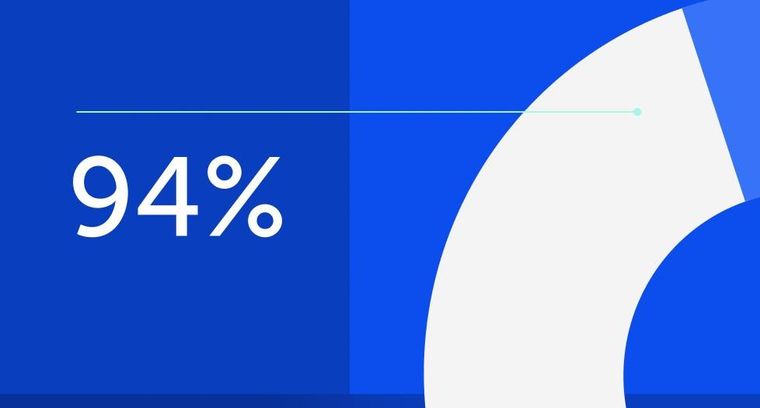
94% of researchers rate our articles as excellent or good
Learn more about the work of our research integrity team to safeguard the quality of each article we publish.
Find out more
ORIGINAL RESEARCH article
Front. Earth Sci., 18 November 2020
Sec. Biogeoscience
Volume 8 - 2020 | https://doi.org/10.3389/feart.2020.558823
This article is part of the Research TopicThe Oceanic Particle Flux and its Cycling Within the Deep Water ColumnView all 17 articles
We measured sedimentation fluxes around JA06 Seamount (Xufu Guyot; 19°30′N, 158°00′E) as part of an environmental baseline survey in Japan’s exploration area for cobalt-rich crusts in the subtropical northwest Pacific. Sinking particles were collected at the flat top (sediment trap depths: 900 and 1,000 m) and northeastern base (sediment trap depths: 1,000 and 4,720 m) of the seamount from June 2016 to April 2017. Total mass fluxes were very low, with average values of 4.3–4.9 and 9.3 mg m−2 d−1 in the shallow traps at the flat top and base, respectively, which is consistent with an oligotrophic system. The lower fluxes at the flat top probably reflect lower productivity of siliceous microplankton, such as diatoms. However, we were unable to substantiate any potential mechanisms for this difference in productivity and cannot evaluate whether this is representative of typical conditions. When combined with previous observations at two adjacent seamounts, our results indicate widespread seasonality in sediment fluxes with a peak in late summer (August–September). However, satellite data indicate that summer is the season with the lowest primary production. This discrepancy could be explained either by phytoplankton blooms fueled by symbiotic nitrogen fixation that only cause minor increases in surface-ocean chlorophyll or short-lived blooms induced by passing typhoons under thick cloud cover. At the base site, we also analyzed material and element transport rates from shallow to deep waters. Half of the organic matter and >80% of the carbonate in sinking particles was not degraded in the water column, suggesting that most of the regeneration of these materials occurs near or on the sediment surface. Furthermore, four major processes appeared to control elemental fluxes in the area: lithogenic (Al, Ti, Fe), carbonate (Mg, Ca, Sr), biogenic (+scavenging) (Ni, Zn, Cd, Pb), and scavenging (V, Mn, Co, Cu, rare earth elements) processes. The estimation of excess flux based on the composition of upper continental crust demonstrated that >85% of total Mn, Co, Ni, Cu, Zn, Cd, and Pb fluxes were attributable to scavenging (+biogenic uptake). Scavenging-dominant metal fluxes are likely ubiquitous in the oligotrophic open ocean.
Deep-sea mining has attracted increased interest in recent years due to rising demand for metallic elements that are crucial for cutting-edge and green technologies. One potential target for deep-sea mining is cobalt-rich ferromanganese crusts, which are marine polymetallic mineral deposits composed of hydrogenetic manganese oxide layers that slowly precipitate on seamount basement rocks. The subtropical Northwest Pacific is considered a prime area for exploration because of the presence of thick crusts on old seamount groups. On the high seas of the northwest Pacific, several countries including Japan, China, Korea, and Russia have already signed exploration contracts for cobalt-rich crusts with the International Seabed Authority (ISA). To minimize environmental impacts from any future deep-sea mining, careful environmental surveys, and assessments are essential. The ISA environmental guidelines (ISBA/25/LTC/6) mandate the study of six environmental baseline data groups: 1) physical oceanography, 2) chemical oceanography, 3) geological properties, 4) biological communities, 5) bioturbation, and 6) fluxes to the sediment.
The subtropical northwest Pacific is strongly oligotrophic. Since seamount ecosystems are mainly sustained by sinking organic matter, fluxes to the sediment are extremely important. Furthermore, a baseline assessment of fluxes and sinking particle compositions around the seamount is necessary to assess the impact of sediment plumes and discharge water from mining activities. Sediment traps are used to quantitatively study the seasonality and vertical transport of sinking particles in the ocean. Sediment trap observations are usually conducted in highly productive regions and have been used to improve marine carbon-cycle modeling (e.g., Ittekkot et al., 1991; Honjo, 1996; Kawahata, 2002). Observations in oligotrophic regions, however, are very limited (see the map compiled by Lutz et al., 2007). Seasonal variations in primary production and resulting export fluxes are generally small in the stratified north Pacific subtropical gyre (e.g., Field et al., 1998). However, enigmatic phytoplankton blooms have been recorded in the eastern north Pacific during the summer, which is the season when the ocean is most stratified (Karl et al., 2001; Wilson, 2003), and subsequent studies have revealed some of the unique mechanisms involving nitrogen fixation that support these blooms (e.g., Dore et al., 2008; Karl et al., 2012). Many knowledge gaps about fundamental processes in the oligotrophic open ocean remain unaddressed today, such as the influence of large seamounts on particulate fluxes, relationships between primary production and export fluxes, differences in particle composition between shallow and deep water, and particulate elemental fluxes from the shallow ocean to seafloor.
In 2016, four sediment traps were deployed and moored for a year on the flat top and base of JA06 seamount (Xufu Guyot) in the ISA-licensed area as part of an environmental baseline survey. In this paper, we discuss ISA baseline data group six (fluxes to the sediment) based on spatial/seasonal differences in flux rate and chemical compositions of sinking particles. Other environmental baseline data we obtained in the vicinity of the seamount have been reported elsewhere (Nagao et al., 2018; Sugishima et al., 2018; Iguchi et al., 2020; Yamaoka et al., submitted).
JA06 seamount (Xufu Guyot; 19°30′N, 158°00′E) is a large Guyot located in international waters in the northwest Pacific (Figure 1). The seamount summit has a depth of ∼1,500 m, and the rise is located at >5,000 m. JA06 seamount is the southernmost of six seamounts located within Japan’s exploration area for cobalt-rich ferromanganese crusts, and it is located within the oligotrophic subtropical gyre throughout the year. According to CTD measurements taken around the seamount in May 2016, surface water temperature and salinity are 27.7°C and 34.8, respectively; there were no marked differences between water column profiles taken at the flat top and base (Yamaoka et al., submitted).
FIGURE 1. (A) Map of Japan’s exploration area for cobalt-rich ferromanganese crusts, covering six seamounts (JA02, 03, 04, 06, 12, and 17) southeast of Minamitori Shima Island (also known as Marcus Island) in the northwest Pacific. Yellow circle indicates Japan’s exclusive economic zone (EEZ). (B) Bathymetry of JA06 Seamount showing locations of mooring sites JA06-M01 and M02 (red circles) and sampling sites for deep-sea sediments (pelagic clay) (black squares; JA06-B02 and B04). (C) Deployment depths of sediment traps used in our study.
Time-series sediment traps (SMD-26S, NiGK Corporation, Tokyo, Japan) comprising 22 sample bottles, each with a 0.5 m2 inlet aperture, were moored to the seafloor at two sites. Site JA06-M01 was located on the northeastern side of the seamount at the seamount base (water depth, 5,220 m), and site JA06-M02 was located on the flat top (water depth, 1,270 m). Two traps were deployed at each site. At site JA06-M01, traps were deployed at depths of 1,000 m (“Shallow-3”) and 4,720 m (“Deep-1”). The deep trap was located 500 m above the seafloor. At site JA06-M02, traps were deployed at 900 m (“Shallow-1”) and 1,000 m (“Shallow-2”). According to the pressure data of sensors attached to the sediment traps, the actual water depths of Shallow-2, Shallow-3, and Deep-1 traps were 990, 839, and 4,792 m, respectively. Before deployment, all sample bottles were filled with filtered seawater (filter pore size: 0.45 µm). Formaldehyde was then added to produce a 3% solution buffered with sodium borate. Traps were deployed at site JA06-M01 from June 7, 2016 to April 20, 2017 (318 days) and at site JA06-M02 from June 7, 2016 to April 21, 2017 (319 days). Sampling interval (sampling period per one collecting cup) was 15 days. Upon recovery, sample bottles were immediately refrigerated at about 4°C onboard the vessel.
The trapped material was carefully wet-sieved through a 1-mm sieve after all recognizable zooplankton swimmers were removed. The <1 mm fraction was freeze-dried and homogenized in preparation for bulk analysis. Carbonate and opal contents were determined by using modified versions of methods developed by Engleman et al. (1985) and Mortlock and Froelich (1989). For each sample, total carbon and nitrogen contents were measured with an elemental analyzer (Flash 2000, Thermo Scientific, Waltham, MA, USA), and then organic carbon content was calculated by subtracting carbonate content from total carbon content. The amount of lithogenic matter in each sample was calculated as follows: Lithogenic matter = total matter − carbonate − opal − organic matter (where organic matter was assumed to be 1.8 × organic carbon content, Kawahata et al., 2000). For elemental analysis, ∼5 mg of the powdered sample was weighed in a Teflon vial and completely digested in an ultrapure HNO3-HF-HClO4 mixture. After the sample was dried down, the residue was dissolved in 2% HNO3. Concentrations of major elements (Na, Mg, Al, P, K, Ca, Fe) were measured by using inductively coupled plasma atomic emission spectroscopy (ICP-AES SPS7800, Seiko Instruments, Chiba, Japan). Concentrations of trace elements were determined by using inductively coupled plasma mass spectroscopy (ICP-MS 7700x, Agilent Technologies, Santa Clara, CA, USA) combined with the indium internal standard technique. Analytical precision was better than 7% for each elemental analysis, except for the Na, K, and Ti analyses, which had an analytical precision of 10%.
Ocean color data, including chlorophyll a concentration, photosynthetically active radiation, and sea surface temperature were obtained from the Moderate Resolution Imaging Spectroradiometer (MODIS) mounted on the aqua satellite through NASA’s ocean color Web. The Aqua satellite passes over the equator from south to north in the afternoon, and aqua MODIS views the entire surface of the Earth every 1 to 2 days, acquiring data in 36 spectral bands or wavelength groups. Further details on data collection are compiled in Table S1. To estimate depth-integrated monthly-averaged net primary production (NPP), defined as the difference between gross photosynthetic production rate and respiration rate, the Vertically Generalized Production Model (Behrenfeld and Falkowski, 1997) was used in combination with the two-phytoplankton community model of Kameda and Ishizuka (2005).
At Site JA06-M02 (on the flat top), mean total mass fluxes were 4.3 and 4.9 mg m−2 d−1 for traps Shallow-1 (900 m) and Shallow-2 (1,000 m), respectively (Table 1). For Shallow-1, mean fluxes of organic matter, carbonate, biogenic opal, and lithogenic matter were 0.5, 3.7, 0.1, and 0.4 mg m−2 d−1, respectively, and the corresponding mean contents were 11%, 78%, 2%, and 9%. C/N atomic ratios for most of the samples were about seven. Mean opal/carbonate and Corg/Cinorg ratios were 0.03 and 0.67, respectively. Samples from Shallow-2, which was deployed at a similar depth, had similar characteristics.
TABLE 1. Mean fluxes, average compositions, and ratios (C/N, opal/carbonate, and Corg/Cinorg) of sinking particles collected at JA06 seamount.
At Site JA06-M01 (on the northeastern side of the seamount base), mean total mass fluxes were 9.3 mg m−2 d−1 for trap Shallow-3 (1,000 m; Table 1). Mean fluxes of organic matter, carbonate, biogenic opal, and lithogenic matter for this trap were 1.7, 5.7, 0.7, and 1.2 mg m−2 d−1. Mean contents of organic matter, carbonate, biogenic opal, and lithogenic matter were 18%, 62%, 7%, and 13%, respectively. C/N atomic ratios for most of the samples were about 8. Mean opal/carbonate and Corg/Cinorg ratios were 0.12 (mass ratio) and 1.4 (molar ratio), respectively.
These obtained total mass fluxes were the lowest among reported sediment trap observations in the global ocean and comparable to the mass flux of 4.8 mg m−2 d−1 obtained at 1,200 m water depth in the western Pacific below the north equatorial current (Kempe and Knaack, 1996), with similar mean percentage composition (15% organic matter, 70% carbonate, 9% biogenic opal, and 6% lithogenic matter). In the shallow traps at both sites around JA06 Seamount, seasonal variations in total mass fluxes showed similar patterns (Figures 2A–C, Table S2). However, average total mass fluxes at the base site were twice as large as those at the flat top site. One possible explanation is that the two sites have different rates of primary production; sinking fluxes roughly correspond to production rate in the surface ocean. Previous studies have posited the existence of a “seamount effect,” in which local upwelling caused by circular currents at the seamount summit can boost primary production (Furuya et al., 1995; Oliveira et al., 2016; Turnewitsch et al., 2016). This effect, however, is inconsistent with the lower primary productivity at the flat top inferred from the sinking fluxes in this study. In fact, seamount effects have only been reported for seamounts with shallow summits (<300 m depth). Due to the much greater depth at the summit of JA06 seamount (∼1,500 m), any interaction between the seamount and ocean currents might have little effect on surface ocean structure. This is supported by physicochemical parameters (temperature, salinity, dissolved oxygen, and inorganic nutrient concentrations) measured around the seamount in May, which showed no obvious differences in the vertical profiles measured at the flat top and base (Yamaoka et al., submitted).
FIGURE 2. Fluxes of organic matter, carbonate, opal, and lithogenic elements from June 2016 to April 2017 at four sediment traps around JA06 seamount (A) shallow-1 (depth, 900 m) and (B) shallow-2 (1,000 m) at site JA06-M02 on the seamount flat top, and (C) Shallow-3 (1,000 m) and (D) Deep-1 (4,720 m) at site JA06-M01 on the seamount base.
Organic matter C/N ratios were similar (around 7–8) in both flat-top traps and in Shallow-3 (the shallow trap at the base site). This range is consistent with the C/N ratios of living marine phytoplankton (6.6–7.0; Redfield et al., 1963; Takahashi et al., 1985). However, Shallow-3 had an elevated opal/carbonate ratio (0.12) and Corg/Cinorg ratio (1.4) compared to the flat-top traps (opal/carbonate ratio, 0.03; Corg/Cinorg ratio, 0.53–0.67). This suggests that settling organic matter is also associated with other materials besides carbonate.
Figure 3 shows relationships between organic matter flux and fluxes of various components (carbonate, biogenic opal, and lithogenic), as well as total flux, at all the sediment traps. The larger organic matter flux observed in Shallow-3 is associated with increased opal and lithogenic fluxes (Figures 3C, D). The lithogenic fraction at sediment traps in the north Pacific is mainly composed of fine clay minerals, which originate in eolian dust transported from the Asian continent by westerly winds (Kawahata, 2002). Atmospheric dust concentrations peak in spring across wide areas of the north Pacific, reflecting frequent dust storms in the source region, with the greatest concentrations occurring at mid-latitudes (Uematsu et al., 1983). However, lithogenic fluxes at JA06 Seamount were relatively low in spring, suggesting small loads and little seasonality of atmospheric dust. Since secular differences in atmospheric input between flat top and base sites are unlikely, lithogenic fluxes probably depend on organic matter flux.
FIGURE 3. Organic matter (OM) flux vs. (A) total mass, (B) carbonate, (C) opal, and (D) lithogenic fluxes. Dashed lines show regression lines for data from shallow and deep sediment traps.
Planktic organisms such as diatoms are known to play an important ballasting role in particulate organic carbon transport due to their propensity to form aggregates and sink rapidly (Smetacek, 1985; Honda and Watanabe, 2010). A two-year observation timeseries at station ALOHA in the oligotrophic north Pacific subtropical gyre demonstrated that diatoms account for only 3–7% of annual primary production but support 9–20% of organic carbon flux at 150 m (Brzezinski et al., 2011). Radiolarians and phaeodarians are another major siliceous microplankton taxon. Although rates of organic carbon export by them have not been quantitatively estimated, it is likely that they also effectively transport organic carbon downward due to their larger size. The mean contents of organic matter, biogenic opal, and lithogenic matter in Shallow-3 were 9%, 5%, and 3% higher, respectively, than in the flat-top traps. By contrast, the mean carbonate content was 17% lower (Table 1). Despite the fact that biogenic opal was the smallest constituent of the sinking particles, its efficient vertical transport capacity seems to contribute greatly to total mass fluxes. Based on these observations, we speculated that growth rates of siliceous microplankton may be constrained at the flat top site. However, the environmental basis of this constraint is unclear. Further comparative studies are needed to clarify the mechanisms controlling primary production and particulate fluxes on and off the seamount.
In Figure 4, the total mass fluxes at all three shallow traps at JA06 seamount are compared with those from two other seamounts in Japan’s exploration area. The first sediment trap experiment conducted within the area was conducted on the flat top of JA04 seamount (20°50′N, 157°18′E; water depth 2,257 m), where a single trap was deployed 23 m above the seafloor from April 8, 2001 to May 18, 2002. Another sediment trap was deployed above the flat top of JA02 Seamount (21°19′N, 159°47′E; water depth 1,600 m), where a single trap was deployed 23 m above the seafloor from August 5, 2006 to July 31, 2007. The results from these two seamounts showed a similar low total mass flux with a strong peak in September. These observations suggest that low total mass fluxes with late summer peaks are common in Japan’s exploration area in the north Pacific subtropical gyre. The range of total mass fluxes observed in our study is similar to those observed in the oligotrophic region under influence of the equatorial counter current. Kawahata et al. (2000) reported a mean total mass flux of 13.2 mg m−2 d−1 at their site 4 (8°N, 175°E; water depth 1,637 m), although they did not observe any seasonal patterns.
FIGURE 4. Seasonal variations in total mass fluxes at shallow sediment traps on the (A) JA02, (B) JA04, and (C,D) JA06 seamounts in Japan’s exploration area. Three-letter month designations show the month of peak flux for each sediment trap.
Interestingly, the observed seasonality in total mass fluxes is inconsistent with satellite-based estimates of NPP in the area. This is an important inconsistency that needs to be addressed. Figure 5 shows monthly average NPP at three seamounts (JA02, JA04, and JA06) estimated by using a 17-year timeseries (July 2002 to October 2018) of NASA Aqua/MODIS data. The estimated NPP varied seasonally, with an early spring bloom from February to May. This early spring bloom may be related to broader and lower-amplitude peaks in total mass fluxes observed around April in sediment traps at JA02 Seamount (Figure 4). Kawahata (2006) reported one broad maximum from January through March in total mass fluxes obtained from a sediment trap (site 6; 30°N, 175°E; water depth 3,873 m). However, the August to October NPP minimums estimated from the satellite data conflict with the late summer peaks in total mass flux observed at JA02, JA04, and JA06 seamounts.
FIGURE 5. Annual climatology of satellite-based net primary production for the period 2002–2018 around the (A) JA02, (B) JA04, and (C,D) JA06 seamounts.
On the basis of a global-scale compilation of sediment trap experiments, Lutz et al. (2007) found that sediment flux maxima are generally synchronous with peaks in primary production and that the oligotrophic subtropical gyres in the Pacific, where production is relatively constant year-round, are characterized by reduced seasonality. Indeed, seasonal maximum-to-minimum monthly flux ratios in the shallow traps on and around JA06 Seamount ranged from 2 to 3. This is clearly lower than the ratios observed at high latitudes and in polar regions, where maximum-to-minimum (summer to winter) flux ratios are ∼20 (Lutz et al., 2007). However, it is still unclear why we obtained very high maximum-to-minimum flux ratios (6–10) on JA04 and JA02 seamounts.
The late summer phytoplankton booms and subsequent particle export pulses appear to be a recurring phenomenon in the oligotrophic eastern north Pacific, especially to the northeast of the Hawaiian islands (Karl et al., 2001; Wilson, 2003). The blooms are not associated with deep mixing events or with dust deposition (Wilson, 2003), but rather are fueled by N2 fixation by Trichodesmium and/or diatom–cyanobacteria symbiosis assemblages and nitrogen influx to the euphotic zone from vertical diatom migrations (Wilson et al., 2008; Church et al., 2009; Karl et al., 2012). When analyzing satellite remote sensing data on ocean color, Wilson (2003) used a threshold of chlorophyll a concentration >0.15 mg m−3 to define a bloom. Based on this definition, blooms have never been observed in the western north Pacific subtropical gyre (Wilson et al., 2008; Wilson and Qiu, 2008). A shallower nutricline and convergent surface conditions in the eastern gyre are considered to be more favorable for the accumulation of buoyant organisms and nitrogen fixers (Dore et al., 2008; Wilson et al., 2008). However, summer blooms are not always detected by satellites and do not always reach the arbitrary 0.15 mg m−3 chlorophyll threshold (White et al., 2007; Villareal et al., 2011). Furthermore, Karl et al. (2012) concluded that there is no direct coupling between the appearance of increased surface chlorophyll and the summertime export pulse, based on analysis of a 13-year sediment trap dataset obtained at Station ALOHA (22°45′N, 158°W). Therefore, it is possible that the late summer peak of total mass fluxes observed in this study reflects blooms supported by N2 fixation that are invisible to satellite observation, although the trigger for these blooms has not yet been thoroughly resolved.
Alternatively, the presence of unusual seasonal peaks in sedimentation fluxes invokes the possibility of an episodic climate event. In late summer, our study area is frequently influenced by hurricanes and tropical depressions. According to historical hurricane tracks obtained from NOAA (Figure 6), a tropical depression formed 450 km east-southeast of JA06 seamount on August 8, 2016, then developed into a tropical storm the next day and moved in a clockwise direction from southeast to west around 200–350 km off the seamount over a period of 4 days. Although one more tropical depression quickly passed by the seamount on 7–8 October, its impact would have been limited. The numerical simulations suggested that typhoon-induced blooms in the western north Pacific depend on the moving speeds of typhoons, that is, slow-moving typhoons induce stronger upwelling and vertical mixing (Shibano et al., 2011; Lin, 2012). From 16 to September 19, 2006, a tropical depression rapidly developed into an H3 hurricane 100–350 km southwest of JA02 seamount. In 2001, from the end of August to early September, three tropical depressions appeared 240–600 km south, southeast, and east of JA04 seamount. Hurricane (typhoon)-induced blooming is well established, although its impact on sinking particle flux is still unknown. Strong upper-ocean mixing by intense typhoon winds and the upwelling of nutrient-rich waters have been proposed as a mechanism for storm-induced blooms (Price, 1981; Dickey et al., 1998; Lin et al., 2003; Sanford et al., 2011). Especially, many typhoons-induced phytoplankton blooms have been reported as enhancement of chlorophyll a concentration and sea surface temperature cooling of −2 to −7°C in the south China sea (Sun et al., 2010; Lin, 2012; Ye et al., 2013; Zhao et al., 2015; Liu and Tang, 2018; Wang et al., 2020). Based on analyses of satellite data in the subtropical northwest Pacific, Lin (2012) proposed that typhoon-induced phytoplankton blooms require intense wind speed, sufficiently long transit time, and the absence of a warm ocean eddy. A recent study also suggested that heavy rainfall from typhoons could enhance blooming (Lin and Oey, 2016). In addition to the fact that heavy cloud cover inhibits satellite observation, the local and short-lived nature of these blooms may hinder their detection in average monthly NPP data. It is also important to consider the possibility of subsurface phytoplankton bloom. Subsurface chlorophyll a maximum (SCM) has been observed frequently in tropical and subtropical oceans. Ye et al. (2013) showed that subsurface chlorophyll a bloom was stronger and lasted longer than the surface chlorophyll a bloom after the passage of a typhoon in the south China sea. The physical-biogeochemical coupled model supported increase in the chlorophyll a lasted for longer in the subsurface layer than in the surface layer (Pan et al., 2017). According to Honda et al. (2018), in the western Pacific subtropical gyre, mesoscale cyclonic eddies enhanced subsurface primary productivity resulting in increase in sinking particle flux. It is likely that such responses of the subsurface chlorophyll a were invisible from satellite.
FIGURE 6. Tracks of hurricanes (typhoons) passing through Japan’s exploration area in (A) 2001 (B) 2006, and (C) 2016. Data are from NOAA’s historical hurricane tracks (https://coast.noaa.gov/hurricanes/). TD: tropical depression, TS: tropical storm, H1–H5: hurricanes of category 1–5 on the Saffir–Simpson scale. For each year, the seamount where sediment trap observations were conducted is highlighted in yellow. Yellow dashed-line circle indicates radius 500 km from the highlighted seamount.
In trap Deep-1 (depth, 4,720 m) at site JA06-M01 (on the northeastern side of the seamount base), mean total mass flux was 8.6 mg m−2 d−1 (Figure 2D). Mean fluxes of organic matter, carbonate, biogenic opal, and lithogenic matter were 0.9, 4.9, 1.5, and 1.4 mg m−2 d−1, respectively. Mean contents of organic matter, carbonate, biogenic opal, and lithogenic matter were 11%, 55%, 17%, and 17%, respectively. C/N atomic ratios for most of the samples were about 10. Mean opal/carbonate and Corg/Cinorg ratios were 0.32 (mass ratio) and 0.96 (molar ratio).
Compared to the shallow trap (Shallow-3), organic matter and carbonate fluxes in the deep trap were low and fluxes of lithogenic matter were almost the same. This is due to the degradation of organic matter and dissolution of carbonate in the water column. Organic matter fluxes correlated more closely with lithogenic fluxes (Figure 3D), suggesting an important role of atmospheric dust in downward organic matter transport. The slope of the regression between organic matter and lithogenic fluxes was steeper for the deep trap than for other traps around the seamount (Figure 3D). Assuming settling speeds of 160–200 m day−1 (e.g., Takahashi, 1986), degradation would decrease organic matter flux from depths of 1,000–4,720 m by half within 19–23 days. The elevated C/N ratio of ∼10 at the deep trap is also attributable to organic matter degradation because of the preferential decomposition of organic nitrogen compounds relative to organic carbon. As for carbonate, only 14% of the carbonate at 1,000 m dissolved before leaching at 4,720 m. The local carbonate compensation depth in the area occurs at 4,000–4,500 m (Pälike et al., 2012). Although calcium carbonate solubility (Ω) falls to less than 1 (i.e., is undersaturated) at depth due to the large pressure effect (Kawahata et al., 2019), the dissolution rate of carbonate minerals is likely to be much slower than the sinking speed of settling particles. Surface sediments collected from the base of JA06 seamount (St. B02; water depth 5,009 m) were composed of pelagic clay and contained only 0.1 wt% of total organic carbon (=1.8 wt% organic matter) and 0.5 wt% of carbonate (Yamaoka et al., submitted). Thus, the remaining >50% of organic matter and >80% of carbonate would have degraded or dissolved near or on the seafloor. This is consistent with work by Noriki and Tsunogai (1986), which suggested that the regeneration of biogenic particles occurs mainly in the bottom water and at the sediment surface.
The high biogenic opal content in the deep trap is difficult to interpret. Some species of radiolarians inhabit intermediate (200–1,000 m) and deep water (>1,000 m) (e.g., Yamashita et al., 2002). It is likely that the deep trap collected radiolarian shells from these depths.
Figure 7 shows the relationships between Al and trace element fluxes. The slopes for Ti and Fe are close to the mean values for upper crustal rock (McLennan, 2001), suggesting a lithogenic origin for these elements. On the other hand, Ni, Zn, Cd, and Pb showed elevated fluxes with large variations in the shallow traps. These characteristics resemble P fluxes, implying that these elements are closely related to organic matter. The nutrient-like behaviors of Ni, Zn, and Cd and their correspondence to biogenic flux have been reported in the northeast Atlantic (Kuss and Kremling, 1999; Kuss et al., 2010) and the central Baltic Sea (Pohl et al., 2004). Although the interaction of Pb with sinking particles is still unclear, Pb could be intensively absorbed onto organic matter particles (Schüßler et al., 1997; Pohl et al., 2004). The regression of V, Mn, Co, Cu, and ΣREE fluxes with Al also had elevated slopes. In contrast to the biogenic elements (Ni, Zn, Cd, and Pb), however, fluxes of these elements in the shallow and deep traps were continuously correlated with Al fluxes. These observations suggest that scavenging onto lithogenic materials is the primary process that controls fluxes of these elements.
FIGURE 7. Correlations between trace element and Al fluxes in sediment trap samples from JA06 Seamount. Dashed lines indicate average ratios in upper continental crust (McLennan, 2001). Element names in individual figures with bold, italic, and underline indicate the belonging to lithogenic, biogenic, and scavenging groups, respectively.
Although scavenging behaviors of these elements are well known, they have never been detected from the chemical compositions of sinking particles except for Mn due to the large contribution of lithogenic materials. Kuss and Kremling (1999) found a large excess flux of Mn in the deep northeast Atlantic (from 33 to 54°N at ∼20°W) but did not observe a corresponding enrichment of elemental fluxes. Similarly, excess Mn fluxes in the northwest Pacific (47°N, 160°E) did not coincide with any elemental fluxes (Lamborg et al., 2008). This is because these Mn enrichments were derived from lateral Mn input from a nearby continental shelf. In contrast, our study area is far from continental shelves. Therefore, scavenging is the dominant process. That is, dissolved Mn in seawater is scavenged onto suspended fine aluminosilicate (i.e., clay mineral) particles and metals are absorbed onto the Mn oxide coating. Then, the Mn-enriched suspended matter is incorporated into sinking particles. The positive correlations between biogenic elemental fluxes (i.e., Ni, Zn, Cd, and Pb) in the deep trap (Figure 7) suggest that these elements are also affected by scavenging processes. Previous studies have posited the existence of biological Cu and Co uptake (Saito and Moffett, 2002; Lamborg et al., 2008; Sun et al., 2016). However, the relative impacts of such processes in the removal of elements seems small compared to scavenging in our study area. Although Hwang et al. (2010) suggested that the resuspension of sediments is widespread, especially near continental margins, the direct contribution of resuspended sediments is negligible in our study area. In fact, the chemical composition of sediments in the area is distinct from that of the observed Mn-enriched fraction in sinking particles. As shown in Table 2, pelagic clays collected from the base of JA06 Seamount have greater Mn/Al, Co/Al, and Ni/Al ratios by factors of 2–3 than the deep trap samples. Furthermore, the consistent correlations between the shallow and deep traps observed for scavenging elements (V, Mn, Co, Cu, and REE) indicate that the source of the Mn-enriched fraction is independent of distance from the seabed. Loading of resuspended sediments to both the shallow and deep traps is unlikely.
TABLE 2. Average element concentrations, fluxes, and excess fluxes in shallow and deep traps at the base of the JA06 seamount. The corresponding values for pelagic clay sampled at the same seamount are provided for comparison.
We also compared average element fluxes between the shallow and deep traps at Site JA06-M01 (Table 2). Based on the ratio of deep trap/shallow trap fluxes (D/S), the elements can be divided into three groups as follows: group 1: P, Zn, Cd, Pb (D/S < 1); group 2: Mg, Ca, and Sr (D/S = 1); group 3: Al, Ti, V, Mn, Fe, Co, Ni, Cu, and ΣREE (D/S > 1). The group 1 elements correspond to biogenic elements and are characterized by biological uptake and/or adsorption onto organic matter in surface water and low rates of transport to deep waters due to organic matter degradation. These elements (except for P) are also influenced by scavenging, but recycling processes are predominant. The group 2 elements are carbonate elements, which are major and minor of ions of biogenic calcite. The refractory behaviors of these elements are consistent with the low rate of carbonate dissolution in sinking particles, as discussed above. The group 3 elements have larger fluxes in the deep trap, which results from the incorporation of suspended Mn-oxide coated aluminosilicate particles. Despite the biogenic behavior of Ni, its D/S ratio (1.4) is larger than those of other biogenic elements, suggesting the relative importance of scavenging in its vertical transport.
According to Kuss et al. (2010), excess trace element flux (FTEexc) is determined as follows:
where FTE and FAl are the measured fluxes of the trace element and Al, respectively, and (TE/Al)ucc reflects the average TE/Al ratio of the upper continental crust (McLennan, 2001). Based on this calculation, >85% of total fluxes of Mn, Co, Ni, Cu, Zn, Cd, and Pb are attributed to scavenging (+biogenic uptake) processes. These enrichments in excess metal fluxes would be unique to and common across the pelagic and oligotrophic ocean. Recently Hawco et al. (2018) estimated the Co scavenging rate in the water column in the South Pacific to be 0.11 μg m−2 d−1, which is strongly consistent with the excess Co flux in the deep trap (0.12 μg m−2 d−1).
Assuming the same Al flux to the sediments as to the deep trap (74.9 μg m−2 d−1) and a sediment density of 2.6 g cm−3, the sedimentation rate at the base of JA06 seamount can be roughly estimated as 0.16 mm kyr−1 based on the Al concentration in the sediments (66,900 μg g−1). We then calculated elemental fluxes to the sediments based on this sedimentation rate (Table 2). According to the distribution of metal flux into modern Pacific ocean basins (Chester, 2003), the Mn and Fe fluxes to the seabed in the study area are <27 and <164 μg m−2 d−1, respectively. Thus, our calculated values (24.2 μg m−2 d−1 for Mn and 37.7 μg m−2 d−1 for Fe) seem to be reasonable. The Ti, Mn, Co, Ni, and ΣREE fluxes into the sediments are 2–4 times greater than the fluxes in the deep trap, suggesting further accumulations of these metals near and on the sediment surface. In contrast, Cu, Zn, Cd, and Pb show lower fluxes into sediments than those in the deep trap. This could be explained by losses of the labile fraction associated with biogenic materials. The fluxes of carbonate elements, such as Mg, Ca, and Sr, also significantly decreased in the sediments. In other words, the chemical compositions of sinking particles at 500 m above the seafloor are considerably different from those of deep-sea sediments, especially in areas with slow sedimentation rates where sediments are exposed to bottom water for a long period. Sato and Usui (2018) estimated metal fluxes into cobalt-rich crusts on pelagic seamounts in the Northwest Pacific as 1.9–3.0 μg m−2 d−1 for Mn, 1.4–2.5 μg m−2 d−1 for Fe, 0.03–0.11 μg m−2 d−1 for Co, and 0.01–0.11 μg m−2 d−1 for Ni. Interestingly, the Mn and Co fluxes roughly correspond to the excess fluxes of Mn (1.3–7.0 μg m−2 d−1) and Co (0.04–0.1 μg m−2 d−1) observed in sinking particles in this study. This similarity supports the newly proposed model that continuous Mn oxidation occurs even within the oxygen minimum zone (OMZ) such that particulate Fe-Mn oxide forms piled ferromanganese crusts and nodules (Usui et al., 2017). Recently, Usui et al. (2020) presented evidence of ongoing precipitation of Fe-Mn oxide particles (a few micrometers in diameter) from normal seawater at 900–4,500 m depth based on in situ 15-years exposure tests. Such particulate precipitates could be a common source of Mn for both cobalt-rich crusts and sinking particles.
One year of sediment trap observations on and around seamounts in the exploration area for cobalt-rich crusts revealed unique characteristics of sinking particles in the western North Pacific subtropical gyre. The main findings obtained in this study are as follows:
(1) Total mass fluxes on the seamount flat top were half of those on the base throughout the year. This is probably attributable to a lower population of siliceous microplankton, such as diatoms, in the euphotic zone, although the influence of environmental factors including a putative “seamount effect” is still unresolved.
(2) Particle sinking rates in the north Pacific subtropical gyre are characterized by low total mass fluxes with a sharp peak in late summer. However, summer plankton blooms were not detected by satellite observations. The increased fluxes in late summer may be explained by blooms supported by N2 fixation (similar to those in the eastern gyre but on a smaller scale) or short-lived blooms induced by typhoons (hurricanes) and tropical depressions.
(3) During the vertical transport of particles from depths of 1,000–4,720 m, half of the organic matter was decomposed as compared to only 14% of carbonate elements. Most of the biogenic materials in sinking particles could be regenerated mainly in the bottom water and at the sediment surface.
(4) Elemental analyses of the sinking particles demonstrated that fluxes of metal elements, such as V, Mn, Co, Ni, Cu, Zn, Cd, Pb, and REE, were predominantly driven by scavenging (+biogenic uptake) processes. Such scavenging-dominated metal flux is characteristic of oligotrophic oceans. Among these metals, Ti, Mn, Co, Ni, and REE seem to further accumulate on the seabed.
The raw data supporting the conclusions of this article will be made available by the authors, without undue reservation.
AS, YT, TM, SK, NO, and YI designed the sediment trap experiments. AS and TF collected samples and analyzed chemical compositions. MS and AT analyzed satellite data. KY analyzed elemental composition and wrote the main manuscript text. All authors contributed to the writing and editing of the manuscript.
This project was commissioned by the Agency for Natural Resources and Energy in the Japanese Ministry of Economy, Trade and Industry (METI) and Japan Oil, Gas and Metals National Corporation (JOGMEC). This study was also funded by internal grants from the National Institute of Advanced Industrial Science and Technology (AIST).
Authors AS and TF were employed by the company KANSO Co., Ltd. Authors TM, SK, NO, and YI were employed by the company Japan Oil, Gas and Metals National Corporation.
The remaining authors declare that the research was conducted in the absence of any commercial or financial relationships that could be construed as a potential conflict of interest.
The authors express their appreciation to everyone involved in this project. The authors also thank Dr. Hodaka Kawahata for his valuable comments, which helped improve the manuscript. The Associate Editor Dr. Makio Honda and two reviewers provided constructive suggestions.
The Supplementary Material for this article can be found online at: https://www.frontiersin.org/articles/10.3389/feart.2020.558823/full#supplementary-material
Behrenfeld, M. J., and Falkowski, P. G. (1997). Photosynthetic rates derived from satellite-based chlorophyll concentration. Limnol. Oceanogr. 42, 1–20. doi:10.4319/lo.1997.42.1.0001
Brzezinski, M. A., Krause, J. W., Church, M. J., Karl, D. M., Li, B., Jones, J. L., et al. (2011). The annual silica cycle of the North Pacific subtropical gyre. Deep Sea Res. I 58, 988–1001. doi:10.1016%2Fj.dsr.2011.08.001
Church, M. J., Mahaffey, C., Letelier, R. M., Lukas, R., Zehe, J. P., and Karl, D. M. (2009). Physical forcing of nitrogen fixation and diazotroph community structure in the North Pacific Subtropical Gyre. Global Biogeochem. Cyc. 23, GB2020. doi:10.1029%2F2008GB003418
Dickey, T., Frye, D., McNeil, J., Manov, D., Nelson, N., Sigurdson, D., et al. (1998). Upper-ocean temperature response to hurricane Felix as measured by the Bermuda testbed mooring. Mon. Weather Rev. 126, 1195–1201. doi:10.1175/ 1520-0493(1998)126<1195:UOTRTH>2.0.CO;2
Dore, J. E., Letelier, R. M., Church, M. J., and Karl, D. M. (2008). Summer phytoplankton blooms in the oligotrophic North Pacific subtropical gyre: historical perspective and recent observations. Prog. Oceanogr. 76, 2–38. doi:10.1016/j.pocean.2007.10.002
Engleman, E. E., Jackson, L. L., and Norton, D. R. (1985). Determination of carbonate carbon in geological materials by coulometric titration. Chem. Geol. 53, 125–128. doi:10.1016/0009-2541(85)90025-7
Field, C. B., Behrenfeld, M. J., Randerson, J. T., and Falkowski, P. (1998). Primary production of the biosphere: integrating terrestrial and oceanic components. Science 281, 237–240. doi:10.1126/science.281.5374.237
Furuya, K., Odate, T., and Taguchi, K. (1995). “Effects of a seamount on phytoplankton production in the western Pacific Ocean,” in Biogeochemical processes and ocean flux in the Western Pacific. Editors H. Sakai, and Y. Nozaki, 255–273.
Hawco, N. J., Lam, P. J., Lee, J.-M., Ohnemus, D. C., Noble, A. E., Wyatt, N. J., et al. (2018). Cobalt scavenging in the mesopelagic ocean and its influence on global mass balance: synthesizing water column and sedimentary fluxes. Mar. Chem. 201, 151–166. doi:10.1016/j.marchem.2017.09.001
Honda, M. C., Sasai, Y., Siswanto, E., Kuwano-Yoshida, A., Aiki, H., and Cronin, M. F. (2018). Impact of cyclonic eddies and typhoons on biogeochemistry in the oligotrophic ocean based on biogeochemical/physical/meteorological time-series at station KEO. Prog. Earth Planet. Sci. 5, 42.
Honda, M. C., and Watanabe, S. (2010). Importance of biogenic opal as ballast of particulate organic carbon (POC) transport and existence of mineral ballast-associated and residual POC in the Western Pacific Subarctic Gyre. Geophys. Res. Lett. 37, L02605. doi:10.1029/2009GL041521
Honjo, S. (1996). “Fluxes of particles to the Interior of the open oceans,” in Particle flux in the ocean. SCOPE. Editors V. Ittekkot, P. Schafer, S. Honjo and P. J.Depetris. (Toronto, Canada:Wiley), 91–154
Hwang, J., Druffel, E. R. M., and Eglinton, T. I. (2010). Widespread influence of resuspended sediments on oceanic particulate organic carbon: insights from radiocarbon and aluminum contents in sinking particles. Global Biogeochem. Cyc. 24, GB4016. doi:10.1029/2010GB003802
Iguchi, A., Nishijima, M., Yoshioka, Y., Miyagi, A., Miwa, R., Tanaka, Y., et al. (Forthcoming 2020). Deep-sea amphipods around cobalt-rich ferromanganese crusts: taxonomic diversity and selection of candidate species for connectivity analysis. PLoS ONE.
Ittekkot, V., Nair, R. R., Honjo, S., Ramaswamy, V., Bartsch, M., Manganini, S. J., et al. (1991). Enhanced particle fluxes in Bay of Bengal induced by injection of fresh water. Nature 351, 385–387. doi:10.1038/351385a0
Kameda, T., and Ishizaka, J. (2005). Size-fractionated primary production estimated by a two- phytoplankton community model applicable to ocean color remote sensing. J. Oceanogr. 61, 663–672. doi:10.1007%2Fs10872-005-0074-7
Karl, D. M., Björkman, K. M., Dore, J. E., Fujieki, L., Hebel, D. V., Houlihan, T., et al. (2001). Ecological nitrogen-to- phosphorus stoichiometry at station ALOHA. Deep Sea Res. 48, 1529–1566. doi:10.1016%2FS0967-0645(00)00152-1
Karl, D. M., Church, M. J., Dore, J. E., Letelier, R. M., and Mahaffey, C. (2012). Predictable and efficient carbon sequestration in the North Pacific Ocean supported by symbiotic nitrogen fixation. Proc. Natl. Acad. Sci. U. S. A. 109, 1842–1849. doi:10.1073%2Fpnas.1120312109
Kawahata, H., Fujita, K., Iguchi, A., Inoue, M., Iwasaki, S., Kuroyanagi, A., et al. (2019). Perspective on the response of marine calcifiers to global warming and ocean acidification—behavior of corals and foraminifera in a high CO2 world “hot house”. Prog. Earth Planet. Sci. 6, 5.
Kawahata, H. (2006). “Settling particles in the central North Pacific,” in Global climate change and response of carbon cycle in the equatorial Pacific and Indian oceans and adjacent landmasses, Elsevier oceanography series. Editors H. Kawahata and Y. Awaya (Amsterdam: Elsevier), Vol. 73, 107–133.
Kawahata, H. (2002). Suspended and settling particles in the Pacific. Deep-Sea Res. II. 49, 5647–5664. doi:10.1016/S0967-0645(02)00216-3
Kawahata, H., Suzuki, A., and Ohta, H. (2000). Export fluxes in the western pacific warm pool. Deep-Sea Res. 47, 2061–2091. doi:10.1016/S0967-0637(00)00025-X
Kempe, S., and Knaack, H. (1996). “Vertical particle flux in the western pacific below the north equatorial current and the equatorial counter current,” in Particle flux in the ocean. SCOPE. Editors V. Ittekkot, P. SchŠfer, S. Honjo and P.J. Depetris, Toronto, Canada: Wiley, 313–323.
Kuss, J., and Kremling, K. (1999). Particulate trace element fluxes in the deep northeast Atlantic Ocean. Deep-Sea Res. I 46,149–169. doi:10.1016/S0967-0637(98)00059-4
Kuss, J., Waniek, J. J., Kremling, K., and Schulz-Bull, D. E. (2010). Seasonality of particle-associated trace element fluxes in the deep northeast Atlantic Ocean. Deep-Sea Res. I 57, 785–796. doi:10.1016/j.dsr.2010.04.002
Lamborg, C. H., Buesseler, K. O., and Lam, P. J. (2008). Sinking fluxes of minor and trace elements in the North Pacific Ocean measured during the VERTIGO program. Deep-Sea Res. II. 55, 1564–1577. doi:10.1016%2Fj.dsr2.2008.04.012
Lin, I.-I. (2012). Typhoon-induced phytoplankton blooms and primary productivity increase in the western North Pacific subtropical ocean. J. Geophys. Res. 117, C03039. doi:10.1029/2011JC007626
Lin, I., Liu, W. T., Wu, C.-C., Wong, G. T. F., Hu, C., Chen, Z., et al. (2003). New evidence for enhanced ocean primary production triggered by tropical cyclone. Geophys. Res. Lett. 30, 1718. doi:10.1029/2003GL017141
Lin, Y.-C., and Oey, L.-Y. (2016). Rainfall-enhanced blooming in typhoon wakes. Sci. Rep. 6, 31310. doi:10.1038/srep31310
Liu, F., and Tang, S. (2018). Influence of the interaction between typhoons and oceanic mesoscale eddies on phytoplankton blooms. J. Geophys. Res. 123, 2785–2794.
Lutz, M. J., Caldeira, K., Dunbar, R. B., and Behrenfeld, M. J. (2007). Seasonal rhythms of net primary production and particulate organic carbon flux to depth describe the efficiency of biological pump in the global ocean. J. Geophys. Res. 112, C10011. doi:10.1029/2006JC003706.
McLennan, S. M. (2001). Relationships between the trace element composition of sedimentary rocks and upper continental crust. Geochem. Geophys. Geosyst. 2, 2000GC000109. doi:10.1029%2F2000GC000109
Mortlock, R. A., and Froelich, P. N. (1989). A simple method for the rapid determination of biogenic opal in pelagic marine sediments. Deep-Sea Res. Part A 36, 1415–1426. doi:10.1016/0198-0149(89)90092-7
Nagao, M., Takasugi, Y., Suzuki, A., Tanaka, Y., Sugishima, H., Matsui, T., et al. (2018). Confirming the validity of ADCP velocity measurements for physical environmental assessment in Exploration Areas for cobalt-rich ferromanganese crusts. Proc. ISOPE. 2018, 136–143.
Noriki, S., and Tsunogai, S. (1986). Particulate fluxes and major components of settling particle from sediment trap experiment in the Pacific Ocean. Deep-Sea Res. 33, 903–912. doi:10.1016/0198-0149(86)90005-1
Oliveira, A. P., Coutinho, T. P., Cabeçadas, G., Brogueira, M. J., Coca, J., Ramos, M., et al. (2016). Primary production enhancement in a shallow seamount (Gorringe-Northeast Atlantic). J. Mar. Syst. 164, 13–29.
Pälike, H., Lyle, M. W., Nishi, H., Raffi, I., Ridgwell, A., Gamage, K., et al. (2012). A Cenozoic record of the equatorial Pacific carbonate compensation depth. Nature 488, 609–614. doi:10.1038/nature11360.
Pan, S., Shi, J., Gao, H., Guo, X., Yao, X., and Gong, X. (2017). Contributions of physical and biogeochemical processes to phytoplankton biomass enhancement in the surface and subsurface layers during the passage of Typhoon Damrey. J. Geophys. Res. 122, 212–229. doi:10.1002/2016JG003331
Pohl, C., Löffler, A., and Hennings, U. (2004). A sediment trap flux study for trace metals under seasonal aspects in the stratified Baltic Sea (Gotland Basin; 57°19.20’N; 20°03.00’E). Mar. Chem. 84, 143–160. doi:10.1016/J.MARCHEM.2003.07.002
Price, J. F. (1981). Upper ocean response to a hurricane. J. Phys. Oceanogr. 11, 153–175. doi:10.1175/1520-0485(1981)011<0153:UORTAH>2.0.CO;2
Redfield, A. C., Ketchum, B. H., and Richards, F. A. (1963). “The influence of organisms on the composition of seawater,” in The sea. Editor M. H. Hill (New York, NY: Wiley), 26–77.
Saito, M. A., and Moffett, J. W. (2002). Temporal and spatial variability of cobalt in the Atlantic Ocean. Geochem. Cosmochim. Acta. 66, 1943–1953. doi:10.1016/S0016-7037(02)00829-3
Sanford, T. B., Price, J. F., and Girton, J. B. (2011). Upper ocean response to Hurricane Frances (2004) observed by profiling EM-APEX floats. J. Phys. Oceanogr. 41, 1041–1056. doi:10.1175/2010JPO4313.1.
Sato, H., and Usui, A. (2018). Metal flux as an alternative parameter in evaluating the resource potential for co-rich ferromanganese crusts. Mar. Georesour. Geotechnol. 36 (7), 768–780. doi:10.1080/1064119X.2017.1378781.
Schüßler, U., Schulz-Bull, D. E., and Bauerfeind, E. (1997). Annual fluxes of particulate chemical trace compounds during the North-East Water Polynya Experiment. J. Mar. Syst. 10, 391–400. doi:10.1016/S0924-7963(96)00077-2
Shibano, R., Yamanaka, Y., Okada, N., Chuda, T., Suzuki, S., Niino, H., et al. (2011). Responses of marine ecosystems to typhoon passages in the western subtropical North Pacific. Geophys. Res. Lett. 38, L18608. doi:10.1029/ 2011GL048717
Smetacek, V. S. (1985). Role of sinking in diatom life-history cycles: ecological, evolutionary and geological significance. Mar. Biol. 84, 239–251. doi:10.1007%2FBF00392493
Sugishima, H., Matsui, T., Okamoto, N., and Fukushima, T. (2018). Distributions of megabenthic organisms in the areas of north-west Pacific seamounts where are covered with cobalt-rich ferromanganese crusts. Proc. ISOPE. 2018, 74–80.
Sun, L., Yang, Y.-J., Xian, T., Lu, Z.-M., and Fu, Y.-F. (2010). Strong enhancement of chlorophyll a concentration by a weak typhoon. Mar. Ecol. Prog. Ser. 404, 39–50. doi:10.3354/meps08477
Sun, W.-P., Han, Z.-B., Hu, C.-Y., and Pan, J.-M. (2016). Source composition and seasonal variation of particulate trace element fluxes in Prydz Bay, East Antarctica. Chemosphere 147, 318–327. doi:10.1016/j.chemosphere.2015.12.105
Takahashi, K. (1986). Seasonal fluxes of pelagic diatoms in the subarctic Pacific, 1982–1983. Deep-Sea Res. I 33, 1225–1251. doi:10.1016/0198-0149(86)90022-1
Takahashi, T., Broecker, W. S., and Langer, S. (1985). Redfield ratio based on chemical data from isopycnal surfaces. J. Geophys. Res. 3, 43–61. doi:10.1029/JC090iC04p06907
Turnewitsch, R., Dumont, M., Kiriakoulakis, K., Legg, S., Mohn, C., Peine, F., et al. (2016). Tidal influence on particulate organic carbon export fluxes around a tall seamount. Prog. Oceanogr. 149, 189–213.
Uematsu, M., Duce, R. A., Prospero, J. M., Chen, L., Merrill, J. T., and McDonald, R. L. (1983). Transport of mineral aerosol from Asia over the north Pacific Ocean. J. Geophys. Res. 88, 5343–5352. doi:10.1029%2FJC088iC09p05343
Usui, A., Hino, H., Suzushima, D., Tomioka, N., Suzuki, Y., Sunamura, M., et al. (2020). Modern precipitation of hydrogenetic ferromanganese minerals during on-site 15-year exposure tests. Sci. Rep. 10, 3558.
Usui, A., Nishi, K., Sato, H., Nakasato, Y., Thornton, B., Kashiwabara, T., et al. (2017). Continuous growth of hydrogenetic ferromanganese crusts since 17 Myr ago on Takuyo-Daigo Seamount, NW Pacific, at water depths of 800-5500 m. Ore Geol. Rev. 87, 71–87.
Villareal, T. A., Adornato, L., Wilson, C., and Schoenbaechler, C. A. (2011). Summer blooms of diatom-diazotroph assemblages and surface chlorophyll in the North Pacific gyre: a disconnect. J. Geophys. Res. 116, C03001. doi:10.1029%2F2010JC006268
Wang, T., Zhang, S., Chen, F., Ma, Y., Jiang, C., and Yu, J. (2020). Influence of sequential tropical cyclones on phytoplankton blooms in the northwestern South China Sea. J. Ocean. Limnol.
White, A. E., Spitz, Y. H., and Letelier, R. M. (2007). What factors are driving summer phytoplankton blooms in the North Pacific Subtropical Gyre?. J. Geophys. Res. 112, C12006. doi:10.1029/2007JC004129
Wilson, C. (2003). Late summer chlorophyll blooms in the oligotrophic North Pacific subtropical gyre. Geophys. Res. Lett. 30, 1942. doi:10.1029/2003GL017770
Wilson, C., and Qiu, X. (2008). Global distribution of summer chlorophyll blooms in the oligotrophic gyres. Prog. Oceanogr. 78, 107–134. doi:10.1016/j.pocean.2008.05.002
Wilson, C., Villareal, T. A., Maximenko, N., Bograd, S. J., Montoya, J. P., and Schoenbaechler, C. A. (2008). Biological and physical forcings of late summer chlorophyll blooms at 30°N in the oligotrophic Pacific. J. Mar. Syst. 69, 164–176. doi:10.1016/j.jmarsys.2005.09.018
Yamashita, H., Takahashi, K., and Fujitani, N. (2002). Zonal and vertical distribution of radiolarians in the western and central Equatorial Pacific in January 1999. Deep-Sea Res. II 49, 2823–2862. doi:10.1016%2FS0967-0645(02)00060-7
Ye, H. J., Sui, Y., Tang, D. L., and Afanasyev, Y. D. (2013). A subsurface chlorophyll a bloom induced by typhoon in the South China Sea. J. Mar. Syst. 128, 138–145.
Keywords: seamount, sinking particle, sediment trap, trace element, scavenging
Citation: Yamaoka K, Suzuki A, Tanaka Y, Suzumura M, Tsukasaki A, Shimamoto A, Fukuhara T, Matsui T, Kato S, Okamoto N and Igarashi Y (2020) Late Summer Peak and Scavenging-Dominant Metal Fluxes in Particulate Export Near a Seamount in the Western North Pacific Subtropical Gyre. Front. Earth Sci. 8:558823. doi: 10.3389/feart.2020.558823
Received: 04 May 2020; Accepted: 16 October 2020;
Published: 18 November 2020.
Edited by:
Makio Honda, Japan Agency for Marine-Earth Science and Technology (JAMSTEC), JapanReviewed by:
Jing Zhang, University of Toyama, JapanCopyright © 2020 Yamaoka, Suzuki, Tanaka, Suzumura, Tsukasaki, Shimamoto, Fukuhara, Kato, Okamoto and Igarashi. This is an open-access article distributed under the terms of the Creative Commons Attribution License (CC BY). The use, distribution or reproduction in other forums is permitted, provided the original author(s) and the copyright owner(s) are credited and that the original publication in this journal is cited, in accordance with accepted academic practice. No use, distribution or reproduction is permitted which does not comply with these terms.
*Correspondence: Kyoko Yamaoka, ay55YW1hb2thQGFpc3QuZ28uanA=
†Present address: Takaaki Matsui, Marine Biological Research Institute of Japan Co., Ltd., Tokyo, Japan
Disclaimer: All claims expressed in this article are solely those of the authors and do not necessarily represent those of their affiliated organizations, or those of the publisher, the editors and the reviewers. Any product that may be evaluated in this article or claim that may be made by its manufacturer is not guaranteed or endorsed by the publisher.
Research integrity at Frontiers
Learn more about the work of our research integrity team to safeguard the quality of each article we publish.