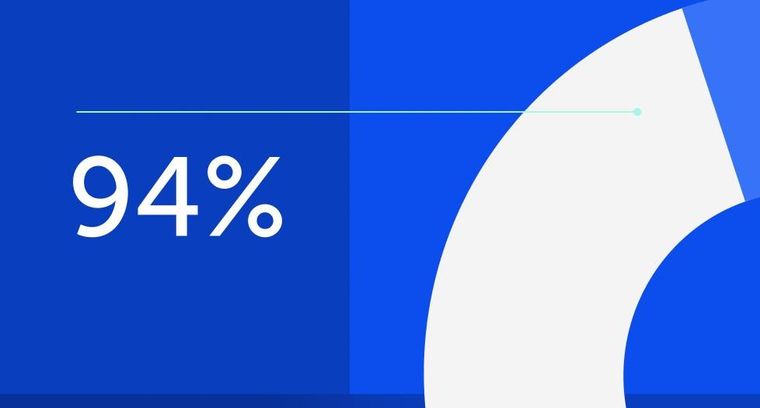
94% of researchers rate our articles as excellent or good
Learn more about the work of our research integrity team to safeguard the quality of each article we publish.
Find out more
REVIEW article
Front. Earth Sci., 30 October 2020
Sec. Petrology
Volume 8 - 2020 | https://doi.org/10.3389/feart.2020.535879
This article is part of the Research TopicUnusual Subduction ProcessesView all 11 articles
This paper briefly reviews the magmatic manifestations of volcanic arcs and aspects of how such magmas form. Common concepts about “how subduction zones work” are reassessed—with particular emphasis on the atypical Cascades volcanic arc. This arc is associated with one of the warmest modern subduction zones, with accelerated slab dehydration and low flux of slab-derived fluids compared to most others. With minimized leverage of fluids, other aspects of arc magmatism become more evident. We see multiple distinct basaltic magma types, all represented by relatively primitive high-MgO end members that allow assessment of their conditions of formation. Dominant types include two distinct intraplate varieties: low-K tholeiite with similarities to mid-ocean ridge basalts and another variety with affinities to many ocean island basalts. In addition, there is a spectrum of more typical calcalkalic basalts that range to fairly alkali-rich compositions (absarokites) as well as high-Mg basaltic andesites. These magma types are variably present throughout much of the arc and all are present coevally in a transect paralleling the Columbia River. Their complex spatial distributions may in part reflect along-strike differences in tectonic controls and/or dynamics of mantle convective flow. The Cascades arc is relatively broad, with significant basaltic volcanism locally in frontal and back arc regions. Prominence of basaltic magmatism has emerged since the late Miocene, and may be related to changes in the regional tectonic regime over time. These mafic magmas provide energy to drive melting in the crust, and perhaps in the lithospheric mantle, that feeds the more prominent stratovolcanoes distributed along the arc axis. Thermobarometry of the most primitive basalts implies unusual relations between depth and composition, as well as conflicting views regarding melt generation. Some traditional interpretations concerning 1) the conditions of magma formation, 2) the role of subducting oceanic plates (±sediments) in forming volcanic arc magmas, and 3) the nature of magmatic source domains in general appear to be inconsistent with current observations to some degree. These topics are reviewed and their implications assessed regarding physical and tectonic aspects of subduction zones.
It is well established that there is a causative relationship between ongoing subduction processes and volcanic arc magmatism (cf. Gill, 1981; Stern, 2002; Tatsumi, 2005). A common paradigm has emerged in which addition of subducted materials (including ocean crust, sediments, and oceanic mantle) in various ways contributes to the formation of arc magmas and significantly influences their compositions. In detail, magmatic outcomes may be determined by compositional variations in subducted materials from place to place or over time, and by varied physical aspects of the subduction process itself. For example, ages of the subducting plate(s), rates of subduction, and other tectonic factors vary widely, resulting in quite different thermal conditions from one subduction zone (SZ) to another (cf. Syracuse et al., 2010; van Keken et al., 2011; Maunder et al., 2019). Likewise, the upper plate can be influential in many ways. The character of its crust and lithospheric mantle also vary considerably from place to place depending on their prior history, leading to dramatic differences between continental and oceanic settings.
The nature of arc magmatism exhibits wide latitude in variability both between and within individual volcanic arcs (cf. Figure 1, showing the global distribution of active subduction). This diversity complicates our understanding of the interrelationships between influential factors and specific details of arc magmatism. Much effort has been expended to understand individual arcs, and global coverage has expanded greatly in recent years, revealing many complexities and enigmas. This paper revisits some basic tenets regarding manifestations and causes of arc magmatism, with dominant focus on formation of primitive arc magmas and their implications regarding subduction processes overall. In assessing familiar paradigms, the Cascades volcanic arc is discussed in some detail because it offers unusual perspectives.
FIGURE 1. Slab2 image showing global distribution of active subduction zones (Hayes et al., 2018). Contours indicate depth to slab surface and illustrate variations in slab geometry as defined by distributions of SZ seismicity. Major subduction zones are labeled as follows: Cascades (CAS), Aleutian (AL), Kamchatka (KAM), Kurile (KUR), Japan (JAP), Mariana (MAR), Izu-Bonin (IB), SW Japan (SWJ), Philippine (PH), New Britain (NB), Vanuatu (VAN), Tonga-Kermadec-New Zealand (TKNZ), Andes (AND), Central America (CA), Mexico (MX), Lesser Antilles (LA), South Sandwich (SSW), Indonesia (INDO), Hellenic (HEL), and Eolian (AOL) arcs. Continent-continent collision zones near India are not labeled.
To begin, I briefly outline some common generalizations regarding arc magmatism:
(1) Global studies of arc volcanoes suggest that arc magmas are commonly of “calcalkalic” (or “CA”) affinity (cf. Miyashiro, 1974; Le Maitre, 2002) and generally differ in important respects from typical magmas formed at mid-ocean ridge or oceanic island settings (so-called intraplate magmas). This dominant view (cf. Gill, 1981; Pearce, 1982) has often been invoked in assigning a tectonic setting to ancient igneous suites. But from today’s perspective of more detailed and systematic analytic investigations, considerable diversity between individual arc volcanoes is apparent and there can be multiple, essentially contemporaneous, magmatic series present at both the intra-volcano and intra-arc scales. This is strongly evident in the Cascades (cf. Leeman et al., 1990; Bacon et al., 1997; Conrey et al., 1997; Leeman et al., 2005) and Trans-Mexican arcs (cf. Righter, 2000) and elsewhere to varying degree. A major challenge is to understand the causes and significance of such diversity.
(2) CA type lavas tend to have distinctive geochemical characteristics. Notably, many exhibit variable enrichments in large-ion lithophile elements (LILE; e.g., Ba, Sr, K, Rb, and Cs) and fluid-mobile elements (FME; e.g., B, Pb, As, and Sb) whereas they are commonly depleted in high field-strength elements (HFSE; e.g., Nb, Ta, Zr, and Hf) relative to typical intraplate oceanic island basalts (OIB) and mid-ocean ridge basalts (MORB) (Sun and McDonough, 1989; Pearce and Peate, 1995; Leeman, 1996; Noll et al., 1996). These features are usually attributed to selective enrichment of arc magma sources by slab-derived LILE-enriched aqueous fluids or melts (cf. Pearce, 1982; McCulloch and Gamble, 1991; Plank, 2014). A link between FME enrichments and subducted slabs is convincingly demonstrated by strong correlations between B/Nb and similar ratios with enrichment of short-lived 10Be in arc lavas (Morris et al., 1990). Slab-derived transfer agents (aqueous fluids, melts, or supercritical fluids) commonly are thought to be liberated during progressive heating and dehydration of hydrous minerals within subducting plates or incorporated in melts of slab components such as sediments or altered oceanic crust (cf. Walowski et al., 2016; Cerpa et al., 2018; Zheng, 2019). Our understanding of mass transfer processes in SZs is in a state of flux—so to speak.
(3) Tectonic and geophysical studies also demonstrate wide variance in factors that likely influence magmatism. For example, differences in age and geologic nature of upper and lower (i.e., subducting) plates, in convergence rates, etc., are predicted to produce significant differences in thermal conditions within subducting slabs. Such differences are assumed to affect the dehydration, metamorphic, and perhaps melting behavior of subducted materials, hence the character and compositions of any fluids or melts extracted therefrom (cf. Spandler and Pirard, 2013; Bebout and Penniston-Dorland, 2015). The full impact of these processes are unclear.
(4) It is commonly believed that andesitic lavas are dominantly erupted in many if not most volcanic arcs, and that they are essential building blocks for continental crust—that itself is estimated to be generally “andesitic” in composition (cf. Rudnick and Gao, 2003). The origins of andesitic magmas are widely debated as discussed below. Most fundamental is the question of what types of magmas are produced in subduction environments (and where), and how and to what extent are they modified during ascent. It is also unclear what proportion of available magma is erupted vs. intruded into the mantle or crust. Thus, another basic question: how representative of deep processes are the erupted volcanic products in any given place?
To address such questions, it is essential to understand in greater detail what factors control magmatic diversity in subduction settings. This need has promoted diverse investigations including: 1) systematic petrologic and geochemical studies of arc volcanic products at scales ranging from whole rocks to minerals to melt inclusions (MIs), 2) experimental studies of melting and crystallization of relevant materials, 3) development of suitable geochemical tracers to quantify involvement of particular materials proposed to contribute to forming or modifying arc magmas, 4) acquisition of geophysical data to image the physical properties of SZs and the nature of the crust and mantle beneath volcanic arcs, and 5) theoretical studies and models to simulate physical and chemical conditions in such settings. Many attempts have been made to define the dynamics of SZs, and to develop a global perspective of how they work.
A critical concensus is that thermal structures of SZ systems vary considerably worldwide and even along-strike in local areas (Syracuse et al., 2010). We can view them as a spectrum (Figure 2) ranging from cold to hot in terms of the predicted maximum slab-surface temperature at depths below the volcanic front (defined as the alignment of the most proximal volcanoes to the modern trench). This convenient reference temperature does not fully correspond to actual temperature distributions throughout the upper and subducting plates as these may be influenced by other factors. But it carries implications regarding the dehydration history of the subducting plate and, indirectly, the potential availability and nature of slab-derived fluids and likelihood of partial melting of the subducted slab. Such models can be tested against known metamorphic conditions in exhumed subduction complexes to evaluate how well they reproduce thermal conditions within subducting plates. Because predicted temperatures do not always closely reproduce estimates based on mineral assemblages or mineral thermometry (cf. Penniston-Dorland et al., 2015), it is clear that the physical and conceptual models can be improved. Similarly, temperature and pressure estimates based on volcanic rocks (cf. Lee et al., 2009; Perrin et al., 2016) can theoretically be used to constrain temperature distributions below volcanic arcs. At this point, there remain issues to resolve to improve such estimates (cf. Herzberg and Asimow, 2015; Matzen et al., 2017; Till, 2017). Most importantly, are the estimated conditions of melt equilibration with the mantle representative of the conditions at which melting occur, or simply points along much longer ascent paths? Also, how does the nature of the source (e.g., proportions of lherzolite vs. pyroxenite, overall fertility, etc.) affect actual melting, as well as the accuracy of estimates for melt equilibration conditions? Theoretical models provide some sense of the relative differences in the thermal structures between subduction systems, and these have been compared with compositional features of arc lavas (e.g., water content) to evaluate how they may be linked to temperature (cf. van Keken et al., 2011; Cooper et al., 2012; Ruscitto et al., 2012). They can also be compared with geophysical observations (e.g., Wannamaker et al., 2014; McGary et al., 2014) that are sensitive to thermal signatures for independent assessment of their validity.
FIGURE 2. Depth profiles of slab-surface temperature are shown for global subduction zones (gray shaded field for most) based on numerical models of Syracuse et al. (2010), assuming full coupling between slab and mantle to a depth of 80 km. Extrema are shown for the coolest (Tonga) and hottest (Cascadia) SZs. The upper stability limit of serpentine is shown by the “serp-out” curve (Ulmer and Trommsdorff, 1995), and wet-melting solidus curves are shown for oceanic crust (“OC”; Vielzeuf and Schmidt, 2001) and marine sediments (“Sed”; Poli and Schmidt, 2001; Hermann and Spandler, 2007). For wet-melting of mantle peridotite, two solidus curves are shown owing to unresolved controversy between Grove et al. (2012; lower T solidus*) and Green et al. (2014, higher T solidus#); both are substantially cooler than the dry peridotite solidus (∼1,450°C at 100 km depth; Hirschmann, 2000). At sub-arc depths, the hot Cascadia slab is expected to have undergone significant dehydration. If water is present (e.g., from the slab interior), the ocean crust and sediment portions of the slab are likely to partially melt, as might deep parts of the overlying mantle wedge. At similar depths (ca. 100 km) beneath most other SZs, the slabs are likely to retain a significant part of their water inventory and in many cases be too cool to undergo partial melting. Whether or not the lowermost mantle wedge can produce hydrous melts depends on which wet-solidus curve is applicable, and on the manner in which slab-derived fluids or melts are transferred into the mantle wedge (cf. Cerpa et al., 2018).
One important insight is that a greater diversity of magma types is seen in hot arcs compared to cold arcs (cf. Leeman et al., 2005; Kimura et al., 2014). Also, a larger proportion of primitive basaltic lavas erupt in hot arcs compared to cold arcs (Schmidt and Jagoutz, 2017). Is this a consequence of lesser input of slab-derived fluids beneath hot arcs (cf. Ruscitto et al., 2012)? And does a stronger slab-fluid input beneath cooler arcs somehow dominate the production of particular magma types, or more strongly overprint the magma sources, and thereby skew the compositional distribution of erupted magmas? Conversely, does slab-melting play a more significant role where subducted slabs are anomalously hot (cf. Yogodzinski et al., 2015; Walowski et al., 2016)? Such factors potentially bias the estimated composition of magmas from one arc to another. For example, is the perceived dominance of hydrous magmas in arcs a consequence of the fact that most arcs are on the cooler side of the thermal spectrum? At the least, there must be varied magmatic responses to this range of thermal conditions.
Another possible bias relates to the fact that, in many arcs, primitive magmas with >7–8 wt% MgO are rare to absent whereas they are relatively common in some arcs, like the Cascades (cf. Schmidt and Jagoutz, 2017; Pitcher and Kent, 2019). This is an important issue, in that the nature of the upper plate (e.g., continental vs. oceanic) can influence the ascent of a given magma, and may determine whether or not it can actually reach the surface and erupt, or the degree to which it evolves from its initial composition. This hinders our ability to accurately assess the nature of the magma sources because lower-MgO magmas almost certainly have undergone significant degrees of magmatic differentiation and/or interaction with wall rocks (and possibly older stored magmas) during their ascent. Where magmas considered to be primitive do erupt, their calculated eruptive temperatures are generally high (Leeman et al., 2005; Schmidt and Jagoutz, 2017). Projected to realistic mantle equilibrium depths, they commonly approach or even exceed the dry solidus for typical mantle lithologies (see below). This observation implies that some primitive arc basaltic magmas form under lower H2O water conditions, as opposed to the prevalent view that many/most are products of flux-melting and are water-rich (Cooper et al., 2012; Grove et al., 2012). The interplay of temperature and H2O fundamentally controls mantle melting (cf. Portnyagin et al., 2007).
If this view is correct, it implies that the role of slab-derived fluids must be carefully assessed in terms of their effect on arc magma production. For example, if slab-derived fluids or melts are invoked to account for enrichment of LILE components in an arc magma source, this could occur over long time periods rather than in a single stage associated with the active generation of a given melt. In such cases, source modification could be a multi-stage process and not require large spikes of fluid to catalyze melt production. Importantly, the occurrence of intra-plate type magmas in arcs demonstrates that either 1) the mantle source domains cannot be pervasively modified by SC infiltration or 2) that multiple distinct source domains must be tapped—in which case, how are they distributed and how do the respective melts remain distinctive during extraction and migration? Finally, if source modification does occur over protracted time intervals, it is also possible that the fluids or melts involved could vary in composition over time.
A prime example of this point is the occurrence of so-called absarokite-shoshonite-banakite series lavas that are extremely enriched in K2O and LILE. Such lavas are found in some modern arcs (e.g., Fiji-Vanuatu, Marianas, Cascades, Mexico), and also in extensional settings (e.g., Turkey, American Rocky Mountains). In Vanuatu and Fiji, these unusual lavas erupted during the rift stage of the Vitiaz arc, several Ma after the active peak of subduction (Peate et al., 1997; Leslie et al., 2009). In Anatolia, examples of such magmas occur in currently extensional settings, but that had experienced subduction in the past, and thus appear to involve mantle domains that could have been modified or “refertilized” long before (cf. Coban et al., 2019). Finally, such lavas associated with intra-arc rifting in the northern Mariana arc are interpreted to be melts of previously enriched upper mantle domains that experienced decompression melting at the onset of extension within the arc (Ishizuka et al., 2010). These examples are consistent with the idea that parts of the lithospheric (?) mantle can retain a cumulative record of prior subduction-related magmatism, and that rejuvenated melting of such domains can produce magmas having geochemical similarities to those in active subduction settings.
Here, I briefly address the nature of the crust and mantle at depth. For mantle-derived magmas this could pertain to lithospheric mantle (i.e., in the case of SZs, a preserved remnant of the upper plate that has not been actively involved in mantle convection), or to deeper asthenospheric mantle that may be undergoing convective flow in association with subduction tectonics. Such mantle domains presumably impart a compositional signature on primitive arc magmas that reflects the time-integrated history of said domains.
The crustal section is likewise important in two significant ways. First, it comprises a potentially complex geologic section with which ascending or stored magmas can interact. Such interactions include assimilation, chemical reaction, and partial melting, each of which can modify the original composition of ascending magmas and thereby obscure their primary nature (e.g., type of mantle source). Second, the nature of the crustal section can influence magma ascent dynamics. Assuming that magma rise is driven primarily by its buoyancy relative to surrounding or overlying crustal rocks, efficiency of ascent is inversely related to overburden density. Ascent and eruption of basaltic magmas is inherently more efficient in oceanic settings where the crust on average is denser than the magma. Conversely, the comparatively lower average density of continental crust does not favor easy ascent of basaltic magmas, as they are prone to stagnate and intrude at depths (lower to middle crust?) where neutral buoyancy is attained. In the latter case, mantle-derived mafic magmas are likely to interact with crustal wallrocks (or earlier stored magmas) and more likely to undergo significant compositional modification due to magmatic differentiation and assimilation processes (cf. Annen et al., 2006). A seminal example of these relations is provided by the southern Chilean Andes (cf. Hildreth and Moorbath, 1988), where along-strike crustal thickness varies more than two-fold from >60 km in the north to ∼30 km in the south. Notably, 1) Quaternary mafic volcanism is most pronounced in the south, whereas there are no true basaltic lavas in the north and 2) compositions of the most mafic lavas reflect progressively greater “crustal” input toward the north.
Intra-oceanic arcs (e.g., Tonga, Marianas, South Sandwich, western Aleutians) are built upon essentially oceanic basement that has a comparatively simple geologic history and restricted compositional range–similar to that produced at mid-ocean ridges. However, in such areas complications sometimes arise where oceanic plateaux are involved (e.g., near New Britain; Woodhead et al., 1998). Such basement is presumed to have formed in part over an upwelling mantle plume, and therefore presumably adds an additional OIB-like component. Moreover, later post-subduction magmatism may reactivate relict mantle wedge lithosphere (e.g., as in Fiji and some parts of the Izu arc). Remelting of such mantle has been proposed to explain the origin of certain alkalic lavas within these arcs (as noted above).
It is useful to reflect on what a volcanic arc actually is. Modern volcanic arcs are evident along plate margins inboard of active SZs, where usually oceanic lithospheric plates are being thrust beneath other oceanic or continental plates or fragments thereof. Volcanic arcs typically comprise a chain or belt of young volcanoes oriented more or less parallel to the strike of the SZ; in detail they can be more complex in structure. Overall, erupted products commonly comprise a compositional spectrum including basalt, andesite, and dacite ± rhyolite—often with a preponderance of intermediate composition products. Leeman (1983) observed that the median composition of arc lavas from global localities varied rather systematically with the thickness of the underlying arc crust; this observation is well confirmed by the current vastly larger global database (cf. Farner and Lee, 2017). Median SiO2 content is lowest (dominantly basaltic) in oceanic arcs, and generally higher (andesitic or more evolved) in continental margin arcs. And, on an intra-arc scale, SiO2 content and a variety of other compositional parameters of recent volcanoes clearly vary with crustal thickness. Analogous and more global studies (e.g., Plank and Langmuir, 1988) have repeatedly shown that crustal processes appear to exert strong control on the nature of erupted volcanic material.
Implicitly, there is a complementary, but generally inaccessible, intrusive component to arc volcanism (cf. DeBari and Greene, 2011; Kelemen and Behn, 2016; Mullen et al., 2018). Clearly, significant fractions of arc magma stagnate within the crust and gradually modify its overall composition as well as impact its thermal structure (cf. Karlstrom et al., 2015; Rees-Jones et al., 2018). There are myriad ways that ascending magmas can be modified by combinations of crystallization, mixing with other stored magmas or partial melts of crustal rocks, etc.—the mixing, assimilation, storage, and hybridization (MASH) process (cf. Murphy et al., 2000; Annen et al., 2006; Hildreth, 2007; Solano et al., 2012). These processes tend to obscure the compositions of primitive magmas formed deep in the mantle. Thus, many investigations have focused on what exactly is the nature of the most primitive magmas in volcanic arcs (cf. Schmidt and Jagoutz, 2017). This is extremely important because it bears on where and how parental arc magmas form as well as on the nature of their source rocks (see later discussion).
Andesitic rocks are common in many volcanic arcs (especially continental ones), and have been considered characteristic of arc volcanism (e.g., Gill, 1981). Kelemen et al. (2003) summarize arguments favoring the origin of so-called “high-Mg andesites” (HMA, or adakites) as direct melts of mantle lithologies, and it is well known that hydrous melting of mafic compositions (e.g., subducted oceanic crust; Beard and Lofgren, 1991; Rapp and Watson, 1995; Grove et al., 2003; Sisson and Kelemen, 2018) can produce a variety of intermediate to silicic melts depending on water availability. However, the concept that andesitic magmas form by direct melting of ultramafic mantle is questionable for several reasons. First, although “andesitic” lavas and intrusive rocks happen to be common (Gómez-Tuana et al., 2013; Kent, 2013), the occurrence of natural andesitic magmas (actual liquids) is extremely rare (cf. Reubi and Blundy, 2009), as are quenched glasses (e.g., in the form of MIs in phenocryst minerals; Naumov et al., 1997; Zhu et al., 2013). In contrast, many investigations of andesitic rocks have shown them to be products of mixing between more mafic and more silicic liquids ± other crystalline components (cf. Anderson, 1976; Tatsumi, 2005; Humphreys et al., 2013; Kent, 2013; Sisson et al., 2014). An important example is (adakitic) HMA from near Mt. Shasta in the Cascade arc—considered to be a near-primary magma by Grove et al. (2002, 2003), or a mixture of mantle-derived basaltic andesite and high-Mg andesite (Ruscitto et al., 2011). These interpretations are questioned by Streck and Leeman (2018), who show this lava to be a hybrid mixture of basaltic and dacitic magmas plus ultramafic xenocrysts, and unlikely to be a direct mantle melt. Also, early views that a magmatic continuum can develop dominantly via fractional crystallization have now shifted to include the likelihood that there is an essentially bimodal distribution of mafic (basaltic) and silicic (dacitic-rhyolitic depending on the nature of the local arc crust) magma populations in most volcanic arcs, and that these to varied extent mix and interact with country rocks to form the observed spectrum of arc magmas (cf. Reubi and Blundy, 2009; Kent, 2013; Blum-Oeste and Wörner, 2016; Beier et al., 2017; Conway et al., 2020).
An increasingly prevalent view is that arc magmatism is driven primarily by ascent of mantle-derived basaltic magmas that 1) interact with pre-existing crustal rocks to form silicic partial melts (e.g., dacite, rhyolite), 2) undergo stagnation largely at crustal depths where mixing and contamination may occur, and 3) undergo fractional crystallization to produce mafic to ultramafic cumulate lenses during eventual solidification. To reconcile the “andesitic” nature of continental crust vs. the inferred basaltic nature of mantle inputs, it is necessary to remove large portions of the latter mafic cumulate component. This may be accomplished by delamination and foundering of lower crust segments containing relatively dense cumulate materials (cf. Kelemen et al., 2003; Tatsumi, 2005), or by “relamination” or underplating by new relatively evolved magmas (Kelemen and Behn, 2016).
The exact nature of mafic magmas in volcanic arcs may be to some extent masked by the effects of the pre-existing crust as a density filter. This is manifest by the skewness in magma compositions relative to crustal type and thickness noted above. A notable example is Mount St. Helens, in the Washington Cascades (cf. Smith and Leeman, 1993; Leeman and Smith, 2018). There, the prevalent erupted magmas are dacitic with subordinate andesite. Basaltic magmas of two distinct types have erupted only during a brief period (∼300 years), but their presence is required over the 300 ka lifetime of the volcano as a mixing component to produce observed compositional trends in the more evolved intermediate composition rocks, and as a heat source to drive crustal level processes—including partial melting of crustal rocks and stabilization of shallow magma reservoirs.
Many factors have been invoked to account for differences between arc vs. oceanic intraplate magma suites. Examples include the perceived roles of oxidation and/or water content, both of which are inferred to be generally greater in arc magma suites than in intraplate magmas. It is widely accepted that the calcalkalic (CA) magma series forms under relatively oxidizing conditions that promote crystallization of magnetite in addition to other liquidus phases (olivine, plagioclase, pyroxenes, etc.) so as to minimize Fe-enrichment in evolving magmas. Conversely, repression of magnetite as a crystallizing phase allows evolving magmas to generate a more iron-rich tholeiitic (TH) magma series. The presence of magnetite as a liquidus phase is widely attributed to whether redox conditions in the magma are 1) significantly above vs. 2) near or below the quartz-fayalite-magnetite (QFM) redox buffer (cf. Blatter et al., 2013). Notably, both magma series can be found in many volcanic arcs (e.g., Japan, Cascades). The CA series seemingly requires more oxidized conditions in the magmas that implicitly arise owing to inputs from subducted materials—notably sediment melts and aqueous fluids (cf. Kelley et al., 2010). However, considering redox sensitive indicators like V/Sc or Zn/Fe elemental ratios (Lee et al., 2005; Lee et al., 2010), it appears that at least the most primitive basalts in the CA series from the Cascades and several other arcs are no more oxidized than typical intraplate basalts (ocean islands, mid-ocean ridges) that have essentially no apparent subduction relationship. Thus, to the extent that CA compositional trends in arc lava suites actually can be replicated by mixing between basaltic and more evolved magmas (cf. Eichelberger et al., 2006), the inferred redox differences may be artifacts of processes that occur after formation of primary magmas—and thus may not necessarily reflect source differences. The stage at which arc magmas acquire their “oxidized signature” remains a topic of contention (cf. Kelley and Cottrell, 2009; Rowe et al., 2009; Walowski et al., 2015).
Water contents are elevated in many arc lavas relative to most intraplate lavas of similar bulk composition (cf. Wallace, 2005; Zimmer et al., 2010; Ruscitto et al., 2012). This is usually attributed to high water contents in the magma sources, that in turn can be explained by influx of aqueous fluids or hydrous melts derived from water-rich subducted materials (cf. Schmidt and Poli, 1998; van Keken et al., 2011). In evaluating direct measurements of magmatic water content, it is noteworthy that the solubility of water in silicate melts is pressure and temperature dependent. This means that if ascending magmas become water-saturated, any further rise will result in partial losses of water vapor. Thus, the only potential direct record of water content is in MIs trapped at high pressures in phenocrystic minerals, olivine usually being the first to form in mafic magmas. The extent to which ascending magmas have outgassed prior to phenocryst formation is generally unknown. Also, many published water determinations are for olivine-hosted MIs that are variably evolved from primitive compositions, and often the host olivines are less magnesian than expected for mantle source compositions (i.e., ∼Fo90 or higher). Thus, “reverse fractionation” corrections (e.g., addition of olivine) commonly are applied to the studied samples to approximate compositions of primary mantle melts. Measured water and incompatible element contents are thus diluted to some extent relative to the analyzed values (cf. Schmidt and Jagoutz, 2017). Adjusted MI water contents of ∼4 wt% are typical for basaltic liquids from most arcs, and slightly lower (∼3 wt%) for the Cascades arc (Ruscitto et al., 2012; Plank et al., 2013; Walowski et al., 2015). However, detailed studies of MIs from the Cascades suggest that measured water content varies systematically with magma type, and also may vary according to along-strike position in the arc; this is revisited later in the paper.
A major question concerns the timing and place where arc magmas acquire their water (and other volatile) contents. Presumably, the water is largely derived from dehydration of subducted materials. In a flux-melting scenario (cf. Cooper et al., 2010), water released from subducting slabs lowers the mantle solidus and promotes hydrous melting at near-slab depths (≥∼80 km). Such models are supported by the occurrence in some arc magmas (cf. discussion of the Tonga-Kermadec-New Zealand arc by Leeman et al., 2017) of short-lived radioisotopes (e.g., 10Be; excess 238U, 226Ra) that must be recently added to arc magma sources, as well as fluid-mobile elements (FMEs; B, Pb, As, Sb, etc.), all of which are likely slab-derived to some degree. Grove and Till (2019) review such melting scenarios, and discuss how such magmas may evolve during ascent, first through an upward-increasing temperature gradient in the lower mantle wedge, then through an upward-decreasing gradient in the uppermost mantle. Clearly, such an ascent path provides myriad possibilities of magma modification including interaction with multiple mantle wall rocks (including previously stagnated melt columns or intrusive bodies).
An alternative scenario involves adiabatic melting of upwelling asthenospheric (?) mantle material, likely derived from back-arc regions (cf. Long et al., 2012; Turner and Langmuir, 2015; Perrin et al., 2018), but also potentially mantle rising through tears or holes in the subducting slab (cf. Ferrari et al., 2012; Mullen and Weis, 2013). In this case, the types of melts produced and overall melt productivity are influenced by heterogeneities in the upwelling mantle (cf. Turner et al., 2017) and by the thermal structure of the mantle wedge beneath a given arc (cf. Turner et al., 2016). A third potential source of some arc magmas, that may be decoupled from direct slab inputs, is lithospheric mantle. Such domains may have a complex history, including prior melting events and/or modifications from earlier fluid and melt infiltrations as noted above. Modeling by Rees-Jones et al. (2018) suggests that lithospheric mantle temperatures can be elevated by several hundred degrees at depths as shallow as 60 km in response to heat transfer by magma migration over extended time. Such mantle domains may contribute significantly to arc magma production, particularly in continental margin settings where the lithosphere is likely to be thick and have a distinctive composition (cf. Pearce, 1982).
Corresponding magmatic liquid temperatures calculated (Lee et al., 2009; Perrin et al., 2016) for many primitive arc basaltic magmas are at most about 100°C below solidus conditions for dry peridotite (e.g., ∼1,450°C at 3.0 GPa; cf. Hirschmann, 2000). Estimated magma P–T conditions that fall below the dry solidus require the presence of some water in the magma source (cf. Katz et al., 2003; Green et al., 2014). The amount required depends on ∆T below the solidus (cf. Portnyagin et al., 2007). In contrast, where temperatures above the dry solidus are predicted, source H2O content may be negligibly small. These relationships have not been widely incorporated into geodynamic models for SZs, but they potentially provide useful constraints on depths of primary melt generation. In flux-melting scenarios, where near-slab melts might initially form at temperatures below 900°C (cf. Grove et al., 2012), there must be significant heating and re-equilibration of the melt column to produce the erupted magmas (cf. Mallik et al., 2016), and this may occur as low temperature melts ascend through the inverted thermal gradient in the mantle wedge. This introduces considerable uncertainty regarding which initial parameters (including slab-derived tracers) the final melts actually retain vs. what are likely to be significant modifications during a tortuous ascent. It is uncertain what fraction of the near-slab melt actually persists relative to melt increments added in the process of reaching the highest estimated mantle equilibration temperatures (possibly ≥600°C heating based on thermobarometry cited above). Conversely, adiabatic melting also seems capable of reproducing the thermobarometric estimates, but leaves open the question of how such sources acquire the geochemical signatures attributed to SC inputs. And if non-convecting lithospheric mantle is a significant magma source, reactivated via intense rejuvenated magma flux from below, it is possible that slab geochemical signatures could be cumulative over time, and even partly related to earlier magmas in the rejuvenating pulse. Realistically, all of these general processes could be involved in arc magmatism. What is especially puzzling is how the observed diversity of magmas in some arcs can be produced, often in single volcanoes and for restricted intra-arc areas. Not only does this seem to dictate that there are significant small-scale heterogeneities in source domains, but that physical processes of magma segregation and ascent must operate on small spatial scales.
Geochemical characteristics have been utilized in creative ways to address diversity of arc magmas as well as to identify unique features that can discriminate among potential contributions to their formation. Based on earlier discussion, such approaches are best applied to the most primitive lavas that are available–ideally basaltic lavas with ≥7–8 wt% MgO for which nominal equilibrium olivine composition approaches Fo90 or higher, which would imply that said magmas could have been in equilibrium with typical peridotitic or pyroxenitic mantle lithologies (cf. Leeman et al., 2005; Herzberg and Asimow, 2015). Where such primitive basalts are rare or absent (e.g., central Andes, portions of western Pacific arcs), primary source characteristics may not be faithfully retained in available samples. Schmidt and Jagoutz (2017) have attempted to assess variations in “primitive” arc magmas on a global scale; interestingly, from the enormous GEOROC volcanic arc database, only 938 samples from 30 arcs passed their filter—primarily that Mg#s are consistent with near-equilibration with mantle olivine compositions. They recognize at least six distinct magma types, two of which are varieties of mafic andesites, and observe that most arcs have more than one melt type—this is attributed to heterogeneity in the mantle wedge coupled with additional contributions of varied slab components. Below, I review in greater detail the diversity of primitive magmas in the Cascade arc, and note that the picture there is more complex.
In assessing overall geochemistry, two fundamentally different groups of elements are considered: 1) those that are incompatible in dominant mineral phases in the mantle and in common phenocrysts in crystallizing magmas, and 2) all of the rest, for which magmatic relative abundances can be altered during melting or crystallization processes that involve physical separation of liquids, solid phases, and/or fluids. The highly incompatible elements (e.g., LILE, FME) are of greatest utility in assessing source compositions as their elemental ratios are expected to be little affected by either melt formation or crystallization processes during magma ascent. Of course, such ratios can be modified by open system processes such as assimilation of distinct country rocks or melts thereof, or by mixing with other distinct magma types. Theoretically, it can be expected that a primitive mantle-derived basaltic magma that ascended directly to the surface will retain an incompatible element record characteristic of its mantle source. Much the same is true regarding isotopic ratios of elements such as B, Sr, Nd, Pb, Hf, etc., that are unchanged by normal closed-system magmatic processes (cf. Ryan and Chauvel, 2014).
Locally, there are significant spatial variations in the geochemistry of arc basalts. For example, lavas from most cross-arc transects display increasing proportions of fluid-mobile elements approaching the arc front (trenchward), that are suggestive of progressively decreasing contributions of slab-derived fluids with increasing depth along the subducting slabs (Woodhead et al., 1993; Noll et al., 1996; Elliot et al., 1997; Tonarini et al., 2001). These trends are consistent with predicted fluxes of water released from progressively dehydrated slabs (van Keken et al., 2011). Similarly, along-strike variations in some arcs (e.g., Tonga-Kermadec-New Zealand; Leeman et al., 2017) appear to reflect lateral changes in overall slab thermal structure or volumes of subducted material corresponding to changes in age or convergence rate of the subducting plate.
Superimposed on such variations, is the surprising occurrence of two or more distinct basalt variants within local arc regions, and in some cases at a single volcanic center or stratovolcano (e.g., Reagan and Gill, 1989; Leeman et al., 1990; Bacon et al., 1997; Kimura et al., 2002; Leeman et al., 2005; Hickey-Vargas et al., 2016; Rawson et al., 2016; many others). Such examples imply that the sub-arc mantle cannot be considered to be compositionally homogeneous. An obvious question concerns the extent to which inherent heterogeneities in the sub-arc mantle can be masked or effectively overprinted by progressive infiltration of slab-derived fluids and/or melts. And, to what extent are such effects related to the thermal conditions (hence, dehydration history or melting) in local subducting slabs?
Slab-derived fluid contributions are expected to be greatest in cooler SZs typically associated with the majority of modern volcanic arcs. It has been proposed that slab-derived melt contributions might arise from diapiric upwelling of mélange material that then undergoes decompression melting, possibly enhanced by fluxing due to any entrained aqueous fluid (cf. Gerya and Stöckhert, 2006; Behn et al., 2011; Marschall and Schumacher, 2012; Codillo et al., 2018). Also, availability of slab-derived fluids is likely to be strongly enhanced in places where fracture zones in the downgoing plate are subducted (Singer et al., 1996; Peate et al., 1997; Manea et al., 2014; Singer et al., 2007; Cooper et al., 2020). In contrast, at relatively warm SZs (Cascades, southwest Japan, western Mexico) slab dehydration effects may occur at predominantly shallow depths, largely in the frontal arc region, such that slab fluid contributions beneath the main body and rear of the arc are likely minimal (cf. Harry and Green, 1999; Manea et al., 2005; Ferrari et al., 2012; Kimura et al., 2014). However, release of water from deep serpentinized parts of hot slabs could potentially trigger wet melting of altered oceanic crust or sediments (cf. van Keken et al., 2011; Yogodzinski et al., 2015; Sisson and Kelemen, 2018).
From the perspective of subduction-related magmatism, it is useful to examine more closely the well-characterized Cascade arc of western North America (Figure 1). This ∼1,300 km-long arc is an important end member example in that it is one of the warmest modern SZs. After an early compressive and accretionary history (Mesozoic to Eocene time; cf. Sigloch and Mihalynuk, 2017), subduction beneath the Pacific Northwest migrated westward to its present location following accretion of the Siletzia oceanic plateau (ca. 50 Ma; McCrory and Wilson, 2013; Wells et al., 2014). Remnants of the Siletzia terrane comprise much of the Oregon-Washington Coast Ranges, and pieces likely extend further east in the subsurface (cf. Bedrosian and Feucht, 2014; Gao and Shen, 2014). Some have proposed that the westward jump in subduction could have been triggered directly by impingement of a mantle plume on the PNW, that presumably produced the Siletzia and related oceanic volcanics, and is now situated beneath Yellowstone (Stern and Dumitru, 2019). The ancestral Cascade arc was initiated by ca. 40–45 Ma (du Bray and John, 2013; du Bray et al., 2014), and has been intermittently active to the present. High convergence rates during early stages (Verplanck and Duncan, 1987) would have caused dehydration of the slab to persist well inboard of the trench compared to the present, thereby modifying the composition of the mantle wedge and accounting for initially high volcanic productivity. Over time, convergence rate has declined about four-fold resulting in warming of the slab, accelerated dehydration of subducted materials, release of slab-derived fluids predominantly beneath the forearc region, and diminished fluid flux beneath the modern volcanic arc and back-arc region (cf. Bostock et al., 2002; van Keken et al., 2011).
Because of better preservation and exposure, the Pliocene to Quaternary activity (<∼5 Ma) has been investigated in greatest detail as summarized by Hildreth (2007). Cascadia is more complex than the standard paradigm for volcanic arcs. Owing to the curvature of the coastline, convergence is oblique along the US coast and nearly orthogonal along the British Columbia coast (Figure 3). Volcanic activity forms a narrow, near-linear belt in Canada with three major stratovolcano complexes and associated mafic monogenetic vent fields. Isolated centers of Mt. Baker and Glacier Peak extend the northern Cascades into Washington State. Following a gap of ∼100 km in which there has been no Quaternary volcanic activity, the arc becomes more continuous and progressively broadens going from southern Washington, through Oregon, and into northern California. Oblique convergence resulted in fragmentation and clockwise rotation of tectonic blocks underlying the forearc and much of the southern Cascades in Oregon and California (Wells et al., 1998; McCaffrey et al., 2007; Wells and McCaffrey, 2013). This is accompanied by westward slab rollback beneath much of the US Cascades (cf. Humphreys and Coblentz, 2007; Long et al., 2012). Consequently, parts of the arc have been in a state of extension dating back to ∼16 Ma in the south (Priest et al., 2012; Wells and McCaffrey, 2013), and ∼7 Ma in central Oregon where a central graben initiated and has propagated as far north as southern Washington (Conrey et al., 1997). Also the large, dominantly basaltic, Simcoe, Newberry, and Medicine Lake lava fields developed immediately behind (east of) the main Cascades arc since late Pliocene time. High frequency mafic volcanism in these latter regions likely reflects increasingly more efficient transmissivity of basaltic magmas through the extending crust over time (cf. Hildreth, 2007; Porritt et al., 2011; Till et al., 2019).
FIGURE 3. General tectonic setting of the Cascade volcanic arc that spans from British Columbia through Washington and Oregon to northern California (after Mullen et al., 2017). Triangles indicate locations of prominent Quaternary stratovolcanoes; selected ones are identified as Mts. Baker (B), Glacier Peak (GP), Rainier (R), St. Helens (SH), Adams (A), Hood (H), Shasta (S), and Lassen (L). Large backarc volcanic fields are identified as Simcoe (Sim), Newberry (New), and Medicine Lake (MedL). Dashed field in Oregon is the High Cascades central graben. Also shown are general distributions of Pliocene-Recent High Cascades (yellow field) and Eocene-Miocene Western Cascades (green field) volcanic rocks (cf. Luedke and Smith, 1982). Large arrows show azimuth and velocity of subducting oceanic plates (dark green field) relative to North America. Selected inset rectangle is expanded in Figure 4.
The nature of the underlying crust varies along strike: accretionary Klamath terrane (Paleozoic-to-Cretaceous) in northern CA and southern OR, accreted Silezia oceanic terrane in northern OR-southern WA, and accretionary North Cascades terrane (Paleozoic-to-Paleocene) in northern WA and southern British Columbia (cf. Schmidt et al., 2008; Mullen et al., 2017). The northern and southern sectors have a more “continental” character whereas the central part of the arc is more mafic and denser. Presumably the associated lithospheric mantle domains differ as well and, together with the distinct crustal assemblages, may partly account for along-strike variations in the arc magmas. Several groups have investigated this possibility, focusing on relatively young and primitive basaltic lavas, with somewhat different outcomes (e.g., Schmidt et al., 2008; Mullen et al., 2017; Pitcher and Kent, 2019). It should be noted that these studies focus almost exclusively on mafic samples from lava fields and flank eruptions peripheral to the major stratovolcanoes (that are andesitic or more evolved in composition). All of the cited works find that the northern (Canadian) Cascades are distinctive in having dominantly intraplate OIB-like basalts accompanied by smaller proportions of CA type basalts. Mount Baker and Glacier Peak areas have few basaltic lavas but include some high-Mg andesites of CA type. In southern Washington, the Mt. Rainier area has few basalts, largely of CA type with minor LKT (cf. Reiners et al., 2000; Sisson et al., 2014). Beginning near Mount St. Helens and Mt. Adams, basaltic activity is increasingly prolific and quite diverse with abundant LKT and OIB-like lavas coupled with diverse CA type lavas including basalt, absarokite, and mafic basaltic andesite (see below).
Proceeding further south through Oregon, mafic products increase in proportion but are dominantly of CA and LKT types. Schmidt et al. (2008) proposed that the chemical variances define four distinct segments to the arc, whereas Pitcher and Kent (2019) subdivide the arc into six statistically distinct segments based on a much larger data set. An interesting outcome of their work is a tally of the proportions of each magma type found in each of their segments (see below). Both studies advocate subtle differences in the mantle sources for basalts along strike, with possible modifications in the nature and/or magnitude of slab-derived contributions (increasing toward the south; cf. Walowski et al., 2016). In contrast, Mullen et al. (2017) argue that primitive Cascade LKT and CA basalts are very similar with regard to their Sr-Nd-Hf and particularly Pb isotopic compositions, and propose that they could be derived from a common “background” mantle, modified by varied input from subducted sediments or oceanic crust to produce respective LKT and CA type sources. They consider that the “background” mantle was isotopically similar to the Juan de Fuca MORB source mantle, which poses the question of how such material is now accessible—presumably in the mantle wedge. They also propose the involvement of a distinct source of OIB-like character for dominant basalts of the Canadian sector and for the Simcoe backarc volcanic area, and attribute its presence to upwelling of asthenospheric mantle through tears in the subducting slab.
The Cascades arc is unusual in that modern volcanism occurs in a wide arc-normal swath, including vents up to 90 km trenchward from the main volcanic axis. The southern part shares similarities with the Trans-Mexican Volcanic arc including oblique subduction and extensional tectonism. Except in the Canadian sector, there is no well-defined volcanic front but rather a broad distribution of vents that suggests magma availability beneath an extensive area. A good example is seen in a west-east transect proximal to the Columbia River near latitude 46°N (Figure 4; the Cascade Columbia Transect, or CCT, of Leeman et al., 2005). The vent distribution in this area is possibly influenced by oblique convergence (Hildreth, 2007; Fleck et al., 2014), and by northern reaches of the central graben, both of which may be facilitated by complex material flow in the underlying mantle. Representative lavas from this area have been described in detail (cf. Leeman et al., 1990; Conrey et al., 1997; Leeman et al., 2005; Jicha et al., 2009). Mafic lavas in this sector of the Cascades are more diverse than anywhere else in the arc, and often erupted from vents as close as a few km apart (cf. Hildreth, 2007). See Supplementary Table S3 for typical data.
FIGURE 4. Map of volcanic fields, monogenetic vents, and stratovolcano complexes (Mt. St. Helens, Adams, and Hood) in the Cascades Columbia Transect (CCT), near latitude 46°N (after Hildreth, 2007). Indian Heaven (green shading) and Simcoe (red shading) are coherent volcanic fields of dominantly mafic lavas. Dashed rectangle is location of Boring Volcanic Field, shown in more detail in Figure 8.
Leeman and Smith (2018) describe two varieties of basaltic lavas erupted within a period of ≤300 years (∼1,700–2,000 years BP) from flank and near-summit vents at Mount St. Helens (MSH) separated by only 3 km. These magmas were essentially contemporaneous and fed by close-spaced conduits, but show no evidence of interacting with one another. Both magmas are of intraplate type—slightly evolved LKT and OIB-like basalts with up to ∼7% MgO, but very distinctive overall geochemistry. Their proximity raises interesting questions. Can they be formed by melting a common heterogeneous source domain, or must their sources be separated in space or depth? And, in either case, how do proximal magma streams retain their compositional uniqueness? Based on thermobarometry, it was concluded that these eruptions originated from distinct magma reservoirs located at depths near 24 and ∼28 km, respectively, and that each originally segregated from mantle depths of ∼80 and ∼50–60 km. As a hint to the complexity of this system, Figure 5 shows an interpretive view of the magma plumbing system beneath MSH. Depths to shallower magma reservoirs are also based on thermobarometry of more evolved basaltic and intermediate composition lavas, and on the work of Blatter et al. (2013, 2017) on the andesites and dacites. It is generally assumed that all Cascade stratovolcanoes are underlain by analogous complex systems of stacked magma reservoirs and conduits where opportunities for melting wall rocks and mixing of magmas likely abound (cf. Hildreth, 2007; Kent, 2013). It is surprising that discrete basaltic magma types largely survived the transit to the surface through such an obstacle course, although the MSH data do show that mixing occurred between the least-evolved basaltic end members and a component similar to MSH andesite (Leeman and Smith, 2018). Indeed, many of the CCT vents seen in Figure 4 produced chemically diverse basalts that are more primitive than those at MSH (cf. Leeman et al., 2005; Pitcher and Kent, 2019).
FIGURE 5. Schematic cross-section showing inferred depth relations for crustal-level Mount St. Helens (MSH) magma reservoirs and plumbing system as described in Leeman and Smith (2018); note that horizontal scale is greatly expanded. In this case, depths are computed using the thermobarometer of Lee et al. (2009) and based on compositions of the most MgO-rich Cave basalt (LKT) and Castle Creek basalts (OIB-like) of both nepheline- and hypersthene-normative character. Reservoirs feeding these eruptions are, respectively, “A,” “B1,” and “B2-S”/“B2-N”—the latter for hy-normative lavas vented high on the south and north flanks of MSH. Multiple flows from these vents exhibit compositional variations due to mixing with andesitic material within the magmatic system. Diagram also shows implied feeder conduits to deeper mantle sources of the respective LKT and OIB-like primary magmas (below diagram). Additional shallower reservoirs are inferred to exist as ultimate sources for more evolved magmas at MSH; formation and storage depths of evolved dacitic magmas are discussed by Blatter et al. (2017).
The diversity issue is further exemplified in the Boring Volcanic Field (BVF) situated in the Portland Basin and contiguous areas at the far western end of the CCT (see inset in Figure 4). The forearc BVF is dispersed over an area of ∼4,000 km2, of which ∼500 km2 is covered by volcanic rocks with a cumulate volume of ∼10 km3 erupted from at least 80 distinct monogenetic vents that were active in late Pliocene–Pleistocene time (Evarts et al., 2009; Evarts et al., 2017; Leeman et al., 2019). Over 100 distinct map units have been defined, many consisting of multiple flow units. Most of the field has been systematically sampled for chemical and paleomagnetic analyses, and many of the distinct volcanic map units have been dated by high-precision 40Ar/39Ar geochronology (Fleck et al., 2014; Hagstrum et al., 2017). Those from local vents range in age from ∼2,700 to 50 ka; these are locally underlain or interfingered with numerous late Tertiary (3,600–1,400 ka) LKT flows that originated from vents just east of the Portland Basin, in the western High Cascades. There are minor equivalents further south in the western Cascades as young as 42 ka (Rowe and Tepley, 2016).
High-precision XRF analyses are available for 1,271 samples, and these data are used to assess magmatic diversity. Overall, the suite is relatively mafic (SiO2 range: 47–60 wt%), and many of the basaltic lavas have greater than 7.5% MgO, ranging up to 10 wt% for some samples. All samples have been assigned to compositional groups, similar to those of Leeman et al. (2005); distinguishing criteria given in Supplementary Table S4 are for high-MgO (>7.5 wt%) samples. Groups from the BVF are given a “B” prefix, whereas the slightly older LKTs are distinguished with a “T” prefix: specifically, low-K tholeiites (BLKT, 5%; TLKT, 14%), OIB-like lavas (BOIB, 12%), calcalkalic basalts (BCA, 37%), absarokites (BABS, 7%), and rocks with greater than 56% SiO2 are classified as andesites (BAND, 9%); cited percentages indicate proportions of the total sample population. And finally, samples that did not precisely meet the classification criteria are simply called “transitional” (BTran, 15%). Many of the latter have affinity to one of the other groups, but are anomalous in some fashion and do not fall cleanly into one of the prescribed groups; it is suspected that many are products of mixing between two or more of the defined magma types. Key discriminants used to classify the entire population are: 1) LKTs have less than 0.5% K2O; 2) OIBs have K2O >0.5% as well as elevated Nb and Nb/Zr ratios; 3) both intraplate groups have low Ba- and Sr-enrichment relative to Ti, Zr, or Nb; 4) CA types are distinct in having strong enrichments of Ba and Sr; and 5) ABS types are more extremely enriched in K2O, Ba, and Sr; all CA type samples with more than 500 ppm Ba are arbitrarily assigned to the ABS group (that now includes what Leeman et al., 2005, called high-K CA lavas.
Although details of the petrology and geochemistry of the BVF will be presented elsewhere, diagrams in Figure 6 illustrate several unique aspects of the magma types with respect to their SiO2 content and Mg# (values > 63 correspond to equilibrium olivine of ∼Fo85 or higher, and are considered to be primitive). First, a plot of total alkalis (Na2O + K2O) vs. SiO2, shows the general petrologic groupings to which samples are assigned. Although fields for the CA and OIB-like magma types overlap considerably in this diagram, the LKTs are systematically lower in alkalis. Some of the OIB-like and CA types with highest alkali content fall into either the absarokite (ABS) or shoshonite (SHO) field. In the other diagrams, the LKTs are again clearly distinguished, as are the OIB-like group with respect to their Nb-enrichment, and the ABS group forms a distinct cluster with respect to Ba/Zr and Ba/TiO2 ratios.
FIGURE 6. Variation diagrams for lavas of the Boring Volcanic field (BVF) are based on XRF analyses of 1,271 samples (Leeman et al., 2005, Leeman et al., 2019); these show range in (A) SiO2 vs. total alkalis, (B) Mg# (=Mg/[Mg + Fe], molar ratio) vs. K2O, (C) Nb/Zr vs. Ba/Zr, and (D) SiO2 vs. Ba/TiO2. Symbols denote assigned magma type based on these and other discriminant diagrams. Fields in (A) are labeled to indicate compositional space for basalt (BAS), basaltic andesite (BA), andesite (AND), absarokite (ABS, or Trachy-basalt), shoshonite (SHO, or Basaltic trachy-andesite), and banakite (BAN) as referred to in text. Symbol key: age differences are denoted by prefix “T” for Pliocene (3.0–3.6 Ma) or “B” for Quaternary (<2.6 Ma); magma types include “low-K tholeiite” (LKT), “ocean island-like” (OIB), and “calcalkalic” (CA) basalts, and “absarokitic” (ABS), “andesitic” (AND), and “transitional” (Tran; mixtures or otherwise ill-defined) lavas. Dashed lines denote upper limit values for K2O, Ba/Zr, and Ba/TiO2 used to distinguish the LKT group.
Figure 7 illustrates Ba-, Sr-, and K- enrichment vs. both Mg# and wt% MgO. Primitive LKT types are readily distinguished from other magma types in all of these diagrams, albeit a few overstep the plotted discriminant lines as MgO content decreases below 7 wt%. OIB-like lavas are likewise distinguished from CA types by their low Ba/Nb ratios, and from the LKTs by their higher Sr/Y, Ba/TiO2, and K2O/TiO2 ratios. Primitive ABS type lavas are clearly strongly enriched in Ba, Sr, and K2O, but enrichments in these elements diminish with decreasing MgO content, and there is a possible continuum between ABS and the less enriched CA types. These patterns likely signify that some local mixing occurs between different magma types. However, clear compositional differences between most of the LKTs and the other groups implies their formation from distinctly lower K2O and Ba sources. The OIB-like lavas also require a source with lower Ba as well as higher Nb than any of the CA type lavas. Finally, because many of the CA type lavas have higher MgO (many exceeding 9 wt%, and Mg#s ≥ 70) than the most mafic LKT or OIB-like lavas, it appears that they are derived from more refractory (i.e., relatively melt-depleted) sources; this is supported by the presence of magnesian olivine phenocrysts (up to Fo92) in some of these samples (Smith and Leeman, 2005).
FIGURE 7. Additional discriminant diagrams for BVF lavas; same key as in Figure 6. Ratios of Ba/Nb, Sr/Y, Ba/TiO2, and K2O/TiO2 are plotted vs. Mg# or MgO wt%. Note that all chemical types include a significant number of “primitive” lavas with MgO > 8 wt%. Ba/Nb clearly distinguishes all intraplate lavas (both LKT and OIB-like) from those of CA affinity (CA and ABS types). Primitive LKT types are easily distinguished in all of these diagrams, albeit some overstep the plotted discriminant lines as MgO content decreases below 7 wt%. Primitive ABS type lavas are clearly strongly enriched in Ba, Sr, and K2O, but enrichments in these elements diminish as MgO content decreases. Dashed lines indicate discriminant values for abscissa parameters in defining magma groups.
For purposes of this paper, the data set was further filtered to exclude evolved lavas by considering only samples with Mg# > 57 and MgO wt% > 8.0; lavas with as little as 7.48% MgO were included for the BLKT group to obtain a more representative population. Average compositions of these groups are presented in Table 1 and are believed to approximate distinctive end member magma types that fed the BVF. It is inferred that such MgO-rich magmas ascended more or less directly from the underlying mantle.
The map distribution of BVF samples is provided in Figure 8, where plot symbols indicate magma type. The striking impression is that, despite some clustering, all magma types are dispersed across the entire Portland Basin as far west as the Portland Hills, some 90 km west of the main High Cascades axis (e.g., Mt. Hood). Figure 9 shows the age and spatial distribution of the magma types, and again there is considerable overlap indicating that all types were generally available throughout the early history of the BVF. LKT magmas dominated the earliest stages, and last appeared at ∼1,400 ka. Other magma types collectively have been dominant in the area since that time. It also appears that volcanism generally expanded westward over the lifespan of the field. This is particularly noticeable for the OIB-like lavas, with exception of a ca. 2,500 ka group in the southern Portland Basin. As at MSH, a fundamental question regards how these diverse magma types are produced, and where.
FIGURE 8. Locations of analyzed samples within the Portland Basin forearc region of the Cascade arc (Leeman et al., 2019). Base map is from Evarts et al. (2009). Symbols denote chemical affinity for individual samples collected at those locations. Map colors show distribution of locally erupted volcanic rocks (in orange except for BLKT tholeiites (blue-green), and TLKT tholeiites (purple) from High Cascades vents east of the map area.
FIGURE 9. Distribution of magma types over time in western sectors of the Cascades Columbia Transect (CCT) proximal to Portland Basin (Fleck et al., 2014; Hagstrum et al., 2017; Leeman et al., 2019). Local eruptions in the Portland Basin area initiated as early as ∼2,600 ka and have continued intermittently to ∼50 ka. LKT activity was restricted to the early history of the field, whereas other magma types erupted from the onset to the near-present.
In an attempt to answer this question, a thermobarometric approach was taken using primitive lavas of each distinct BVF magma type as well as representative samples from across the CCT. The methodology is described in the Supplementary Material, and uses basaltic samples with high MgO content (≥8 wt% for all groups except the less magnesian BLKT, for which 7.5 wt% was used as a cutoff) that are essentially olivine-phyric (ideally of ∼Fo85 or higher), such that their compositions are closest to equilibrium with assumed mantle peridotite. As described by Leeman et al. (2005), the bulk compositions are corrected by incremental addition of equilibrium olivine until they approach final equilibrium with a target source Mg#. Calculations were done assuming that the magmas were only slightly more oxidized than the QFM redox buffer (Smith and Leeman, 2005), assuming a value of 0.15 for the mole fraction of ferric iron. This value is consistent with actual olivine phenocrysts (typically ca. Fo84–86 in LKTs, and higher for OIB-like and CA type lavas). It is also consistent with measured V/Sc and Zn/Fe ratios that in primitive CCT lavas overlap with values for magnesian intraplate basalts (cf. Lee et al., 2005, Lee et al., 2010). Detailed examples of recalculated “primary” melt compositions of representative CCT lava types are presented in Supplementary Table S2. Partial results are also shown in Table 1 for the BVF average magma types. These are calculated on a water-free basis. However, inclusion of water (up to 3 wt%) lowers estimated temperatures by <30°C per wt% H2O and has little effect on calculated pressures of equilibration (cf. Plank and Forsythe, 2016). Existing MI data for CCT lavas indicate that water contents are less than ∼0.3 wt% for LKTs and about 2 wt% for the most water-rich CA and ABS lavas that have been studied (e.g., Rea et al., 2012). Also, because the fertility of actual magma sources is unknown, results were computed for a range of mantle Mg#s (90 all groups; also 91 and 92 for the more magnesian CA type magmas; see below). As a caveat, these calculations are based on melt equilibration with olivine + orthopyroxene-bearing mantle sources, but are applicable to lherzolitic sources (cf. Supplementary Material). Compositions of primitive LKTs are consistent with this assumption; compositions of many of the other magma types suggest possible fractionation of clinopyroxene or involvement of pyroxenitic sources (cf. Herzberg and Asimow, 2015; Lambart et al., 2016). Future work is needed to evaluate the effects of source variability on estimated segregation (i.e., final equilibration) depths and temperatures.
Figure 10 shows estimated final equilibration depths vs. longitudinal position for a number of representative CCT lavas, including several from the frontal arc BVF. These are near 65–80 km for the most primitive LKTs, decrease to ∼50–60 km for primitive OIB-like basalts within the arc, and increase to ∼100 km for some backarc Simcoe lavas. Note that P–T loci for these samples lie above the dry peridotite solidus (cf. Figure 11). If derived from a source with similar Mg#, primitive CA and ABS types appear to segregate from shallower depths (∼45–60 km). Yet shallower depths obtained for mafic basaltic andesites are likely minimal values considering their comparatively evolved compositions. For example, at MSH, shallowing of calculated depths is correlated with increasing andesite component mixed into mafic lavas; this essentially lowers the Mg# of the mixture (Leeman et al., 2005; Leeman and Smith, 2018). Taken at face value, the relative depths are surprising in that the CA type magmas with the strongest “slab” influence appear to have equilibrated with mantle domains furthest above the subducting slab. Equilibration depths for the westernmost LKTs appear to be near or possibly below the seismically imaged slab (McCrory et al., 2012; Mann et al., 2019), but this may be an artifact because some originated from vents many kms to the east. In any case, LKT depths are considered most robust and leave little wiggle room to produce other magma types from greater depths within the mantle wedge.
FIGURE 10. Cross-section of the Cascadia subduction zone near latitude 46°N provides a broad view illustrating calculated segregation P–T relations for representative CCT lavas. The thermobarometry depicted is based on primitive magma compositions corrected by olivine addition to attain equilibrium with Fo90 mantle olivine (cf. Leeman et al., 2005; Lee et al., 2009; see Supplementary Material for details and Supplementary Table S2 for example calculations). Sample points are positioned assuming vertical ascent to the actual sample localities, but westernmost LKTs likely vented tens of kms to the east; symbols denote magma type. Reference localities are Boring Volcanic Field (BVF), Mt. St. Helens (MSH), Hood (MH), and Adams (MA), and the Simcoe volcanic field (SIM). Temperature contours based on interpolation of calculated segregation temperatures suggest that the upper mantle is very warm in this region, approaching the dry peridotite solidus at depths near 80–90 km. These data suggest a tiered compositional structure with intraplate type magmas rising from systematically deeper levels than the calcalkalic magma types. “SW” is inferred serpentinized portion of the mantle wedge (after Bostock et al., 2002). Heavy green dashed lines indicate top of the subducting slab near this part of the arc from (A)Michaelson and Weaver (1986) and (B)Mann et al. (2019).
FIGURE 11. Estimated P–T conditions for last equilibration of primitive Cascades basaltic lavas (from Figure 10) assuming lherzolitic mantle sources (Mg# = 90) for all. These are compared with representative mantle solidi curves. Gray lines are calculated peridotite solidi with 0, 0.05, and 0.10 wt% H2O (Katz et al., 2003). Equivalent dry solidus of Hirschmann (2000) (for plot, see Supplementary Table S2) is slightly warmer than labeled “Dry solidus.” Bold black line is experimental solidus for pargasite lherzolite (PL) with ∼0.02% ppm H2O; bold dashed and solid line at p above 3 GPa represent mantle vapor-saturated solidus (Green et al., 2014). Blue and green curves (H2O-sat 1 and H2O-sat 2), solidi for water-saturated peridotite (also see Fig. 2), are considered less realistic by Green et al. (2014). Cascades LKT and OIB lavas are consistent with essentially anhydrous sources. CA type melts with temperature adjustments (arrow) for up to 3 wt% H2O, lie near or plot below the dry solidus—consistent with their formation from slightly hydrated sources. Alternative source models need to be evaluated further for these melts.
Average primitive CA and ABS group lavas have high Mg#s consistent with equilibrium olivine compositions near Fo88, and some individual lavas carry olivine phenocrysts of up to Fo92. Depth estimates for both the CA and ABS averages increase on the order of 10–15 km for each 1% increase in source Mg#, placing them closer (possibly equivalent?) to segregation depths for some of the LKT/OIB magmas. Scenarios that bring the respective sources spatially closer carry the implication that the mantle source domain is heterogeneous, in which case production and effective separation of the respective distinct magma types becomes a challenge.
If the magmas ascend vertically from their source domains, this creates a spatial problem in getting LKT (and perhaps other) source material into the forearc part of the mantle wedge. Moreover, this region is interpreted to be extensively serpentinized and thus much cooler (e.g., <700°C) than the estimated magmatic temperatures, as verified by geophysical investigations in southern Washington (Brocher et al., 2003; Hansen et al., 2016; Abers et al., 2017). This problem is further compounded because even the eruptive temperatures (those calculated from uncorrected lava compositions) are above 1,200°C for each magma type (Table 1). This issue has been addressed in several geophysical studies proximal to MSH, and Hansen et al. (2016) propose that magmas feeding MSH migrate there from more easterly sources. However, this idea is difficult to reconcile with the demonstrated diversity of magmas erupted there and at points even further west (i.e., BVF).
The broad and persistent distribution of mafic magmatism in the BVF and nearby areas suggests that there has been sustained melt generation in the forearc region for ≥2.5 Ma. Its manifestation may be facilitated by tectonic factors related to oblique subduction. But the source of thermal energy to drive melt generation remains unclear. Two possibilities are that: 1) there is a disruption or tear in the subducting slab, that allows ascent and decompression melting of asthenospheric mantle to produce the OIB-like basalts; and 2) there is a broad upwelling of asthenospheric mantle from the backarc region that can melt, possibly producing LKT magmas (Sisson and Bronto, 1998; Elkins-Tanton et al., 2001; Lewis et al., 2001; Tamura et al., 2002). Ascent of the latter (or both) may provide sufficient heat to partially melt the shallower lithospheric mantle to produce CA type magmas. This scenario is consistent with the larger segregation depths and early arrival of LKT type magmas. Although Mann et al. (2019) find no break in the slab, there is tomographic evidence for thinning or a gap in the Farallon slab near latitude 46°N (Liu and Stegman, 2011; Porritt et al., 2011) and, more convincingly, evidence for a tear in the Juan de Fuca plate further south (Hawley and Allen, 2019). A similar “slab-tear” scenario has been proposed for the Canadian Cascades to explain the abundance of OIB-like basalts in that region (Mullen and Weis, 2015). There is also considerable evidence for mantle upwelling in the backarc (Currie et al., 2004; Long et al., 2012; Till et al., 2013; Gao and Shen, 2014) as well as under portions of the Cascades (Bodmer et al., 2018; Zhou et al., 2018).
It is interesting to compare such suggested upwelling with models proposed for mantle plumes. In Hawaii, evidence for compositional heterogeneities has been explained in terms of domains of relatively enriched material (e.g., pyroxenites) embedded within more refractory peridotitic mantle. The kinematic modeling of Hofmann and Farnetani (2013) suggests that, with large-scale convection or flow, such impurities can be effectively drawn into long string-like structures (“fibers”) that may preferentially melt during ascent. At low degrees of melting the impurities dominate early melts that are relatively LILE-rich, whereas at high degrees of melting this component is diluted or depleted and melts become less enriched in incompatible elements. In the case of SZs, there is also the added factor that impurities can be in the form of slab-derived contributions, and these may have a more dominating effect in cooler systems as opposed to warm systems like the Cascades. Another possibility is that portions of lithospheric mantle, associated with blocks like the Siletzia oceanic plateau, are subducted and comprise “impurities” (or domains) of significant volume (cf. Schmandt and Humphreys, 2011). Such materials, if melted, would be expected to produce magmas of “oceanic” compositional character–as has been proposed by Straub et al. (2008) for the Mexican volcanic arc. It is clear that some Simcoe basalts have Pb isotopic compositions (Leeman et al., 1990; Mullen et al., 2017) that uniquely resemble such data for the Siletzia volcanics (Phillips et al., 2017) and are isotopically distinct from Pb in other Cascades magma types. At present, it remains a challenge to explain the diversity of the CCT magma array, not only regarding the nature and proportions of source components, but also how so many of the erupted basaltic lavas retain their distinct primitive compositions as they make their way toward the surface.
There is seismic and magnetotelluric evidence for the presence of warm domains both near the subducting slab and at Moho depths (∼40 km), for example near the latitude of Mount Rainier (McGary et al., 2014), and in the deep crust beneath parts of the CCT (Hansen et al., 2016). Such evidence supports the presence of melt at depths consistent with the thermobarometry approach summarized above. However, the relatively small-scale diversity among the erupted basaltic magmas suggests that conduits and magma chambers must commonly be isolated at the scale of a few km as opposed to forming large homogenizing reservoirs.
At this point, it is useful to consider the geographic distribution of magma types in the Cascades. This approach has been used previously (e.g., Pearce et al., 2005) to investigate links between magma geochemistry and kinematic development of parts of the Marianas arc. Pitcher and Kent (2019) effectively have done this to some extent for the Cascades. They present their results in the form of averages of the magma types present in their six arc segments, weighted according to numerical proportions in each area. It is informative to simply look at the spatial distribution of the most primitive lavas. This obviously restricts the number of samples being considered, and may leave some spatial gaps, but does provide a general view of how distinct mantle sources are likely distributed at depth. This map (Figure 12) shows locations of samples screened as before for primitive character; also juxtaposed is another map from Hildreth (2007) showing tectonic elements in the same area. What stands out first is the concentration of OIB-like samples (and to lesser extent ABS samples) in the CCT traverse, but also in a southeast-trending belt that runs from the BVF area across the Oregon graben area toward the Newberry volcanic field and perhaps further east. Data are sparse for the latter trend, and there are few OIB-like samples in the far back-arc High Lava Plains (HLP) region. Compared with the tectonic map, this distribution shows a close correspondence with the boundary between extensional and transpressional regimes, and the concentration of OIB-like basalts roughly corresponds to the region impacted by the Siletzia accretion event. Also, the OIB-like lavas are less than ∼5 Ma in age; older ones have not been recognized so far. Does their appearance record a significant tectonic transition?
FIGURE 12. (Left) Map showing locations of primitive Cascades basaltic lavas, with symbols denoting magma type. Only samples with Mg# > 60 and MgO > 7.5 wt% are plotted. Additional stratocones identified include Mts. Garibaldi (G), Mazama (Maz) and the Three Sisters (TS). The distribution of Pliocene-Recent Cascades volcanic rocks is shown in darker green shading, and a region of transtensional deformation (pale green shading) includes much of the southern Cascades as well as a broad High Lava Plains area behind the arc in southern Oregon; the distribution of LKT type basalts is concentrated in this area. (Right) Map (after Hildreth, 2007) shows tectonic elements in the Cascades and nearby areas, as well as along-strike differences in the nature of the underlying basement terranes (after Schmidt et al., 2008). These figures emphasize the restricted distribution of primitive OIB-like basalts. Those in Oregon-Washington are concentrated in the CCT swath near 46°N and in a diffuse array extending southeastward from the Portland area, following an inferred transition zone between dominantly transpressional (to the west) and extensional (to the east) tectonic regimes. Their close association with the region of the accreted Siletzia terrane may not be coincidental. Also note that the preponderance of LKT in the southern portions of the arc and backarc regions appears to be associated with extension that began propagating into the region (blue arrow) in late Miocene time (cf. Till et al., 2013).
A second feature that stands out is the distribution of LKT basalts. With very few exceptions, these are concentrated south of about 46°N latitude, are very common and widespread across the Cascades in southern Oregon and California, and similar lavas are common in the backarc regions (Newberry-HLP and Medicine Lake areas). This has been noted in previous surveys (cf. Till et al., 2013), and it is clear that the LKT basalts are associated with areas that have been in extensional mode beginning in the late Miocene in the backarc regions, and propagating into the Cascades up to modern times. Pitcher and Kent (2019) estimate LKT proportions (of all samples in a given segment) to be approximately 25% for southern Washington, 20% for the Oregon graben area, and 50% for the southern Cascades, with similar proportions in the immediate backarc fields (Medicine Lake and Newberry).
Although few CA type basalts are plotted in Figure 12 (only those with Mg# > 63), such lavas (including high-Mg basaltic andesites) comprise significant proportions in all but the Canadian segment; they dominate northern Washington, and comprise about half of the samples in more southerly segments (Pitcher and Kent, 2019). It has been suggested that CA and LKT magmas may be produced from a common source as a consequence of flux melting (i.e., degree of melting varies in response to the amount of aqueous fluid that is available; Reiners et al., 2000; Rowe et al., 2009). Considering the warmth of the Cascadia slab, it has also been proposed that primitive CA type lavas may form by flux/reaction between wedge peridotite and infiltrating hot-slab hydrous melts (Lassen region, Walowski et al., 2016; Mt. Baker and Glacier Peak, Sas et al., 2017). Rather than forming these different magma types by flux melting of a common source, Mullen et al. (2017) visualize them having distinct sources evolved from a common “background” depleted mantle (∼MORB source) via different degrees of enrichment by slab-derived SC. Relative to LKTs, it is enigmatic that the higher Mg# CA types (presumably from more refractory sources) are most enriched in so-called slab-derived elements.
Here, I compare calculated eruptive temperatures for primitive Cascades and representative backarc (HLP) basalts (Figure 13). Such estimates are based on uncorrected sample analyses using thermometry of Lee et al. (2009); where measured, water contents are <1 wt% for most LKTs and rarely exceed 3 wt% for other magma types (see earlier discussion). Relative to latitudinal position, there are several notable points. First, OIB-like basalts have maximal Ts in excess of 1,300°C, and a range of about 100°C, which is positively correlated with sample MgO content (∼8–10 wt%), or increasing “primitiveness.” Higher Ts would seem consistent with segregation of OIB-like magmas from deep mantle as it welled up through gaps in the subducting slabs. Second, Cascades LKTs exhibit a robust along-strike increase in T (also Mg#; cf. Leeman et al., 2005) going south from Washington to California. Essentially, the southern LKTs and those from the HLP are derived from more refractory mantle than those near the CCT. These two groups are associated with highly extensional areas accompanied by significant mantle upwelling due to slab rollback (cf. Long et al., 2012; Gao and Shen, 2014) and by opening of a tear or hole in the subducting Juan de Fuca plate beneath the southern Cascades (Hawley and Allen, 2019). There is also a southward increase in Ba content of LKTs (cf. Leeman et al., 2005) that may reflect higher contributions of slab-derived SC beneath the southern segment of the arc. If this implies greater influx of water or melt diapirs from the subducting slab, it might be expected to see this reflected in lowered magmatic temperatures—but this is not apparent. The mantle in this region was possibly depleted in magmaphile elements such that slab contributions have greater leverage there than further north, hence enrichments of Ba, etc. are proportionally larger (Borg et al., 1997; Borg et al., 2002). Also, segregation depths calculated for these LKTs are consistent with their formation near the dry mantle peridotite solidus (Hirschmann, 2000) at depths ca. 75 km. On an anhydrous basis, apparent Ts for primitive CA type basalts overlap those of the other groups. However, with corrections for up to 3 wt% H2O (cf. Walowski et al., 2015), maximum Ts are close to 1,200°C. These Ts are well above water-saturated peridotite or pyroxenite solidi, yet below the dry mantle solidus–thus, consistent with slightly hydrated sources (cf. Katz et al., 2003). These conditions also seem consistent with flux-melting models involving hydrous silicic slab melts as proposed by Walowski et al., 2015). A plausible alternative scenario is that melt generation is primarily due to upwelling or reheating of mantle (asthenosphere or remobilized lithosphere) that had been enhanced previously by additions of Ba and other slab-derived components (Borg et al., 2002).
FIGURE 13. Estimated eruptive temperatures for samples of primitive magma types along strike in the Cascade arc (cf. Leeman et al., 2005). Data for far backarc LKTs from the High Lava Plains in eastern Oregon (cf. Till et al., 2013) are superimposed for comparison (outlined in green field). Temperatures are calculated for lavas with MgO >7 wt% (see Supplementary Material for details). Thermometry is most robust for the LKTs owing to their low water contents. Of significance is the general increase in magmatic temperatures for Cascades LKT going southward from southern Washington (Mt. St. Helens area) to northern California (Lassen area).
Current views on subduction-related magmatism are based on observations of diverse volcanic arcs that differ in significant ways. It is important to recognize fundamental processes that create suitable sources for arc magmas, but also consider that they have been operating over long periods of time and have cumulative effects such as storage of subduction signatures in static lithospheric mantle and crustal reservoirs. Very sparse data for early Cascades magmatism precludes definitive statements about its inception and early evolution. But the post-Miocene warm Cascades arc provides perspective on SZ processes where fluid contributions from the downgoing slab are relatively low. This is supported by generally low abundances of FMEs like boron (cf. Leeman et al., 2004; Manea et al., 2014) as well as the occurrence of numerous lavas with intraplate-like trace element contents. Warm slab temperatures are expected to promote melting of subducted oceanic crust or sediments, and high precision Sr-Nd-Pb-Hf isotopic data seem consistent with such contributions to many arc magmas (e.g., Cascades CA types and some LKTs; Mullen et al., 2017). The relative importance of these vs. lithosphere contributions is unresolved, but in cooler arcs (most SZs), larger inputs of slab-derived fluids may promote a dominance of CA type magmas. This in turn may mask (or simply overwhelm) melt productivity within the lithosphere. This leaves open questions regarding the timing and magnitude of slab contributions. For example, to what extent are observed subduction signatures in a given magma directly induced by coeval direct slab inputs vs. inherited from the shallow lithospheric mantle (i.e., recycling of older magmatic products)?
These concepts are incorporated into Figure 14 that portrays a Cascadia-like continental margin SZ. Three general modes of magma formation are envisaged: [1] CA type magmas produced in response to SC additions to the deep mantle wedge, followed by ascent with modification; [2] LKT and possibly OIB-like magmas produced by decompression melting of asthenospheric mantle ascending from backarc regions or through torn slab; and [3] calcalkalic-like magmas produced by melting of lithospheric mantle (including earlier frozen-in magmatic contributions) in response to magma infusion from the other domains. In post-Miocene Cascadia, modes [2] and [3] have become increasingly assertive at the expense of mode [1], and vary in intensity along-strike in response to varied tectonic influences.
FIGURE 14. Schematic cross-section of a generalized continental margin subduction zone showing important petrologic and geochemical domains. Boundary between non-convecting lithospheric mantle portion of the upper plate and the underlying convecting asthenospheric mantle is highly schematic. Light arrows rising from the subducting slab signify transfer of fluids and/or melts from slab to lower mantle wedge; in warm Cascadia the shallow wedge is considered to be strongly serpentinized due to shallow slab dehydration. A blue-shaded arrow symbolizes how subduction components (SC) may be distributed into the convecting mantle wedge (cf. Cerpa et al., 2018). This and the distribution of partial melts within the wedge are highly dependent upon many factors (cf. Figure 2 of this paper). It is generally believed that many arc magmas are 1) generated within the mantle wedge partly in response to the catalytic effect of adding slab-derived water and/or melts, 2) enriched in other components that produce distinctive calcalkalic charateristics, and 3) modified by reactions with progressively warmer mantle as they rise, thus accounting for their elevated temperatures (cf. Grove et al., 2012). In the figure, location “1” signifies a likely position from which such magmas may ascend beneath arcs (bold vertical arrows). A second style of magmatism is related to decompression of convective limbs in the mantle wedge; e.g., region “2” in the figure. Such melts are formed and released over a broad backarc region and in the uppermost part of the ascending asthenospheric limb. If direct inputs of SC from the currently subducting slab are small to negligible, these melts are likely to resemble intraplate magmas (LKT and possibly OIB-like variants), depending upon the history and compositional structure of the convecting asthenosphere. It is probable that the transfer of heat (and possibly fluids) by magmas ascending from domains “1” and “2” will promote warming and partial melting of parts of the lithospheric mantle; e.g., region “3.” Such melts could form by remelting earlier emplaced magmatic bodies as well as more refractory components of the old lithospheric mantle. Considering the unusually warm nature of the Cascadia SZ, involvement of slab-derived fluids is likely relatively small during Quaternary time, and appears unlikely to dominate the style of magma productivity in this arc. The smaller leverage of fluid-related processes may contribute to the apparent eminence of intraplate-like magmatism, to increased production of slab melting locally, and to generally high magmatic temperatures in primitive basaltic magmas—overall a higher involvement of adiabatic vs. aqueous flux melting. Conversely, in cooler SZs, greater influence of slab-derived fluids is likely to shift the magmatic spectrum toward the calcalkalic flavor.
Other take away points that can be applicable elsewhere include the following:
(1) An important impetus driving overall arc magmatism is from ascending mafic magmas (largely basalt) that energize the whole system and instigate remelting of shallower mantle and crust. In most arcs, certainly continental ones, significant fractions of the basaltic magma produced never make it to the surface owing to impediments (zero buoyancy, structural compression, etc.) to their ascent. They provide an efficient mechanism to transfer heat and mass to shallower domains (uppermost mantle near Moho or lower crust). But to make continental crust from an arc, a large fraction of that intruded basalt ultimately must be removed (delamination?) to get the correct mass balance.
(2) Some primitive arc basalts are surprisingly hot! Cascade arc LKT and OIB-like magmas appear to have last equilibrated with mantle at conditions above the anhydrous peridotite solidus; their formation requires no slab-derived fluid, and they likely are products of decompression melting of ascending mantle in diapirs or convective flow. In contrast, primitive Cascade CA type magmas appear to be derived from more refractory sources and have predicted P–T equilibration conditions near or below the anhydrous mantle solidus; their formation is inferred to involve some degree of flux-melting, consistent with their relatively higher water contents.
(3) In general, many compositional features of arc magmas point to significant contributions of subducted materials in their formation and/or evolution. But it is often difficult to ascertain the processes or time-scales by which these additions occur. Selective enrichments of strongly fluid-mobile components point to influxes of aqueous fluids, whereas some components are more readily mobilized in melts. Which transport pathway is involved, and which components are transported may be strongly influenced by thermal regime. And, of course, there is considerable variability in what is being subducted, hence in the nature of slab-derived components.
(4) There is potential to use magma chemistry as a “monitor” of spatial-temporal processes operating in volcanic arcs. This is one of the few ways to obtain a direct signal for what is happening at depth. Coupled with geophysical and other means of probing the Earth’s interior, it provides a broader base to interpret what is going on. In this regard, it is unsettling that there seems to be uncertainty as to where the magmas feeding Mount St. Helens actually come from!
The author confirms being the sole contributor of this work and has approved it for publication.
Portions of this work were funded by National Science Foundation in the form of research grants, most recently Grant No. 0003612.
The author declares that the research was conducted in the absence of any commercial or financial relationships that could be constructed as a potential conflict of interest.
I especially thank Vlad Manea for his invitation to prepare this paper on a topic that I have considered for most of my career. I also thank numerous colleagues and associates who worked with me on past projects and contributed ideas and data. And as an impetus for this particular paper, I had the pleasure of working with Russ Evarts who really kick-started new studies of the Boring Volcanic Field, and provided access to much of the analytical data mentioned here. We lost Russ in 2017, and my goal is to formally see that his contributions see the light of day. Likewise, I have benefitted greatly from my association with Rick Conrey, Martin Streck, and Diane Smith—my Cascades coauthors and friends for many years. Finally, I thank Paul Wallace, Robert Stern, and Diane Smith for their contributions to helping me improve this paper.
The Supplementary Material for this article can be found online at: https://www.frontiersin.org/articles/10.3389/feart.2020.535879/full#supplementary-material
Abers, G. A., van Keken, P. E., and Hacker, B. R. (2017). The cold and relatively dry nature of mantle forearcs in subduction zones. Nat. Geosci. 10 (5), 333–337. doi:10.1038/ngeo2922
Anderson, A. T. (1976). Magma mixing: petrological process and volcanological tool. J. Volcanol. Geoth. Res. 1, 3–33. doi:10.1016/0377-0273(76)90016-0
Annen, C., Blundy, J. D., and Sparks, R. S. J. (2006). The genesis of intermediate and silicic magmas in deep crustal hot zones. J. Petrol. 47, 505–539. doi:10.1093/petrology/egi084
Bacon, C. R., Bruggman, P. E., Christiansen, R. L., Clynne, M. A., Donnelly-Nolan, J. M., and Hildreth, W. (1997). Primitive magmas at five cascade volcanic fields: melts from hot, heterogeneous sub-arc mantle. Can. Mineral. 35, 397–423.
Beard, J. S., and Lofgren, G. E. (1991). Dehydration melting and water-saturated melting of basaltic and andesitic greenstones and amphibolites at 1, 3, and 6. 9 kb. J. Petrol. 32, 365–401. doi:10.1093/petrology/32.2.365
Bebout, G. E., and Penniston-Dorland, S. C. (2016). Fluid and mass transfer at subduction interfaces-the field metamorphic record. Lithos. 240–243, 228–258. doi:10.1016/j.lithos.2015.10.007
Bedrosian, P. A., and Feucht, D. W. (2014). Structure and tectonics of the northwestern United States from EarthScope USArray magnetotelluric data. Earth Planet Sci. Lett. 402, 275–289. doi:10.1016/j.epsl.2013.07.035
Behn, M. D., Kelemen, P. B., Hirth, G., Hacker, B. R., and Massonne, H.-J. (2011). Diapirs as the source of the sediment signature in arc lavas. Nat. Geosci. 4, 641–646. doi:10.1038/NGEO1214
Beier, C., Haase, K. M., Brandl, P. A., and Krumm, S. H. (2017). Primitive andesites from the Taupo Volcanic Zone formed by magma mixing. Contrib. Mineral. Petrol. 172, 33. doi:10.1007/s00410-017-1354-0
Blatter, D. L., Sisson, T. W., and Hankins, W. B. (2013). Crystallization of oxidized, moderately hydrous arc basalt at mid- to lower-crustal pressures: implications for andesite genesis. Contrib. Mineral. Petrol. 166, 861–886. doi:10.1007/s00410-013-0920-3.
Blatter, D. L., Sisson, T. W., and Hankins, W. B. (2017). Voluminous arc dacites as amphibole reaction-boundary liquids. Contrib. Mineral. Petrol. 172, 27. doi:10.1007/s00410-017-1340-6
Blum-Oeste, M., and Wörner, G. (2016). Central Andean magmatism can be constrained by three ubiquitous end-members. Terra. Nova. 28, 434–440. doi:10.1111/ter.12237
Bodmer, M., Toomey, D. R., Hooft, E. E. E., and Schmandt, B. (2018). Buoyant asthenosphere beneath Cascadia influences megathrust segmentation. Geophys. Res. Lett. 45, 6954–6962. doi:10.1029/2018GL078700
Borg, L. E., Blichert-Toft, J., and Clynne, M. (2002). Ancient and modern subduction zone contributions to the mantle sources of lavas from the Lassen region of California inferred from Lu-Hf isotopic systematics. J. Petrol. 43, 705–723. doi:10.1093/petrology/43.4.705
Borg, L. E., Clynne, M., and Bullen, T. (1997). The variable role of slab-derived fluids in the generation of a suite of primitive calc-alkaline lavas from the southernmost Cascades, California. Can. Mineral. 35, 425–452.
Bostock, M. G., Hyndman, R. D., Rondenay, S., and Peacock, S. M. (2002). An inverted continental Moho and serpentinization of the forearc mantle. Nature. 417, 536–538. doi:10.1038/417536a
Brocher, T. M., Parsons, T., Tréhu, A. M., Snelson, C. M., and Fisher, M. A. (2003). Seismic evidence for widespread serpentinized forearc upper mantle along the Cascadia margin. Geology. 31 (3), 267–270. doi:10.1130/0091-7613(2003)031<0267:SEFWSF>2.0.co;2
Cerpa, N. G., Wada, I., and Wilson, C. R. (2018). Effects of fluid influx, fluid viscosity, and fluid density on fluid migration in the mantle wedge and their implications for hydrous melting. Geosphere. 15, 1–23. doi:10.1130/GES01660.1
Çoban, H., Karsli, O., Caran, S., and Yilmaz, K. (2019). What processes control the genesis of absarokite to shoshonite-banakite series in an intracontinental setting, as revealed by geochemical and Sr-Nd-Pb isotope data of Karadağ Stratovolcano in Central Anatolia, Turkey. Lithos. 324–325, 609–625. doi:10.1016/j.lithos.2018.11.034
Codillo, E. A., Le Roux, V., and Marschall, H. R. (2018). Arc-like magmas generated by mélange-peridotite interaction in the mantle wedge. Nat. Commun. 9 (9), 2864. doi:10.1038/s41467-018-05313-2
Conrey, R. M., Sherrod, D. R., Hooper, P. R., and Swanson, D. A. (1997). Diverse primitive magmas in the Cascade Arc, northern Oregon and southern Washington. Can. Mineral. 35, 367–396.
Conway, C. E., Chamberlain, K. J., Harigane, Y., Morgan, D. J., and Wilson, C. J. N. (2020). Rapid assembly of high-Mg andesites and dacites by Magma mixing at a continental arc stratovolcano. Geology, 1033–1037. doi:10.1130/G47614.1
Cooper, G. F., Macpherson, C. G., Macpherson, C. G., Blundy, J. D., Maunder, B., Allen, R. W., et al. (2020). Variable water input controls evolution of the Lesser Antilles volcanic arc. Nature. 582 (7813), 525–529. doi:10.1038/s41586-020-2407-5
Cooper, L. B., Ruscitto, D. M., Plank, T., Wallace, P. J., Syracuse, E. M., and Manning, C. E. (2012). Global variations in H2O/Ce: 1. Slab surface temperatures beneath volcanic arcs. Geochem. Geophys. Geosyst. 13. doi:10.1029/2011GC003902
Currie, C., Wang, K., Hyndman, R. D., and He, J. (2004). The thermal effects of steady-state slab-driven mantle flow above a subducting plate: the Cascadia subduction zone and backarc. Earth Planet Sci. Lett. 223, 35–48. doi:10.1016/j.epsl.2004.04.020
DeBari, S. M., and Greene, A. R. (2011). “Vertical stratification of composition, density, and inferred magmatic processes in exposed arc crustal sections.” in Arc-continent collision. Berlin, Germany: Springer, 121–144. doi:10.1007/978-3-540-88558-0_5
du Bray, E. A., and John, D. A. (2013). Petrologic, tectonic, and metallogenic evolution of the Ancestral Cascades magmatic arc, Washington, Oregon, and northern California. Geosphere. 7, 1102–1133. doi:10.1130/GES00669.1
du Bray, E. A., John, D. A., and Cousens, B. L. (2014). Petrologic, tectonic, and metallogenic evolution of the southern segment of the ancestral Cascades magmatic arc, California and Nevada. Geosphere. 10, 1–39. doi:10.1130/GES00944.1
Eichelberger, J. C., Izbekov, P. E., and Browne, B. L. (2006). Bulk chemical trends at arc volcanoes are not liquid lines of descent. Lithos. 87, 135–154. doi:10.1016/j.lithos.2005.05.006
Elkins Tanton, L. T., Grove, T. L., and Donnelly-Nolan, J. (2001). Hot, shallow mantle melting under the Cascades volcanic arc. Geology. 29(7), 631–634. doi:10.1130/0091-7613(2001)0290631:HSMMUT2.0.co;2
Elliott, T., Plank, T., Zindler, A., White, W., and Bourdon, B. (1997). Element transport from slab to volcanic front at the Mariana arc. J. Geophys. Res. 102, 14991–15019. doi:10.1029/97JB00788
Evarts, R. C., Conrey, R. M., Fleck, R. J., and Hagstrum, J. T. (2009). “The boring volcanic field of the Portland-Vancouver area, Oregon and Washington: tectonically anomalous forearc volcanism in an urban setting.” in Volcanoes to vineyards: geologic field trips through the dynamic landscape of the Pacific Northwest. Editors O’Connor, J. E., Dorsey, R. J., and Madin, I. P. (Boulder, CO: Geological Society of America Field Guide), Vol. 15, 253–270.
Evarts, R. C., Conrey, R. M., Leeman, W. P., and Streck, M. J. (2017). Insights into magmatic diversity in the Columbia Cascades Transect (CCT). Portland, Oregon: International Association of Volcanology and Chemistry of the Earth’s Interior General Session, Abstract 1232.
Farner, M. J., and Lee, C.-T. A. (2017). Effects of crustal thickness on magmatic differentiation in subduction zone volcanism: a global study. Earth Planet Sci. Lett. 470, 96–107. doi:10.1016/j.epsl.207.04.025
Ferrari, L., Orozco-Esquivel, T., Manea, V., and Manea, M. (2012). The dynamic history of the Trans-Mexican Volcanic Belt and the Mexico subduction zone. Tectonophysics. 522–523, 122–149. doi:10.1016/j.tecto.2011.09.018
Fleck, R. J., Hagstrum, J. T., Calvert, A. T., Evarts, R. C., and Conrey, R. M. (2014). 40Ar/39Ar geochronology, paleomagnetism, and evolution of the Boring volcanic field, Oregon and Washington, USA. Geosphere. 10 (6): 1283–1314. doi:10.1130/GES00985.1
Gao, H., and Shen, Y. (2014). Upper mantle structure of the cascades from full-wave ambient noise tomography: evidence for 3D mantle upwelling in the back-arc. Earth Planet Sci. Lett. 390, 222–233. doi:10.1016/j.epsl/2014.01.012
Gerya, T., and Stöckhert, B. (2006). Two-dimensional numerical modeling of tectonic and metamorphic histories at active continental margins. Int. J. Earth Sci. 95, 250–274. doi:10.1007/s00531-005-0035-9
Gómez-Tuana, A., Straub, S. M., and Zellmer, G. F. (2013). “An introduction to orogenic andesites and crustal growth,” in Orogenic andesites and crustal growth. Editors Gómez-Tuena, A., Straub, S. M., and Zellmer, G. F. (London, UK: Geological Society of London Special Publication) Vol. 385, 1–13.
Green, D. H., Hibberson, W. O., Rosenthal, A., Kovács, I., Yaxley, G. M., Falloon, T. J., et al. (2014). Experimental study of the influence of water on melting and phase assemblages in the upper mantle. J. Petrol. 55, 2067–2096. doi:10.1093/petrology/egu050
Grove, T. L., Elkins-Tanton, L. T., Parman, S. W., Chatterjee, N., Muntener, O., and Gaetani, G. A. (2003). Fractional crystallization and mantle-melting controls on calc-alkaline differentiation trends. Contrib. Mineral. Petrol. 145, 515–533. doi:10.1007/s00410-003-0448-z
Grove, T. L., and Till, C. B. (2019). H2O-rich mantle melting near the slab-wedge interface. Contrib. Mineral. Petrol. 174, 80. doi:10.1007/s00410-019-1615-1
Grove, T. L., Till, C. B., and Krawczynski, M. J. (2012). The role of H2O in subduction zone magmatism. Annu. Rev. Earth Planet Sci. 40, 413–439. doi:10.1146/annurev-earth-042711-105310
Grove, T., Parman, S., Bowring, S., Price, R., and Baker, M. (2002). The role of an H2O-rich fluid component in the generation of primitive basaltic andesites and andesites from the Mt. Shasta region, N California. Contrib. Mineral. Petrol. 142, 375–396. doi:10.1007/s004100100299
Hagstrum, J. T., Fleck, R. J., Evarts, R. C., and Calvert, A. T. (2017). Paleomagnetism and 40Ar/39Ar geochronology of the Plio-Pleistocene Boring Volcanic Field: implications for the geomagnetic polarity time scale and paleosecular variation. Phys. Earth Planet. Int. 262, 101–115. doi:10.1016/j.pepi.2016.07.008
Hansen, S. M., Schmandt, B., Levander, A., Kiser, E., Vidale, J. E., Abers, G. A., et al. (2016). Seismic evidence for a cold serpentinized mantle wedge beneath Mount St Helens. Nat. Commun. 7, 1–6. doi:10.1038/ncomms13242.
Harry, D. L., and Green, N. L. (1999). Slab dehydration and basalt petrogenesis in subduction systems involving very young oceanic lithosphere. Chem. Geol. 160, 309–333. doi:10.1016/S0009-2541(99)00105-9
Hawley, W. B., and Allen, R. M. (2019). The fragmented death of the Farallon plate. Geophys. Res. Lett. 46, 7386–7394. doi:10.1029/2019GL083437
Hayes, G. P., Moore, G. L., Portner, D. E., Hearne, M., Flamme, H., Furtney, M., et al. (2018). Slab2, a comprehensive subduction zone geometry model. Science. 362, 58–61. doi:10.1126/science.aat4723
Hermann, J., and Spandler, C. J. (2007). Sediment melts at sub-arc depths: an experimental study. J. Petrol. 49 (4), 717–740. doi:10.1093/petrology/egm073
Herzberg, C., and Asimow, P. D. (2015). PRIMELT3 MEGA.XLSM software for primary magma calculation: peridotite primary magma MgO contents from the liquidus to the solidus. Geochem. Geophys. Geosyst. 16, 563–578. doi:10.1002/2014GC005631
Hickey-Vargas, R., Holbik, S., Tormey, D., Frey, F. A., and Moreno Roa, H. (2016). Basaltic rocks from the Andean Southern Volcanic Zone: insights from the comparison of along-strike and small-scale geochemical variations and their sources. Lithos. 258–259, 115–132. doi:10.1016/j.lithos.2016.04.014
Hildreth, W. (2007). Quaternary magmatism in the cascades – geologic perspectives. U. S. Geol. Surv. Prof. Pap. 174, 125.
Hildreth, W., and Moorbath, S. (1988). Crustal contributions to arc magmatism in the Andes of Central Chile. Contrib. Mineral. Petrol. 98, 455–489. doi:10.1007/BF00372365
Hirschmann, M. M. (2000). Mantle solidus: experimental constraints and the effects of peridotite composition. Geochem. Geophys. Geosyst. 1, 1042. doi:10.1029/2000GC000070
Hofmann, A. W., and Farnetani, C. G. (2013). Two views of Hawaiian plume structure. Geochem. Geophys. Geosyst. 14, 5308–5322. doi:10.1002/2013GC004942
Humphreys, E. D., and Coblentz, D. D. (2007). North American dynamics and western U.S. tectonics. Rev. Geophys. 45. doi:10.1029/2005RG000181
Humphreys, M. C. S., Edmonds, M., Plail, M., Barclay, J., Parkes, D., and Christopher, T. (2013). A new method to quantify the real supply of mafic components to a hybrid andesite. Contrib. Mineral. Petrol. 165, 191–215. doi:10.1007/s00410-012-0805-x
Ishizuka, O., Yuasa, M., Tamura, Y., Shukuno, H., Stern, R. J., Naka, J., et al. (2010). Migrating shoshonitic magmatism tracks Izu-Bonin-Mariana intra-oceanic arc rift propagation. Earth Planet Sci. Lett. 294, 111–122. doi:10.1016/j.epsl.2010.03.016
Jicha, B. R., Hart, G. L., Johnson, C. M., Hildreth, W., Beard, B. L., Shirey, S. B., et al. (2009). Isotopic and trace element constraints on the petrogenesis of lavas from the Mount Adams volcanic field, Washington. Contrib. Mineral. Petrol. 157, 189–207. doi:10.1007/s00410-008-0329-6
Karlstrom, L., Wright, H. M., and Bacon, C. R. (2015). The effect of pressurized magma chamber growth on melt migration and pre-caldera vent locations through time at Mount Mazama, Crater Lake, Oregon. Earth Planet Sci. Lett. 412, 209–219. doi:10.1016/j.epsl.2014.12.001
Katz, R. F., Spiegelman, M., and Langmuir, C. H. (2003). A new parameterization of hydrous mantle melting. Geochem. Geophys. Geosyst. 4 (9), 1073. doi:10.1029/2002GC000433
Kelemen, P. B., and Behn, M. D. (2016). Formation of lower continental crust by relamination of buoyant arc lavas and plutons. Nat. Geosci. 9, 197–205. doi:10.1038/NGEO2662
Kelemen, P. B., Hanghj, K., and Greene, A. R. (2003). One view of the geochemistry of subduction-related magmatic arcs, with an emphasis on primitive andesite and lower crust. Treatise on Geochemistry. 3, 593–659. doi:10.1016/B0-08-043751-6/03035-8
Kelley, K. A., and Cottrell, E. (2009). Water and the oxidation state of subduction zone magmas. Science. 325, 605–607. doi:10.1126/science.1174156
Kelley, K. A., Plank, T., Newman, S., Stolper, E. M., Grove, T. L., Parman, S., et al. (2010). Mantle melting as a function of water content beneath the Mariana arc. J. Petrol. 51, 1711–1738. doi:10.1093/petrology/egq036
Kent, A. J. R. (2013). “Preferential eruption of andesitic magmas: implications for volcanic magma fluxes at convergent margins.” in: Orogenic andesites and crustal growth. Editors Gómez-Tuena, A., Straub, S. M., and Zellmer, G. F. (London: Geological Society of London Special Publication) 385, 257–289. doi:10.1144/SP385.10
Kimura, J.-I., Gill, J. B., Kunikiyo, T., Osaka, I., Shimoshioiri, Y., Katakuse, M., et al. (2014). Diverse magmatic effects of subducting a hot slab in SW Japan: results from forward modeling. Geochem. Geophys. Geosyst. 15, 691–739. doi:10.1002/2013GC005132
Kimura, J.-I., Manton, W. I., Sun, C.-H., Iizumi, S., Yoshida, T., and Stern, R. J. (2002). Chemical diversity of the Ueno basalts, Central Japan: identification of mantle and crustal contributions to arc basalts. J. Petrol. 43, 1923–1946. doi:10.1093/petrology/43.10.1923
Lambart, S., Baker, M. B., and Stolper, E. M. (2016). The role of pyroxenite in basalt genesis: melt‐PX, a melting parameterization for mantle pyroxenites between 0.9 and 5 GPa. J. Geophys. Res. Solid Earth. 121, 5708–5735. doi:10.1002/2015JB012762
Le Maitre, R. W. (2002). Igneous rocks—a classification and glossary of terms. 2nd Edn. Cambridge, England: Cambridge University Press, 236.
Lee, C.-T. A., Leeman, W. P., Canil, D., and Li, Z. A. (2005). Similar V/Sc systematics in MORB and arc basalts: implications for the oxygen fugacities of their mantle source regions. J. Petrol. 46, 2315–2336. doi:10.1093/petrology/egi056
Lee, C.-T. A., Luffi, P., Le Roux, V., Dasgupta, R., Albaréde, F., and Leeman, W. P. (2010). The redox state of arc mantle using Zn/Fe systematics. Nature. 468, 681–685. doi:10.1038/nature09617
Lee, C.-T. A., Luffi, P., Plank, T., Dalton, H., and Leeman, W. P. (2009). Constraints on the depths and temperatures of basaltic magma generation on Earth and other terrestrial planets using new thermobarometers for mafic magmas. Earth Planet Sci. Lett. 279, 20–33. doi:10.1016/j.epsl.2008.12.020
Leeman, W. P. (1983). The influence of crustal structure on compositions of subduction-related magmas. J. Volcanol. Geoth. Res. 18, 561–588. doi:10.1016/0377-0273(83)90026-4
Leeman, W. P. (1996). “Boron and other fluid-mobile elements in volcanic arc lavas: implications for subduction processes.” in Subduction: top to bottom. Geophysical Monograph series. Editors Bebout, G. E., Scholl, W., Kirby, H., and Platt, P. (Washington, DC: American Geophysical Union), Vol. 96, 269–276.
Leeman, W. P., Evarts, R. C., Conrey, R. M., and Streck, M. J. (2019). Magmatic diversity in the boring volcanic field, Portland Basin, Oregon and Washington: implications for subduction zone magmatism. Geological Society of America Abstracts with Program. 51, 33–3. doi:10.1130abs/2019CD-329545
Leeman, W. P., Lewis, J. F., Evarts, R. C., Conrey, R. M., and Streck, M. J. (2005). Petrologic constraints on the thermal structure of the Cascades arc. J. Volcanol. Geoth. Res. 140, 67–105. doi:10.1016/j.jvolgeores.2004.07.016
Leeman, W. P., and Smith, D. R. (2018). The role of magma mixing, identification of mafic magma inputs, and structure of the underlying magmatic system at Mount St. Helens. American Mineralogy. 103, 1925–1944. doi:10.2138/am-2018-6555
Leeman, W. P., Smith, D. R., Hildreth, W., Palacz, Z., and Rogers, N. (1990). Compositional diversity of Late Cenozoic basalts in a transect across the southern Washington Cascades: implications for subduction zone magmatism. J. Geophys. Res. 95, 19561–19582. doi:10.1029/JB095iB12p19561
Leeman, W. P., Tonarini, S., Chan, L. H., and Borg, L. E. (2004). Boron and lithium isotopic variations in a hot subduction zone-the southern Washington cascades. Chem. Geol. 212, 101–124. doi:10.1016/j.chemgeo.2004.08.010
Leeman, W. P., Turner, S. P., and Tonarini, S. (2017). Boron isotope variations in Tonga-Kermadec-New Zealand arc lavas: implications for origin of subduction components and mantle influences. Geochem. Geophys. Geosyst. 18, 1126–1162. doi:10.1002/2016GC006523
Leslie, R. A. J., Danyushevsky, L. V., Crawford, A. J., and Verbeeten, A. C. (2009). Primitive shoshonites from Fiji: geochemistry and source components. Geochem. Geophys. Geosyst. 10, 1–24. doi:10.1029/2008GC002326
Lewis, J. F., Leeman, W. P., and Evarts, R. C. (2001). Petrological constraints on the thermal structure of the southern Washington cascades. EOS Trans. AGU. 82 (47). Fall Meet. Suppl., Abstract V21C-0981.
Liu, L., and Stegman, D. R. (2011). Segmentation of the Farallon slab. Earth Planet Sci. Lett. 311 (1–2), 1–10. doi:10.1016/j.epsl.2011.09.027
Long, M. D., Till, C. B., Druken, K. A., Carlson, R. W., Wagner, L. S., Fouch, M. J., et al. (2012). Mantle dynamics beneath the Pacific Northwest and the generation of voluminous back-arc volcanism. Geochem. Geophys. Geosyst. 13. doi:10.1029/2012GC004189
Luedke, R. G., and Smith, R. L. (1982). Map showing distribution, composition, and age of late Cenozoic volcanic centers in Washington and Oregon. United States Geological Survey Miscellaneous Investigations Map I-1091-D, scale 1:1,000,000.
Mallik, A., Dasgupta, R., Tsuno, K., and Nelson, J. (2016). Effects of water, depth and temperature on partial melting of mantle-wedge fluxed by hydrous sediment-melt in subduction zones. Geochem. Cosmochim. Acta. 195, 226–243. doi:10.1016/j.gca.2016.08.018
Manea, V. C., Leeman, W. P., Gerya, T., Manea, M., and Zhu, G. (2014). Subduction of fracture zones controls mantle melting and geochemical signature above slabs. Nat. Commun. 5, 5095. doi:10.1038/ncomms6095
Manea, V. C., Manea, M., Kostoglodov, V., and Sewell, G. (2005). Thermo-mechanical model of the mantle wedge in Central Mexican subduction zone and a blob tracing approach for the magma transport. Phys. Earth Planet. In. 149, 165–186, doi:10.1016/j.pepi.2004.08.024.
Mann, M. E., Abers, G. A., Crosbie, K., Creager, K., Ulberg, C., Moran, S., et al. (2019). Imaging subduction beneath Mount St. Helens: implications for slab dehydration and magma transport. Geophys. Res. Lett. 46. doi:10.1029/2018GL081471
Marschall, H. R., and Schumacher, J. C. (2012). Arc magmas sourced from mélange diapirs in subduction zones. Nat. Geosci. 5 (12), 862–867. doi:10.1038/ngeo1634
Matzen, A. K., Wood, B. J., Baker, M. B., and Stolper, E. M. (2017). The roles of pyroxenite and peridotite in the mantle sources of oceanic basalts. Nat. Geosci. 10, 530–535. doi:10.1038/NGEO2968
Maunder, B., van Hunen, J., Bouilhol, P., and Magni, V. (2019). Modeling slab temperature: a reevaluation of the thermal parameter. Geochem. Geophys. Geosyst. 20. doi:10.1029/2018GC007641
McCaffrey, R., Qamar, A. I., King, R. W., Wells, R., Khazaradze, G., Williams, C. A., et al. (2007). Fault locking, block rotation and crustal deformation in the Pacific Northwest. Geophys. J. Int. 169, 1315–1340. doi:10.1111/j.1365-246X.2007.03371.x
McCrory, P. A., Blair, J. L., Waldhauser, F., and Oppenheimer, D. H. (2012). Juan de Fuca slab geometry and its relation to Wadati-Benioff zone seismicity. J. Geophys. Res. 117, B09306. doi:10.1029/2012JB009407
McCrory, P. A., and Wilson, D. S. (2013). A kinematic model for the formation of the Siletz-Crescent forearc terrane by capture of coherent fragments of the Farallon and Resurrection plates. Tectonics. 32, 718–736. doi:10.1002/tect.20045
McCulloch, M. T., and Gamble, J. A. (1991). Geochemical and geodynamical constraints on subduction zone magmatism. Earth Planet Sci. Lett. 102, 358–374. doi:10.1016/0012-821X(91)90029-H
McGary, R. S., Evans, R. L., Wannamaker, P. E., Elsenbeck, J., and Rondenay, S. (2014). Pathway from subducting slab to surface for melt and fluids beneath Mount Rainier. Nature. 511, 338–341. doi:10.1038/nature13493
Michaelson, C. A., and Weaver, C. S. (1986). Upper mantle structure from teleseismic Pwave arrivals in Washington and northern Oregon. J. Geophys. Res. 91, 2077–2094. doi:10.1029/JB091iB02p02077
Miyashiro, A. (1974). Volcanic rock series in island arcs and active continental margins. Am. J. Sci. 274. 321–355. doi:10.2475/ajs.274.4.321
Morris, J. D., Leeman, W. P., and Tera, F. (1990). The subducted component in island arc lavas: constraints from Be isotopes and B-Be systematics. Nature. 344, 31–36. doi:10.1038/344031a0
Mullen, E. K., Paquette, J.-L., Tepper, J. H., and McCallum, I. S. (2018). Temporal and spatial evolution of Northern Cascade Arc magmatism revealed by LA-ICP-MS U-Pb zircon dating. Can. J. Earth Sci. 55, 443–462. doi:10.1139/cjes-2017-0167
Mullen, E. K., and Weis, D. (2015). Evidence for trench-parallel mantle flow in the Northern Cascade Arc from basalt geochemistry. Earth Planet Sci. Lett. 414, 100–107. doi:10.1016/j.epsl.2015.01.010
Mullen, E. K., Weis, D., Marsh, N. B., and Martindale, M. (2017). Primitive arc magma diversity: new geochemical insights in the Cascade Arc. Chem. Geol. 448, 43–70. doi:10.1016/j.chemgeo.2016.11.006
Mullen, E. K., and Weis, D. (2013). Sr-Nd-Hf-Pb isotope and trace element evidence for the origin of alkalic basalts in the Garibaldi Belt, northern Cascade arc. Geochem. Geophys. Geosyst. 14, 3126–3155. doi:10.1002/ggge.20191
Murphy, M. D., Sparks, R. S. J., Barclay, J., Carroll, M. R., and Brewer, T. S. (2000). Remobilization of andesite magma by intrusion of mafic magma at the Soufriere Hills Volcano, Montserrat, West Indies. J. Petrol. 41, 21–42. doi:10.1093/petrology/41.1.21
Naumov, B. V., Kovalenko, V. I., Babansky, A. D., and Tolstykh, M. L. (1997). Genesis of andesites: evidence from studies of melt inclusions from minerals. Petrology. 5, 586–596.
Noll, P. D., Newsom, H. E., Leeman, W. P., and Ryan, J. G. (1996). The role of hydrothermal fluids in the production of subduction zone magmas: evidence from siderophile and chalcophile trace elements and boron. Geochem. Cosmochim. Acta. 60, 587–611. :doi:10.1016/0016-7037(95)00405-X.
Pearce, J. A. (1982). “Trace element characteristics of lavas from destructive plate boundaries.” in Andesites: orogenic andesites and related rocks. Editor Thorpe, R. S. (Chichester, UK: John Wiley), 525–548.
Pearce, J. A., and Peate, D. W. (1995). Tectonic implications of the composition of volcanic arc magmas. Annu. Rev. Earth Planet Sci. 23, 251–285. doi:10.1146/annurev.ea.23.050195.001343
Pearce, J. A., Stern, R. J., Bloomer, S. H., and Fryer, P. (2005). Geochemical mapping of the Mariana Arc-Basin system: implications for the nature and distribution of subduction components. Geochem. Geophys. Geosyst. 6, 1–27. doi:10.1029/2004GC000895
Peate, D. W., Pearce, J. A., Hawkesworth, C. J., Colley, H., Edwards, C. M. H., and Hirose, K. (1997). Geochemical variations in Vanuatu arc lavas: the role of subducted material and a variable mantle wedge composition. J. Petrol. 38, 1331–1358. doi:10.1093/petroj/38.10.1331
Penniston-Dorland, S. C., Kohn, M. J., and Manning, C. E. (2015). The global range of subduction zone thermal structures from exhumed blueschists and eclogites: rocks are hotter than models. Earth Planet Sci. Lett. 428, 243–254. doi:10.1016/j.epsl.2015.07.031
Perrin, A., Goes, S., Prytulak, J., Davies, D. R., Wilson, C., and Kramer, S. (2016). Reconciling mantle wedge thermal structure with arc lava thermobarometric determinations in oceanic subduction zones. Geochem. Geophys. Geosyst. 17, 4105–4127. doi:10.1002/2016GC006527
Perrin, A., Goes, S., Prytulak, J., Rondenay, S., and Davies, D. R. (2018). Mantle wedge temperatures and their potential relation to volcanic arc location. Earth Planet Sci. Lett. 501, 67–77. doi:10.1016/j.epsl.2018.08.011
Phillips, B. A., Kerr, A. C., Mullen, E. K., and Weis, D. (2017). Oceanic mafic magmatism in the Siletz terrane, NW North America: fragments of an Eocene oceanic plateau? Lithos. 274–275, 291–303. doi:10.1016/j.lithos.2017.01.005
Pitcher, B. W., and Kent, A. J. R. (2019). Statistics and segmentation: using Big Data to assess Cascades arc compositional variability. Geochem. Cosmochim. Acta. 265, 443–467. doi:10.1016/j.gca.2019.08.035
Plank, T., and Forsyth, D. W. (2016). Thermal structure and melting conditions in the mantle beneath the Basin and Range province from seismology and petrology. Geochem. Geophys. Geosyst. 17, 1312–1338. doi:10.1002/2015GC006205
Plank, T., Kelley, K. A., Zimmer, M. M., Hauri, E. H., and Wallace, P. J. (2013). Why do mafic arc magmas contain ∼4 wt% water on average? Earth Planet Sci. Lett. 364, 168–179. doi:10.1016/j.epsl.2012.11.044
Plank, T., and Langmuir, C. H. (1988). An evaluation of the global variations in the major element chemistry of arc basalts. Earth Planet Sci. Lett. 90, 349–370. doi:10.1016/0012-821x(88)90135-5
Plank, T. (2014). “The chemical composition of subducting sediments.” in Treatise on geochemistry. 2nd Edn. Amsterdam, Holland: Elsevier, Chap. 4.17, 607–629.
Poli, S., and Schmidt, M. W. (2002). Petrology of subducted slabs. Annu. Rev. Earth Planet Sci. 30, 207–235. doi:10.1146/annurev.earth.30.091201.140550
Porritt, R. W., Allen, R. M., Boyarko, D. C., and Brudzinski, M. R. (2011). Investigation of Cascadia segmentation with ambient noise tomography. Earth Planet Sci. Lett. 309, 67–76. doi:10.1016/j.epsl.2011.06.026
Portnyagin, M., Hoernle, K., Plechov, P., Mironov, N., and Khubunaya, S. (2007). Constraints on mantle melting and composition and nature of slab components in volcanic arcs from volatiles (H2O, S, Cl, F) and trace elements in melt inclusions from the Kamchatka Arc. Earth Planet Sci. Lett. 255, 53–69. doi:10.1016/j.epsl.2006.12.005
Priest, G. R., Hladky, F. R., Mertzman, S. A., Murray, R. B., and Wiley, T. J. (2013). Volcanic signature of basin and range extension on the shrinking Cascade arc, Klamath Falls-Keno area, Oregon. J. Geophys. Res. 118, 4013–4038. doi:10.1002/jgbr.50290.
Rapp, R. P., and Watson, E. B. (1995). Dehydration melting of metabasalt at 8-32 kbar: implications for continental growth and crust-mantle recycling. J. Petrol. 36, 891–931. doi:10.1093/petrology/36.4.891
Rawson, H., Keller, T., Fontijn, K., Pyle, D. M., Mather, T. A., Smith, V. C., et al. (2016). Compositional variability in mafic arc magmas over short spatial and temporal scales: evidence for the signature of mantle reactive melt channels. Earth Planet Sci. Lett. 456, 66–77. doi:10.1016/j.epsl.2016.09.056
Rea, J., Wallace, P. J., and Clynne, M. A. (2012). Pre-eruptive volatile contents of mafic magmas from the 2.0–1.7 ka Castle Creek eruptive period. Mount St. Helens, WA: EOS, American Geophysical Union, Fall Meeting. 2012, Abstract V53C-2853.
Reagan, M. K., and Gill, J. B. (1989). Coexisting calcalkaline and high-niobium basalts from Turrialba Volcano, Costa Rica: implications for residual titanates in arc magma sources. J. Geophys. Res. 94, 4619–4633. doi:10.1029/JB094iB04p04619
Rees Jones, D. W., Katz, R. F., Tian, M., and Rudge, J. F. (2018). Thermal impact of magmatism in subduction zones. Earth Planet Sci. Lett. 481, 73–79. doi:10.1016/j.epsl.2017.10.015
Reiners, P. W., Hammond, P. E., McKenna, J. M., and Duncan, R. A. (2000). Young basalts of the central Washington Cascades, flux melting of the mantle, and trace element signatures of primary arc magmas. Contrib. Mineral. Petrol. 138, 249–264. doi:10.1007/s004100050561
Reubi, O., and Blundy, J. (2009). A dearth of intermediate melts at subduction zone volcanoes and the petrogenesis of arc andesites. Nature. 461, 1269–1273. doi:10.1038/nature08510
Righter, K. (2000). A comparison of basaltic volcanism in the Cascades and western Mexico: compositional diversity in continental arcs. Tectonophysics. 318, 99–117. doi:10.1016/S0040-1951(99)00308-X
Rowe, M. C., Kent, A. J. R., and Nielsen, R. L. (2009). Subduction influence on oxygen fugacity and trace and volatile elements in basalts across the Cascade Volcanic Arc. J. Petrol. 50, 61–91. doi:10.1093/petrology/egn072
Rowe, M. C., and Tepley, F. J.. (2016). Origin and petrogenetic implications of anomalous olivine from a Cascade forearc basalt. Am. Mineral. 101, 1807–1819. doi:10.2138/am-2016-5651
Rudnick, R. L., and Gao, S. (2003). Composition of the continental crust. Treatise of Geochemistry. 3, 1–64. doi:10.1016/B0-08-043751-6/03016-4
Ruscitto, D. M., Wallace, P. J., Cooper, L. B., and Plank, T. (2012). Global variations in H2O/Ce: 2.Relationships to arc magma geochemistryand volatile fluxes. Geochem. Geophys. Geosyst. 13. doi:10.1029/2011GC003887.
Ruscitto, D. M., Wallace, P. J., and Kent, A. J. R. (2011). Revisiting the compositions and volatile contents of olivine-hosted melt inclusions from the Mount Shasta region: implications for the formation of high-Mg andesites. Contrib. Mineral. Petrol. 162, 109–132. doi:10.1007/s00410-010-0587-y
Ryan, J. G., and Chauvel, C. (2014). “The subdiction-zone filter and the impact of recycled material on the evolution of the mantle.” in Treatise on geochemistry. 2nd Edn. Amsterdam, Holland: Elsevier, Chap. 3.13, 479–508.
Sas, M., DeBari, S. M., Clynne, M. A., and Rusk, B. G. (2017). Using mineral geochemistry to decipher slab, mantle, and crustal input in the generation of high-Mg andesites and basaltic andesites from the northern Cascade Arc. Am. Mineral. 102, 948–965. doi:10.2138/am-2017-5756
Schmandt, B., and Humphreys, E. (2011). Seismically imaged relict slab from the 55 Ma Siletzia accretion to the northwest United States. Geology. 39, 175–178. doi:10.1130/G31558.1
Schmidt, M. E., Grunder, A. L., and Rowe, M. C. (2008). Segmentation of the Cascade Arc as indicated by Sr and Nd isotopic variation among diverse primitive basalts. Earth Planet Sci. Lett. 266, 166–181. doi:10.1016/j.epsl.2007.11.013
Schmidt, M. W., and Jagoutz, O. (2017). The global systematics of primitive arc melts. Geochem. Geophys. Geosyst. 18, 2817–2854. doi:10.1002/2016GC006699
Schmidt, M. W., and Poli, S. (1998). Experimentally based water budgets for dehydrating slabs and consequences for arc magma generation. Earth Planet Sci. Lett. 163, 361–379. doi:10.1016/S0012-821X(98)00142-3
Sigloch, K., and Mihalynuk, M. G. (2017). Mantle and geological evidence for a late jurassic–cretaceous suture spanning north America. Geol. Soc. Am. Bull. 129, 1489–1520. doi:10.1130/B31529.1
Singer, B. S., Jicha, B. R., Leeman, W. P., Rogers, N. W., Thirlwall, M. F., Ryan, J., et al. (2007). Along-strike trace element and isotopic variation in Aleutian Island arc basalt: subduction melts sediments and dehydrates serpentine. J. Geophys. Res. 112, B06206. doi:10.1029/2006JB004897
Singer, B. S., Leeman, W. P., Thirlwall, M. W., and Rogers, N. W. (1996). “Does fracture zone subduction increase sediment flux and mantle melting in subduction zones? Trace element evidence from Aleutian arc basalts.” in Subduction top to bottom geophysical monograph series. Editors Bebout, G. E.et al. (Washington, DC: American Geophysical Union), Vol. 96, 285–291.
Sisson, T. W., and Bronto, S. (1998). Evidence for pressure-release melting beneath magmatic arcs from basalt at Galunggung, Indonesia. Nature. 391, 883–886. doi:10.1038/36087
Sisson, T. W., and Kelemen, P. B. (2018). Near-solidus melts of MORB+4 wt% H2O at 0.8-2.8 GPa applied to issues of subduction magmatism and continent formation. Contrib. Mineral. Petrol. 173:70. doi:10.1007/s00410-018-1494-x
Sisson, T. W., Salters, V. J. M., and Larson, P. B. (2014). Petrogenesis of Mount Rainier andesite: magma flux and geologic controls on the contrasting differentiation styles at stratovolcanoes of the southern Washington Cascades. Geol. Soc. Am. Bull. 126, 122–144. doi:10.1130/B30852.1
Smith, D. R., and Leeman, W. P. (1993). The origin of Mount St. Helens andesites. J. Volcanol. Geoth. Res. 55, 271–303. doi:10.1016/0377-0273(93)90042-P
Smith, D. R., and Leeman, W. P. (2005). Chromian spinel-olivine phase chemistry and the origin of primitive basalts of the southern Washington Cascades. J. Volcanol. Geoth. Res. 140, 49–66. doi:10.1016/j.jvolgeores.2004.07.015
Solano, J. M. S., Jackson, M. D., Sparks, R. S. J., Blundy, J. D., and Annen, C. (2012). Melt segregation in deep crustal hot zones: a mechanism for chemical differentiation, crustal assimilation and the formation of evolved magmas. J. Petrol. 53, 1999–2026. doi:10.1093/petrology/egs041
Spandler, C., and Pirard, C. (2013). Element recycling from subducting slabs to arc crust: a review. Lithos. 170–171, 208–223. doi:10.1016/j.lithos.2013.02.016
Stern, R. J., and Dumitru, T. A. (2019). Eocene initiation of the Cascadia subduction zone: a second example of plume-induced subduction initiation? Geosphere. 15, 1–23. doi:10.1130/GES02050.1
Straub, S. M., LaGatta, A. B., Martin-Del Pozzo, A. L., and Langmuir, C. H. (2008). Evidence from high-Ni olivines for a hybridized peridotite/pyroxenite source for orogenic andesites from the central Mexican Volcanic Belt. Geochem. Geophys. Geosyst. 9. doi:10.1029/2007GC001583
Streck, M. J., and Leeman, W. P. (2018). Petrology of “Mt. Shasta” high-magnesian andesite (HMA): a product of multi-stage crustal assembly. American Mineralogy. 103, 216–240. doi:10.2138/am-2018-6151
Sun, S.-S., and McDonough, W. F. (1989). “Chemical and isotopic systematics of oceanic basalts: implications for mantle composition and processes.” in Magmatism in the ocean basins. Editors Saunders, A. D., and Norry, M. J. (London, UK: Geological Society Special Publication), Vol. 42, 313–345.
Syracuse, E. M., van Keken, P. E., and Abers, G. A. (2010). The global range of subduction zone thermal models. Phys. Earth Planet. In. 183, 73–90. doi:10.1016/j.pepi.2010.02.004
Tamura, Y., Tatsumi, Y., Zhao, D., Kido, Y., and Shukuno, H. (2002). Hot fingers in the mantle wedge: new insights into magma genesis in subduction zones. Earth Planet Sci. Lett. 197, 105–116. doi:10.1016/S0012-821X(02)00465-X.
Tatsumi, Y. (2005). The subduction factory: how it operates in the evolving Earth. GSA Today (Geol. Soc. Am.) 15, 4–10. doi:10.1130/1052-5173(2005)015[4:tsfhio]2.0.co;2
Till, C. B. (2017). A review and update of mantle thermobarometry of primitive arc magmas. American Mineralogy. 102, 931–947. doi:10.2138/am-2017-5783
Till, C. B., Grove, T. L., Carlson, R. W., Donnelly-Nolan, J. M., Fouch, M. J., Wagner, L. S., et al. (2013). Depths and temperatures of <10.5 Ma mantle melting and the lithosphere-asthenosphere boundary below southern Oregon and northern California. Geochem. Geophys. Geosyst. 14, 864–879. doi:10.1002/ggge.20070
Till, C. B., Kent, A. J. R., Abers, G. A., Janiszewski, H. A., Gaherty, J. B., and Pitcher, B. W. (2019). The causes of spatiotemporal variations in erupted fluxes and compositions along a volcanic arc. Nat. Commun. 10:1350. doi:10.1038/s41467-019-09113-0
Tonarini, S., Leeman, W. P., and Ferrara, G. (2001). Boron isotopic variations in lavas of the Aeolian volcanic arc, South Italy. J. Volcanol. Geoth. Res. 110, 155–170. doi:10.1016/S0377-0273(01)00203-7
Turner, S. J., and Langmuir, C. H. (2015). The global chemical systematics of arc front stratovolcanoes: evaluating the role of crustal processes. Earth Planet Sci. Lett. 422, 182–193. doi:10.1016/j.epsl.2015.03.056
Turner, S. J., Langmuir, C. H., Dungan, M. A., and Escrig, S. (2017). The importance of mantle wedge heterogeneity to subduction zone magmatism and the origin of EM1. Earth Planet Sci. Lett. 472, 216–228. doi:10.1016/j.epsl.2017.04.051
Turner, S. J., Langmuir, C. H., Katz, R. F., Dungan, M. A., and Escrig, S. (2016). Parental arc magma compositions dominantly controlled by mantle-wedge thermal structure. Nat. Geosci. 9, 772–776. doi:10.1038/ngeo2788
Ulmer, P., and Trommsdorff, V. (1995). Serpentine stability to mantle depths and subduction-related magmatism. Science. 268, 858–861. doi:10.1126/science.268.5212.858
van Keken, P. E., Hacker, B. R., Syracuse, E. M., and Abers, G. A. (2011). Subduction factory: 4. Depth-dependent flux of H2O from subducting slabs worldwide. J. Geophys. Res. 116, B01401. doi:10.1029/2010JB007922
Verplanck, E. P., and Duncan, R. A. (1987). Temporal variations in plate convergence and eruption rates in the western Cascades, Oregon. Tectonics. 6, 197–209. doi:10.1029/tc006i002p00197
Vielzeuf, D., and Schmidt, M. W. (2001). Melting relations in hydrous systems revisited: application to metapelites, metagreywackes and metabasalts. Contrib. Mineral. Petrol. 141, 251–267. doi:10.1007/s004100100237
Wallace, P. J. (2005). Volatiles in subduction zone magmas: concentrations and fluxes based on melt inclusion and volcanic gas data. J. Volcanol. Geoth. Res. 140, 217–240. doi:10.1016/j.jvolgeores.2004.07.023
Walowski, K. J., Wallace, P. J., Clynne, M. A., Rasmussen, D. J., and Weis, D. (2016). Slab melting and magma formation beneath the southern Cascade arc. Earth Planet Sci. Lett. 446, 100–112. doi:10.1016/j.epsl.2016.03.044
Walowski, K. J., Wallace, P. J., Hauri, E. H., Wada, I., and Clynne, M. A. (2015). Slab melting beneath the Cascade Arc driven by dehydration of altered oceanic peridotite. Nat. Geosci. 8, 404–408. doi:10.1038/NGEO2417
Wannamaker, P. E., Evans, R. L., Bedrosian, P. A., Unsworth, M. J., Maris, V., and McGary, R. S. (2014). Segmentation of plate coupling, fate of subduction fluids, and modes of arc magmatism in Cascadia, inferred from magnetotelluric resistivity. Geochem. Geophys. Geosyst. 15, 4230–4253. doi:10.1002/2014GC005509
Wells, R., Bukry, D., Friedman, R., Pyle, D., Duncan, R., Haeussler, P., et al. (2014). Geologic history of Siletzia, a large igneous province in the Oregon and Washington Coast Range: correlation to the geomagnetic polarity time scale and implications for a long-lived Yellowstone hotspot. Geosphere. 10, 692–719. doi:10.1130/GES01018.1
Wells, R. E., and McCaffrey, R. (2013). Steady rotation of the Cascade arc. Geology 41, 1027–1030. doi:10.1130/G34514.1
Wells, R. E., Weaver, C. S., and Blakely, R. J. (1998). Fore-arc migration in Cascadia and its neotectonic significance. Geol. 26, 759–762. doi:10.1130/0091-7613(1998)0260759:famica2.3.co;2
Woodhead, J. D., Eggins, S. M., and Johnson, R. W. (1998). Magma genesis in the New Britain island arc: further insights into melting and mass transfer processes. J. Petrol. 39, 1641–1668. doi:10.1093/petroj/39.9.1641
Woodhead, J., Eggins, S., and Gamble, J. (1993). High field strength and transition element systematics in island arc and back-arc basin basalts: evidence for multi-phase melt extraction and a depleted mantle wedge. Earth Planet Sci. Lett. 114, 491–504. doi:10.1016/0012-821X(93)90078-N
Yogodzinski, G. M., Brown, S. T., Kelemen, P. B., Vervoort, J. D., Portnyagin, M., Sims, K. W. W., et al. (2015). The role of subducted basalt in the source of island arc magmas: evidence from seafloor lavas of the Western Aleutians. J. Petrol. 56, 441–492. doi:10.1093/petrology/egv006
Zheng, Y.-F. (2019). Subduction zone geochemistry. Geoscience Frontiers. 10, 1223–1254. doi:10.1016/j.gsf.2019.02.003
Zhou, Q., Liu, L., and Hu, J. (2018). Western US volcanism due to intruding oceanic mantle driven by ancient Farallon slabs. Nat. Geosci. 11(1), 70–76. doi:10.1038/s41561-017-0035-y
Zhu, M.-S., Miao, L.-C., and Yang, S.-H. (2013). Genesis and evolution of subduction-zone andesites: evidence from melt inclusions. Int. Geol. Rev. 55, 1179–1190. doi:10.1080/00206814.2013.767527
Keywords: subduction, magmatism, basalt, volcanic arc, thermobarometry, Cascades, lithosphere
Citation: Leeman WP (2020) Old/New Subduction Zone Paradigms as Seen From the Cascades. Front. Earth Sci. 8:535879. doi: 10.3389/feart.2020.535879
Received: 17 February 2020; Accepted: 02 September 2020;
Published: 30 October 2020.
Edited by:
Matthias Konrad-Schmolke, University of Gothenburg, SwedenReviewed by:
Robert J. Stern, The University of Texas at Dallas, United StatesCopyright © 2020 Leeman. This is an open-access article distributed under the terms of the Creative Commons Attribution License (CC BY). The use, distribution or reproduction in other forums is permitted, provided the original author(s) and the copyright owner(s) are credited and that the original publication in this journal is cited, in accordance with accepted academic practice. No use, distribution or reproduction is permitted which does not comply with these terms.
*Correspondence: William P. Leeman, bGVlbWFuQHJpY2UuZWR1
Disclaimer: All claims expressed in this article are solely those of the authors and do not necessarily represent those of their affiliated organizations, or those of the publisher, the editors and the reviewers. Any product that may be evaluated in this article or claim that may be made by its manufacturer is not guaranteed or endorsed by the publisher.
Research integrity at Frontiers
Learn more about the work of our research integrity team to safeguard the quality of each article we publish.