was used to constrain the relative ages of faulting, where Holocene (∼10,000 years) sediment filled basins provide a standard substratum to date the faulting events. The entire mapping exercise was done in ArcGIS software, which is a competent tool to handle multilayer data and especially designed to process and manage large quantity of data.
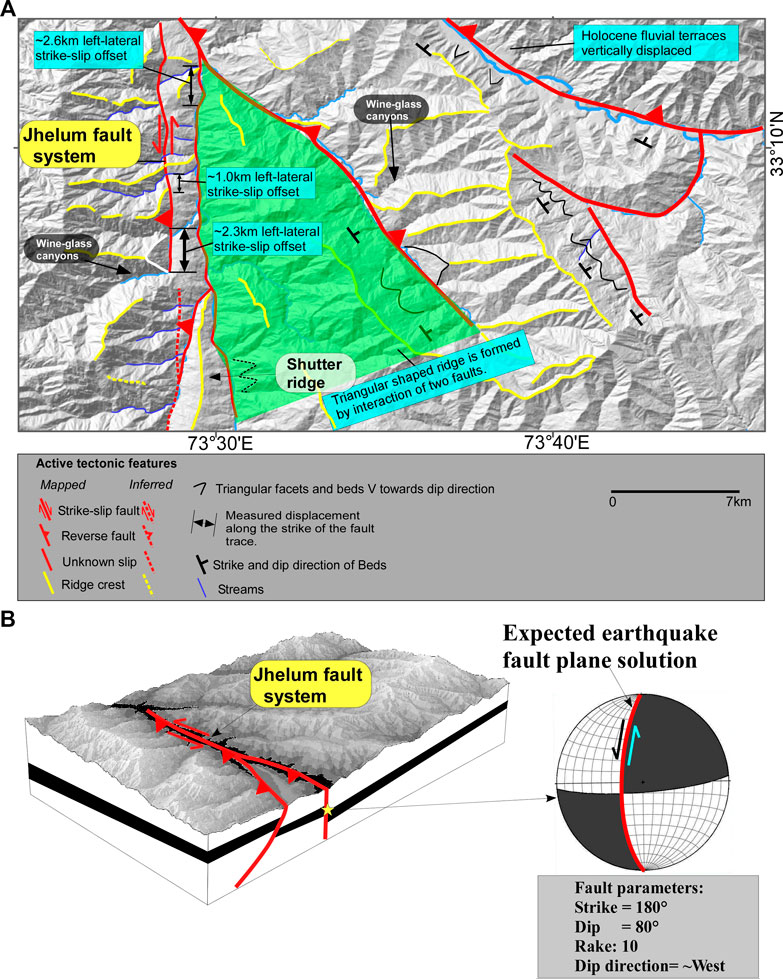
FIGURE 5. (A) Shows the interpreted shuttle radar topography (30 m resolution) of the upper portion of the Jhelum fault (see location in Figure 1A) with evidence of active faulting. The prominent structural discontinuity with ∼NS strike is mapped as a left-lateral strike-slip fault as the ridges and streams are displaced laterally for a few meters to >2 km. (B) 3D view of the region that shows active faults and the potential earthquake with an estimated centroid moment tensor solution. The structural details used for centroid moment tensor solution are derived from GeoMapApp (www.geomapapp.org)/CC BY/CC BY (Ryan, 2009).
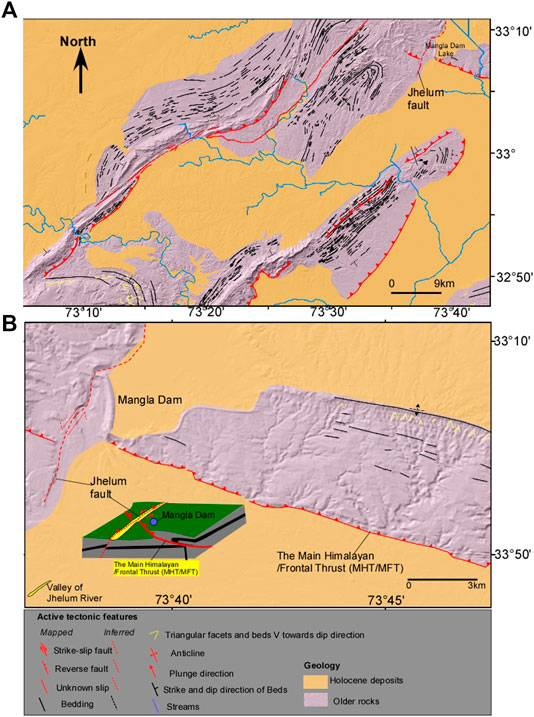
FIGURE 6. the interpreted shuttle radar topography (30 m resolution) of the middle (A) and lower (B) portions of the Jhelum fault (JF) system (see location in Figure 1) with evidence of active faulting. Note the Main Himalayan/Frontal Thrust is cut by the JF, which clearly indicates active faulting. The plunging folds mapped in the eastern portions of the JF are truncated at the emergence of the JF system, and this indicates that their deep structural roots are linked with the fault.
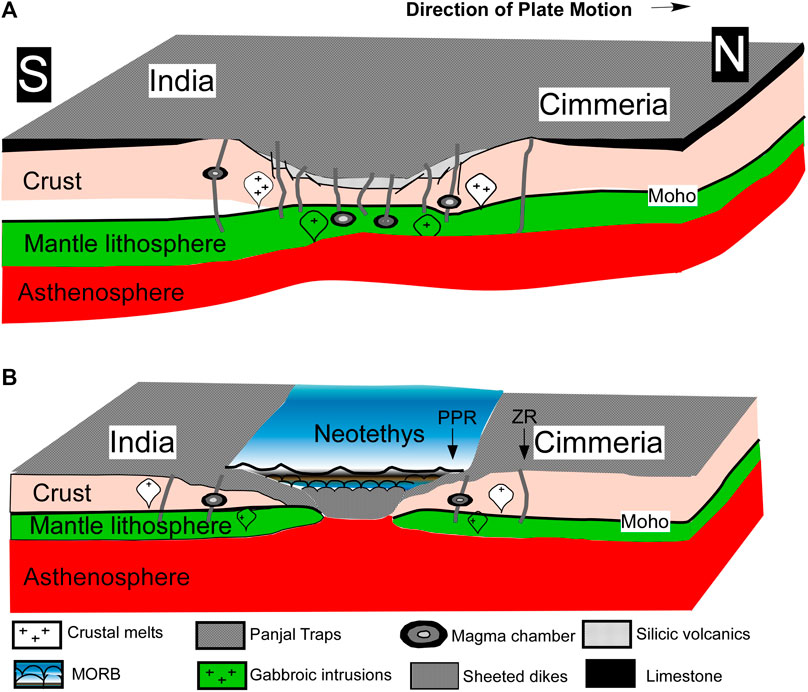
FIGURE 7. The formation of Panjal Traps is shown in two stages. (A) The rifting of India and Cimmeria resulted in the formation of normal faults, which at the later stage of rifting facilitated the eruption of Panjal Traps. (B) The opening of the Neotethys ocean occurred during the final stages of rifting, and later this ocean was closed during the subduction and subsequent collision of India with Tibet occurred sometime in Eocene (modified after Shellnutt, 2018).
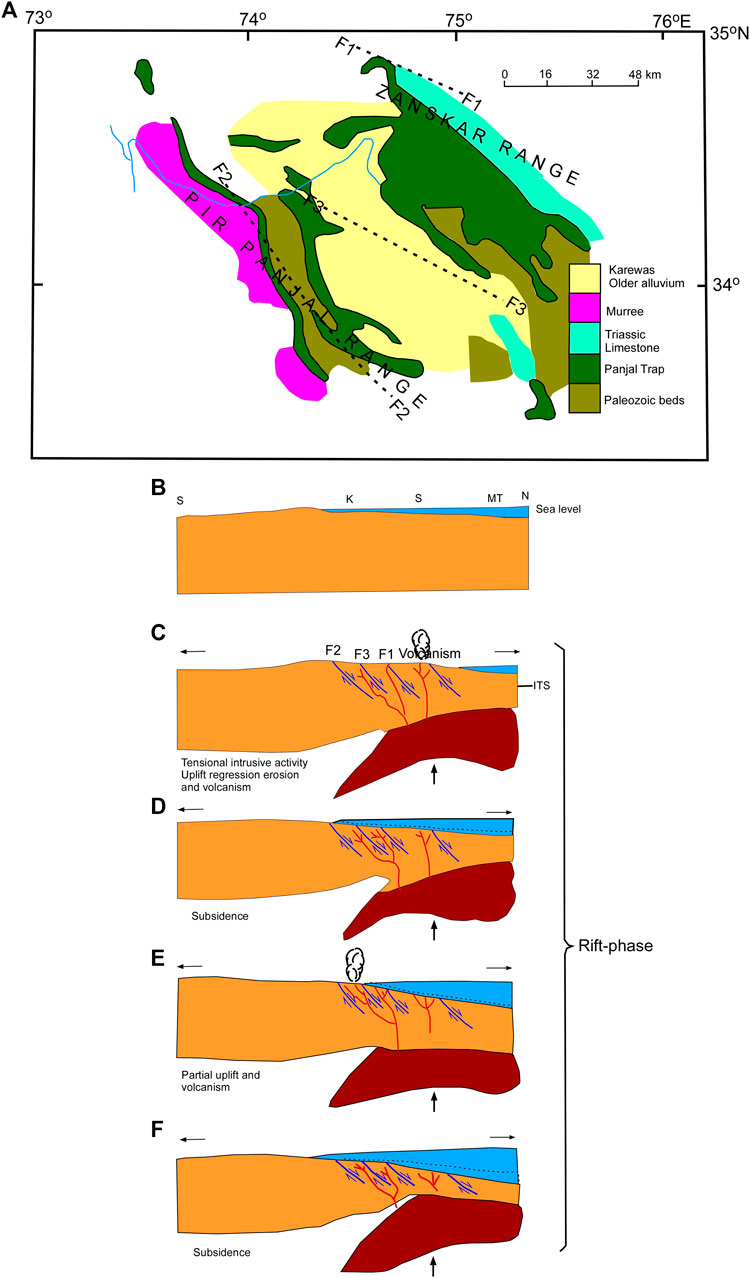
FIGURE 8. The Kashmir basin as a product of tectonic rifting requires at least two major faults to pierce through the basin, and that will form a typical horst and graben topography (A). The various stages of rifting and basin development are shown. The scenario before the basin development (B), and after the first episode of normal faulting and volcanism (C). The faulting continued (D) with basin development as a result of subsidence during rifting. The partial uplift and new episode of volcanism (E) was followed by subsidence (F), and basin development (after Bhat, 1982). ITS, Indus-Tsangpo Suture; K, Kashmir; MT, Main Tethyan basins; S, Spiti.
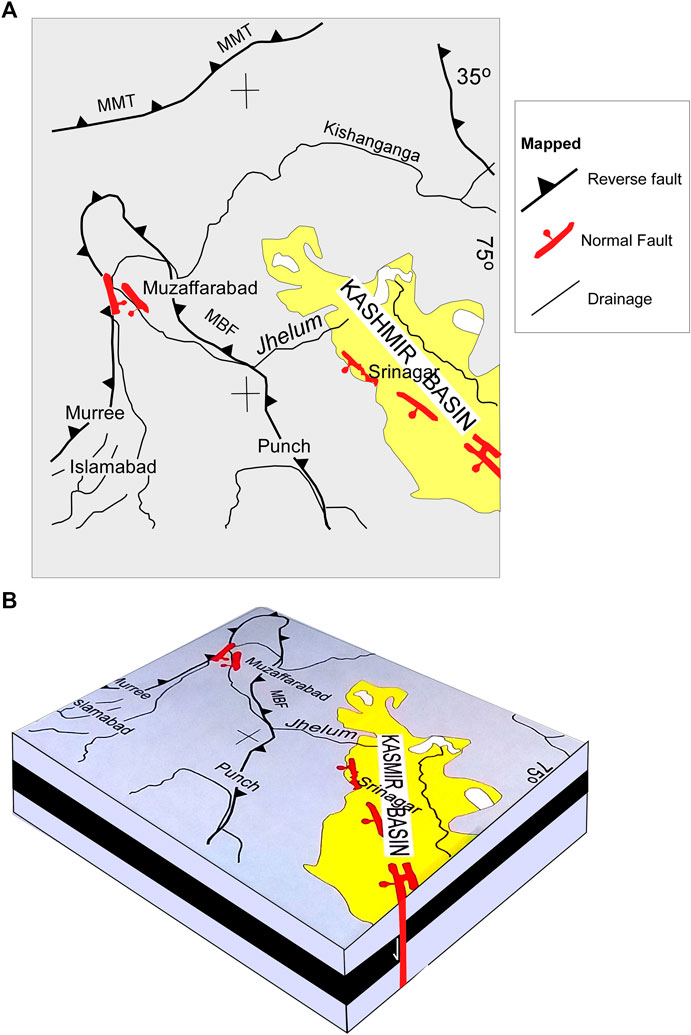
FIGURE 9. The only major normal fault (A) that has been reported earlier using satellite data in the Kashmir region is the ∼SW dipping normal fault (Yeats et al., 1992). It has the Kashmir basin on the footwall side (B), and it does not support the idea that Kashmir basin was formed by rifting process much earlier than the India–Eurasia collision. The normal fault of Yeats et al. (1992) is mapped as an active fault, and therefore, cannot be related to the rifting process that led to the formation of Kashmir basin according to Bhat (1982).
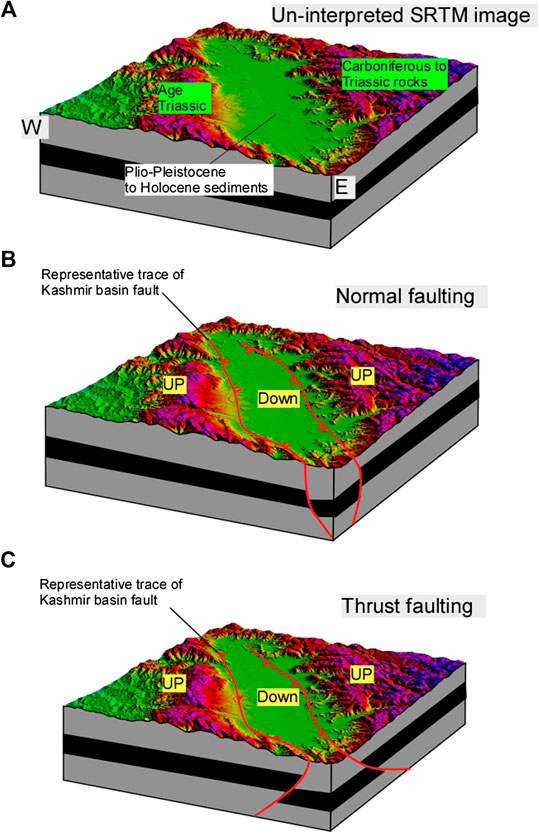
FIGURE 10. The un-interpreted shutter radar topography shows the extent of Kashmir basin (A), and the present topography where the basin is bounded by mountains on all sides. Broadly, the rift tectonic model suggests that such a topography requires at least two major normal faults (B), however, reverse faults can also form a similar topography (C).
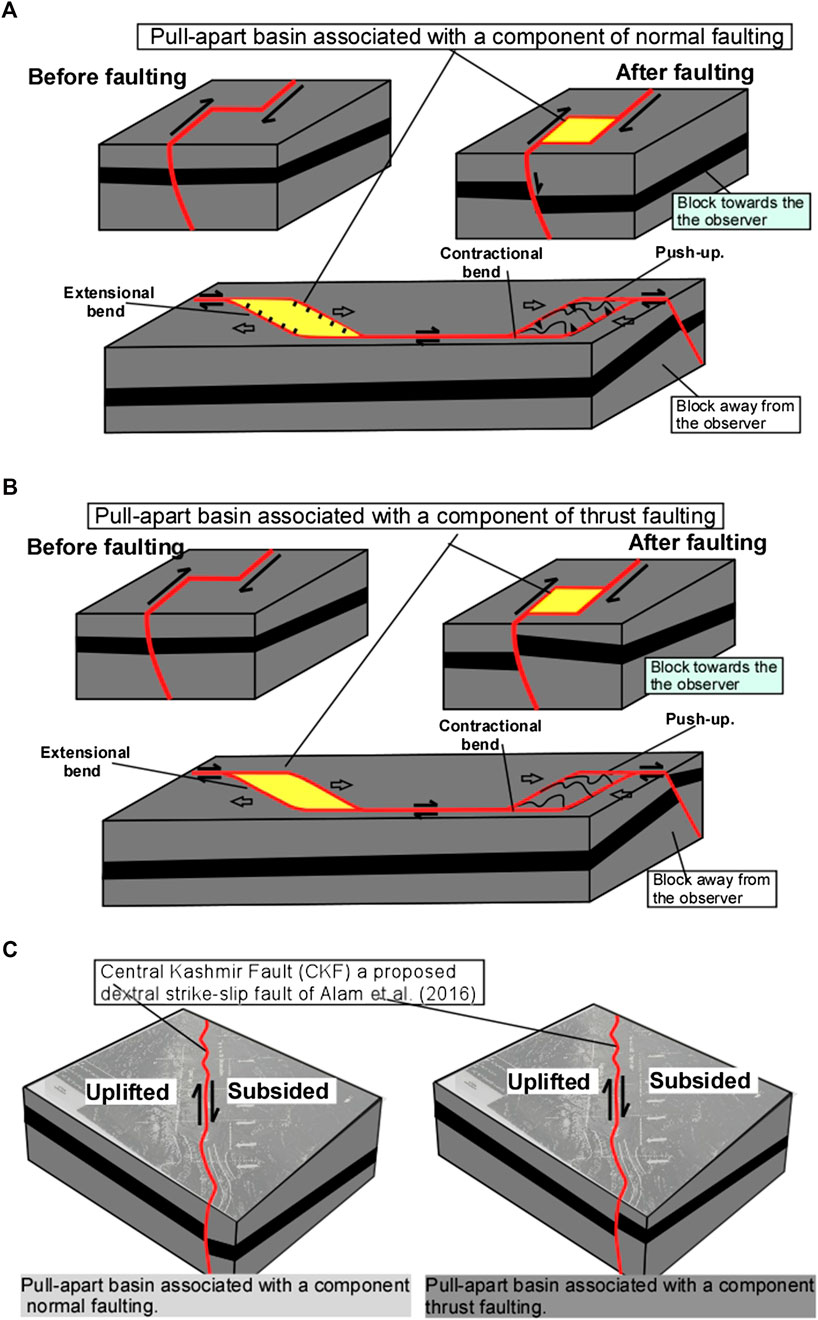
FIGURE 11. A series of cartoons are shown to demonstrate the major structures that are usually associated with a typical oblique strike-slip fault system. Strike-slip with a normal (A) or thrust (B) components are related to the drowned and uplifted topography (C) of the Kashmir basin (Shah, 2013) and what type of fault slip could be expected on the Central Kashmir fault (CKF), if it exists. Only a strike-slip fault with a normal component can make the present topography of the Kashmir basin.
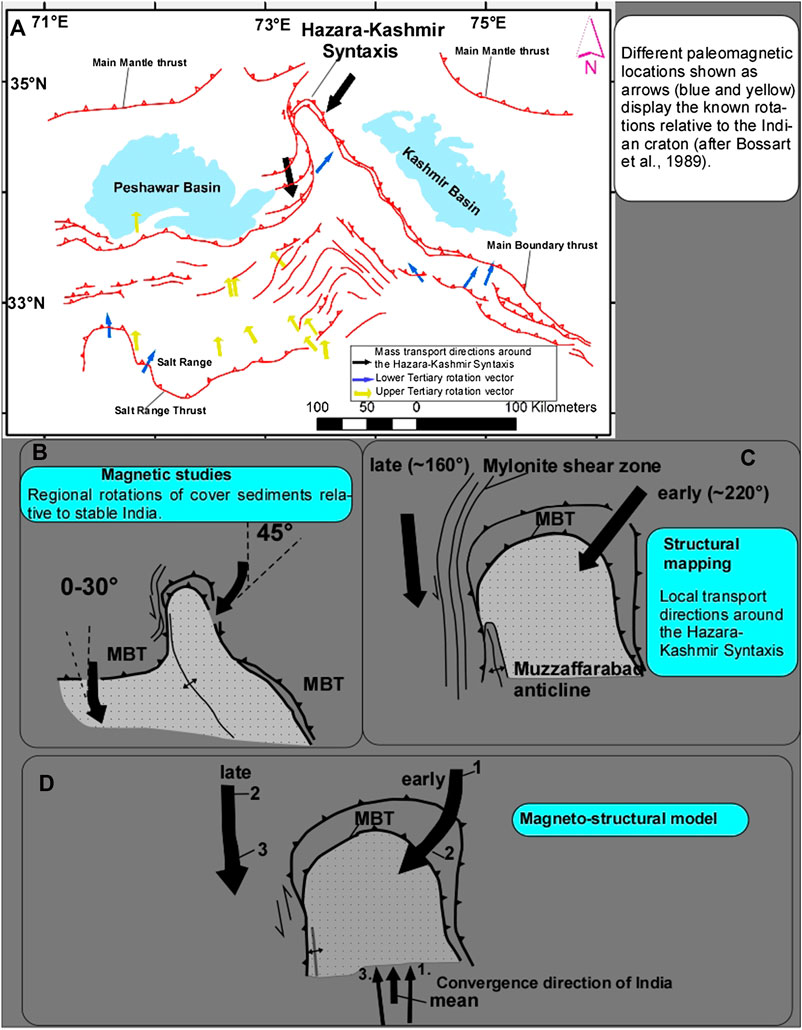
FIGURE 12. (A) Different paleomagnetic locations shown as arrows (blue and yellow) display the known rotations relative to the Indian craton (after Bossart et al., 1989). The clockwise, and anticlock rotations on either side of the Hazara-Kashmir syntaxis complement our model (Figure 13). (B–D) The magneto-structural model for the formation of the Hazara-Kashmir syntaxis are shown in three stages model (after Bossart et al., 1989; see Discussion for details).
Seismological and Paleomagnetic Data
The satellite based structural mapping was followed by the examination of seismological (Figure 3), structural, geological (e.g., Jackson and McKenzie, 1984; Nakata, 1989), and paleomagnetic data (Figures 7–12). The earthquake centroid moment tensor solutions from the open source GeoMap App. software (Ryan et al., 2009) are used to extract the earthquake parameters (dip, strike, rake), which are later compared with the mapped structures (Figure 2).
The earthquake hypocenters were obtained from the National Earthquake Information Center (NEIC), and it covers the temporal window from 1960 to 2018 with events of magnitude >4.5 (Figure 1). These data are plotted on the satellite image of the region to interpret and understand the distribution of earthquakes, and the pattern of active faulting. The earthquake CMT solutions are extracted from the GeoMap App. (Ryan, 2009) and it covers events from Jan 1976 until July 2019 (Figure 2). The selected events that were used for structural analysis are shown in Figure 1B. We matched the strike and rake values with our mapped faults, and used this information to create 3D illustrations to represent the brittle faulting pattern in the region, and its relationship with the regional plate convergence.
The expected earthquake moment tensor solutions are derived by using the strike of the mapped fault and we follow the right hand rule. For example, in Figure 5 we have mapped N–S strike, so the value has to be either 0° or 180°, we selected 180° because it is dipping west, and we interpreted that from the study of tectonic geomorphology where hanging wall block is up, and the fault become oblique westward. Therefore, we used the strike, and dip direction from our structural mapping, and the amount of dip and rake is obtained by selecting the closest CMTs event, which is three for the JF (Figure 2B). These parameters are then used in Faultkin, which is a competent structural geological application to use stereonet plots, beachballs, etc. (Marrett and Allmendinger, 1990). The expected CMT solutions are a powerful representation of the future earthquake event on a mapped fault because it will suggest the possible geometry of the future rupture zone on a fault.
We have used the best available paleomagnetic data (Bossart et al., 1988; Bossart et al., 1990) where the measurements from the weakly deformed southernmost section in the Jhelum valley are useful for our work because we aim to understand the primarily undeformed state of the Murree formation, and how it might have rotated on either side of the JF (Figure 12). These data are suitable for our tectonic interpretations where primary paleomagnetic directions are used to determine the relative rotations of the Murree formation across the HKS.
Review of the Existing Models
The previous geological literature shows that the formation of the Kashmir basin is broadly attributed to three tectonic models, which are related to a) the rifting of India and Cimmeria, b) the classic piggyback basin development during thrusting, and c) the pull-apart basin formation during the dextral strike-slip faulting. All these models are critically examined below.
Kashmir Basin as a Product of Continental Rifting
The earliest tectonic model for the formation of Kashmir basin is of Bhat (1982) and it shows that basin is formed during the rifting process, and it is bounded by two major horsts (Figure 8). The evidence of rifting is mainly attributed to the presence of Panjal Traps in Kashmir (Shellnutt, 2018). These volcanic rocks are of Early Permian (290 Ma) and are one of the largest outpourings of volcanics (basaltic, andesitic and silicic) in the Himalayas. The formation for Panjal Traps is linked with the breakup of Gondwana during the Late Palaeozoic (Figure 7) (Shellnutt, 2018). This means Bhat (1982) argues that the basin is much older and possibly formed during the rifting process some >290 Ma ago. However, the Kashmir basin is very young because it is filled with Plio-Pleistocene to Holocene sediments (Figure 1) and therefore, rifting cannot be the reason for the origin of Kashmir basin. Rifting of India from Cimmeria is argued to have caused the formation of several ∼E–W trending normal faulting, and later the rifting process had led to the opening of the Neotethys, and the formation of Kashmir basin (Figures 7, 8B–F). The closing of this ocean occurred when India drifted toward the Tibet, and collided with it and formed the Himalayas. The normal faults related to the rifting process, if exist, would have been reactivated as thrusts during the India–Tibet collisional phase. This is mainly because the orientation of collisional forces (∼N–S) favors the E–S strike and ∼north and south-dipping fault planes. This could mean that some of the faults in Kashmir Himalaya are basically normal faults, which are reactivated during the collision. Tectonic inversion could explain this but the model is irrelevant because it invokes rifting, which is against the structural configuration, and age of the Kashmir basin.
Furthermore, the presence of three major ∼NW–SE trending normal faults shown in Bhat (1982) have not been confirmed on the ground. The only major normal fault that has been reported earlier, using satellite data, in the region is the ∼SW dipping normal fault (Yeats et al., 1992) that has the Kashmir basin on its footwall (Figures 9A,B). This does not support the idea that the Kashmir basin is a valley that has formed through the rifting process much earlier than the initiation of India–Tibet collision. The normal fault of Yeats et al. (1992) is mapped as an active fault, and therefore, cannot be related to the formation of Kashmir basin because it pierces through the SW portion of the basin and is younger than the basin. Subsequent works have established that a major ∼NE dipping thrust fault system cuts through the Kashmir basin (Shah, 2013) and tilts it toward NE (Shah, 2013; Shah, 2016c; Shah and Malik, 2017). The existence of this fault system questions the rift model where major normal faulting is expected. Further, the ∼NW–SW termination of the basin cannot be explained by a rift-related earlier structures because why would rifting of large scale continents, India and Cimmeria (Shellnutt, 2018), only produce small scale normal faults, and why such fault systems have not been reported at both the plate margins of India (Figure 1) and Cimmeria? The fault movement chronology of the two major Himalayan thrust faults that bound the Kashmir basin to south (Figure 1) shows that the major displacement on the main central thrust (MCT) occurred at ∼22–20 Ma (DeCelles et al., 1998; Gavillot et al., 2016) and on the MBT at ∼11–10 Ma (Meigs et al., 1995; Gavillot et al., 2016) and at ∼5 Ma (Sangode and Kumar, 2003). None of the previous studies show that the major Himalayan thrust fault systems inherit older faults, and have recently flipped movement from normal to reverse. There is no evidence of large scale tectonic inversion at the plate margins of India and Tibet (Figure 1).
The sediments that have filled the Kashmir basin are ∼4 Ma old (Burbank and Johnson, 1983). These are dominantly affiliated to fluvioglacial and lacustrine environments and are known as Karewas. The Pliocene to Pleistocene Karewas are suggested to have resulted from the rising mountains that surround the Kashmir basin and are mainly composed of ∼290 Ma old volcanic sequences known as Panjal traps (Shellnutt, 2018) that have erupted underwater and pierced through the oceanic crust that once existed. The eruption of the Panjal Traps was initially within continental rift setting but eventually transitioned into the oceanic setting that turned into the seafloor spreading center (opening of Neotethys ocean) and formation of ribbon-like continent, Cimmeria. The Neotethys ocean was subsequently closed during the collision of India’s passive margin with the Eurasian plate since Eocene. The initiation of collision nucleated several ∼NE dipping thrusts, which are younging toward the foreland (Figure 1). This also resulted in the forward propagation of foreland basins, and this is when the Kashmir basin started to form (see below the Piggyback Basin Model section). Further, the rift model (Bhat, 1982) cannot explain why the Kashmir basin was formed by regional extension during the Himalayan orogeny, and what could cause such local scale extension and that too parallel to the regional thrusts. The existence of thrust parallel normal faulting is not common in collisional orogens (Shah, 2016a), the Kashmir Basin is peculiar because nothing similar to this basin occurs further south of the basin. What controls the extent of the basin needs a robust model (see refined Piggyback Basin Model below).
Piggyback Basin Model
The piggyback basin model of Burbank and Johnson (1982) is a well-accepted explanation for the formation of the Kashmir basin. It needs major thrust system(s) that should have the Kashmir basin on the hanging-wall portion (Figure 1). Such basins have formed throughout the collisional orogenesis (Yin and Harrison, 2000), and in NW Himalaya many such basins exist (Figure 1). The ∼0–4 Ma old sedimentation history of the Kashmir basin (Burbank and Johnson, 1982; Burbank and Johnson, 1983) supports the formation during the later stages of India–Eurasian collision. However, what controls the lateral extent and the orientation of piggyback basins in NW Himalaya remains elusive (Shah, 2015; Shah et al., 2017). The presence of ∼0–3 Ma old Peshawar basin at the west of the Kashmir basin indicates that these basins have developed simultaneously (Figures 1,2). Nevertheless, the ∼NW–SE orientation of Kashmir basin is in contrast to the ∼NE–SW trend of the Peshawar basin, and similar other basins in the region (Figure 2). The regional thrust faults (e.g., MCT, MBT, and MHT) verge ∼SW to the east of JF and these faults turn ∼90° at Tunda (Figures 1,2) and continue as ∼SE verging thrusts (Figure 1) west of the JF. This change in the orientation of tectonic transport on the Himalayan thrusts in NW Himalayan is a significant tectonic event (see the new synthesis below) that has largely shaped the present configuration of the structures, and landforms there. Therefore, although the piggyback model fits the structural configuration of the Kashmir basin it however cannot explain the lateral limits, dimensions, and rotation of the basins, and why Kashmir basin has received much thickener (∼1,300 m) sediment blanket than its neighbor the Peshawar basin (∼300 m). Furthermore, the existence of a new generation of active faults through the Kashmir basin has complicated the tectonics of the region (see details immediately below).
Is Balapur Fault a Reverse or a Normal Fault?
Shah (2012, 2013) demonstrated by using the tectonic geomorphological techniques that a major fault pierces through the southwestern margin of the Kashmir basin. A portion of the fault is mapped as a small out-of-sequence reverse fault by Shabir and Bhat (2012). The subsequent works have reinforced the existence of the fault (references herein), however, not a single convincing field evidence (Shah, 2016a; Shah, 2016b; Shah, 2016c) has been produced since its discovery to compliment or contradict its existence, and instead a number of well documented normal faults are reported in Kashmir, which are wrongly related to reverse faults (Shah, 2016a). Therefore, the field details of the fault largely remain unknown. This is mainly because the paleoseismological work (Kozhurin et al., 2006) has not been carried out on any portion of the fault due to the ongoing and perpetual political turmoil in the region (Shah et al., 2018). Although Meigs et al. (2010) argue that trenches were cut across a portion of the fault to study the details but that work has not been published except as an extended abstract. Nevertheless, a number previous studies (references herein) have used satellite images to map the evidence of active faults where past fault ruptures have displaced young geomorphic landforms (e.g., alluvial fans, river terraces, etc.) that range in age from 0–4 Ma (Burbank and Johnson, 1983).
The topographic expression of the basin indicates tilting toward NE, and the fieldwork at Anantnag in South Kashmir (Figures 3,4) has confirmed the ∼NE dip of the undeformed limestone bedrock sequence onto which the basin rides. The tilting suggests that the entire basin is standing on a structural ramp that dips NE, which is consistent with a major thrust fault that splays off on the main frontal thrust (MFT) (Shah, 2013; Shah, 2016b). Later on the Kashmir basin fault/Balapor fault has pierced through the basin, which has resulted in further tilting of the basin toward NE. Since the structural details about the slip on the Balapur fault are controversial therefore the fault is reinterpreted below (Figure 10). There are at least two possibilities to form the Kashmir basin by faulting: 1) if the basin bounding faults dip toward each other (NE and SW) that would fit a normal (Figure 10B) fault configuration, were downthrown block will be in the center and it will form a typical horst and graben structure, and 2) if the faults are reverse then it would also form a similar basin in the middle but the faults would dip differently (SW and NE; Figure 10C). The regional ∼SW dipping normal fault (Figure 9) has been mapped by Yeats et al. (1992), however, the overall tilting of the basin is toward ∼NE, which may conflict with the existence of such a normal fault. The listric geometry at depth could cause tilting on the footwall but the geometry of the Kashmir basin does not fit such a configuration. The previous mapping has shown that basin bounding faults are not observed in the basin, and the only reverse fault, the Balapur fault/Kashmir basin fault, pierces the basin, and could not be the reason for the development of the basin. These faults are young structures, and largely fit ∼NE dipping reverse faulting. Alternatively, if the fault is normal then it should dip SW.
The examination of regional earthquake CMT solutions (Figure 2) suggest the possibility of NE dipping reverse fault in the region (Shah, 2013). These data show that Holocene faulting occurs on ∼NW–SE trending reverse faults that dip either ∼NE or ∼SW, and these earthquakes events are the closest to the fault that runs under the Kashmir basin, and this suggests that reverse faulting is what one would expect in the region. Ideally, if this has to be true then the dip of such a fault system should be steeper than 50° as one would expect in the interior of the mountains where such a fault system will have to root from the Himalayan Frontal, and the expected structural geometry would produce steeper dip for the crustal ramp that will shoulder the Kashmir basin. However, the field evidence in Anantnag Kashmir where Precambrian limestone deposits are exposed shows that the beds are dipping <30° toward NE (Figures 3,4). This means that the fault system through Kashmir basin has not tilted the hanging wall block homogenous, which also suggests the slip along and across the fault zone has not been the same as would be expected in a typical fault zone. The previous fieldwork in frontal portions have produced data that shows that the basement rocks in Jammu are cut through by the active ∼45°–50° NE dipping Main Raisi thrust system (Gavillot et al., 2016) that places older rocks against the Holocene sediments, and also pierces through the younger sedimentary deposits, which suggests a similar ramp to run under Kashmir basin, and produce bedding data with a preferentially steeper dip values.
Serious Problems with Pull-Apart Model
Tectonic or gravity-induced faulting can form a typical pull-apart basin if strike-slip faults curve, step, or change strike (Figure 11A), and often during the development of such a fault system a variety of extensional and compressional zones form along the strike of the fault (Mann et al., 1983; Sylvester, 1988; Burchfiel and Stewart, 1966; Xu et al., 2014; Shah, 2018). The crustal extension is usually accommodated by the formation of normal faults, and compression by thrusting and folding (Figure 11), and if the strike-slip faulting is not pure then various types of secondary strike-slip faults will also form with a restricted orientation that should satisfy the regional or local convergence direction and stress orientation (Xu et al., 2014). If the Kashmir basin is a byproduct of the movement on the major strike-slip fault that runs ∼parallel to the ∼NW–SE orientation of the basin then the related secondary structures (faults, folds, etc.) must satisfy the geometrical constrains that are observed during such faulting (Figures 11A,B). However, such a typical association of strike-slip fault related structures completely contradict the pull-apart model of Alam et al. (2015) for the formation of the Kashmir basin (Shah, 2016b; Shah, 2018). Alam et al. (2015, 2017) argue that the Kashmir basin is a pull-apart outcome of a major active dextral-strike slip fault (Figures 11B,C), the Central Kashmir fault (CKF). But the critical evaluation of the evidence provided to support such a model does not prove it, and on the contrary it highlights the lack of geomorphic, geological and field evidence (Shah, 2016a; Shah, 2018). Shah (2018) argues that CKF does not exist. The criticism of this model has been discussed in a number of papers (Shah, 2016a; Shah, 2016b; Shah et al., 2017; Shah, 2018), and recently it has been endorsed by a recent paper by Bilham (2019) where the author has categorically objected to such a claim by writing a strong statement against it, and we quote “Alam et al. (2015) speculate that the Kashmir Valley represents a relict dextral pull-apart basin with an invisible dextral fault along its axis. There are several problems with this suggestion, not the least being the absence of strike-slip mechanisms in local earthquakes, and the absence of evidence for strike-slip faulting in the mountains beyond the ends of the valley. Shah (2016a) offers a litany of additional problems. In the absence of identifying a major strike-slip, the Riasi thrust system or a high-level thrust must host an oblique slip component.”
The lack of any major structural break at the NW and SE termination of the basin (Figures 1,10) clearly proves that such a basin cannot be produced by a major strike-slip fault that runs through it (Figure 11). The lack of evidence to prove the truncation of lithological boundaries at the termination of the basin makes it imperative to argue that such a model is structurally problematic, and particularly if the mapped trace of the fault is linear (Figure 11). The basin sits on top of the >292 Ma old Panjal Traps, and Mesozoic basement (Figures 3,4). These rocks preserve evidence of thrusting and not of strike-slip faulting (Shellnutt, 2018). No one has ever reported any major strike-slip fault in Kashmir basin (Bhat, 1982; Burbank and Johnson, 1982; Shah, 2016b; Shellnutt, 2018) and such a structure is kinetically incompatible with the ∼SW verging of the major Himalayan thrust fault systems (Figure 2) that bound the Kashmir basin at the south and southwest. The geomorphic works (Shah, 2013; Shah, 2016b) have shown that ∼half of the Kashmir basin is subsided and the other half is uplifted (Figure 2), such a geomorphic expression of landforms requires an oblique strike-slip fault with a normal component and not a reverse component as is shown to support the model (Figure 11C, compare right and left). Alam et al. (2015, 2016) have not suggested any large scale normal faulting in Kashmir basin, which are required to accommodate the oblique strike-slip movement that could explain the topographic up and low of Kashmir basin (Figure 2), which the authors have highlighted to be one of the consequences of the strike-slip faulting. Since the trace of the CKF runs through the center of Kashmir basin (Figure 11C), and if it has produced the basin it cannot pass through the center of that basin (Shah, 2016b; Shah, 2018), which makes this model unrealistic (Shah, 2018; Bilham, 2019).
New Data
New Structural Mapping Using Satellite Data
Our new mapping shows (Figures 5,6) clear evidence for sinistral strike-slip faulting, that is, associated with the JF. The ∼N–S trending fault cuts through the rocks, and displaces ridges and streams for >26 km, and the displacement of geomorphic markers varies from meters to >2 km (Figure 5). The total extent of the fault system extends until the frontal ranges (Figure 1) and covers >120 km, but the pattern of faulting is more oblique to the south. We suggest this variation as a reflection of curvature of fault and variation in dip amount, which has to be steeper in north and gentler to the south where component of thrusting seems to dominate. The faulting is much dispersed in south where faults are widespread, and stacking of thrusting is common. The evidence of folding is predominant to the south, and faulting is observed at the south of the Mangla Dam (Figure 6B) where the JF cuts the trace of MFT and displaces it left-laterally for more than 400 m. The dam sits on the hanging wall of the active MFT, which is extremely dangerous because it has the potential to slip during a future medium to large magnitude earthquake and could cause widespread destruction.
Further south the faulting disappears under the Jhelum Valley, and seems to diffuse in a much wider deformation zone toward the Potwar Plateau–Salt Range, Pakistan. The details of the structural mapping (Figures 5, 6) suggest dominant control of oblique strike-slip faulting in the formation of ∼NE plunging anticlines that root at thrust faults, and could be classified as typical example of fault bend folds (Figure 6A). Such type of folding is missing in regions that are located at the east of JF (Figure 1), which clearly suggests that these structures are driven by oblique shearing, which on surface is manifested as ∼N–S to NE–SW trending oblique faults (reverse fault with a strong component of left lateral shearing). The entire structural configuration appears to suggest that during the India–Eurasia collision journey the JF has emerged as a lateral oblique fault with more strike-slip component in the north, and less in south where reverse faulting dominates (see Discussion for details).
Field Data
The geological outcrops exposed at several quarry sections (Figures 3,4) reveal that bedrock units are unconformably overlain by Plio-Pleistocene to Holocene sediments. One section (Figure 3B) shows apparent dip while as the other section (Figure 4B) shows true dip of the beds. The details suggest bedrocks are limestone units that preserve no evidence of deformation and metamorphism, which indicates that these were not buried at greater depths, and were uplifted at shallow crustal levels during the thrusting events that followed the India–Eurasia collision. The entire sequence dips ∼30° toward NE, and the younger Plio-Pleistocene Karewa deposits are deposited on top of these rocks (Figure 3). These field relations suggest that the Kashmir basin occupies a central position in the NW Himalaya, and resides on top of the thrust system (Figure 1), which makes it a typical example of an intermontane basin that was formed by thrusting of the Mesozoic basement rocks (Figure 3), and subsequently filled with younger Plio-Pleistocene and Holocene sediments during the later stages of the collision. Morphology of Karewa deposits suggests deposition in a lacustrine setting (Figures 3, 4), and subsequently uplift that involved reverse faults with Kashmir valley as the hanging wall block that tilts ∼NE.
Seismological, Geological, and Paleomagnetic Data
The data on the distribution of significant earthquakes (shown as colored dots in Figure 2A) are plotted on the satellite image of the NW Himalaya to show active faulting. The clustering of earthquakes at the two ends of the Kashmir basin are mainly related to the two major earthquakes (Figure 1A), the 2005 Kashmir, and 1905 Kangra events. The earthquakes within the Kashmir and Peshawar basins are dominantly breaking the shallow portions of the crust (Figure 1A). One event has occurred in the field region in 2010 at a crustal depth of 35 km (Figure 1B).
The presence of large scale Quaternary intermontane basins, that includes the Kashmir basin and the similar such basins in NW Himalaya (Figure 1), highlights the occurrence of such basins throughout the region. The regional seismicity and CMT solutions suggest that Kashmir basin is located at the center of two major seismicity clusters (Figure 2) and the CMT solutions are dominated by ∼NW–SE trending thrust faults that are either hosted on ∼NE or ∼SW dipping fault planes (Figure 2). Since the regional trend of the major Himalayan thrust faults (MBT, MCT, and MHT) is ∼NW–SE, therefore the ∼NE dipping fault planes are our preferred planes for the observed thrust fault related earthquakes (Figure 2B). The new geomorphic evidence of active strike-slip faulting along the JF has been produced (Figures 5, 6) and related to the seismological data that are used as supplementary evidence for the new tectonic model which is presented below. The regional geological map (Figure 1A) shows a sharp truncation across the trace of the JF, and the lithological units are displaced, which is consistent with left-lateral displacement. The Higher Himalaya, Lesser Himalaya, Murree, and Siwalik sequences are truncated, and displaced along the surficial trace of the JF (Figure 1A). The structural and geological evidence for displacement change coincides with the location of HKS which indicates the role of JF in the seemingly complex structural and geological juxtaposition of lithological and structural units (Figure 1).
The paleomagnetic data shown as (blue and yellow) arrows (Figure 12A) display the known rotations relative to the Indian craton (Bossart et al., 1988). These data show ∼45° clockwise rotation of magnetic vectors in the core of the syntaxis and southeast of it. These are observed in the Murree formation and are relative to the Indian craton. The data on the west of the syntaxis show both no rotation and ∼30° counterclockwise rotation, which coincides with the emergence of the JF. The data also show that the earlier tectonic transport on the eastern portion of the syntaxis is parallel to the clockwise rotated magnetic vectors, which is in contrast to the tectonic transport at the western portions where it is parallel to the anticlockwise rotated magnetic vectors (Figure 12A). These contrasting changes occur at the JF (Figure 12). The clockwise, and anticlockwise rotations on either side of the Hazara-Kashmir syntaxis complement our model (Figure 13), and shows similar rotation as our model expected (see below).
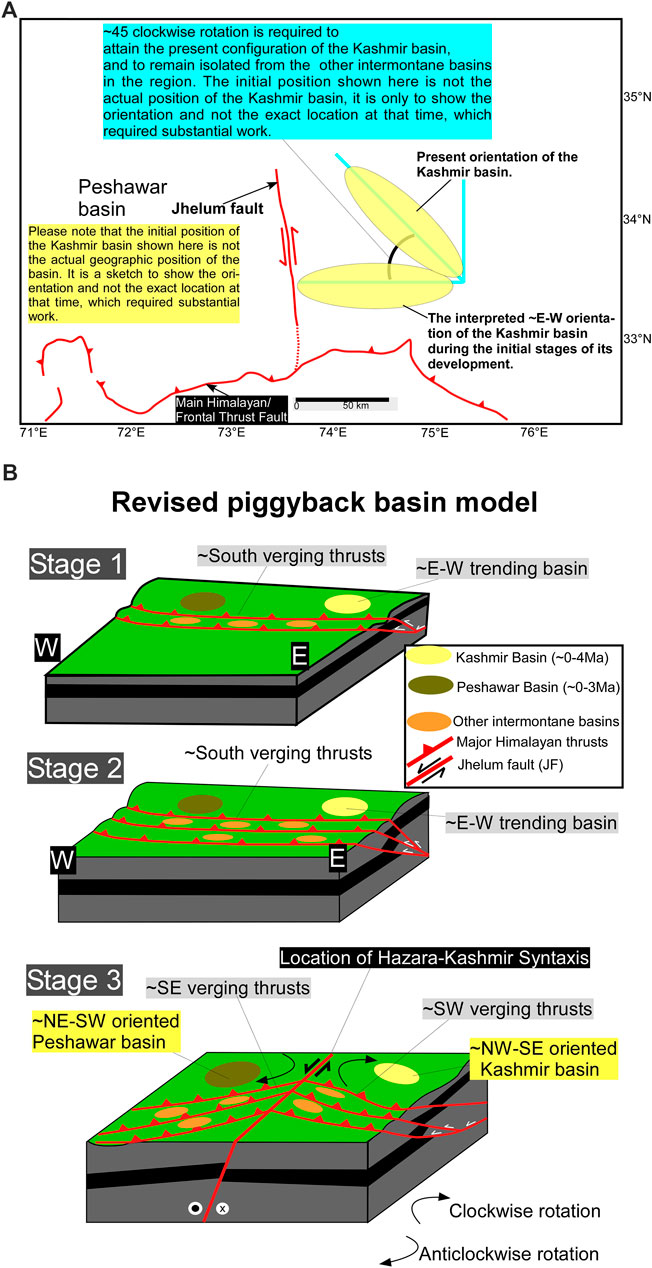
FIGURE 13. The initial orientation of the Kashmir basin could have been ∼E–W and later doing the emergence of Jhelum fault the basin was potentially translated and rotated ∼45° clockwise to acquire the present ∼NW–SE structural configuration (A). The revised piggyback basin model for the formation of Kashmir and Peshawar basins is presented (B). Stage 1: the formation of the internal Himalayan thrust system (MBT, MHT) and intermontane basins (Kashmir, Peshawar, and other similar basins. Stage 2: shows the formation of the main frontal thrust, and other younger intermontane basins. Stage 3: the formation/reactivation of Jhelum fault system resulted in ∼45° clockwise rotation of Kashmir and other basins, which are now located at the east of the Hazara-Kashmir syntaxis, and anticlockwise rotation of those at the west.
Discussion
Rotation of Basins in Northwest Himalaya, and the Modified Piggyback Basin Model
The ∼NW–SE trend of the major Himalayans thrusts (MCT and MBT) abruptly changes to ∼NE–SW when the faults cross the regional sinistral strike-slip fault, the JF (Figures 1,12). This abrupt turn of the major Himalayan thrusts cannot possibly occur without the existence of the fault, which indicates that JF is a younger structure and has formed syn or post the formation of major Himalayan thrust systems (Figure 13). The tectonic geomorphology of the fault suggests ∼NS trending fault zone records left-lateral strike-slip displacement of ridges and streams and the slip varies from <50 m to >2 km (Figures 5,6). This region mark the position of an abrupt change in the vergence of the major Himalayans fault systems (Figure 1) from ∼SW to ∼SE (Figures 1,13), and this indicates that JF has a strong structural control on the evolution, and rotation of basins and fault systems in the region (Figure 13). The formation of Kashmir and Peshawar basins must have started somewhere after the birth of the MCT (∼10 Ma ago) when the orientation of the thrust system was ∼E–W and north dipping (Figure 13, Stage 1). As the India–Tibet collision progressed the fault started to progressively shift southwards (Burbank and Johnson, 1982). This would have resulted in the formation of piggyback basins ∼parallel to the strike of major thrust faults (∼E–W). The amount of displacement on the thrusts that are located to the east of JF has been relatively more compared to thrusts at the west of JF. Such variations are observed in topography on either side of the HKS (Figure 1A), and it seems the thrusts have accumulated more topographic heights in the east as compared to the west (Figure 1A). The present structural orientation of the Kashmir basin (Figure 1) is ∼NW–SE, which is parallel to the regional trend of the major Himalayan thrust systems (Figure 1A). This configuration of the Kashmir basin requires clockwise rotation of the regions east of the JF (Figure 13A). Our estimates indicate that the Kashmir basin has rotated >45° to acquire the present orientation (Figure 13). In contrast, the Peshawar basin has rotated <45° and anticlockwise (Figure 13). These calculations are based on the present day structural orientation of the basins (Figure 13A) and what it will require to physically translate and rotate the basins to the present configurations (Figure 13A). This explains the isolated nature of the Kashmir basin, and its unique structural setting. We think the abrupt curvature of Himalayan thrusts at JF has evolved post the formation of MCT and MBT, and it continues today. The asymmetrical and oblique nature of India–Tibet collision is the possible explanation for the structural development and formation of the region, which is influenced by the tectonic transport on either side of the HKS. This is also reflected by our model, which shows that slip accumulation and release has been higher on eastern portion of HKS because it has relatively narrow map view spread of the structures, and the basins are also constricted and lie tightly parallel to regional thrust faults. This is in contract to structures and basins observed in the western portions of HKS where the basins are wide, and topography is relatively subdued.
Therefore, the oblique convergence of India and Eurasia has been accommodated differently on either side of the HKS, and this has contributed to the overall development of the region. This is shown by the plate convergence rates, which are higher east of the JF and lower at the west of the structure (Schiffman et al., 2013). This directly controls the slip on faults, and therefore the formation of topography, basin formation and development, etc. The existence of JF is a clear indication that plate convergence rates, and hence the displacement on faults at east and west of this structure differ, and that has perhaps caused the formation of the JF structure that runs oblique to the main trend of the Himalayan orogenic belt in the west. This might have initiated early during the collision phase but because the normal convergence dominates in the Himalayan arc therefore the thrusting has dominated over the strike-slip movement during the early phases. However, once the thrusts accumulate load and start southward propagation the strike-slip systems at the both ends of the Himalayan arc would activate to compensate the oblique convergence at the margin of the orogenic belt. The western portions at the HKS has mainly developed because of the easy transport along a system of strike-slip faults with the Chaman fault system as the major ∼southwest tectonic transport facilitator.
The development of the Himalayan foreland basins started during the collision when developing thrusts (e.g., MBT and MHT) pierced through the basins and caused the formation of a number of piggyback basins throughout the frontal portions. These basins are shaped by the interaction of faults, which are mainly controlled by the dip and strike of the fault traces, and it seems the MBT has a strong control on the formation of Kashmir basin because it delimits its extent on the SE, and on the NW the newly formed JF has a major control (Figure 13). We think that the major fold and thrust belt in NW Himalaya was previously oriented ∼E–W, and later with the emergence of JF the whole sequence of thrusting changed to ∼NW–SE on east of JF, and ∼NE–SW on the west of JF. This needs a clockwise rotation of the Kashmir basin, which was facilitated by the sinistral strike-slip JF, and paleomagnetic data (Bossart et al., 1990) confirms this (Figure 12).
Abrupt Variation in Fault Vergence Across the Hazara-Kashmir Syntaxis, and the Role of Jhelum and Chaman Faults
The cause of the formation of Kashmir and Peshawar basins and their present structural configuration has attracted attention in the past, and therefore various studies have attempted to resolve it (Bhat, 1982; Burbank and Johnson, 1982; Burbank and Johnson, 1983; Alam et al., 2015), and as such broadly three tectonic models have been proposed (Figures 7–11). However, these models suffer from various problems that are covered above. Our results show that piggyback tectonic model of Burbank and Johnson (1982) remains a powerful depiction of the geological conditions that led to the formation of the Kashmir, and similar other basins in Himalayas (Burbank and Johnson, 1982; Burbank et al., 1996). This model however has been revisited here with the help of new data that are presented. The new data are combined with the previous works to synthesize the tectonic developmental history of Kashmir and the Peshawar basins. Our results show that oblique convergence India, and Eurasia has contributed to the formation of HKS, and the structural complexity, that is, observed in the region. The major left lateral strike-slip fault systems are accompanied by a stack of reverse faults, and together these faults dictate the tectonics of the western portion of the HKS, which makes it a typical transpresssional deformation regime. This is in contract to how the deformation has been accumulated in the east of the HKS were thrusting is predominant, and slightly oblique convergence is expected. This suggests that transpression related structures have formed during the collisional journey because of the free boundary conditions that exist in the west, and not in the east where faulting has to stack, and lateral movement is restricted. This makes the Chaman fault as the major structure to compensate the oblique convergence, but our data show that JF is also a very important structure and has played a key role in the formation, deformation, rotation, and present configuration of the Kashmir and Peshawar basins (Figure 13). It marks the position where major structures turn on opposite sides (Figure 12), and that makes it a special structure to contribute to the formation of structurally controlled hairpin bend (Figures 12,13), which is not observed at the Chaman fault system, and reinforces the importance of the JF in the regional tectonics.
The ∼N–S trending JF has caused the rotation of MCT and the MBT, which suggests that continuous plate convergence has contributed to the emergence of the JF system. This is also suggested by the field relationships where JF truncates the major Himalayan thrusts (Figures 5,6,12). The young geomorphic evidence of strike-slip faulting is clearly visible where the strike-slip displacement of marker streams and ridges ranges from meters to >2 km. The triangular-shaped ridge (Figure 5) has been created by the intersection of ∼NW–SE trending reverse fault and ∼N–S trending Jhleum fault, and the latter has carved the path for the Jhelum River to follow, which flows through the Kashmir basin, and it suggests these faults have formed/reactivated during or before the formation of ∼4 Ma old Kashmir basin. The region also marks the position where strike and tectonic vergence of Himalayans faults systems abrupt change. Such a sharp turn at east and west of JF is the result of the sinistral strike-slip movement, which is shown by the topographic breaks along the trace of the fault (Figures 5,6). The existence of the sinistral strike-slip movement on the JF is an outcome of the oblique plate convergence of India and Eurasia (Figure 1A). The variation in the plate convergence rates across the trace of the JF has perhaps led to the formation of the fault and resulted in the hairpin curvature of the Himalayan thrusts (Figures 1,13). The rotation of thrusts is shown by the changes in vergence (Figure 13), which varies from ∼SW to ∼SE across the JF, and since the slip on the fault systems has varied during the later stages of the Himalayan orogeny because of the possible changes in the convergence rates, therefore deformation products in the form of structures, geology, geomorphology, and topography have registered these changes (Figures 1A,13).
The age of the formation of Kashmir and Peshawar basins is constrained from the sedimentation history of the basins, which is 3–4 Ma. This age provides a strong base for the depositional age (Burbank and Johsnosn, 1982; Burbank and Johsnosn, 1983), and proves that the basins have formed very recently during the tectonic collision. The major movement on the MCT fault system has occurred ∼22–10 Ma ago (DeCelles et al., 1998), and there is evidence for reactivation again during Miocene-Pliocene (Nakata, 1989; Macfarlane, 1993; Hodges et al., 1996; Harrison et al., 1997). Similarly, the fault movement on the MBT is constrained to have developed ∼11–10 Ma ago (Meigs et al., 1995; Burbank et al., 1996) and continued until ca. 5 Ma (Kumar et al., 2003a; 2003b). Therefore, the age limit on the movement of these Himalayan thrusts suggests that the Kashmir and Peshawar basins have formed before the formation of MCT and possibly syn and post MBT (Figure 13). The initial orientation of MBT and MCT might have been ∼E–W (Figure 13, Stage 1), and therefore, the Kashmir basin started as an ∼E–W trending basin (Figures 13A,B, Stage 1). The present configuration of the Kashmir basin (Figure 1) is ∼NW–SE, which is parallel to the regional trend of the major Himalayan thrust systems (Figure 1), and the basin piggybacks the thrust. This configuration requires clockwise rotation of the regions east of the JF (Figure 13), and our calculations show that the Kashmir basin has rotated >45° from its initiation orientation to acquire the present position (Figure 13). This is in contrast to the Peshawar basin that has rotated <45° anticlockwise (Figure 13). The rotation exactly fits the sinistral strike-slip movement on the JF (Figure 13) provided it is an oblique fault and not a typical steeply dipping strike-slip fault system where translation is expected without rotation. We have mapped JF as an oblique fault where dominantly strike-slip movement is observed at the north, and oblique slip in south, and that favors rotation along with thrusting (Figures 5,6). Therefore, the abrupt curvature of Himalayan thrusts at JF has evolved post the formation of MCT and MBT, and it continues today. This is clearly supported by the paleomagnetic data (Bossart et al., 1990) where the Tertiary age formations are observed to have magnetic vectors orthogonal to the structural trend of the major structures (faults and folds), and such observations are independent of the age of the formations. These vectors show (Figure 12) 45° clockwise rotation of the geological units of the Murree formation in relation to the Indian craton (Bossart et al., 1990), and this exactly corroborates our data. The Late Paleocene age (55 million years) Murree beds are the oldest beds in eastern Pakistan, which suggests deposition in a foreland basin during the initial stage of India–Eurasia collision and subsequent displacement (∼2,600 km to the north) along with the Indian craton. The previous structural data indicates that the actual orientation of the overthrust shear direction has changed and rotated counterclockwise from an early NE–SW direction (220°) to a later NNW–SSE direction (160°), and this has caused the formation of the HKS bend (Bossart et al., 1988; Bossart et al., 1990). However, the paleomagnetic data show that the tectonic rotation is 45° clockwise relative to India in east of the HKS (Bossart et al., 1988; Bossart et al., 1990). We have also observed a clear sharp truncation, and left-lateral displacement of major geological units that include the Higher Himalaya, Lesser Himalaya, Murree, and Siwalik sequences (Figure 1A). This occurs exactly at the JF system, and strongly supports our interpretation, and the evidence for rotation across the trace of fault, which coincides with the HKS (Figure 1).
Conclusion
The asymmetrical and oblique nature of the ongoing lithospheric plate collision in South Asia is the possible explanation for the formation of JF system, and our geomorphic evidence shows sinistral strike-slip movement in the northern portions, which gets oblique to the south. The formation of basins on either side of HKS (Figure 13) is a reflection of oblique convergence between India and Eurasia has been accommodated in western Himalaya syntaxis. The regional topography reveals that strain has been building relatively higher topographical expressions in the eastern portions as compared to the western portions, and this could be related to the presence of evaporites in west, and absence in east (Seeber and Armbruster, 1981). The sharp turn of major geological structures at HKS, and the change in the tectonic vergence from ∼southwest to ∼east could be explained by the presence of lateral ramp that should run ∼parallel to the Jhelum River, and JF fits this configuration. The possible cause of the observed >45° clockwise rotation of the geological structures at east of HKS and <45° at west might be due to the presence of evaporites. The absence of evaporates at the HKS and southeast of it means a strong coupling of the Indian basement and cover sequences, that makes the rocks to resist thrusting, and therefore more topographic relief will be expected along with more potential for devastating earthquakes. This is observed at southeast of HKS where topography is well developed (Figure 1A), and faults are observed to cut through rock sequences with a clear indication of rotation. This could change however in a region where evaporites exist because these rocks offer least resistance to the tectonic forcing thereby causing less strain to buildup, and therefore less potential for major and devastating earthquakes. Such conditions exist in southwest of HKS where extensive and thick Cambrian evaporite deposits work as ideal surfaces to act as decollement horizons between the basement and cover sequence (Bossart et al., 1990). This regions will have less chances of structural rotations, and the expected topography would be subdued with less potential for major devastating earthquakes. Thus the oblique convergence of India–Eurasia has actively contributing to the overall development of the region, and it is still doing it. The variations in convergence rates directly control the strain accumulation and release on faults, and therefore, the formation of topography, basins, etc.
Data Availability Statement
The raw data supporting the conclusions of this article will be made available by the authors, without undue reservation.
Author Contributions
AS, NA, and JM have conceptualized and planned the outline of the manuscript. CC has mainly helped in making of cartons and figures. NM has contributed in the geomorphological and structural mapping of the Jhelum fault. NB has contributed in examination of SRTM and paleomagnetic data. All authors have discussed the results and contributed to writing of the manuscript.
Conflict of Interest
The authors declare that the research was conducted in the absence of any commercial or financial relationships that could be construed as a potential conflict of interest.
Acknowledgments
The authors are very thankful to all the people who have helped us in data collection in Kashmir regions. We are especially thankful to Asst. Prof. Bilal Ahmad Shah and Mr. Saadat Khaki (Geologist) from Anantnag Kashmir for help and support in the field. Financial support from the National Geographic Society is highly appreciated. Without their support, fieldwork would have been impossible. The English language editing was performed by Dr. Sheeba Khwaja, which is highly appreciated.
References
Agrawal, D. P., Dodia, R., Kotlia, B. S., Razdan, H., and Sahni, A. (1989). The Plio-Pleistocene geologic and climatic record of the Kashmir valley, India: a review and new data. Palaeogeogr. Palaeoclimatol. Palaeoecol. 73 (3–4), 267–286. doi:10.1016/0031-0182(89)90008-4.
Alam, A., Ahmad, S., Bhat, M. S., and Ahmad, B. (2015). Tectonic evolution of Kashmir basin in northwest Himalayas. Geomorphology 239, 114–126. doi:10.1016/j.geomorph.2015.03.025.
Alam, A., Ahmad, S., Bhat, M. S., and Ahmad, B. (2016). Response to the commentary by Shah, A.A. (2015) and further evidence supporting the dextral strike–slip pull-apart evolution of the Kashmir basin along the central Kashmir fault (CKF). Geomorphology 253, 558–563. doi:10.1016/j.geomorph.2015.06.017.
Alam, A., Bhat, M. S., Kotlia, B. S., Ahmad, B., Ahmad, S., Taloor, A. K., et al. (2017). Coexistent pre-existing extensional and subsequent compressional tectonic deformation in the Kashmir basin, NW Himalaya. Quatern. Int. 444, 201–208. doi:10.1016/j.quaint.2017.06.009.
Avouac, J. P., Ayoub, F., Leprince, S., Konca, O., and Helmberger, D. V. (2006). The 2005, Mw 7.6 Kashmir earthquake: sub-pixel correlation of ASTER images and seismic waveforms analysis. Earth Planet Sci. Lett. 249 (3–4), 514–528. doi:10.1016/j.epsl.2006.06.025.
Bhat, M. I. (1982). Thermal and tectonic evolution of Kashmir basin vis-à-vis petroleum prospects. Tectonophysics 88, 117–132. doi:10.1016/0040-1951(82)90205-0.
Bhatt, D. K. (1975). On the Quaternary geology of the Kashmir Valley with special reference to stratigraphy and sedimentation. Geol. Surv. India Misc. Publ. 24, 188–203.
Bhatt, D. K. (1976). Stratigraphical status of Karewa Group of Kashmir, India. Himal. Geol. 6, 197–208.
Bilham, R. (2019). Himalayan earthquakes: a review of historical seismicity and early 21st century slip potential. Geol. Soc. Lond. Spec. Publ., 483, SP483.16.
Bossart, P., Dietrich, D., Greco, A., Ottiger, R., and Ramsay, J. G. (1988). The tectonic structure of the Hazara‐Kashmir syntaxis, southern Himalayas, Pakistan. Tectonics 7 (2), 273–297. 10.1029/TC007i002p00273.
Bossart, P., Ottiger, R., and Heller, F. (1990). Rock magnetic properties and structural development in the core of the Hazara‐Kashmir syntaxis, NE Pakistan. Tectonics 9 (1), 103–121. 10.1029/TC009i001p00103.
Burbank, D. W., Beck, R. A., and Mulder, T. (1996). The Himalayan foreland basin. World Reg. Geol. 149–190.
Burbank, D. W., and Johnson, G. D. (1982). Intermontane-basin development in the past 4 Myr in the north-west Himalaya. Nature 298, 432–436. doi:10.1038/298432a0.
Burbank, D. W., and Johnson, G. D. (1983). The late Cenozoic chronologic and stratigraphic development of the Kashmir intermontane basin, northwestern Himalaya. Palaeogeogr. Palaeoclimatol. Palaeoecol. 43, 205–235. doi:10.1016/0031-0182(83)90012-3.
Burbank, D. W., and Reynolds, R. G. (1984). Sequential late Cenozoic structural disruption of the northern Himalayan foredeep. Nature 311 (5982), 114–118.
Burchfiel, B. C., and Stewart, J. H. (1966). “Pull-apart” origin of the central segment of Death Valley, California. Geol. Soc. Am. Bull. 77 (4), 439–442. doi:10.1130/0016-7606.
Butler, R. W. (2019). Tectonic evolution of the Himalayan syntaxes: the view from Nanga Parbat. Geol. Soc. Lond. Spec. Publ. 483 (1), 215–254.
Critelli, S., and Garzanti, E. (1994). Provenance of the lower Tertiary Murree redbeds (Hazara-Kashmir syntaxis, Pakistan) and initial rising of the Himalayas. Sed. Geol. 89 (3–4), 265–284. doi:10.1016/0037-0738(94)90097-3.
DeCelles, P. G., Gehrels, G. E., Quade, J., and Ojha, T. P. (1998). Eocene-early Miocene foreland basin development and the history of Himalayan thrusting, western and central Nepal. Tectonics 17 (5), 741–765. 10.1029/98TC02598.
Gavillot, Y., Meigs, A., Yule, D., Heermance, R., Rittenour, T., Madugo, C., et al. (2016). Shortening rate and Holocene surface rupture on the Riasi fault system in the Kashmir Himalaya: active thrusting within the Northwest Himalayan orogenic wedge. Geol. Soc. Am. Bull. 128 (7–8), 1070–1094. doi:10.1130/B31281.1
Greco, A., and Spencer, D. A. (1993). A section through the Indian plate, Kaghan valley, NW Himalaya, Pakistan. Geol. Soc. Lond. Spec. Publ. 74 (1), 221–236. doi:10.1144/GSL.SP.1993.074.01.16
Harrison, T. M., Ryerson, F. J., Le Fort, P., Yin, A., Lovera, O. M., and Catlos, E. J. (1997). A late Miocene-Pliocene origin for the central Himalayan inverted metamorphism. Earth Planet Sci. Lett. 146 (1–2), 1–7. doi:10.1016/S0012-821X(96)00215-4
Hodges, K. V., Parrish, R. R., and Searle, M. P. (1996). Tectonic evolution of the central Annapurna range, Nepalese Himalayas. Tectonics 15, 1264–1291. 10.1029/96TC01791
Jackson, J., and McKenzie, D. (1984). Active tectonics of the Alpine‐Himalayan belt between western Turkey and Pakistan. Geophys. J. R. Astron. Soc. 77, 185–264. doi:10.1016/0012-821X(83)90198-X
Kaneda, H., Nakata, T., Tsutsumi, H., Kondo, H., Sugito, N., Awata, Y., et al. (2008). Surface rupture of the 2005 Kashmir, Pakistan, earthquake and its active tectonic implications. B. Seismol. Soc. Am. 98, 521–557.
Kozhurin, A., Acocella, V., Kyle, P. R., Lagmay, F. M., Melekestsev, I. V., Ponomareva, V., et al. (2006). Trenching studies of active faults in Kamchatka, eastern Russia: Palaeoseismic, tectonic and hazard implications. Tectonophysics 417 (3–4), 285–304.
10.1016/j.tecto.2006.01.004CrossRef Full Text | Google Scholar
Kumar, R., Ghosh, S. K., and Sangode, S. J. (2003a). Mio-Pliocene sedimentation history in the northwestern part of the Himalayan Foreland Basin, India. Curr. Sci. 84 (8), 1006–1013.
Kumar, R., Sumit, K., Ghosh, R. K., and Sangode, S. J. (2003b). Tectonic impact on the fluvial deposits of Plio-Pleistocene Himalayan foreland basin, India. Sediment. Geol. 158 (3–4), 209–234.
10.1016/S0037-0738(02)00267-1CrossRef Full Text | Google Scholar
Macfarlane, A. M. (1993). Chronology of tectonic events in the crystalline core of the Himalaya, Langtang National Park, central Nepal. Tectonics 12 (4), 1004–1025. 10.1029/93TC00916
Malik, J. N., and Nakata, T. (2003). Active faults and related late Quaternary deformation along the northwestern Himalayan frontal zone, India. Ann. Geophys. 46 (5), 917–936.
Malik, J. N., Sahoo, A. K., Shah, A. A., Rawat, A., and Chaturvedi, A. (2007). Farthest recorded liquefaction around Jammu caused by 8 October, 2005 Muzaffarabad earthquake of Mw = 7.6. J. Geol. Soc. India. 69 (1), 39.
Mann, P., Hempton, M. R., Bradley, D. C., and Burke, K. (1983). Development of pull-apart basins. J. Geol. 91 (5), 529–554.
Marrett, R., and Allmendinger, R. W. (1990). Kinematic analysis of fault-slip data. J. Struct. Geol. 12 (8), 973–986. doi:10.1016/0191-8141(90)90093-E
Meigs, A. J., Burbank, D. W., and Beck, R. A. (1995). Middle-late Miocene (> 10 Ma) formation of the main boundary thrust in the western Himalaya. Geology 23 (5), 423–426. doi:10.1130/0091-7613
Meigs, A. J., Madden, C., Yule, J. D., Gavillot, Y., Hebeler, A., Hussain, A., et al. (2010). “Distributed deformation, distributed earthquakes in the northwest Himalaya,” in Proceedings of the 25th Himalaya-Karakoram-Tibet workshop: US Geological Survey open-file report, San Francisco State University, California, June 7-10, Vol. 1099.
Nakata, T. (1989). Active faults of the Himalayas of India and Nepal. Spec. Pap. Geol. Soc. Am. 232, 243–264.
Pathier, E., Fielding, E. J., Wright, T. J., Walker, R., Parsons, B. E., and Hensley, S. (2006). Displacement field and slip distribution of the 2005 Kashmir earthquake from SAR imagery. Geophys. Res. Lett. 33 (20), 1–5. 10.1029/2006GL027193
Ryan, W. B. F. (2009). Global Multi-Resolution Topography (GMRT) synthesis data set. Geochem. Geophys. Geosyst. 10, Q03014. 10.1029/2008GC002332
Sangode, S. J., and Kumar, R. (2003). Magnetostratigraphic correlation of the late Cenozoic fluvial sequences from NW Himalaya, India. Curr. Sci. 84, 1014–1024.
Schiffman, C., Bali, B. S., Szeliga, W., and Bilham, R. (2013). Seismic slip deficit in the Kashmir Himalaya from GPS observations. Geophys. Res. Lett. 40, 5642–5645. doi:10.1002/2013GL057700
Seeber, L., and Armbruster, J. G. (1981). “Great detachment earthquakes along the Himalayan arc and long-term forecasting,” in Earthquake prediction: an international review. Editors D. W. Simpson and P. G. Richards (AGU: Washington DC), Maurice Ewing Series, Vol. 4, 259–279.
Shabir, A., and Bhat, M. I. (2012). Tectonic geomorphology of the Rambiara basin, SW Kashmir Valley reveals emergent out-of-sequence active fault system. Himal. Geol. 33 (2), 162–172.
Shah, A. A. (2012). Active faults in the Kashmir valley. Geophys. Res. Abstr. 14, 5294. doi:10.1029/2004JB00325
Shah, A. A. (2013). Earthquake geology of the Kashmir Basin and its implication for large earthquakes. Int. J. Earth Sci. 102 (7), 1957–1966. doi:10.1007/s00531-013-0874-8
Shah, A. A. (2015). Kashmir Basin fault and its tectonic significance in NW Himalaya, Jammu and Kashmir, India. Int. J. Earth Sci. 104 (7), 1901–1906. doi:10.1007/s00531-015-1183-1
Shah, A. A. (2016a). Comment on: Alam Akhtar, Ahmad Shabir, Sultan Bhat, M., Ahmad Bashir, 2015. Tectonic evolution of Kashmir Basin in northwest Himalayas. Geomorphology doi:10.1016/j.geomorph.2015.03.025. Geomorphology 253, 553–557. doi:10.1016/j.geomorph.2015.04.032
Shah, A. A. (2016b). No major active backthrust bounds the Pir Panjal Range near Kashmir basin, NW Himalaya. J. Asian Earth Sci. 123, 95–99.
10.1016/j.jseaes.2016.03.017CrossRef Full Text | Google Scholar
Shah, A. A. (2016c). Reply to comments by Ahmad et al. on: Shah, AA, 2013. Earthquake geology of Kashmir Basin and its implications for future large earthquakes International Journal of Earth Sciences doi:10.1007/s00531-013-0874-8 and on Shah, AA, 2015. Kashmir Basin Fault and its tectonic 861 significance in NW Himalaya, Jammu and Kashmir, India, International Journal of Earth Sciences doi:10.1007/s00531-015-1183-1. Int. J. Earth Sci. 105 (2), 689–694. doi:10.1007/s00531-015-1274-z
Shah, A. A. (2018). Comment on: Coexistent pre-existing extensional and subsequent compressional tectonic deformation in the Kashmir basin, NW Himalaya by Akhtar et al. Quatern. Int. 468, 206–210. doi:10.1016/j.quaint.2017.10.034
Shah, A. A., Khwaja, S., Shah, B. A., Reduan, Q., and Jawi, Z. (2018). Living with earthquake and flood hazards in Jammu and Kashmir, NW Himalaya. Front. Earth Sci. 6, 179. doi:10.3389/feart.2018.00179
Shah, A. A., and Malik, J. N. (2017). Four major unknown active faults identified, using satellite data, in India and Pakistan portions of NW Himalaya. Nat. Hazards. 1–21.
10.1007/s11069-017-2949-5CrossRef Full Text | Google Scholar
Shah, A. A., Mohammad Noor, F. Y., and Muhammad Izzat, I. H. I. (2017). Is pull-apart basin tectonic model feasible for the formation of Kashmir basin, NW Himalaya? Scientia Bruneiana. 16, 10–17. doi:10.46537/scibru.v16i1.27
Shah, A. A., Navakanesh, B., Sheeba, K., Reduan, Q., Jawi, Z., Shah, B. A., et al. (2019). “Making living with earthquake hazards a culture,” in Geoscience & Society Summit, Stockholm, Sweden. Amer. Geophy. Union.
Shellnutt, J. G. (2018). The Panjal traps. Geol. Soc. Spec. Publ. 463 (1), 59–86. doi:10.1144/SP463.4
Sylvester, A. G. (1988). Strike–slip faults. Geol. Soc. Am. Bull. 100, 1666–1703. doi:10.1130/0016-7606
Tapponnier, P., and Molnar, P. (1977). Active faulting and tectonics in China. J. Geophys. Res. 82, 2905–2930. doi:10.1029/JB082i020p02905
Taylor, M., and Yin, A. (2009). Active structures of the Himalayan-Tibetan orogen and their relationships to earthquake distribution, contemporary strain field, and Cenozoic volcanism. Geosphere 5 (3), 199–214. doi:10.1130/GES00217.1
Vassallo, R., Mugnier, J. L., Vignon, V., Malik, M. A., Jayangondaperumal, R., Srivastava, P., et al. (2015). Distribution of the late-Quaternary deformation in northwestern Himalaya. Earth Planet Sci. Lett. 411, 241–252.
10.1016/j.epsl.2014.11.030CrossRef Full Text | Google Scholar
Xu, J., Ben-Avraham, Z., Kelty, T., and Yu, H. S. (2014). Origin of marginal basins of the NW Pacific and their plate tectonic reconstructions. Earth Sci. Rev. 130, 154–196. doi:10.1016/j.earscirev.2013.10.002
Yeats, R. S., Nakata, T., Farah, A., Mizra, M. A., Pandey, M. R., and Stein, R. S. (1992). The Himalayan Frontal fault system, in DeJong, K.A., and Farah, A., eds., Geodynamics of Pakistan: geological survey of Pakistan: seis-micity of the Hazara arc in northern Pakistan: decollement vs. basement faulting. Ann. Tectonics Spec. Publ. 6, 85–98.
Yin, A., and Harrison, T. M. (2000). Geologic evolution of the Himalayan-Tibetan orogen. Ann. Rev. Earth Planet. Sci. 28 (1), 211–280. 10.1146/annurev.earth.28.1.211
Keywords: Kashmir basin, faults, main boundary thrust, main central thrust, Jhelum fault, piggyback basin
Citation: Shah AA, Abd Manan N, Aliudin NAB, Cahyaningsih C, Batmanathan NM and Malik JN (2020) Formation, Rotation, and Present-Day Configuration of Kashmir and Peshawar Basins in NW Himalaya. Front. Earth Sci. 8:569771. doi:10.3389/feart.2020.569771
Received: 05 June 2020; Accepted: 11 September 2020;
Published: 14 October 2020.
Edited by:
Alessandro Tibaldi, University of Milano-Bicocca, ItalyReviewed by:
Elena Russo, University of Milano-Bicocca, ItalyFederico Pasquaré Mariotto, University of Insubria, Italy
Copyright © 2020 Shah, Abd Manan, Aliudin, Cahyaningsih, M Batmanathan and Malik. This is an open-access article distributed under the terms of the Creative Commons Attribution License (CC BY). The use, distribution or reproduction in other forums is permitted, provided the original author(s) and the copyright owner(s) are credited and that the original publication in this journal is cited, in accordance with accepted academic practice. No use, distribution or reproduction is permitted which does not comply with these terms.
*Correspondence: Afroz Ahmad Shah, afroz.shah@ubd.edu.bn