- 1Instituto de Oceanografía y Cambio Global, IOCAG, Universidad de Las Palmas de Gran Canaria, Las Palmas de Gran Canaria, Spain
- 2OCEOMIC, Marine Bio and Technology S.L., Parque Tecnológico de Fuerteventura, Puerto del Rosario, Spain
- 3Department of Functional and Evolutionary Ecology, University of Vienna, Vienna, Austria
- 4Department of Climate Geochemistry, Max Planck Institute for Chemistry, Mainz, Germany
- 5Red Sea Research Center and Computational Bioscience Research Center, King Abdullah University of Science and Technology, Thuwal, Saudi Arabia
Plankton respiration (R) is a key factor governing the ocean carbon cycle. However, although the ocean supports respiratory activity throughout its entire volume, to our knowledge there are no studies that tackle both the spatial and temporal variability of respiration in the dark ocean and its dependence on organic carbon sources. Here, we have studied the variability of epipelagic and mesopelagic R via the enzymatic activity of the electron transport system (ETS) in microbial communities, along two zonal sections (21°N and 26°N) extending from the northwest African coastal upwelling to the open-ocean waters of the North Atlantic subtropical gyre, during the fall 2002 and the spring 2003. Overall, integrated R in epipelagic (Repi; 0–200 m) waters, was similar during the two periods, while integrated mesopelagic respiration (Rmeso; 200–1000 m) was >25% higher in the fall. The two seasons, however, exhibited contrasting zonal and meridional patterns of ETS distribution in the water column, largely influenced by upwelling effects and associated mesoscale variability. Multiple linear regression between average R and average concentrations of dissolved organic carbon (DOC) and slow-sinking (suspended) particulate organic carbon (POCsus) indicates that POCsus is the main contributor to Rmeso, supporting previous results in the same area. Rmeso exceeded satellite-derived net primary production (NPP) at all stations except at the most coastal ones, with the imbalance increasing offshore. Moreover, the export flux of sinking POC collected at 200 m with sediment traps, represented on average less than 6% of the NPP. All this indicates that Rmeso depends largely on small particles with low sinking rates, which would be laterally advected at mid water depths from the continental margin toward the open ocean, or transported by mesoscale features from the surface to the mesopelagic ocean, providing support to inferences from modeling studies in the region.
Introduction
Microplankton (<200 μm) respiration is one of the main metabolic processes controlling the organic carbon cycle in the ocean. The classical view of the biological pump considers that organic matter synthesized in the ocean is mostly respired by microplankton within surface waters, leaving a small surplus of organic matter to be transported vertically into the dark ocean. However, in contrast to this prevailing 1D conceptualization of organic carbon supply to the dark ocean, several studies have shown that lateral particulate organic carbon (POC) inputs from the ocean margins to the ocean interior could be more than an order of magnitude greater than inputs of vertically transported organic carbon derived from the surface (e.g., Bauer and Druffel, 1998; Alonso-González et al., 2009). These lateral inputs of organic matter would be particularly intense within eastern boundary currents (EBC), because of the high productivity of the nearby upwelling regions (Lovecchio et al., 2017) and the importance of horizontal advective processes in upwelling systems.
The Canary Current (CanC) is one of the four main EBC. Past studies in the coastal transition zone of this EBC have shown that upwelling filaments may transport offshore up to 50% of the carbon originated by primary production in the coastal upwelling (García-Muñoz et al., 2004, 2005; Santana-Falcón et al., 2017, 2020), accounting for 2.5–4.5 times the offshore carbon export driven by Ekman transport (Álvarez-Salgado et al., 2007) and up to 80% of the total offshore carbon flux (Lovecchio et al., 2018). Most of this exported carbon takes place at surface as semi-labile dissolved material (DOC). Nevertheless, it has been estimated that <20% of this DOC is respired in the coastal transition zone waters (Álvarez-Salgado et al., 2007), the remainder being transported and accumulated in the subtropical gyre (Hansell, 2002). In the mesopelagic zone, however, the off-shelf carbon transport is thought to be mainly supported by suspended particles transported in a prevailing horizontal direction along density gradients (e.g., Alonso-González et al., 2009; Vilas et al., 2009), except in regions of water mass subduction (Santana-Falcón et al., 2017), since the strong surface stratification limits semi-labile DOC mixing from surface waters to the deep ocean. Indeed, Alonso-González et al. (2009) estimated, through a box-model approach, that slow-sinking POC in the mesopelagic zone of the CanC may account for up to 60% of the total mesopelagic respiration during a low productivity period of the annual cycle. They suggested that a large fraction of this POC may originate in the nearby coastal upwelling region or be transported to depth by mesoscale eddies, being respired in the upper 1000 m of the CanC. However, limited information on the temporal and spatial variability of water-column respiration and its dependence on carbon sources in this EBC, as well as in any other EBC, have precluded empirical testing of the inferences derived from models.
We hypothesize that a significant fraction of the Rmeso will be supported by laterally advected POC due to the intense mesoscale activity characteristic of this and other EBC. To test this hypothesis, we characterized the spatial and temporal variability of microplankton respiration (R) in the CanC region, and quantify the contribution of both DOC and POC to R in the water column. We do so on the basis of results from two cruises that took place in the CanC from September 10 to October 1, 2002 (COCA I) and from May 21 to June 7, 2003 (COCA II), in a region affected by a strong mesoscale activity (Arístegui et al., 2004, 2009; Baltar et al., 2009a). We measured microplankton respiratory activity (ETS activity), as well as dissolved (DOC) and slow-sinking (suspended) particulate organic carbon (POCsus) down to 1000 m, at 10 stations placed along two sections extending from the African coast to the open ocean. Moreover, sinking POC was collected with free-floating sediment traps at the same stations.
Materials and Methods
Data Sources
The study was conducted along two zonal sections (21°N and 26°N) extending from the coastal upwelling to the open ocean at 26°W (Figure 1), and focused on the epipelagic (0–200 m) and mesopelagic (200–1000 m) zones, where microplankton contributes on a global average to > 90% of water column respiration (Arístegui et al., 2005). The cruises consisted of a total of 31 hydrographic stations and 10 biogeochemical stations, half of them along each section, which were roughly equidistant. At each station, conductivity, temperature and depth were determined by means of a Seabird 911 + CTD, mounted on a General Oceanics rosette sampler equipped with 24 10L-Niskin bottles, lowered down to 1000 m. Seawater samples were collected at fixed depths in the biogeochemical stations every 50 m for POCsus, DOC and ETS activity. Free-drifting sediment traps were deployed for 24 h at each biogeochemical station to collect sinking particles at 200 m depth.
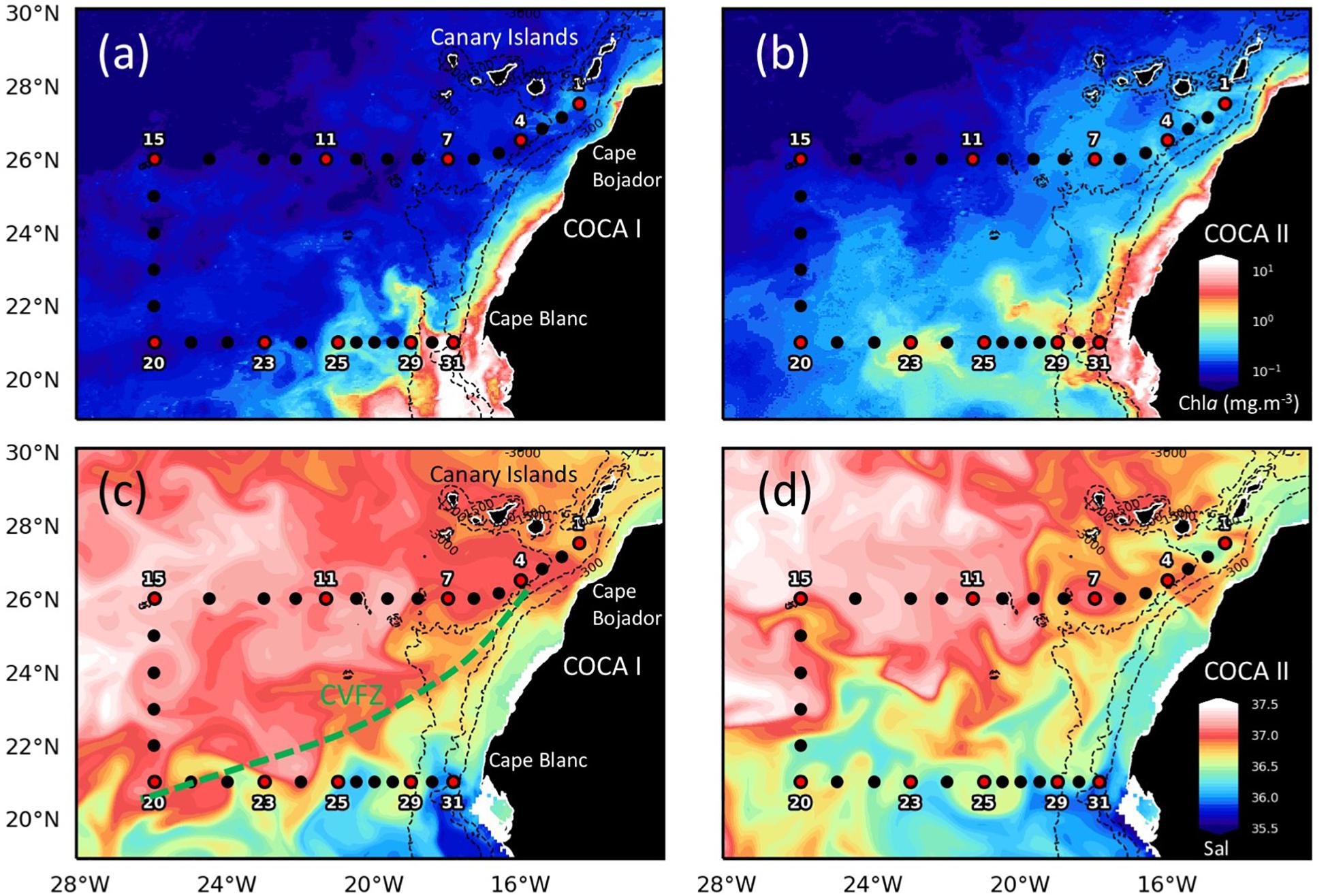
Figure 1. Maps of surface chlorophyll (a,b; Chla, mg m–3) and salinity at 25 m depth (c,d; Sal) indicating stations’ location along two sections (21°N and 26°N), extending from the NW African coast to the open ocean, during cruises COCA I (fall, 2002) and COCA II (spring, 2003). Black dots indicate CTD stations. Red dots with numbers indicate biogeochemical stations. The dashed green line represents the Cape Verde Frontal Zone (CVFZ), the boundary between North and South Atlantic Central Waters. Notice the northward displacement of the coastal upwelling and the CVFZ during the spring. Chlorophyll satellite observations for September 27, 2002 (COCA I) and May 31, 2003 (COCA II) were obtained from Global Ocean Satellite Observations. The images are based on the merging of the sensors SeaWiFS, MODIS, MERIS, VIIRS-SNPP&JPSS1 and OLCI-S3A&S3B. Salinity maps were plotted for the same dates, from numerical reanalysis, model NEMO (resolution 8 km), obtained from Copernicus Marine Environment Monitoring Services (CMEMS).
DOC Analysis
Water samples for dissolved organic carbon (DOC) were passed through an online acid-cleaned polycarbonate filter cartridge, holding a pre-combusted (500°C, 12 h) GF/F filter. The initial filtrate was discarded and the subsequent filtrate was dispensed into 10 ml glass ampoules pre-combusted likewise. 50 μL of H3PO4 was added immediately to the sample, sealed and stored at 4°C until analyzed with a Shimadzu TOC-5000 analyzer (Sharp et al., 1993). Before analysis, samples were sparged with CO2-free air for several minutes to remove inorganic carbon. DOC concentrations were determined from standard curves (30–200 μM) of potassium hydrogen phthalate produced every day (Thomas et al., 1995). To check accuracy and precision, reference material of deep-sea water (DSW, 42–45 μmol C L–1) and low carbon water (LCW, 1–2 μmol C L–1) provided by D. A. Hansell laboratory (University of Miami) was analyzed daily.
POC Analysis for Water Samples (POCsus) and Particles Collected With Sediment Traps
Water samples (2–4 L) for the analysis of POCsus were filtered onto pre-combusted (450°C, 12 h), 25-mm Whatman GF/F filters. For the measurement of sinking POC, particles were collected using a free-drifting multi-trap array holding eight cylinders (9 cm diameter: 50 cm length and 0.005 m2 collection area), similar to the model described by Knauer et al. (1979). NaCl (∼45 g L–1; analytical reagent-grade) was added to increase the salinity inside the traps in order to reach a high-density water. No poisons were used to retard bacterial decomposition. Upon recovery 24 h after deployment, samples were filtered onto pre-combusted (450°C, 12 h), 25 mm Whatman GF/F filters. Large swimming organisms were removed by wet sieving through a 1 mm nylon mesh, while organisms <1 mm were hand- picked under a microscope with fine-tweezers after filtration. All the filters for POC were wrapped in pre-combusted aluminum foil and frozen at −20°C until processed. In the laboratory the filters were thawed and dried overnight at 60°C, then placed overnight in a desiccator saturated with HCl fumes, dried again in a new desiccator with silica gel and packed in pre-combusted nickel sleeves. The carbon analyses were carried out on a Perkin–Elmer 2400 CHN elemental analyzer (UNESCO, 1994). The DOC adsorption onto GF/F filters (<12% of the POC signal) was subtracted from the samples to avoid overestimation of POC (Turnewitsch et al., 2007). For this, we analyzed blank filters in which water filtered by 0.4 μm pore was re-filtered through a pre-combusted 25 mm GF/F filter.
Respiratory Electron Transport System (ETS) Activity and Conversions to Actual Respiration Rates
Pre-filtered (<200 μm) seawater samples (10–20 L) were filtered through 47-mm Whatman GF/F glass-fiber filters at a low vacuum pressure (<0.3 atm). The filters were immediately stored in liquid nitrogen until being assayed in the laboratory within a few weeks. ETS determinations were carried out according to the Kenner and Ahmed (1975) modification of the tetrazolium reduction technique proposed by Packard (1971) as described in Arístegui and Montero (1995). The frozen filters were mechanically ground for 2 min by means of a drill equipped with a teflon bit in 5 ml of a cold homogenization buffer. The homogenate was centrifuged at 14,000 r.p.m. for 15 min at 0–3°C. An incubation time of 20 min at 18°C was selected from an earlier time and temperature kinetic study. ETS activities measured at 18°C were converted to activities at in situ temperatures by using the Arrhenius equation. A calculated mean activation energy of 16 kcal mol–1 was used. This activation energy value is similar to those obtained from other studies in oceanic regions (Arístegui and Montero, 1995).
Respiration rates in the epipelagic (Repi) were derived from temperature-corrected ETS activity applying the regression equation between R and ETS obtained by Arístegui and Montero (1995) for the global surface ocean. Respiration rates in the mesopelagic zone (Rmeso) were estimated by applying a R:ETS of 0.68, as obtained by Arístegui et al. (2005) in experiments performed with mesopelagic communities during COCA II. This ratio lies in the range of the ratios (R:ETS = 1.1, range = 0.6–1.7) observed by Christensen et al. (1980) for bacteria cultures during their exponential growth phase, being about 7–8 times higher that the ratio observed for the same cultures when reaching the senescent phase. This gives evidence that bacteria in the mesopelagic waters of the CanC were actively growing. To convert oxygen to carbon units we used respiratory quotients (RQ = CO2 per O2 by moles) of 0.719 and 0.645, estimated for the epipelagic and mesopelagic zones of this region, respectively (Fernández-Castro et al., 2019).
Satellite-Derived Net Primary Production (NPP)
Net primary production estimates at each station were obtained from the Eppley modified version of the Vertically Generalized Production Model (VGPM-Eppley; Behrenfeld and Falkowski, 1997). Eight-day averaged estimation with a 1/12° spatial resolution provided by the Oregon State University (OSU)1 were used. MODIS-aqua satellite-derived chlorophyll was selected as model input.
Data Analysis
In order to determine which source of organic matter (POCsus or DOC) present higher influence over R in the different zones (epipelagic and mesopelagic), multiple linear regression analysis was applied. Residuals from every model were tested for normality (Shapiro-Wilk test), homoscedasticity (Q-Q plots) and multicollinearity (Variance Inflation Factors, VIF). Following, the relative importance contribution (RI,%) of each predictor to the total explained variance (R2) of each model was calculated using the Lindeman, Merenda and Gold method (LMG; Lindeman et al., 1980). All paired comparisons were statistically tested by means of a Welch’s t-test. The confidence level was set at 95% (p = 0.05). All statistical analyses were performed with R-software2.
Results
Regional Oceanographic Settings
The two surveyed sections stretched across an area of high mesoscale variability, spanning from the NW Africa coastal upwelling region to >500 miles offshore into the subtropical open ocean waters of the CanC (Figure 1). The 21°N section intersected the Cape Verde Frontal Zone (CVFZ), the inner boundary of the CanC, characterized by the presence of numerous meanders and eddies, resulting from the interaction of high-salinity (>36.5) North Atlantic Central Water (NACW) and low-salinity (<36.5) South Atlantic Central Water (SACW). As a result, patches of high and low temperature and salinity waters are observed across this section in the upper 500 m (Figures 1–3). The 26°N section was placed north of the CVFZ and hence was entirely affected by NACW. Several upwelling filaments and mesoscale eddies were observed stretching off the coastal jet of the upwelling region, with the most intense at Cape Blanc (Figure 1). During the spring, the location of the CVFZ shifted to the north with respect to the fall, and the coastal upwelling was displaced and intensified northwards. Moreover, surface chlorophyll a (Chla) was higher in the spring than in the fall, particularly in open ocean waters (Figures 1a,b). A detailed description on the hydrography, circulation and mass transport in the region of study during the two cruises is described in Burgoa et al. (2020).
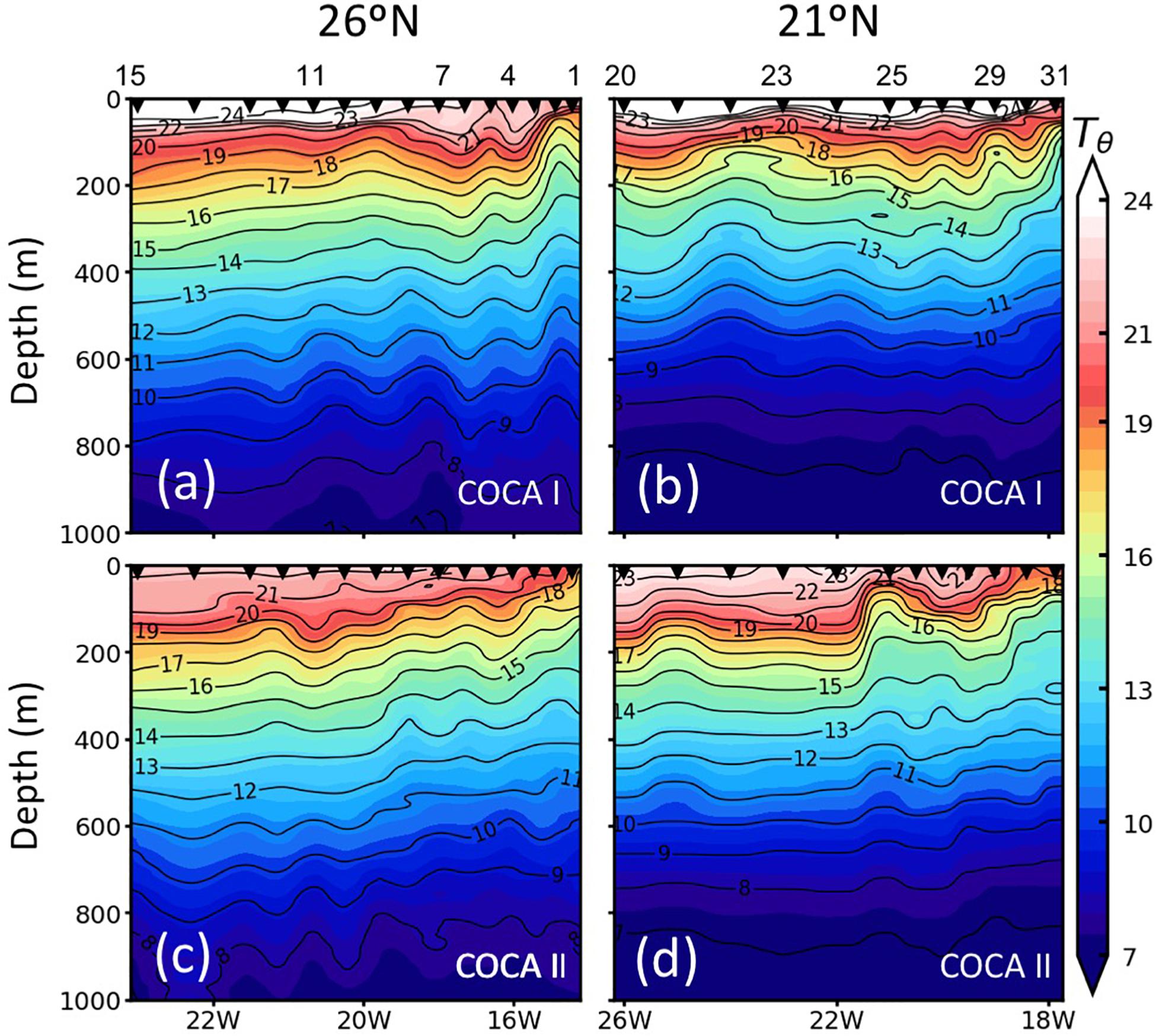
Figure 2. Vertical distribution (0–1000 m) of potential temperature (Tθ;°C) along the two sections (21°N and 26°N), during cruises COCA I (a,b) and COCA II (c,d).
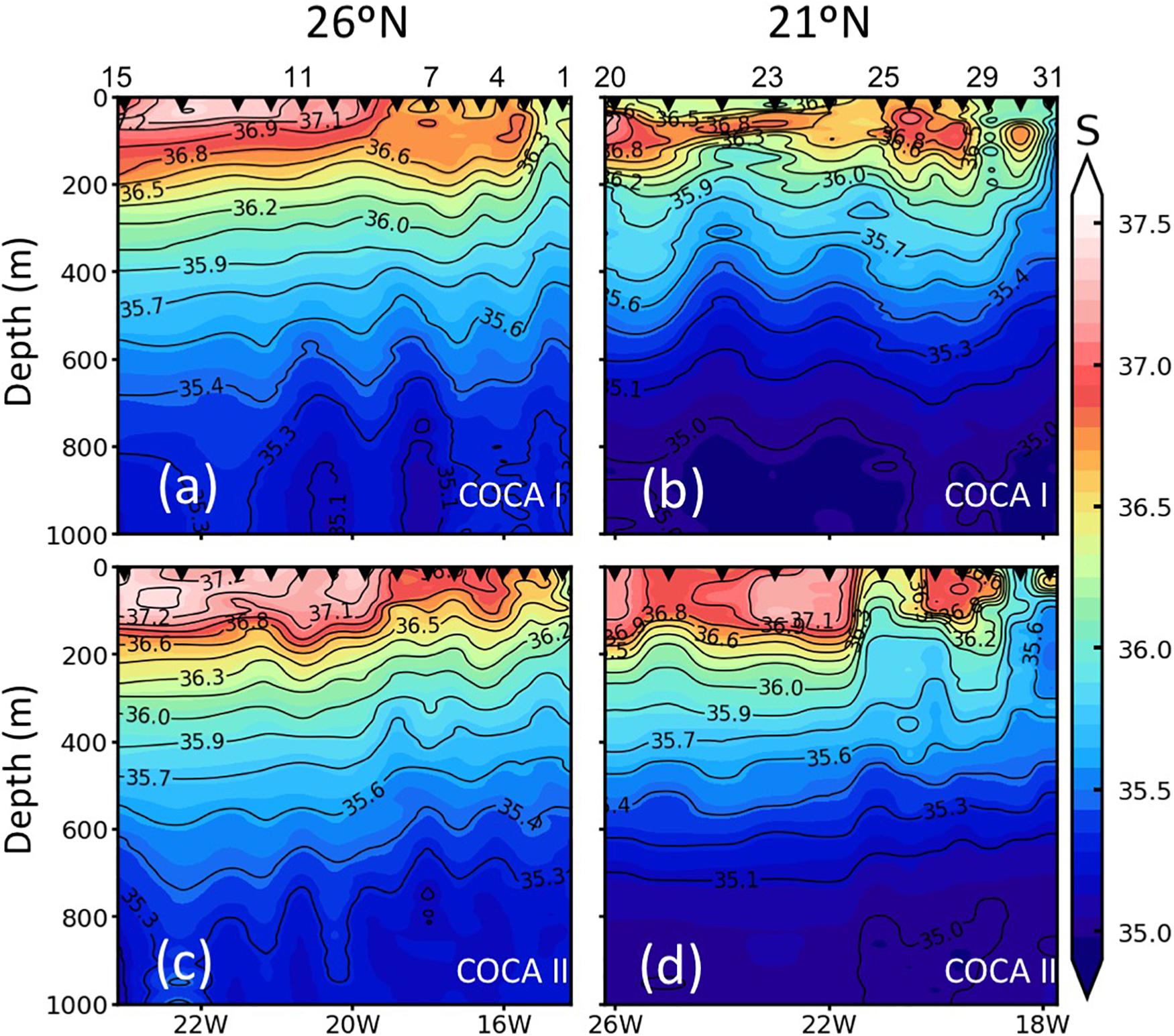
Figure 3. Vertical distribution (0–1000 m) of salinity (S) along the two sections (21°N and 26°N), during the COCA I (a,b) and COCA II (c,d).
Spatial and Temporal Variability in Respiratory Activity
Coastal-offshore sections of ETS activity for the two periods are shown in Figure 4. The epipelagic waters exhibited contrasting meridional and zonal patterns of distribution. ETS activity presented high values in the epipelagic waters (consuming 32.3 ± 26.0 and 36.4 ± 23.3 μmol O2 m–3 h–1 in the fall and the spring, respectively). ETS activity decreased with depth, although relative peaks were frequently observed in the mesopelagic zone, exceeding 10 μmol O2 m–3 h–1. At 21°N (Figures 4b,d), epipelagic ETS was highest at the two most coastal stations, due to the intense upwelling at the Cape Blanc region, decreasing toward the open ocean, although zonal variability across the CVFZ favored patchiness of lower and higher ETS associated with intrusions of water from the Cape Blanc filament. In contrast, at 26°N the surface open-ocean waters of the most distant stations presented a two to fivefold higher ETS activity (in the spring and the fall, respectively) compared to the two stations closer to the upwelling region (Figures 4a,c). The averaged integrated values in the epipelagic zone were always higher at 21°N than at 26°N (Table 1), but the differences were not significant due to the large zonal variability.
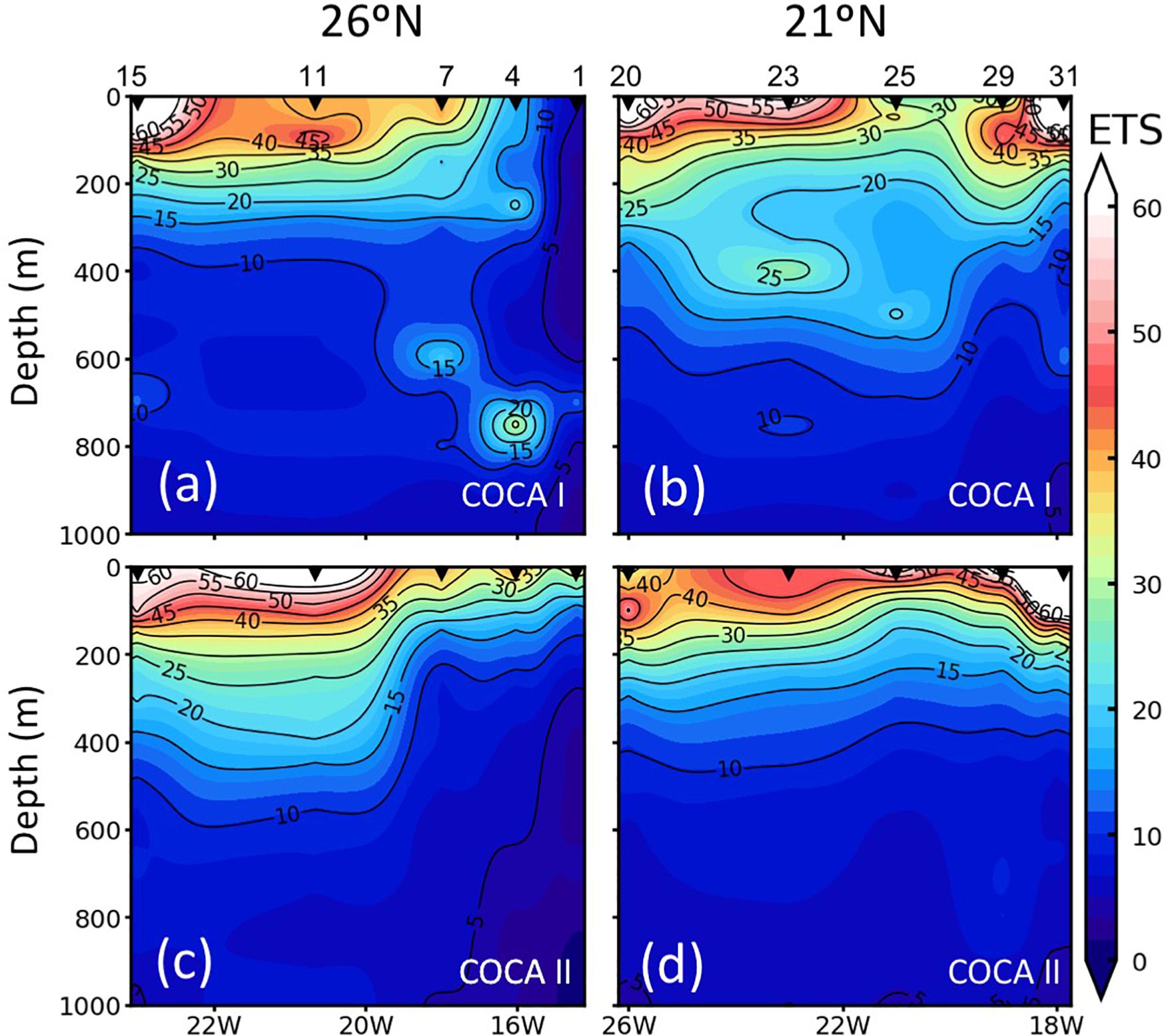
Figure 4. Vertical distribution (0–1000 m) of enzymatic respiratory activity (ETS; μmol O2 m–3 h–1) along the two sections (21°N and 26°N), during cruises COCA I (a,b) and COCA II (c,d).

Table 1. Average integrated enzymatic respiratory activity (ETS) and actual respiration (R) in the epipelagic and mesopelagic zones for cruises COCA I (fall 2002) and COCA II (spring 2003).
In contrast to the epipelagic, ETS activity in the mesopelagic zone did not display a clear meridional trend, in contrast to the epipelagic layer (Figure 4). However, averaged integrated ETS rates for the mesopelagic showed significantly (p < 0.01) higher values in the fall than in the spring at 21°N (Table 1), coinciding also with significantly higher DOC concentrations (see below).
DOC and POC Distribution and Contribution to Water Column Respiration
The depth distribution of average POCsus concentrations in the mesopelagic followed a similar pattern as the ETS activity, suggesting that this pool of organic matter was a main substrate supporting respiratory activity (Figure 5). Nevertheless, POCsus did not decrease exponentially with depth, as would be expected due to carbon remineralization of sinking material under the conventional assumption of 1D (vertical) dominance of transport, but rather showed similar high concentrations (>5 μM) across the mesopelagic zone; sometimes even higher than in the epipelagic zone (Figure 5). Average integrated values of POCsus presented meridional and temporal variability (Table 2). In the epipelagic zone POCsus was significantly higher at 21°N during the fall, while at 26°N it was higher in the spring than in the fall. In the mesopelagic zone POCsus was however significantly higher at 21°N during the spring.
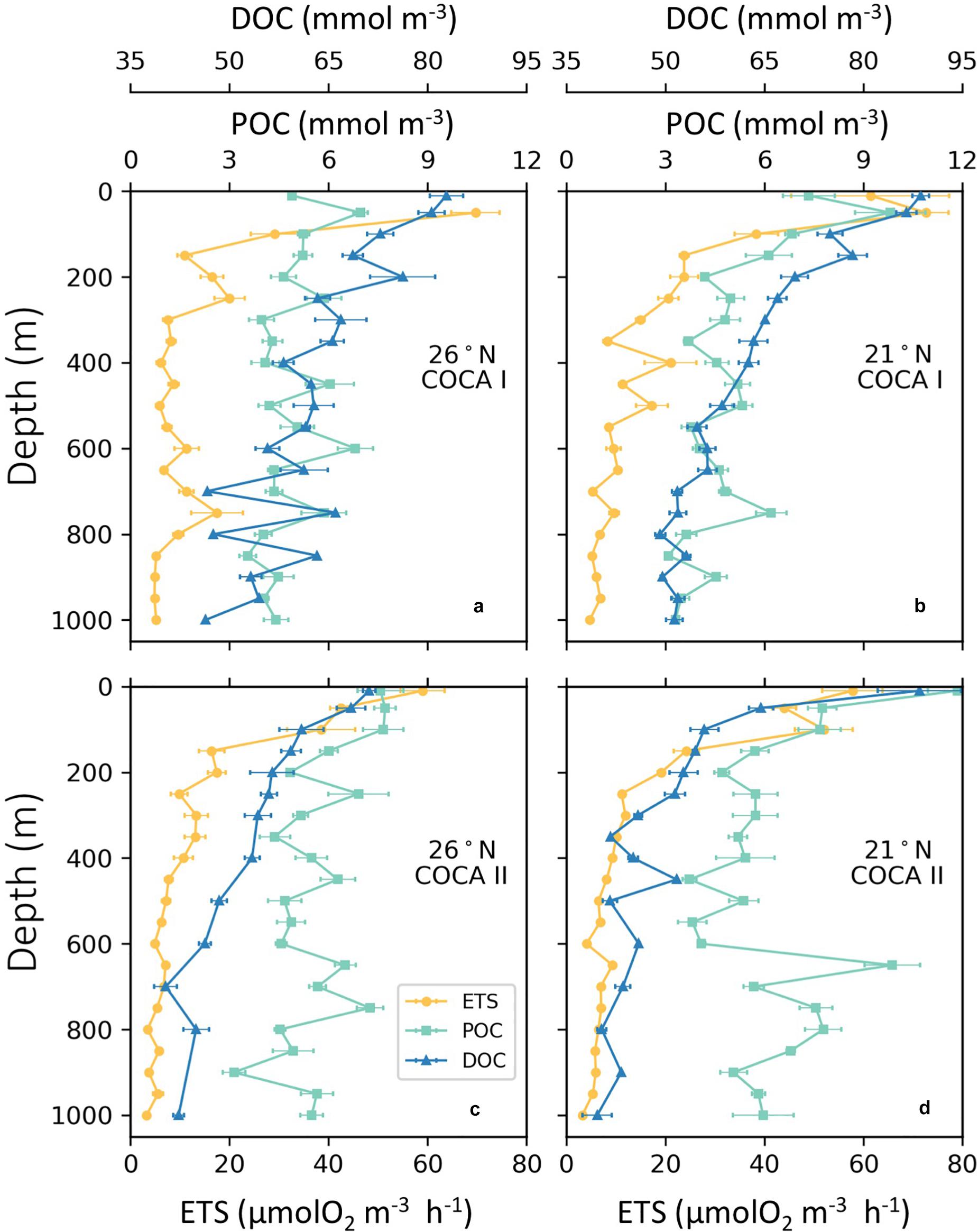
Figure 5. Vertical average distributions of enzymatic respiratory activity (ETS; μmol O2 m–3 h–1), dissolved organic carbon (DOC; μM) and suspended particulate organic carbon (POC; μM) of the two sections (21°N and 26°N), during cruises COCA I (a,b) and COCA II (c,d).

Table 2. Average integrated suspended particulate organic carbon (POCsus) and dissolved organic carbon in the epipelagic and mesopelagic zones for cruises COCA I (fall 2002) and COCA II (spring 2003).
DOC concentrations generally decreased with depth, although average integrated values (0–200 m and 200–1000 m) were significantly higher at 21°N during the fall than at 26°N in the same period, and higher than at the two sections in the spring (Table 2). Nevertheless, despite these differences in DOC concentrations between the two cruises (>8 mmol C m–2 d–1 for the epipelagic and >25 mmol C m–2 d–1 for the mesopelagic), multiple regression analyses of R, POCsus and DOC (Table 3) indicate that the relative importance (RI,%) of POCsus to R was always higher than of DOC, both in the epipelagic and mesopelagic zones.
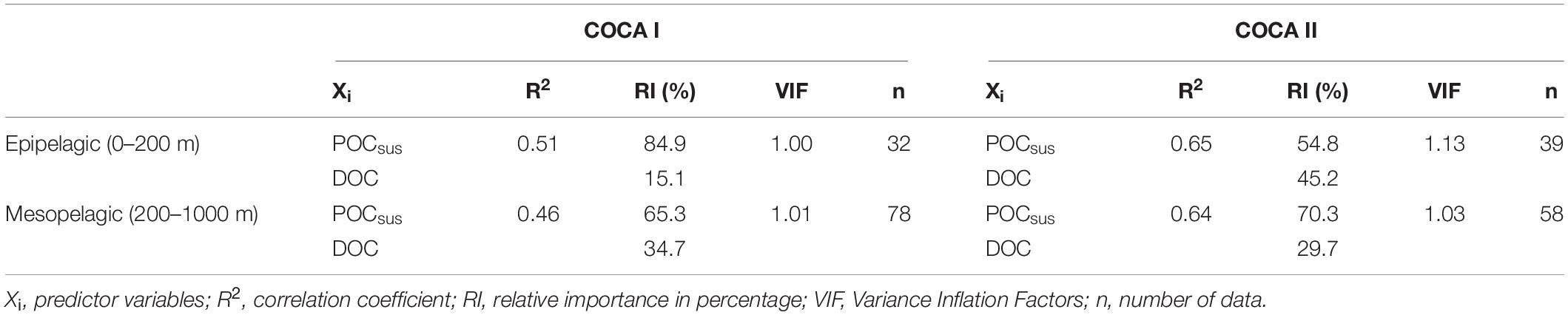
Table 3. Multiple linear regression statistics between actual respiration rates and suspended particulate organic carbon (POCsus) and dissolved organic carbon (DOC), for the epipelagic and mesopelagic zones in cruises COCA I and COCA II.
Discussion
Epipelagic Respiration
Average integrated epipelagic R rates in this study (75–125 mmol C m–2 d–1) are higher than those reported from open ocean waters of the CanC north of the Canary Islands (<60 mmol C m–2 d–1; Arístegui and Harrison, 2002; Fernández-Castro et al., 2016), but similar to those previously estimated for the coastal transition zone of the CanC (60–120 mmol C m–2 d–1; Arístegui et al., 2003b). The high epipelagic R coincides with high POC concentrations in the two studies south of the islands (Table 2; Arístegui et al., 2003b). The epipelagic average integrated values of POCsus in these two studies (>1 mol C m–2) are also significantly higher than values observed in previous studies at the ESTOC station, north of the Canary Archipelago (Neuer et al., 2007), or in the more oceanic sector of the CanC (Alonso-González et al., 2009).
POC inputs to the epipelagic zone in our region of study can be due to local primary production or lateral advection from the coast. Stations closer to the coastal upwelling present NPP values up to 10 times higher than in the open ocean (Table 4), although epipelagic R is sometimes higher in the open ocean and clearly exceeds NPP (Figure 4). Therefore, in addition to the in situ production rates, the high respiratory activity needs be supported by horizontal inputs of coastal organic matter, consistent with existing modeling predictions. Indeed, offshore POC and DOC transports from the continental margin by upwelling filaments in this region have been reported, from both observational (Gabric et al., 1993; García-Muñoz et al., 2004, 2005; Santana-Falcón et al., 2017) and modeling (Lovecchio et al., 2017, 2018; Santana-Falcón et al., 2020) studies, representing an important carbon source to the open ocean, reaching up to 50% of the NPP in the coastal upwelling (Álvarez-Salgado et al., 2007). This offshore export would explain former studies carried out in this same area describing a metabolic imbalance in the balance between primary production and microplankton respiration in the epipelagic zone (Duarte et al., 2001; Robinson et al., 2002; Arístegui et al., 2003b; Fernández-Castro et al., 2016). Indeed, assuming data that NPP in the coastal upwelling ranged approximately from 100 to 300 mmol C m–2 d–1 and that average epipelagic R ranged from 75 to125 mmol C m–2 d–1 (Table 4), a 50% export of NPP would suffice in excess to explain the metabolic imbalance, summing up the local NPP at each station.
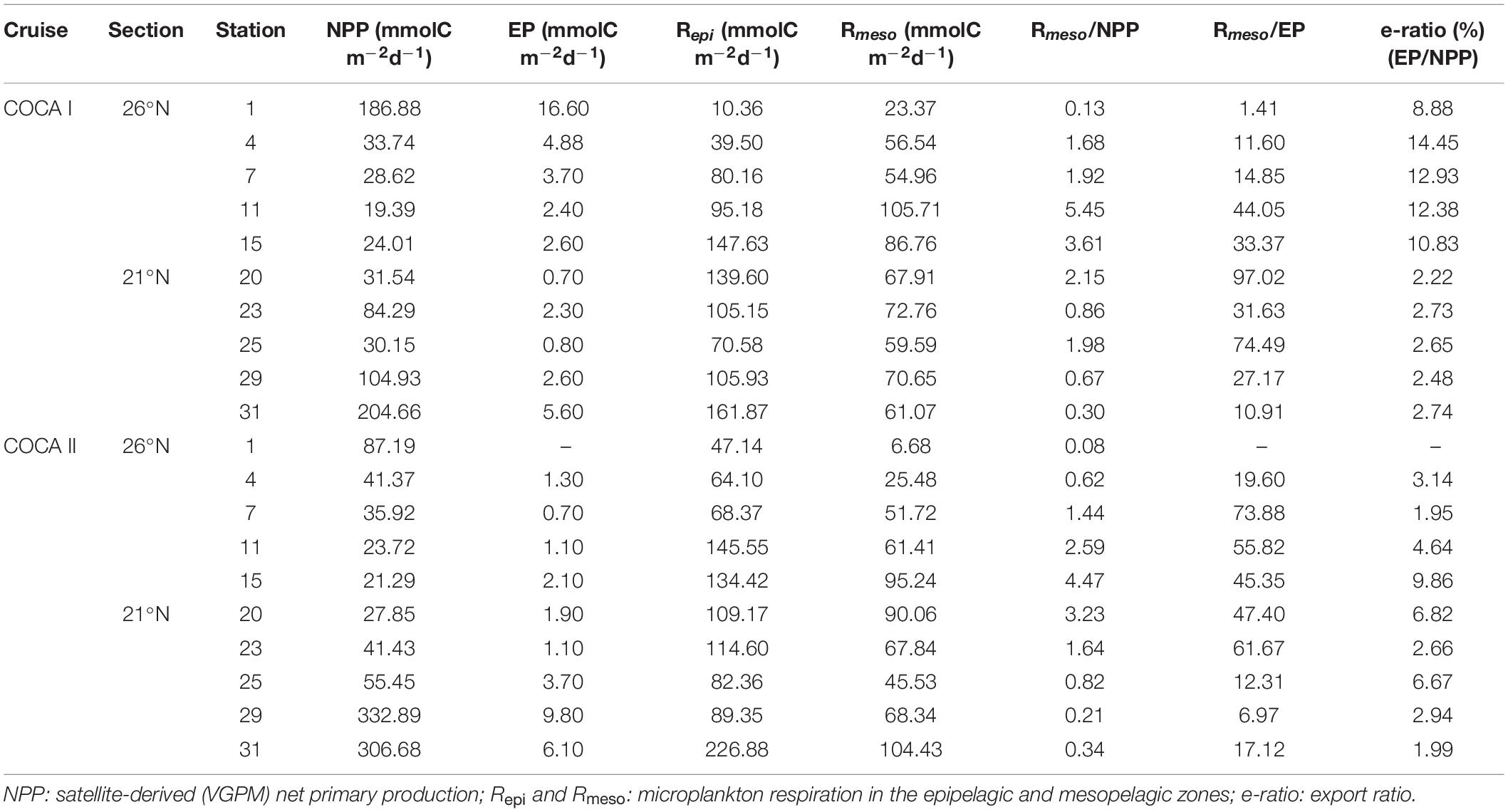
Table 4. Integrated plankton metabolic rates (NPP, R; see methods for calculations) and export production (EP: sinking POC flux collected with sediment traps at 200 m depth) at each station during the two cruises.
Mesopelagic Respiration
The mesopelagic layer supports intense organic matter remineralization (Arístegui et al., 2003a, 2005). Indeed, the major decrease in molecular-characterized material occurs in the mesopelagic zone, even though the larger relative decrease in POC flux of sinking particles is in the euphotic zone (Lee et al., 2004). However, in spite of the key role of the mesopelagic waters as the site where the bulk of exported matter from the epipelagic zone is remineralized (Arístegui et al., 2005; Burd and Jackson, 2009), to our knowledge there are no studies that tackle both the spatial and temporal variability of respiration in the dark ocean, which has been hitherto assumed to be rather invariant.
In our study, the mesopelagic waters did not reveal any clear meridional or zonal trend in respiratory activity for the two cruises, although on average there was >25% increase in R in the fall with respect to the spring (Figure 4 and Table 1). This difference could be partly explained by significantly higher DOC concentrations in the fall, particularly at 21°N (Table 2), when the relative importance of DOC to R accounts for up to 35% (Table 3). In any case, POCsus contributes more than DOC to R in all cases (Table 3). This agrees with the results obtained by Alonso-González et al. (2009) from a box-model approach in the more oceanic section of our region of study. It coincides also with the estimations obtained from a tracer conservation model at the ESTOC station north of Gran Canaria, where lateral supply of POCsus would be the main carbon source to explain mesopelagic R (Fernández-Castro et al., 2016). Baltar et al. (2009b), also found significant correlations between POCsus and ETS in bathypelagic waters (1000–3000 m) of the subtropical North Atlantic. All these findings, together with our results, indicate that microbial metabolism in the mesopelagic waters of the CanC region is largely supported by suspended organic particles. These finding are also consistent with a recent global ocean multi-omics study suggesting that organic matter cleavage is mediated mainly by particle-associated prokaryotes releasing their extracellular enzymes into diffusion-limited particles in the meso- and bathypelagic realm (Zhao et al., 2020).
The correlation between the particulate fraction of the organic matter pool and ETS activity of planktonic communities (and hence R) may be expected beforehand, taking into account that ETS activity also represents a living biomass (enzyme) measurement. However, total prokaryote abundances in the sampled mesopelagic waters represented only a small fraction of total POCsus. Free living prokaryote abundances ranged from 1 to 3 × 108 cells l–1 (Gasol et al., 2009). Assuming an average carbon conversion factor of 20 fg cell–1 (Fukuda et al., 1998) the maximum prokaryote abundance (3 × 108 cells l–1) yields a prokaryotic carbon content of 0.5 μmol C l–1. This value represents less than 10% of total POCsus and suggests that the correlation between ETS activity and POCsus is independent on the living biomass fraction.
Overall, average integrated estimates of R in the mesopelagic zone (65–98 mmol C m–2 d–1) are of the same order as in the epipelagic, indicating that prokaryotes are metabolically active in the dark ocean, as evidenced in a former study from COCA II (Arístegui et al., 2005). The upper average integrated range of Rmeso in our study is however up to 10 times higher than the upper range of Rmeso inferred from physical-biogeochemical models at ESTOC (Fernández-Castro et al., 2016) and the more oceanic waters of our region of study (Alonso-González et al., 2009). A priori, one would expect Rmeso to be higher in our region of study, due to enhanced offshore export of organic matter from the coastal upwelling and the higher POCsus values observed in the water column compared with the other studies. However, differences in methodological approaches (biogeochemical modeling vs. ETS activity) preclude direct comparison of the magnitude of R determined between these studies.
We must have, however, some precautions with regards to our R estimations from ETS activity in the two cruises. Contrary to other studies that assume a theoretical R:ETS, we measured actual R by oxygen consumption in 29 experiments with water collected from 600 and 1000 m depth, but only during COCA II. We also measured at the same stations and depths ETS activity, obtaining an average R:ETS ratio of 0.68 ± 0.11 (Arístegui et al., 2005). This ratio is 7.5 times higher than the R:ETS obtained from senescent bacteria cultures (R:ETS = 0.09; Christensen et al., 1980), that was used in the past to derive a lower-threshold R for the global ocean (Arístegui et al., 2003a). Nevertheless, during COCA II, other proxies for metabolic activity gave evidence that mesopelagic prokaryotic assemblages were activity growing, rather than in senescent phase. We cannot be sure however that the same conditions applied also for cruise COCA I. If prokaryotic activity were lower during the fall than the spring, then the R:ETS could be lower, as well as the mesopelagic R. In any case, even assuming that the R:ETS ratio was two times lower during the fall, mesopelagic R would exceed by far the export production measured by gravitational flux (EP: sinking POC flux at 200 m depth).
Synthesis: Carbon Fluxes and Metabolic Balance
Table 4 collates the integrated plankton metabolic rates (NPP and R) and compare them with the EP at each station during the two cruises. Only at the very coastal stations of the two sections does NPP exceed Rmeso. In offshore waters, Rmeso is up to five times higher than NPP, suggesting that much of the organic carbon respired must originate in the more productive coastal upwelling region. Moreover, the e-ratio (% EP/NPP) ranges from 2 to 14%, being <3% at most of the stations, indicating that only a small fraction of the NPP is exported from the surface by local gravitational flux.
If we sum up the average gravitational flux (EP = 4.2 ± 4.6) with the active flux mediated by migrant zooplankton (0.8 ± 1.5 mmol C m–2 d–1) estimated for COCA I (Hernández-León et al., 2019), both fluxes fail short of accounting for the respiratory rates in the mesopelagic at any of the stations, with the respiratory imbalance being up to > 90 times higher in one of the most oceanic stations.
The average EP in the fall (4.2 ± 4.6 mmol C m–2 d–1) and the spring (3.1 ± 3.0 mmol Cm–2 d–1) are significantly lower than previous EP reported for late winter (8.3–28.5 mmol C m–2 d–1) and summer (5.5–13–1 mmol C m–2 d–1) in open ocean waters of the CanC (Alonso-González et al., 2010). However, in any case the EP can account for the high Rmeso in the region. This must be maintained by lateral export of organic carbon from the coastal upwelling region. Indeed, Lovecchio et al. (2017), by means of a coupled physical-ecosystem model, described a strong offshore flux of organic carbon from the NW Africa coastal upwelling, that would be particularly efficient near Cape Blanc, transporting as nearly 60% of the local NPP.
The offshore transport is largely mediated by the numerous upwelling filaments (Gabric et al., 1993; García-Muñoz et al., 2004, 2005; Santana-Falcón et al., 2016, 2020) and filament-eddy interactions, produced particularly south of the Canaries Archipelago (Arístegui et al., 2004, 2009). Mesoscale eddies would transport organic carbon to the mesopelagic zone, by means of physical processes (e.g., Omand et al., 2015; Resplandy et al., 2019), explaining the high concentrations and irregular peaks of POCsus (and ETS activity) in deep layers observed at most of the stations. Recently, Amos et al. (2019) used satellite-derived measurements of POC to show that mesocale eddies generated from meanders in the California Current, trap organic carbon and transport it as far as 1000 km offshore, greatly enriching the open ocean waters. Lovecchio et al. (2018) ran simulations with a coupled physical-ecosystem model to show that both filaments and eddies contribute to the offshore flux of organic carbon in the CanC upwelling system, although in a different way. Filaments would transport organic carbon offshore in a very intense way but near the coast, while at distances beyond 500 km from the shore eddies would dominate the mesoscale offshore transport.
Conclusion
The estimated integrated mesopelagic respiratory activity was comparable in magnitude to that in the epipelagic zone, and higher than in other reported studies from more open-ocean waters in the North Atlantic. This finding provides evidence of the importance of the dark waters of the CanC EBC (and probably of other EBC) as a sink of organic carbon in the ocean. The epipelagic waters revealed a clear meridional variability with the highest respiratory rates along the 21°N section, closer to the intense upwelling filament off Cape Blanc. Mesopelagic waters, however, did not show clear zonal or meridional patterns of variability, although integrated respiration rates were significantly different during the two periods of study. Water-column respiration was largely supported by suspended POC, which presented concentrations in depth comparable to the epipelagic zone. Our results suggest that microbial respiratory activity in this area is likely controlled by seasonal fluctuations in suspended particulate organic carbon advected from productive coastal waters, rather than by sinking particles from the euphotic zone or the active flux by migrants, which together represented a very small fraction of the carbon respired by microorganisms. The main source of the respired POC is through offshore export from the coastal upwelling, mostly mediated by filaments, mesoscale eddies and the interaction among them. This study also underlines the fact that respiration in the mesopelagic zone does not occur uniformly over time and space in the ocean. Hence, the urgent need to expand our database on mesopelagic respiration at regional and basin scales, reconciling ecological, biogeochemical and model estimates, to gain a better understanding of the global carbon cycle in the ocean.
Data Availability Statement
The raw data supporting the conclusions of this article will be made available by the authors, without undue reservation, to any qualified researcher.
Author Contributions
JA and CD designed the field work. JA, MC, and CD participated in the sampling. JA wrote the manuscript with inputs from all co-authors. All authors contributed to samples’ analyses.
Funding
This work was a contribution to projects COCA (REN2000 1471-C02-01-MAR), FLUXES (CTM2015- 69392-C3-1-R), and e-IMPACT (PID2019-109084RB-C2), funded by the Spanish “Plan Nacional de I+D” and to projects SUMMER (AMD-817806-5) and TRIATLAS (AMD-817578-5) funded from the European Union’s Horizon 2020 Research and Innovation Programme.
Conflict of Interest
The authors declare that the research was conducted in the absence of any commercial or financial relationships that could be construed as a potential conflict of interest.
Acknowledgments
We thank the captain, crew, and technical staff of the B.I.O Hespérides for their support at sea, and to J. C. Vilas and M. Espino for their help in sampling and data analyses. Some of the contents of this manuscript formed part of the Ph.D. thesis of Alonso-González (2011).
Footnotes
References
Alonso-González, I. J. (2011). New Insights into POC Dynamics in the Subtropical Northeast Atlantic Ocean. Ph.D. Thesis, Universidad de Las Palmas de Gran Canaria Las Palmas.
Alonso-González, I. J., Arístegui, J., Lee, C., and Calafat, A. (2010). Regional and temporal variability of sinking organic matter in the subtropical northeast Atlantic Ocean: a biomarker diagnosis. Biogeosciences 7, 2101–2115. doi: 10.5194/bg-7-2101-2010
Alonso-González, I. J., Arístegui, J., Vilas, J. C., and Hernández-Guerra, A. (2009). Lateral POC transport and consumption in surface and deep waters of the Canary Current region: a box model study. Glob. Biogeochem. Cycles 23, 1–12. doi: 10.1029/2008GB003185
Álvarez-Salgado, X. A., Arístegui, J., Barton, E. D., and Hansell, D. A. (2007). Contribution of upwelling filaments to offshore carbon export in the subtropical Northeast Atlantic Ocean. Limnol. Oceanogr. 52, 1287–1292. doi: 10.4319/lo.2007.52.3.1287
Amos, C. M., Castelao, R. M., and Medeiros, P. M. (2019). Offshore transport of particulate organic carbon in the California Current System by mesoscale eddies. Nat. Commun. 10:4940. doi: 10.1038/s41467-019-12783-12785
Arístegui, J., Agustí, S., and Duarte, C. M. (2003a). Respiration in the dark ocean. Geophys. Res. Lett. 30:1041. doi: 10.1029/2002GL016227
Arístegui, J., Barton, E. D., Álvarez-Salgado, X. A., Santos, A. M. P., Figueiras, F. G., Kifani, S., et al. (2009). Sub-regional ecosystem variability in the Canary Current upwelling. Prog. Oceanogr. 83, 33–48. doi: 10.1016/j.pocean.2009.07.031
Arístegui, J., Barton, E. D., Montero, M. F., García-Muñoz, M., and Escánez, J. (2003b). Organic carbon distribution and water column respiration in the NW Africa-Canaries Coastal Transition Zone. Aquat. Microb. Ecol. 33, 289–301. doi: 10.3354/ame033289
Arístegui, J., Barton, E. D., Tett, P., Montero, M. F., García-Muñoz, M., Basterretxea, G., et al. (2004). Variability in plankton community structure, metabolism, and vertical carbon fluxes along an upwelling filament (Cape Juby, NW Africa). Prog. Oceanogr. 62, 95–113. doi: 10.1016/j.pocean.2004.07.004
Arístegui, J., Duarte, C. M., Gasol, J. M., and Alonso-Sáez, L. (2005). Active mesopelagic prokaryotes support high respiration in the subtropical northeast Atlantic Ocean. Geophys. Res. Lett. 32, 1–4. doi: 10.1029/2004GL021863
Arístegui, J., and Harrison, W. G. (2002). Decoupling of primary production and community respiration in the ocean: implications for regional carbon studies. Aquat. Microb. Ecol. 29, 199–209. doi: 10.3354/ame029199
Arístegui, J., and Montero, M. F. (1995). The relationship between community respiration and ETS activity in the ocean. J. Plankton Res. 17, 1563–1571. doi: 10.1093/plankt/17.7.1563
Baltar, F., Arístegui, J., Gasol, J. M., Sintes, E., and Herndl, G. J. (2009a). Evidence of prokaryotic metabolism on suspended particulate organic matter in the dark waters of the subtropical North Atlantic. Limnol. Oceanogr. 54, 182–193. doi: 10.4319/lo.2009.54.1.0182
Baltar, F., Arístegui, J., Montero, M. F., Espino, M., Gasol, J. M., and Herndl, G. J. (2009b). Mesoscale variability modulates seasonal changes in the trophic structure of nano- and picoplankton communities across the NW Africa-Canary Islands transition zone. Prog. Oceanogr. 83, 180–188. doi: 10.1016/j.pocean.2009.07.016
Bauer, J. E., and Druffel, E. R. M. (1998). Ocean margins as a significant source of organic matter to the deep open ocean. Nature 392, 482–485. doi: 10.1038/33122
Behrenfeld, M. J., and Falkowski, P. G. (1997). Photosynthetic rates derived from satellite-based chlorophyll concentration. Limnol. Oceanogr. 42, 1–20. doi: 10.4319/lo.1997.42.1.0001
Burd, A. B., and Jackson, G. A. (2009). Particle aggregation. Ann. Rev. Mar. Sci. 1, 65–90. doi: 10.1146/annurev.marine.010908.163904
Burgoa, N., Machín, F., Marrero-Díaz, Á., Rodríguez-Santana, Á., Martínez-Marrero, A., Arístegui, J., et al. (2020). Mass, nutrients and dissolved organci carbon (DOC) lateral transports off northwest Africa during fall 2002. Ocean Sci. 16, 483–511. doi: 10.5194/os-16-483-2020
Christensen, J. P., Owens, T. G., Devol, A. H., and Packard, T. T. (1980). Respiration and physiological state in marine bacteria. Mar. Biol. 55, 267–276. doi: 10.1007/bf00393779
Duarte, C. M., Agustí, S., Arístegui, J., González, N., and Anadón, R. (2001). Evidence for a heterotrophic subtropical northeast Atlantic. Limnol. Oceanogr. 46, 425–428. doi: 10.4319/lo.2001.46.2.0425
Fernández-Castro, B., Arístegui, J., Anderson, L., Montero, M. F., Hernández-León, S., Marañón, E., et al. (2016). Mesopelagic respiration near the ESTOC (European Station for Time-Series in the Ocean, 15.5°W, 29.1°N) site inferred from a tracer conservation model. Deep Sea Res. I Oceanogr. Res. Pap. 115, 63–73. doi: 10.1016/J.DSR.2016.05.010
Fernández-Castro, B., Mouriño-Carballido, B., and Álvarez-Salgado, X. A. (2019). Non-redfieldian mesopelagic nutrient remineralization in the eastern North Atlantic subtropical gyre. Prog. Oceanogr. 171, 136–153. doi: 10.1016/j.pocean.2018.12.001
Fukuda, R., Ogawa, H., Nagata, T., and Koike, I. I (1998). Direct determination of carbon and nitrogen contents of natural bacterial assemblages in marine environments. Appl. Environ. Microbiol. 64, 3352–3358. doi: 10.1128/aem.64.9.3352-3358.1998
Gabric, A. J., Garcia, L., Van Camp, L., Nykjaer, L., Eifler, W., and Schrimpf, W. (1993). Offshore export of shelf production in the Cape Blanc (Mauritania) giant filament as derived from coastal zone color scanner imagery. J. Geophys. Res. Ocean. 98, 4697–4712. doi: 10.1029/92JC01714
García-Muñoz, M., Arístegui, J., Montero, M. F., and Barton, E. D. (2004). Distribution and transport of organic matter along a filament-eddy system in the Canaries - NW Africa coastal transition zone region. Prog. Oceanogr. 62, 115–129. doi: 10.1016/j.pocean.2004.07.005
García-Muñoz, M., Arístegui, J., Pelegrí, J. L., Antoranz, A., Ojeda, A., and Torres, M. (2005). Exchange of carbon by an upwelling filament off Cape Ghir (NW Africa). J. Mar. Syst. 54, 83–95. doi: 10.1016/j.jmarsys.2004.07.005
Gasol, J. M., Alonso-Sáez, L., Vaqué, D., Baltar, F., Calleja, M. L., Duarte, C. M., et al. (2009). Mesopelagic prokaryotic bulk and single-cell heterotrophic activity and community composition in the NW Africa–Canary Islands coastal-transition zone. Prog. Oceanogr. 83, 189–196. doi: 10.1016/J.POCEAN.2009.07.014
Hansell, D. A. (2002). “DOC in the global ocean carbon cycle,” in Biogeochemistry of Marine Dissolved Organic Matter, eds D. A. Hansell and C. A. Carlson (San Diego: Academic Press), 685–715. doi: 10.1016/b978-012323841-2/50017-8
Hernández-León, S., Putzeys, S., Almeida, C., Bécognée, P., Marrero-Díaz, A., Arístegui, J., et al. (2019). Carbon export through zooplankton active flux in the Canary Current. J. Mar. Syst. 189, 12–21. doi: 10.1016/j.jmarsys.2018.09.002
Kenner, R. A., and Ahmed, S. I. (1975). Measurements of electron transport activities in marine phytoplankton. Mar. Biol. 33, 119–127. doi: 10.1007/BF00390716
Knauer, G. A., Martin, J. H., and Bruland, K. W. (1979). Fluxes of particulate carbon, nitrogen, and phosphorus in the upper water column of the northeast Pacific. Deep Sea Res. A. Oceanogr. Res. Pap. 26, 97–108. doi: 10.1016/0198-0149(79)90089-X
Lee, C., Wakeham, S., and Arnosti, C. (2004). Particulate organic matter in the sea: the composition conundrum. Ambio 33, 565–575. doi: 10.1579/0044-7447-33.8.565
Lindeman, R. H., Merenda, P. F., and Gold, R. Z. (1980). Introduction to Bivariate and Multivariate Analysis. Glenview, IL: Scott, Foresman and Company.
Lovecchio, E., Gruber, N., and Münnich, M. (2018). Mesoscale contribution to the long-range offshore transport of organic carbon from the Canary Upwelling System to the open North Atlantic. Biogeosciences 15, 5061–5091. doi: 10.5194/bg-15-5061-2018
Lovecchio, E., Gruber, N., Münnich, M., and Lachkar, Z. (2017). On the long-range offshore transport of organic carbon from the Canary Upwelling System to the open North Atlantic. Biogeosciences 14, 3337–3369. doi: 10.5194/bg-14-3337-2017
Neuer, S., Cianca, A., Helmke, P., Freudenthal, T., Davenport, R., Meggers, H., et al. (2007). Biogeochemistry and hydrography in the eastern subtropical North Atlantic gyre. Results from the European time-series station ESTOC. Prog. Oceanogr. 72, 1–29. doi: 10.1016/J.POCEAN.2006.08.001
Omand, M. M., D’Asaro, E. A., Lee, C. M., Perry, M. J., Briggs, N., Cetini, I., et al. (2015). Eddy-driven subduction exports particulate organic carbon from the spring bloom. Science 348, 22–25. doi: 10.1126/science.1260062
Packard, T. T. (1971). The measurement of respiratory electron-transport activity in marine phytoplankton. J. Mar. Res. 29, 235–144.
Resplandy, L., Lévy, M., and McGillicuddy, D. J. Jr. (2019). Effects of eddy-driven subduction on ocean biological carbon pump. Glob. Biogeochem. Cycles 33, 1071–1084. doi: 10.1029/2018GB006125
Robinson, C., Serret, P., Tilstone, G., Teira, E., Zubkov, M. V., Rees, A. P., et al. (2002). Plankton respiration in the Eastern Atlantic Ocean. Deep Sea Res. I Oceanogr. Res. Pap. 49, 787–813. doi: 10.1016/S0967-0637(01)00083-88
Santana-Falcón, Y., Álvarez-Salgado, X. A., Pérez-Hernández, M. D., Hernández-Guerra, A., Mason, E., and Arístegui, J. (2017). Organic carbon budget for the eastern boundary of the North Atlantic subtropical gyre: major role of DOC in mesopelagic respiration. Sci. Rep. 7, 1–12. doi: 10.1038/s41598-017-10974-y
Santana-Falcón, Y., Benavides, M., Sangrá, P., Mason, E., Barton, E. D., Orbi, A., et al. (2016). Coastal-offshore exchange of organic matter across the Cape Ghir filament (NW Africa) during moderate upwelling. J. Mar. Sys. 154, 233–242. doi: 10.1016/j.jmarsys.2015.10.008
Santana-Falcón, Y., Mason, E., and Arístegui, J. (2020). Offshore transport of organic carbon by upwelling filaments in the Canary Current System. Prog. Oceanogr. 186:102322. doi: 10.1016/J.POCEAN.2020.102322
Sharp, J. H., Peltzer, E. T., Alperin, M. J., Cauwet, G., Farrington, J. W., Fry, B., et al. (1993). Procedures subgroup report. Mar. Chem. 41, 37–49. doi: 10.1016/0304-4203(93)90104-V
Thomas, C., Cauwet, G., and Minster, J.-F. (1995). Dissolved organic carbon in the equatorial Atlantic Ocean. Mar. Chem. 49, 155–169. doi: 10.1016/0304-4203(94)00061-H
Turnewitsch, R., Springer, B. M., Kiriakoulakis, K., Vilas, J. C., Arístegui, J., Wolff, G., et al. (2007). Determination of particulate organic carbon (POC) in seawater: the relative methodological importance of artificial gains and losses in two glass-fiber-filter-based techniques. Mar. Chem. 105, 208–228. doi: 10.1016/J.MARCHEM.2007.01.017
UNESCO (1994). Protocols for the Joint Global Ocean Flux Study (JGOFS) Core Measurement. Paris: UNESCO.
Vilas, J. C., Arístegui, J., Kiriakoulakis, K., Wolff, G. A., Espino, M., Polo, I., et al. (2009). Seamounts and organic matter—Is there an effect? The case of sedlo and seine seamounts: part 1. Distributions of dissolved and particulate organic matter. Deep Sea Res. II Top. Stud. Oceanogr. 56, 2618–2630. doi: 10.1016/J.DSR2.2008.12.023
Keywords: mesopelagic respiration variability, ETS activity, suspended and sinking particulate organic carbon, dissolved organic carbon, Canary Current upwelling region
Citation: Arístegui J, Montero MF, Hernández-Hernández N, Alonso-González IJ, Baltar F, Calleja ML and Duarte CM (2020) Variability in Water-Column Respiration and Its Dependence on Organic Carbon Sources in the Canary Current Upwelling Region. Front. Earth Sci. 8:349. doi: 10.3389/feart.2020.00349
Received: 28 April 2020; Accepted: 27 July 2020;
Published: 26 August 2020.
Edited by:
Rut Pedrosa Pàmies, Marine Biological Laboratory (MBL), United StatesReviewed by:
Dennis Arthur Hansell, University of Miami, United StatesCraig Alexander Carlson, University of California, Santa Barbara, United States
Copyright © 2020 Arístegui, Montero, Hernández-Hernández, Alonso-González, Baltar, Calleja and Duarte. This is an open-access article distributed under the terms of the Creative Commons Attribution License (CC BY). The use, distribution or reproduction in other forums is permitted, provided the original author(s) and the copyright owner(s) are credited and that the original publication in this journal is cited, in accordance with accepted academic practice. No use, distribution or reproduction is permitted which does not comply with these terms.
*Correspondence: Javier Arístegui, amF2aWVyLmFyaXN0ZWd1aUB1bHBnYy5lcw==