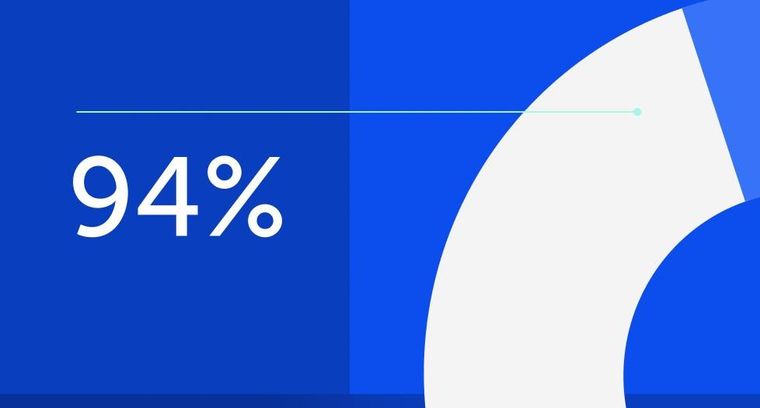
94% of researchers rate our articles as excellent or good
Learn more about the work of our research integrity team to safeguard the quality of each article we publish.
Find out more
ORIGINAL RESEARCH article
Front. Earth Sci., 04 September 2020
Sec. Solid Earth Geophysics
Volume 8 - 2020 | https://doi.org/10.3389/feart.2020.554924
This article is part of the Research TopicAchievements and New Frontiers in Research Oriented to Earthquake ForecastingView all 28 articles
Spatial distribution characteristics and origin of soil gasses were discussed in this study. We also examine the correlation between the spatial variation of soil gasses and fault activity, based on the measurements of Rn, Hg, H2, and CO2 concentrations in the eastern segment of the BLT fault zone in the southern Tianshan Mountains, Xinjiang, China. The results show that the Rn and CO2 concentrations on the hanging wall were higher than those near the fault zone and on the footwall of the fault. Moreover, the Hg and H2 concentrations on the footwall were also higher than those near the fault and on the hanging wall of the fault. The main factors affecting the variations in soil gas spatial distribution were gaseous origin structure of the crust, fault activity, fault fracture degree, stratigraphic lithology, and the cover layer. The results of active structural fault mapping show that the BLT fault has been active since the Late Quaternary and the thrust has broken the fault surface. Under the influence of tectonic compressive stress, the tension zone was formed on the hanging wall of the fault and fractures were developed. This result is consistent with the characteristics of soil gas concentrations measured in this study, indicating that the concentrations of Rn, Hg, H2, and CO2 are closely related to the fault activity. These findings can be applied to identify seismic precursors from gas monitoring data of the studied area.
A fault zone is a channel for deflation of the crust, and the number of micro-cracks distributed in the fault zone randomly increases under the impact of the tectonic stress field. This accelerates the migration and release of deep gasses (Baubron et al., 2002; Pulinets and Dunajecka, 2007; Fu et al., 2017), and changes the concentration of chemical components near the fault (Wiersberg and Erzinger, 2008). General overviews of the geochemical, structural, and seismic features in tectonically active areas have shown some evidence of a correlation between soil gas geochemistry anomalies and tectonic activities. Evidence also shows that soil gas escaping through the fault and fractures in the active fault zones can be enhanced by fault and earthquake activity (Fu et al., 2008; Sciarra et al., 2017; Yuce et al., 2017). As such, analyzing the geochemical variations of soil gas near fault zones has become an important method for investigating earthquake precursors, forecasting earthquakes, and evaluating fault activity (Li et al., 2013; Iovine et al., 2017).
Useful soil gasses include noble gasses, Rn, Hg, H2, CO2, and He, which play important roles in fault delineation and earthquake precursory studies (Chyi et al., 2005; Etiope et al., 2007; Caracausi and Paternoster, 2015; Camarda et al., 2016; Fu et al., 2017). Rn has been used by the scientific community as a tracer of natural phenomena related to outgassing from soil located along faults, fractures, and crustal discontinuities (King et al., 1996; Mazur et al., 1999). Rn concentration changes in soil gas and groundwater are commonly observed prior to earthquakes and volcanic eruptions; consequently, they have attracted considerable attention in studies of precursory geochemical signals (Morawska and Phillips, 1993; King et al., 1995; Giammanco et al., 2009; Walia et al., 2009; Iovine et al., 2017). Hg, H2, He, CO2, and CH4 have also been utilized in revealing the relationship among fluid activities in fault zones (Ware et al., 1984; Sugisaki et al., 1996; Jones et al., 2010; Moore and Castro, 2012). Helium isotopes are of particular interest as they can provide unequivocal evidence for the presence in the crust of mantle-derived fluids; 3He is essentially primordial and retained in the Earth’s interior, whereas 4He is mainly produced in the crust by the decay of U and Th. Hence, any 3He/4He ratio at the Earth’s surface larger than the local and crustal production rates indicates the presence of mantle helium (Sano et al., 2016; Buttitta et al., 2020).
Active faults commonly exhibit anomalously high concentrations of various terrestrially generated gasses in groundwater and soil air (King, 1980, 1986; Sun et al., 2017b). Concentration abnormalities of soil gas were observed in the Tashkent earthquake area in Uzbek, San Andreas Fault in the United States, Hsinhua and Chaochou faults in southern Taiwan, and Longmenshan fault, Beichuan-Yingxiu fault, and Minjiang fault in China (Engle et al., 2001; Li et al., 2006; Fu et al., 2008; Walia et al., 2009; Zhou et al., 2010, 2015). These studies indicate that the application of soil gas concentrations near the fault zone is of great practical significance for studying the fault activity and capturing the precursory information of earthquakes.
In this study, the concentrations of Rn, Hg, H2, and CO2 in soil gas were measured in the field along the profiles approximately perpendicular to the BLT fault. The spatial distribution in the soil gas concentrations in the eastern segment of the BLT fault was analyzed. The BLT fault is a long-term successional active fault zone and has been active since the Late Quaternary. It controls the distribution of the Mesozoic and Cenozoic strata in the northern Tarim Basin (Yao et al., 2018). This study is the first to analyze the distribution characteristics of soil gas in the BLT fault.
The BLT fault is the boundary between the Tarim Basin and the South Tianshan, oriented along the NWW–EW direction. The length of the fault is approximately 300 km, and the fault plane is N-dipping with an inclination of 50–80° (Figure 1). The BLT fault cuts the Late Pleistocene and Holocene alluvial fans and has been active since the Late Quaternary. It forms clear large-scale paleoseismic deformation zones and fault scarps with different heights on the surface. According to the records, only M5.6 earthquakes in 1972 and M5.2 earthquakes in 1988 occurred along the BLT fault, and no large destructive earthquake has ever occurred. In the present study, we examine the Tiemenguan section of the eastern part of the BLT fault (hereinafter referred to as Tiemenguan). As shown in Table 1, since 1970, 12 earthquakes have occurred near the Tiemenguan section, including two earthquakes above M5 and M4 earthquakes. No earthquakes occurred during the study period, Only two earthquakes occurred after 2015, M4.6 on April 1, 2018, and M4.4 on May 9, 2020 (Figure 1A). However, these two earthquakes did not occur on the BLT fault. There are multiple terraces where the fault is faulted and the height of fault scarps varies between 2 and 20 m. According to the results of trench profile images, there have been more than two paleoseismic events in the fault segment since the late Pleistocene. The vertical displacement of the stratum caused by the latest paleoseismic event is 1.1 m (Yao et al., 2018). The surface strata near the fault are bent and flexed, forming a large number of bending faults, and the crustal shortening rate of SN is 1.43–1.81 mm/a. Large-scale earthquakes have not occurred in the BLT fault since the earliest recorded history. This indicates the potential for a large-scale earthquake to occur in the future. At the fault, the surface accumulation material is mainly gravel, primarily composed of granite, and also comprises limestone. The fault is exposed on the surface, and the overburden is mostly composed of fine sand or sandy sedimentary layers.
Figure 1. Geologic structure map and seismic distribution of Tiemenguan section of BLT fault (a), spatial distribution of soil gas survey lines (b). The red circles indicate the earthquake that occurred after the measurement. SSD, Songshudaban fault; HLS, Huolashan fault; BLT, Beiluntai fault.
Table 1. Catalog of earthquakes occurring in the area near Tiemenguan from January 1, 1970 to May 31, 2020.
Figure 2 shows the schematic diagram for measuring Rn, Hg, CO2, and H2 concentrations. Before sampling, 0.8 m deep and 0.03 m wide holes were drilled in the soil at each sampling site. A pyramidal gas sampler was inserted into the hole after removing the drill bit. Before each measurement, the foreign gas in the samplers and in the rubber tube connecting the samplers and detector was removed prior to signal counting. The concentration of Rn in the soil gas was measured using AlphaGuard PQ 2000 PRO (AG) Radon Detector and the Hg concentrations were analyzed with portable RA-915+ Zeeman Mercury Analyzer. The error of measurement was 2 ng × m–3. The concentration of CO2 in the soil gas was measured using KG-3010E Portable Infrared CO2 Analyzer, and the concentration of H2 in the soil gas was measured by ATG-300H Portable Hydrogen Gauge with a detection limit of 0.01–10,000 ppm. In addition, five gas samples were collected following the drainage gas collection method at the fault zone for helium isotope (3He/4He) analysis. The container used for helium isotope detection was a 100 mL saline glass bottle. After the measurement of soil gas concentration, the gas in the sampler, connecting pipe, and air pump was removed using an air pump. The sampling container was cleaned with saturated saltwater three times, and then the gas was collected. The instrument used was MM5400 mass spectrometer with a sensitivity greater than 1.5 × 10–6 a/PA. The gas samples were sent to the Lanzhou Center for Oil and Gas Resources Research, Institute of Geology and Geophysics, Chinese Academy of Sciences, immediately after the collection. Analysis of the samples was completed within 30 days of sampling.
Figure 3A shows that the measured values of Rn concentrations tended to stabilize after the second value of the initial measurement and there was a small amplitude of fluctuation. At each measurement time, 30 readings were taken and analyzed using the boxplot method, yielding a characteristic value of Rn concentration in each measurement site. The other three components, soil gas concentrations of CO2, Hg, and H2 were recorded based on the measurement time and their maximum values were taken. The concentration of soil gas CO2 was relatively stable and the peaks were consistent with repeated measurements (Figure 3B). However, the concentrations of Hg and H2 showed a gradual increase in the measured values and upon reaching the highest value, the concentrations tended to stabilize (Figure 3C) or rapidly decrease (Figure 3D).
Figure 3. The measurement curve of soil gas concentration in different components. The Rn concentration was determined according to the boxplot method to obtain the characteristics of Rn concentration at each site. A total of 30 measurements were acquired for each measurement point (A). The other three components, soil gas concentration of CO2, Hg and H2 were recorded based on the measurement time, respectively. The peak of CO2 concentration was the same after repeated measurements (B), The Hg concentration value showed a steady change after rising to the highest value (C), H2 concentration characteristics showed a rapid decrease after rising to the highest value (D). Therefore, the maximum value of the gas concentration curve of each component at sampling sites is analyzed.
In this study, soil gas measurements were obtained twice across the fault at Tiemenguan, the eastern segment of the BLT fault, Xinjiang. The results are shown in Table 2. During the first phase, the measurements of soil gas Rn and CO2 concentrations were completed between April 20 and April 26, 2015. A total of 15 measuring points were identified from south to north. Measuring points 1–9 (P1–P9) were located at the footwall of the fault, and points 10 –15 (P10–P15) were located at the hanging wall of the fault. To determine whether there were similar changes in other gasses, we selected more measurement points at hanging wall (P13, P14, and P15), fault zone (P7, P8, and P10), and footwall (P2, P3, and P4) during the first phase from August 11–17, 2015, to conduct the second phase of soil gas measurements. The primary measurements recorded were the concentrations of Hg and H2 in soil gas. As shown in Figure 4, due to the characteristics of H2 (active) and Hg (adsorptive), such as the gas diffusion concentration that changes with time, the concentration of H2 rapidly increased to the highest value and then decreased, while the concentration of Hg gradually increased to the highest value and then tended to stabilize. The maximum values of Hg and H2 concentrations at each measuring point were obtained and the various characteristics of soil gas concentrations were analyzed.
Figure 4. Soil gas H2 (A) and Hg (B) concentrations at nine sampling sites across the BLT fault zone at Tiemenguan. Different colors represent the variation cures of H2 (A) and Hg (B) concentration of different measurement sites and the corresponding peaks.
As shown in Figure 5E, the terrain of the hanging wall of the fault is uplifted and that of the footwall is gentle. The survey line is vertical to the fault and extends approximately 2.5–3 km on both sides, with a total length of approximately 6 km. In addition, it should be noted that the study area is arid (i.e., precipitation and vegetation are not developed), with minimal soil moisture and stable temperature throughout the year, and the seasonal influence on soil gas concentrations is minimum in April and August.
Figure 5. Soil gas Concentrations of Rn (A), CO2 (B), Hg (C), and H2 (D) at Tiemenguan measuring point in BLT fault zone and distribution map of measuring points (E). The north side is hanging wall, topographic uplift, south side is footwall, the terrain is gentle.
The results show that in the footwall of the fault, the measured value of soil gas Rn concentration decreases toward the fault. It reaches the lowest value at the fault. However, in the hanging wall of the fault, the measured value increases toward the fault (Figure 5A). As shown in Figure 5B, the measured values of soil gas CO2 concentration in the footwall of the fault (measuring points 1–9) are generally low, and the measured values decrease toward the exterior of the fault (measuring points 11 to 15). The concentrations of Rn and CO2 are similar, and the measured values near the fault are high. The measured values gradually decrease with distance from the fault, and the concentration measured in the hanging wall of the fault is higher than that in the footwall of the fault.
The Hg concentration of soil gas was the lowest near the fault (P7, P8, and P10) and was relatively high in the two walls of the fault. The concentrations in the footwall (P2–P4) were approximately twice as high as that in the hanging wall (P13–P15) (Figure 5C). The H2 concentration of soil gas was higher in the footwall (P2–P4) and lower near the fault (P7, P8, and P10) and hanging wall (P13–P15) (Figure 5D).
In addition, helium isotope test results showed that the 3He/4He ratio ranges from 1.21 × 10–6 to 1.38 × 10–6, which is lower than that of air (1.4 × 10–6).
The measurement results show that the soil gas concentrations (Rn, CO2, Hg, and H2) in the Tiemenguan section in the BLT fault are different on either side of the fault zone. This may be closely related to the physical and chemical characteristics of the gas and its migration mechanism, the geological conditions of the measurement area, and the fracture development degree of the fracture zone.
The differences in soil gas concentrations are closely related to the distribution of fractures. The BLT fault is a thrust fault that is affected by the compressive force caused by regional tectonics. As shown in Figure 1, the bedrock type of BLT fault in the Tiemenguan section is primarily granite. Under regional tectonic compressive stress, a series of bending moment faults were formed in the hanging wall of the fault. Due to differences in the degrees of deformation in the hanging wall and footwall, their fractures exhibit different development degrees (Figure 6). As a result, the amount of deep gas escaping is different. In the hanging wall of the fault, under the regional tension stress environment, the surface at the top of the fault broke and formed a series of bending moment faults with many cracks. These bending moment faults are generally shallow fractures, allowing the gas in the soil to continuously escape to the atmosphere, which is not conducive to the enrichment of soil gas. On the other hand, the footwall topography, which is affected by compressive forces, is quite flat. The surface fractures are mainly closed or semi-closed, which is not suitable for the upward soil gas diffusion from the deep layers and its subsequent emission into the atmosphere. Thus, it is advantageous for the soil gas to be adsorbed on the particle surface of the rock. Then, it gradually accumulates in the soil. In the fault zone, the rock is highly fractured, and the fractures are further developed, which results in upward movement of the gas along the fracture.
The spatial variation characteristics of soil gas concentration are not only related to the number of fractures on the fault, but also to the rock types and overburden properties. For example, the surface sediments in the Tiemenguan section are primarily gravel and the rock types are mainly granite and limestone with high uranium and thorium content. This causes the soil gas Rn concentration in this section to be relatively high. Even if the rocks on either side of the fault are of the same type, different soil gas concentration characteristics will be formed if the overburden above the fault is different (Fu et al., 2005). As shown in Figure 6, there are differences in stratum type and overburden thickness between the two sides of the Tiemenguan fault. The hanging wall of the fault is covered with a thin layer of sandy soil; the fault is almost exposed to the surface, which results in the dilution of soil gas by air, and thus the soil gas concentration is low. The gravel layer in the footwall of the fault is comprised of weathered granite particles and medium coarse sand. The overburden layer is mainly sandy soil. It has strong gas sealing and adsorption properties. There are few channels available for the gas to escape and this results in a high gas concentration. This is reflected by the high concentrations of H2 and Hg in the footwall of the fault.
Hg is a heavy metal of great concern due to its extreme mobility and absorbability. Hg can be easily enriched in faults due to its special physical and chemical properties, and it can migrate from the interior to the surface along a fault or rock fracture due to fluid carrying or pressure gradient (Yangfen et al., 1989; Zhang et al., 2014; Sun et al., 2017a). H2 is a relatively active volatile substance and its diffusion speed is much higher than that of other gasses. It can easily migrate upward from the fault. The concentration of soil H2 in the fault zone is closely related to the internal structure of the active fault and the development degree of fracture (Wakita et al., 1980). The sources of H2 are as follows: microbial activity in shallow soil; deep water and abiogenic decomposition of CH4 (King, 1986); and chemical reaction between fresh rock fracture surfaces and water (Sugisaki and Mizutani, 1983). CO2 in the fault zone is usually a mixture of several source emissions. Surface biological activities or decomposition of organic matter may lead to increased CO2 concentrations. However, active fault zones can directly produce a large amount of CO2 and can also act as a channel to release deeply contained CO2 (Ciotoli et al., 2007; Chiodini et al., 2008). Rn – a radioactive but chemically inert gas – is constantly generated all over the earth, normally in minute amount by radium in crustal materials (King et al., 1995). The short half-life of 222Rn limits its diffusion in the soil; thus, the amount of radon measured at the ground surface cannot be released from a deep origin. Therefore, the concentration of Rn in soil gas is mainly affected by rocks containing radioactive elements U and Th. It also migrates from deep faults to the surface along with other carriers, such as CO2, N2, and CH4 (Etiope and Martinelli, 2002; Yang et al., 2005; Chyi et al., 2010). The small carrier velocity can cause a large change in the concentration of the surface.
He is an inert gas with a small specific gravity and strong permeability. He in nature is mainly comes from the atmosphere, crust, and mantle. The helium in the atmosphere is mainly composed of 4He, and the 3He/4He isotopic ratio is almost constant at 1.4 × 10–6. The crust is dominated by radioactive atoms in rocks and minerals, such as radiogenic helium, with the 3He/4He value of 2.0 × 10–8 and the primary helium in the mantle, with the 3He/4He values ranging from 1.1 × 10–5 to 1.4 × 10–5. R/Ra can represent the helium isotopic characteristics, where R is the 3He/4He ratio of the sample and Ra is the 3He/4He ratio of the atmosphere, i.e., 1.4 × 10–6. When R/Ra <1, it represents the characteristic of shell source helium, while R/Ra > 1 represents the characteristic of mantle source helium. As shown in Table 2, the 3He/4He ratio of P6–P10 ranges from 1.21 × 10–6 to 1.38 × 10–6. The calculation shows that R/Ra < 1 indicates that the helium in fault zone is formed from the crust; however, the 3He/4He ratio (1.21 × 10–6–1.38 × 10–6) at the fault zone is smaller than that in the atmosphere (1.4 × 10–6), which indicates that the helium from the crust has been diluted by atmospheric helium. The main causes of this effect are the U and Th contents in mineral rocks and the sealing characteristics of the system. The higher the content of U and Th in rocks, the more radioactive 4He was that accumulated, and the sealing property of the system declined. The addition of helium outside the system changed the helium isotope ratio. The U and Th contents of the rocks are closely related to the Rn concentration, and the sealing property of the system is closely related to the 4He. The addition of 4He outside the system changes the helium isotope ratio. Therefore, the helium isotope results confirm the conjecture of this study.
Based on the above four aspects, we present the results of this paper. There may be two distinct reasons for the obvious difference in soil gas concentrations on either side of the fracture zone: one is the difference between the overburden layers and the other may be related to the fracture distribution in the fracture zone and its vicinity. As shown in Figure 6, the strain across the thrust faults is contractional. It causes the hanging wall to be highly deformed near the fault at the surface, resulting in local bending and extensional fractures that facilitate the upward escape of soil gas. It is not conducive to the enrichment of gas in shallow soil. Conversely, the surface fractures in the footwall are mostly closed, which is not suitable for the release of soil gas into the atmosphere, thus leading to the accumulation of gas in shallow soil. Therefore, the greater development of fractures in the hanging wall provides a good channel for gas escape, and the deep gas carriers, such as CO2, can carry more Rn to the shallow surface and escape to the atmosphere. In the footwall, because there are fewer fractures, Rn in the deep soil layers cannot escape to the surface because of the short half-life period of 3.82 days and low migration rate; however, Hg and H2 can reach the surface. Moreover, it is easy to enrich the surface of soil particles on the shallow surface with fewer fractures. In comparison, there are relatively high concentrations of Rn and CO2 in the hanging wall (channel effect) and Hg and H2 in the footwall (enrichment).
We obtained measurements of soil gas Rn, Hg, H2, and CO2 concentrations from the eastern section of the Beiluntai fault zone in the southern Tianshan Mountains, Xinjiang, China. The geochemical distribution characteristics of the soil gas along the eastern section of the Beiluntai fault were obtained and the possible reasons for the difference in concentrations of soil gas components in the fault were discussed. The following conclusions were drawn:
1. The concentration of the soil gas in the eastern segment of the Beiluntai fault zone in the southern Tianshan Mountains of Xinjiang showed a significant difference on either side of the fault. The concentration of Rn and CO2 was higher on the hanging wall of the fault zone and the concentration of Hg and H2 was higher on the footwall of the fault zone.
2. The soil gas Rn, Hg, H2, and CO2 concentrations of the fault zone are closely related to the physical and chemical characteristics, geological structure, and fracture distribution of the fault zone.
The raw data supporting the conclusions of this article will be made available by the authors, without undue reservation, to any qualified researcher.
YX and XS wrote the manuscript. All the authors participated in field measurements.
This research was supported by the research grants (ZDJ2019-06 and ZDJ2017-27) from National Institute of Natural Hazards, MEMC (former Institute of Crustal Dynamics, China Earthquake Administration) and National Natural Science Foundation of China (41972253).
The authors declare that the research was conducted in the absence of any commercial or financial relationships that could be construed as a potential conflict of interest.
Baubron, J. C., Rigo, A., and Toutain, J. P. (2002). Soil gas profiles as a tool to characterise active tectonic areas: the Jaut Pass example (Pyrenees, France). Earth Planet. Sci. Lett. 196, 69–81. doi: 10.1016/s0012-821x(01)00596-9
Buttitta, D., Caracausi, A., Chiaraluce, L., Favara, R., and Sulli, A. (2020). Continental degassing of helium in an active tectonic setting (northern italy): the role of seismicity. Sci. Rep. 10:162.
Camarda, M., De Gregorio, S., Di Martino, R. M. R., and Favara, R. (2016). Temporal and spatial correlations between soil CO2 flux and crustal stress. J. Geophys. Res. Solid Earth 121, 7071–7085. doi: 10.1002/2016jb013297
Caracausi, A., and Paternoster, M. (2015). Radiogenic helium degassing and rock fracturing: a case study of the southern Apennines active tectonic region. J. Geophys. Res. Solid Earth 120, 2200–2211. doi: 10.1002/2014jb011462
Chiodini, G., Caliro, S., Cardellini, C., Avino, R., Granieri, D., and Schmidt, A. (2008). Carbon isotopic composition of soil CO2 efflux, a powerful method to discriminate different sources feeding soil CO2 degassing in volcanic-hydrothermal areas. Earth Planet. Scie. Lett. 274, 372–379. doi: 10.1016/j.epsl.2008.07.051
Chyi, L. L., Quick, T. J., Yang, F. T., and Chen, C. H. (2005). Soil gas radon spectra and earthquakes. Terrest., Atmosph. Oceanic Sci. 16, 763–774. doi: 10.3319/TAO.2005.16.4.763(GIG)
Chyi, L. L., Quick, T. J., Yang, T. F., and Chen, C. H. (2010). The experimental investigation of soil gas radon migration mechanisms and its implication in earthquake forecast. Geofluids 10, 556–563. doi: 10.1111/j.1468-8123.2010.00308.x
Ciotoli, G., Lombardi, S., and Annunziatellis, A. (2007). Geostatistical analysis of soil gas data in a high seismic intermontane basin: fucino Plain central Italy. J. Geophys. Res. 112:B05407.
Engle, M. A., Gustin, M. S., and Zhang, H. (2001). Quantifying natural source mercury emissions from the Ivanhoe Mining District, north-central Nevada, USA. Atmos. Environ. 35, 3987–3997. doi: 10.1016/s1352-2310(01)00184-4
Etiope, G., and Martinelli, G. (2002). Migration of carrier and trace gases in the geosphere: an overview. Phys. Earth Planet. Interiors 129, 185–204. doi: 10.1016/s0031-9201(01)00292-8
Etiope, G., Martinelli, G., Caracausi, A., and Italiano, F. (2007). Methane seeps and mud volcanoes in Italy: gas origin, fractionation and emission to the atmosphere. Geophys. Res. Lett. 34:L14303.
Fu, C. C., Yang, T. F., Du, J., Walia, V., Chen, Y. G., Liu, T. K., et al. (2008). Variations of helium and radon concentrations in soil gases from an active fault zone in southern Taiwan. Radiat. Measur. 43, S348–S352.
Fu, C. C., Yang, T. F., Tsai, M. C., Lee, L. C., Liu, T. K., Walia, V., et al. (2017). Exploring the relationship between soil degassing and seismic activity by continuous radon monitoring in the Longitudinal Valley of eastern Taiwan. Chem. Geol. 469, 163–175. doi: 10.1016/j.chemgeo.2016.12.042
Fu, C. C., Yang, T. F., Walia, V., and Chen, C. H. (2005). Reconnaissance of soil gas composition over the buried fault and fracture zone in Southern Taiwan. Geochem. J. 39, 427–439. doi: 10.2343/geochemj.39.427
Giammanco, S., Immè, G., Mangano, G., Morelli, D., and Neri, M. (2009). Comparison between different methodologies for detecting radon in soil along an active fault: the case of the Pernicana fault system, Mt. Etna (Italy). Appl. Radiat. Isot. 67, 178–185. doi: 10.1016/j.apradiso.2008.09.007
Iovine, G., Guagliardi, I., Bruno, C., Greco, R., Tallarico, A., Falcone, G., et al. (2017). Soil-gas radon anomalies in three study areas of Central-Northern Calabria, (Southern Italy). Nat. Hazards 91, 193–219.
Jones, L. C., Rosenbauer, R., Goldsmith, J. I., and Oze, C. (2010). Carbonate control of H2 and CH4 production in serpentinization systems at elevated P-Ts. Geophys. Res. Lett. 37, 381–389.
King, C. Y. (1980). Episodic radon changes in subsurface soil gas along active faults and possible relation to earthquakes. J. Geophys. Res. Solid Earth 85, 3065–3078. doi: 10.1029/jb085ib06p03065
King, C. Y. (1986). Gas geochemistry applied to earthquake prediction: an overview. J. Geophys. Res. Solid Earth 91, 12269–12281. doi: 10.1029/jb091ib12p12269
King, C. Y., King, B. S., Evans, W. C., and Zhang, W. (1996). Spatial radon anomalies on active faults in California. Appl. Geochem. 11, 497–510. doi: 10.1016/0883-2927(96)00003-0
King, C. Y., Koizumi, N., and Kitagawa, Y. (1995). Hydrogeochemical anomalies and the 1995 Kobe earthquake. Science 269, 38–40. doi: 10.1126/science.269.5220.38
Li, Y., Du, J. G., Wang, X., Zhou, X. C., Xie, C., and Cui, Y. J. (2013). Spatial variations of soil gas geochemistry in the tangshan Area of Northern China. Terrestr. Atmos. Ocean. Sci. 24, 323–332. doi: 10.3319/tao.2012.11.26.01(tt)
Li, Y. G., Chen, P., Cochran, E. S., Vidale, J. E., and Burdette, T. (2006). Seismic evidence for rock damage and healing on the San Andreas Fault associated with the 2004 M6.0 Parkfield earthquake. Bull. Seismol. Soc. Am. 96, S349–S363.
Mazur, D., Janik, M., Łoskiewicz, J., Olko, P., and Swakoń, J. (1999). Measurements of radon concentration in soil gas by CR-39 detectors. Radiat. Measur. 31, 295–300. doi: 10.1016/s1350-4487(99)00135-3
Moore, C. W., and Castro, M. S. (2012). Investigation of factors affecting gaseous mercury concentrations in soils. Sci. Total Environ. 419, 136–143. doi: 10.1016/j.scitotenv.2011.12.068
Morawska, L., and Phillips, C. R. (1993). Dependence of the radon emanation coefficient on radium distribution and internal structure of the material. Geochim. Cosmochim. Acta 57, 1783–1797. doi: 10.1016/0016-7037(93)90113-b
Pulinets, S. A., and Dunajecka, M. A. (2007). Specific variations of air temperature and relative humidity around the time of Michoacan earthquake M8.1 Sept. 19,1985 as a possible indicator of interaction between tectonic plates. Tectonophysics 431, 221–230. doi: 10.1016/j.tecto.2006.05.044
Sano, Y., Takahata, N., Kagoshima, T., Shibata, T., Onoue, T., and Zhao, D. (2016). Groundwater helium anomaly reflects strain change during the 2016 Kumamoto earthquake in Southwest Japan. Sci. Rep. 6:37939.
Sciarra, A., Cantucci, B., and Coltorti, M. (2017). Learning from soil gas change and isotopic signatures during 2012 Emilia seismic sequence. Sci. Rep. 7:14187.
Sugisaki, R., Ito, T., Nagamine, K., and Kawabe, I. (1996). Gas geochemical changes at mineral springs associated with the 1995 southern Hyogo earthquake (M = 7.2) Japan. Earth Planet. Sci. Lett. 139, 239–249. doi: 10.1016/0012-821x(96)00007-6
Sugisaki, R., and Mizutani, Y. (1983). Origin of hydrogen and carbon dioxide in fault gases and its relation to fault activity. J. Geol. 91, 239–258. doi: 10.1086/628769
Sun, X., Si, X., Xiang, Y., and Liu, D. (2017a). Soil mercury spatial variations in the fault zone and corresponding influence factors. Terrstr. Atmos. Ocean. Sci. 28, 283–294. doi: 10.3319/tao.2016.09.29.02
Sun, X., Yang, P., Xiang, Y., Si, X., and Liu, D. (2017b). Across-fault distributions of radon concentrations in soil gas for different tectonic environments. Geosci. J. 22, 227–239. doi: 10.1007/s12303-017-0028-2
Wakita, H., Nakamura, Y., Kita, I., Fujii, N., and Notsu, K. (1980). Hydrogen release: new indicator of fault activity. Science 210, 188–190. doi: 10.1126/science.210.4466.188
Walia, V., Yang, T. F., Hong, W. L., Lin, S. J., Fu, C. C., Wen, K. L., et al. (2009). Geochemical variation of soil–gas composition for fault trace and earthquake precursory studies along the Hsincheng fault in NW Taiwan. Appl. Radiat. Isot. 67, 1855–1863. doi: 10.1016/j.apradiso.2009.07.004
Ware, R. H., Roecken, C., and Wyss, M. (1984). The detection and interpretation of hydrogen in fault gases. Pure Appl. Geophys. 122, 392–402. doi: 10.1007/bf00874607
Wiersberg, T., and Erzinger, J. (2008). Origin and spatial distribution of gas at seismogenic depths of the San Andreas fault from drill-mud gas analysis. Appl. Geochem. 23, 1675–1690. doi: 10.1016/j.apgeochem.2008.01.012
Yang, T. F., Walia, V., Chyi, L. L., Fu, C. C., Chen, C. H., Liu, T. K., et al. (2005). Variations of soil radon and thoron concentrations in a fault zone and prospective earthquakes in SW Taiwan. Radiat. Measur. 40, 496–502. doi: 10.1016/j.radmeas.2005.05.017
Yangfen, J., Zonghua, W., Chunsheng, S., Jiazhen, W., and Hongren, Z. (1989). Earthquake prediction through the observation and measurement of mercury content variation in water. J. Geochem. Explorat. 33, 195–202. doi: 10.1016/0375-6742(89)90029-0
Yao, Y., Song, H. P., Chen, J. B., Li, S., and Jia, H. L. (2018). Late quaternary crustal shortening rate of the Beiluntai fault in southern. Seismol. Geol. 40, 71–86.
Yuce, G., Fu, C. C., D’Alessandro, W., Gulbay, A. H., Lai, C. W., Bellomo, S., et al. (2017). Geochemical characteristics of soil radon and carbon dioxide within the Dead Sea fault and Karasu fault in the Amik basin, (Hatay), Turkey. Chem. Geol. 469, 129–146. doi: 10.1016/j.chemgeo.2017.01.003
Zhang, L., Liu, Y., Guo, L., Yang, D., Fang, Z., Chen, T., et al. (2014). Isotope geochemistry of mercury and its relation to earthquake in the Wenchuan Earthquake Fault Scientific drilling project hole-1. (WFSD-1). Tectonophysics 619–620, 79–85. doi: 10.1016/j.tecto.2013.08.025
Zhou, X., Wang, W., Chen, Z., Yi, L., Liu, L., Xie, C., et al. (2015). Hot spring gas geochemistry in western sichuan province, China after the wenchuan ms 8.0 earthquake. Terr. Atmos. Ocean. Sci. 26, 361–373. doi: 10.3319/tao.2015.01.05.01(tt)
Keywords: BLT fault, soil gas, spatial distribution, thrust fault, Xinjiang
Citation: Xiang Y, Sun X, Liu D, Yan L, Wang B and Gao X (2020) Spatial Distribution of Rn, CO2, Hg, and H2 Concentrations in Soil Gas Across a Thrust Fault in Xinjiang, China. Front. Earth Sci. 8:554924. doi: 10.3389/feart.2020.554924
Received: 23 April 2020; Accepted: 14 August 2020;
Published: 04 September 2020.
Edited by:
Antonella Peresan, Istituto Nazionale di Oceanografia e di Geofisica Sperimentale (OGS), ItalyReviewed by:
Iren Adelina Moldovan, Institutul National de Cercetare si Dezvoltare pentru Fizica Pamantului, RomaniaCopyright © 2020 Xiang, Sun, Liu, Yan, Wang and Gao. This is an open-access article distributed under the terms of the Creative Commons Attribution License (CC BY). The use, distribution or reproduction in other forums is permitted, provided the original author(s) and the copyright owner(s) are credited and that the original publication in this journal is cited, in accordance with accepted academic practice. No use, distribution or reproduction is permitted which does not comply with these terms.
*Correspondence: Xiaolong Sun, eGxzdW4wNEAxNjMuY29t
†ORCID: Xiaolong Sun, orcid.org/0000-0003-0807-3784
Disclaimer: All claims expressed in this article are solely those of the authors and do not necessarily represent those of their affiliated organizations, or those of the publisher, the editors and the reviewers. Any product that may be evaluated in this article or claim that may be made by its manufacturer is not guaranteed or endorsed by the publisher.
Research integrity at Frontiers
Learn more about the work of our research integrity team to safeguard the quality of each article we publish.