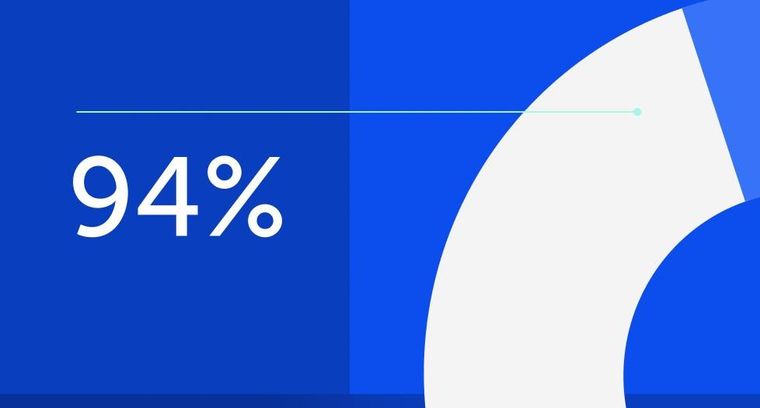
94% of researchers rate our articles as excellent or good
Learn more about the work of our research integrity team to safeguard the quality of each article we publish.
Find out more
ORIGINAL RESEARCH article
Front. Earth Sci., 10 September 2020
Sec. Biogeoscience
Volume 8 - 2020 | https://doi.org/10.3389/feart.2020.00343
A correction has been applied to this article in:
Corrigendum: Leaf Waxes and Hemicelluloses in Topsoils Reflect the δ2H and δ18O Isotopic Composition of Precipitation in Mongolia
Compound-specific hydrogen and oxygen isotope analyzes on leaf wax-derived n-alkanes (δ2Hn–alkane) and the hemicellulose-derived sugar arabinose (δ18Oara) are valuable, innovative tools for paleohydrological reconstructions. Previous calibration studies have revealed that δ2Hn–alkane and δ18Oara reflect the isotopic composition of precipitation, but – depending on the region – may be strongly modulated by evapotranspirative enrichment. Since no calibration studies exist for semi-arid and arid Mongolia so far, we have analyzed δ2Hn–alkane and δ18Oara in topsoils collected along a transect through Mongolia, and we compared these values with the isotopic composition of precipitation (δ2Hp–WM and δ18Op–WM, modeled data) and various climate parameters. δ2Hn–alkane and δ18Oara are more positive in the arid south-eastern part of our transect, which reflects the fact that also the precipitation is more enriched in 2H and 18O along this part of the transect. The apparent fractionation εapp, i.e., the isotopic difference between precipitation and the investigated compounds, shows no strong correlation with climate along the transect (ε2H n–C29/p = −129 ± 14‰, ε2H n–C31/p = −146 ± 14‰, and ε18O ara/p = +44 ± 2‰). Our results suggest that δ2Hn–alkane and δ18Oara in topsoils from Mongolia reflect the isotopic composition of precipitation and are not strongly modulated by climate. Correlation with the isotopic composition of precipitation has root-mean-square errors of 13.4‰ for δ2Hn–C29, 12.6 for δ2Hn–C31, and 2.2‰ for δ18Oara, so our findings corroborate the great potential of compound-specific δ2Hn–alkane and δ18Oara analyzes for paleohydrological research in Mongolia.
Leaf wax-derived n-alkanes and hemicellulose-derived sugars are produced by higher terrestrial plants and stay well-preserved in soils and sediments, because of their resistance against biochemical degradation (Eglinton and Hamilton, 1967). These compounds and their compound-specific stable hydrogen (δ2Hn–alkane) and oxygen (δ18Osugar) isotopic composition get incorporated into soils through above-ground and root litter, abrasion, as well as grazing (dung), and they have a mean residence time of ∼40 years (leaf wax n-alkanes), while pentoses (including the hemicellulose-derived sugar arabinose) average over ∼20 years (Schmidt et al., 2011). Since δ2Hn–alkane and δ18Osugar are not strongly affected by degradation effects (Zech et al., 2011, 2012), they are increasingly used for paleohydrological reconstructions (Aichner et al., 2015; Hepp et al., 2015, 2019; Thomas et al., 2016; Rach et al., 2017; Schäfer et al., 2018; Bliedtner et al., 2020). Usually, they are interpreted to record the isotopic composition of precipitation (Sachse et al., 2006, 2012; Tuthorn et al., 2015; Hou et al., 2018; Hepp et al., 2020), which in turn is long acknowledged as a valuable proxy for paleoclimate reconstructions and controlled by e.g., the temperature and amount effect, continentality, and altitude (Dansgaard, 1964). The isotopic signal of precipitation can be altered by isotopic fractionation at the soil-plant-atmosphere interface, including evaporative enrichment of soil water (εSW), transpirative enrichment of leaf water (εEt) and biosynthetic fractionation (εbio) (Schimmelmann et al., 2006; Sachse et al., 2012; Liu et al., 2016; Cormier et al., 2018; Liu and An, 2019).
εSW and εEt can lead to more positive δ2Hn–alkane and δ18Osugar values relative to precipitation and/or the plants source water. εSW is probably of minor importance under semi-arid and arid conditions because most plants (especially perennial plants) exploit deeper water sources that are not isotopically affected by evaporation (Feakins and Sessions, 2010; Kahmen et al., 2013a; Berke et al., 2015), while εEt has a significant influence on δ2Hn–alkane and δ18Osugar (Hou et al., 2008; Feakins and Sessions, 2010; Tuthorn et al., 2014, 2015; Berke et al., 2015; Cernusak et al., 2016; Liu et al., 2017). During biosynthesis, εbio leads to 2H depletion of ∼−160‰ in δ2Hn–alkane values and 18O enrichment of ∼+27‰ in δ18Osugar values. At the same time, it is reported that εbio can vary among plant life forms and plant physiological metabolisms, for different environmental conditions and latitudes, as well as through relative contributions of the strongly depleted NADPH pool (Sessions et al., 1999; Kahmen et al., 2013b; Newberry et al., 2015; Liu et al., 2016; Sessions, 2016; Lehmann et al., 2017; Cormier et al., 2018; Griepentrog et al., 2019; Liu and An, 2019). The apparent fractionation (ε2H n–alkane/p, ε18O sugar/p), i.e., the difference between the isotopic signature of precipitation/source water (δ2Hp and δ18Op) and δ2Hn–alkane and δ18Osugar, respectively, basically integrates over εSW, εEt and εbio and results in 2H-depleted leaf wax-derived n-alkanes but 18O-enriched hemicellulose-derived sugars relative to δ2Hp and δ18Op (Sachse et al., 2012; Tuthorn et al., 2015; Daniels et al., 2017; Liu and An, 2019; Strobel et al., 2020).
While some studies suggest that ε2H n–alkane/p in plants and topsoils remains relatively constant over latitudinal distances (Liu et al., 2016, Liu and An, 2019; Vogts et al., 2016), many others show correlations of ε2H n–alkane/p with relative humidity, mean annual precipitation (MAP) and the aridity index (AI) (Hou et al., 2008, 2018; Douglas et al., 2012; Tipple and Pagani, 2013; Berke et al., 2015; Herrmann et al., 2017; Li et al., 2019). This can be related to more evapotranspirative enrichment under more arid conditions, but plant physiological adaptations might also play a role, as well as changes in vegetation, as e.g., monocotyledons have more negative δ2Hn–alkane values than dicotyledons (Sachse et al., 2012; Kahmen et al., 2013b; Hepp et al., 2020). For relatively arid regions in the United States and South Africa, Hou et al. (2008), Feakins and Sessions (2010), and Strobel et al. (2020) found no correlations of ε2H n–alkane/p with climate, and they reported constant, but quite different values (−94 ± 21‰, -99 ± 8‰, and −133 ± 12‰, respectively).
So far, only very few calibration studies applied compound-specific δ18Osugar analyzes (Tuthorn et al., 2015; Hepp et al., 2016, 2020; Strobel et al., 2020). For semi-arid and arid regions in South America and South Africa, Tuthorn et al. (2015) and Strobel et al. (2020) suggest enhanced evapotranspirative enrichment and higher ε18O sugar/p values with increasing aridity.
By now, δ2Hn–alkane and δ18Osugar topsoil calibration studies on modern reference material do not exist for semi-arid and arid Mongolia, so it is not clear whether δ2Hn–alkane and δ18Osugar reflect the isotopic composition of precipitation and/or are strongly modulated by evapotranspirative enrichment and climate conditions. Therefore, the aim of this study is to evaluate the influence of different climatic factors on the isotopic composition of leaf wax-derived n-alkanes (δ2Hn–alkane) and the hemicellulose-derived sugar arabinose (δ18Oara) in topsoils from semi-arid and arid Mongolia. More specifically, we addressed the following research questions:
(1) Do δ2Hn–alkane and δ18Oara reflect the isotopic composition of precipitation along the investigated transect?
(2) Do ε2H n–alkane/p and ε18O ara/p indicate a variable and climate-dependent fractionation on δ2Hn–alkane and δ18Oara?
Our study will provide the necessary basis for using δ2Hn–alkane and δ18Oara for paleohydrological and -climatological reconstructions in semi-arid and arid Mongolia and similar regions.
The semi-arid and arid regions of Mongolia are influenced by three major atmospheric circulation systems (Figure 1A). Summer climate is mainly dominated by the Westerlies and the East Asian summer monsoon (EASM) (Wang and Feng, 2013; Rao et al., 2015). Winter climate is dominated by the Siberian high blocking the Westerlies and thus moisture supply during winter (Yamanaka et al., 2007; Liu et al., 2009). This results in short and hot summers and long, cold, and dry winters and overall harsh conditions (Dashkhuu et al., 2015). Especially the vegetation period, during which biosynthesis can take place, is very short and corresponds with the summer months June, July, and August, when ∼75% of the annual precipitation occurs (Figures 1D,E, 2H; Lang et al., 2020).
Figure 1. (A) Map of Mongolia showing δ18Op–OIPC (Bowen, 2019) and the sampling locations. Black circles indicate samples which were analyzed for δ2Hn–alkane only, whereas black/white circles indicate samples which were analyzed for δ2Hn–alkane and δ18Oara. (B) Submap showing a closeup of (A). (C) Map of Mongolia showing mean annual precipitation (Fick and Hijmans, 2017). (D,E) Climate diagrams illustrating the seasonal variability of precipitation and temperature (1970–2000; Fick and Hijmans, 2017), as well as the variability of δ18Op–OIPC (Bowen, 2019) for (D) western Mongolia and (E) southern Mongolia.
Figure 2. δ2H and δ18O signatures of leaf wax-derived n-alkanes and the hemicellulose sugar arabinose as well as their apparent isotope fractionation (εapp), compared to environmental and climatic parameters along the transect. (A) Compound-specific δ2H of the leaf wax-derived n-alkane n-C29 (black) and n-C31 (green) (this study), (B) compound-specific δ18Oara (this study), (C) ε2H n–C29/p (black) and ε2H n–C31/p (green) (this study), (D) ε18O ara/p (this study), (E,F) OIPC isotopic composition of precipitation (black line) and amount-weighted isotopic signature of precipitation (blue line): δ2Hp (E), and δ18Op (F), respectively (Bowen et al., 2005; IAEA/WMO, 2015; Fick and Hijmans, 2017; Bowen, 2019), (G) the potential evapotranspiration (Et0) (Trabucco and Zomer, 2019), (H) mean annual temperature (MAP) (Fick and Hijmans, 2017), (I) averaged summer temperature of June, July, and August (TJJA), (J) black line shows the mean annual precipitation (MAP), blue shaded area the summer precipitation amount (PJJA) (Fick and Hijmans, 2017), (K) the aridity index (AI) (Trabucco and Zomer, 2019), and (L) the altitude (Jarvis et al., 2008).
The climate in Mongolia is characterized by increasing mean annual temperature (MAT) and decreasing mean annual precipitation (MAP) toward the south-east (Figure 1C). This climate gradient is mirrored in regional vegetation biomes, well-adapted to the semi-arid and arid conditions of Mongolia. Northern and central Mongolia are characterized by taiga, mountain- and forest steppe biomes, whereas steppe and desert steppe biomes are dominant in southern Mongolia (Hilbig, 1995; Klinge and Sauer, 2019). The precipitation shows a distinct 2Hp–OIPC and 18Op–OIPC enrichment in southern and eastern arid Mongolia (Figure 1A; Bowen, 2019), and the seasonal pattern is characterized by isotopically 2H- and 18O-depleted precipitation during winter and 2H- and 18O-enriched precipitation during summer (see Figures 1D,E for δ18Op–OIPC).
For this study, topsoils (0–5 cm) were sampled in LDPE plastic bags in June/July 2017 (ID: 1–33) and June 2016 (ID: 34–42). To prevent molding, samples were stored open and dark during the 2-week field campaigns. Our sampling sites were dominated by different Poaceae and Cyperaceae species (grasses) as well as herbaceous and shrubby growth forms of Artemisia spp. and the woody shrub Caragana spp. Larix sibirica occurred at a few sites (for more details, see Struck et al., 2020). The investigated transect follows a west-east and north-south gradient with increasing aridity and 2Hp and 18Op enrichment toward eastern and southern Mongolia (Figures 1A,B, 2 – all sampling sites and respective climate parameters are listed in the Supplementary Material).
Total lipids of 42 topsoils (∼10–35 g) were extracted with dichloromethane:methanol (9:1, v/v) over three cycles using accelerated solvent extraction and ultrasonic extraction. Total lipid extracts were separated over aminopropyl pipette columns (Supelco; 45 μm), n-alkanes were eluted with hexane and additionally cleaned over coupled silver-nitrate (AgNO3) coated silica gel (Supelco, 60–200 mesh) and zeolite (Geokleen Ltd.) pipette columns. n-Alkane identification and quantification were performed on an Agilent 7890B gas chromatograph equipped with an Agilent HP5MS column (30 m × 320 μm × 0.25 μm film thickness) and a flame ionization detector (GC-FID). For identification and quantification, external n-alkane standards (n-alkane mix n-C21 – n-C40, Supelco) were measured with each sequence.
While the n-alkanes had been extracted and quantified in a previous study (Struck et al., 2020) and were available and ready for compound-specific δ2H analyzes, we have selected 28 sampling sites for additional sugar analyzes. Hemicellulose-derived sugars were hydrolytically extracted from 0.1 to 1.4 g topsoil material using 10 ml of 4M trifluoroacetic acid at 105°C for 4 h according to Amelung et al. (1996). Thereafter, samples were vacuum-filtrated over glass fiber filters and the extracted sugars were cleaned over XAD-7 and Dowex 50WX8 columns to remove humic-like substances and cations (Zech and Glaser, 2009). The purified sugar samples were rotary-evaporated and derivatized with methylboronic acid (4 mg in 400 μl pyridine) at 60°C for 1 h. α-Androstane and 3-O-Methyl-Glucose were used as internal standards (Zech and Glaser, 2009).
The compound-specific hydrogen isotopic composition of the most abundant n-alkanes (n-C29, n-C31) were measured on an Isoprime Vision isotope ratio mass spectrometer (IRMS) (Elementar, Langenselbold, Germany) coupled to an Agilent 7890B GC (Agilent, Santa Clara, United States) via a GC5 pyrolysis/combustion interface (Elementar, Langenselbold, Germany). The GC5 was operating in pyrolysis mode with a Cr (ChromeHD) reactor at 1050°C. The GC was equipped with a split/splitless injector and an Agilent HP5GC fused silica column (30 m × 320 μm × 0.25 μm film thickness). Samples were injected in splitless mode and measured as triplicates. For normalization, n-alkane standards (n-C27, n-C29, and n-C33) with known isotopic composition (Schimmelmann standard, Indiana) were measured as duplicates after every third sample triplicate. All measurements were drift-corrected relative to the standards in each sequence. The H-correction factor was checked regularly throughout the sequence and yielded stable values of 3.9 ± 0.02 (n = 4). The standard deviation of the sample triplicates was on average 1.2 and 1.0 for n-C29 and for n-C31, respectively, and not worse than 3.6 and 4.7‰. The standard deviation for all standards was better than 2‰ (n = 36). The hydrogen isotopic composition is given in delta notation (δ2H) vs. Vienna Standard Mean Ocean Water (VSMOW).
The compound-specific oxygen isotope measurements were performed on a Trace GC 2000 coupled to a Delta V Advantage IRMS using an 18O-pyrolysis reactor (GC IsoLink) and a ConFlo IV interface (all devices from Thermo Fisher Scientific, Bremen, Germany). Samples were injected in splitless mode and measured in triplicates. For normalization, derivatized sugar standards with known isotopic composition were measured repeatedly at different concentrations within every sequence. Measured δ18O values were drift- and amount-corrected and corrected for the oxygen from the carbonyl group within the sugar molecules that became introduced during the hydrolysis according to Zech and Glaser (2009). The standard deviation of the sample triplicates was on average 0.4‰, and not worse than 1.2‰. The standard deviation of the arabinose standard was 1.8‰ (n = 24, average over four concentrations). Fucose and xylose concentrations were too low for robust isotope measurements; we therefore refrained from further evaluation of those data. The oxygen isotopic composition is given in delta notation (δ18O) vs. VSMOW.
Apparent fractionation (εapp) of hydrogen- and oxygen isotopes were calculated after Sauer et al. (2001) to test for climatic/environmental controls on δ2Hn–alkane (Eq. 1) and δ18Oara (Eq. 2).
δ2Hp–OIPC and δ18Op–OIPC were extracted from The Online Isotopes in Precipitation Calculator (OIPC, Version 3.1)1, the uncertainty estimates (95% confidence interval) range from 1 to 8‰ for δ2Hp–OIPC, and from 0.2 to 0.8‰ for δ18Op–OIPC, respectively (Bowen et al., 2005; IAEA/WMO, 2015; Bowen, 2019). Since ∼75% of the annual precipitation occurs during the vegetation period in June, July, and August, an amount-weighted mean (δ2Hp–WM and δ18Op–WM) was used to calculate ε2H n–alkane/p and ε18O ara/p (Eqs. 1, 2). MAT and MAP, as well as the temperature and precipitation of June, July and August (TJJA, PJJA) were extracted from the WorldClim 2.0 dataset (1970–2000, 30 s resolution: Fick and Hijmans, 2017)2. The AI and the potential evapotranspiration (Et0) were derived from the Global Aridity Index and Potential Evapotranspiration Climate Database v2 (1970–2000, 30 s resolution: Trabucco and Zomer, 2019)3. The altitude was extracted from the Shuttle Radar Topography Mission (SRTM) data (Jarvis et al., 2008).
Correlations of δ2Hn–alkane and δ18Oara with δ2Hp–WM and δ18Op–WM, respectively were tested using weighted linear regressions. Correlations of ε2H n–alkane/p and ε18O ara/p with climate were tested using unweighted linear regressions. Goodness of fit can be assessed using R2, and the accuracy using root-mean-square errors (RMSE). Differences between the arid part of the transect (ID: 34–42) compared to the rest were analyzed using a t-test. For data sets with unequal variance, the Welsh-corrected t-test was used. Statistical analyzes were done using the statistical software Origin 2019b.
Along our investigated transect, δ2Hn–C29 ranges from −215 to −143‰, with an average of −189 ± 16‰. In comparison to δ2Hn–C29, the δ2Hn–C31 values tend to be more 2H-depleted and range from −228 to −154‰ with an average of −205 ± 15‰ (Figure 2A). The δ18Oara values range from +31‰ to +41‰ with an average of +35 ± 3‰ (Figure 2B). All compounds show the same trend as the isotopic composition of precipitation (Figures 2E,F), and are significantly more positive in the arid part of the transect (ID: 34 – 42) compared to the rest (δ2Hn–C29: p = 0.02, δ2Hn–C31: p = 7.08e–4, δ18Oara: p = 2.95e–5). This reflects the 2H and 18O enrichment of the isotopic composition of precipitation, with values ranging from −101 to −53‰ for δ2Hp–OIPC and from −14 to −7‰ for δ18Op–OIPC, respectively. The amount-weighted mean values are more positive and range from −78 to −46‰ for δ2Hp–WM and −11 to −6‰ for δ18Op–WM, respectively. Like the biomarkers, the precipitation is significantly more positive in the arid part of the transect (δ2Hp–WM: p = 1.39e–15, δ18Op–WM: p = 1.32e–17, Figures 2E,F).
ε2H n–C29/p ranges from −165 to −95‰ with an average of −129 ± 14‰ (Figure 2C). In comparison, ε2H n–C31/p is more negative, ranging from −171 to −100‰ with an average of −146 ± 14‰ (Figure 2C). ε18O ara/p ranges from + 39‰ to + 48‰ with an average of + 44 ± 2‰ (Figure 2D). εapp is not statistically different in the arid part of the transect (ε2H n–C29/p: p = 0.79, ε2H n–C31/p: p = 0.554, ε18O ara/p: p = 824).
Along the investigated transect δ2Hn–C29 is on average ∼15‰ more positive than δ2Hn–C31. As described previously by Struck et al. (2020), n-C29 is the most abundant homolog in the woody shrubs Caragana spp. and Artemisia spp., whereas n-C31 is the most abundant homolog in grasses. Since shrubs and dicotyledonous plants in general are more sensitive to evapotranspirative enrichment than grasses (Sachse et al., 2012; Kahmen et al., 2013b; Hepp et al., 2020), the observed offset might indicate (i) plant-physiological differences affecting the evapotranspirative enrichment of different plants, and (ii) plant-physiological differences affecting εbio.
Grasses grow via the intercalary meristem, where the leaf water is isotopically not as 2H-enriched due to transpiration compared to the exposed part of grasses (Helliker and Ehleringer, 2000, 2002; Lehmann et al., 2017; Liu et al., 2017). The leaf wax n-alkanes, which are produced in the intercalary meristem, do therefore not incorporate the full leaf water enrichment signal (Barbour et al., 2004; Ripullone et al., 2008; Sachse et al., 2012; Kahmen et al., 2013b; Cernusak et al., 2016; Holloway-Phillips et al., 2016). This can be referred to as “dampening effect”.
The δ2Hn–alkane and δ18Oara values correlate significantly with the δ2Hp–WM and δ18Op–WM values (Figure 3, R2 = 0.30, p = 3.22e–4 for δ2Hn–C29; 0.11 and 0.03 for δ2Hn–C31; and 0.36 and 1.60e−3 for δ18Oara). Significant correlations between compound-specific biomarker isotopes and the isotopic composition of precipitation have been observed previously for different regions (a.o.: Sachse et al., 2006; Feakins and Sessions, 2010; Hou et al., 2018; Li et al., 2019; Strobel et al., 2020). In comparison to transects covering larger climate gradients (a.o. Hou et al., 2018), our determination coefficients are small and explain only up to ∼30% of the variability. However, this is due to the fact that our transect covers only a small climate gradient. The RMSE is 13.4‰ for δ2Hn–C29, 12.6‰ for δ2Hn–C31 and 2.2‰ for δ18Oara (Figure 3) and thus indicates that the biomarkers accurately record the isotopic composition of precipitation along our transect. There are several possible explanations for the observed scatter, including: (i) uncertainties related to the modeled OIPC-based isotopic composition of precipitation and the WorldClim 2.0 reanalysis dataset, (ii) the analytical errors of the δ2Hn–alkane and δ18Oara measurements, (iii) micro-climatic effects along the investigated transect, and (iv) metabolic differences affecting εbio (Sachse et al., 2012; Fick and Hijmans, 2017; Cormier et al., 2018; Hou et al., 2018; Bowen, 2019; Strobel et al., 2020; Struck et al., 2020).
Figure 3. δ2H signatures of the leaf wax-derived n-alkanes (A) n-C29 and (B) n-C31, as well as (C) δ18Oara signatures from the topsoil transect through Mongolia plotted against the amount-weighted δ2H and δ18O signature of precipitation (δ2Hp–WM/δ18Op–WM). Red trend lines illustrate linear regressions, gray shaded areas the 95% confidence interval. Bold R2/p-values indicate the level of significance (α = 0.05).
To check for potential climatic influences on δ2Hn–alkane and δ18Oara, we correlated apparent fractionation against TJJA, PJJA, Et0 and AI (Figure 4), as well as MAT, MAP, and altitude (Supplementary Figure 1). None of the correlations with climate is significant, except ε2H n–C29/p and precipitation, which is only weak (R2 = 0.20, p = 4.90e−3 for PJJA, and R2 = 0.12, p = 0.03 for MAP). However, if climatically-controlled one might expect that temperature, potential evapotranspiration and the degree of aridity also affect evapotranspirative enrichment and εapp (Hou et al., 2018). Thus, this correlation should not be overinterpreted. We conclude that εapp is nearly constant with ε2H n–C29/p = −129 ± 14‰, ε2H n–C31/p = −146 ± 14‰, and ε18O ara/p = + 44 ± 2‰.
Figure 4. Apparent fractionation ε2H n–C29/p, ε2H n–C31/p and ε18O ara/p from the topsoil transect through Mongolia plotted against TJJA, PJJA, Et0, AI (Fick and Hijmans, 2017; Trabucco and Zomer, 2019). Red trend lines illustrate linear regressions, gray shaded areas the 95% confidence interval. Bold R2/p-values indicate the level of significance (α = 0.05). Black dashed lines illustrate values for the biosynthetic fractionation, and black arrows indicate the effect of evapotranspirative enrichment (biosynthetic fractionation factors of −160 and +27‰ are assumed for the n-alkanes and arabinose, respectively).
Assuming constant εbio values of −160‰ for leaf wax n-alkanes (Sessions et al., 1999; Hepp et al., 2020), evapotranspirative enrichment would be ∼31‰ for δ2Hn–C29 and ∼15‰ for δ2Hn–C31 (Figure 4). While n-C29 is the most abundant homolog in shrubs, and n-C31 is the most abundant homolog in grasses (Bliedtner et al., 2018; Struck et al., 2020) the observed offset in evapotranspirative enrichment most likely results from plant physiological differences described above, particularly the dampening effect. Assuming a constant εbio factor of +27‰ for arabinose (Lehmann et al., 2017; Hepp et al., 2020) evapotranspirative enrichment would be ∼17‰ for δ18Oara. While we expect arabinose to be mainly synthesized by grasses (Mekonnen et al., 2019), we cannot quantify the contribution from other plants or roots (Schädel et al., 2010). Anyhow, for arabinose synthesized by grasses, we can expect a similar dampening effect as described above for leaf waxes, because leaf water in the leaf growth-and-differentiation zone is usually not as enriched as the exposed part of grasses (Lehmann et al., 2017; Liu et al., 2017; Hepp et al., 2020).
While other calibration studies in relatively arid regions have also not found a strong climatic modulation of ε2H n–alkane/p, our values (−129 ± 14‰ for ε2H n–C29/p and −146 ± 14‰ for ε2H n–C31/p) are only comparable to those from Strobel et al. (2020), i.e., −133 ± 12‰ for n-C31 and n-C33, which are much more negative than those reported by Hou et al. (2008) and Feakins and Sessions (2010), i.e., −99 ± 8‰ and −92 ± 21‰, respectively. Most likely, plant physiological and metabolic adaptations play an important role, as leaf waxes from C4 plants are more enriched in 2H than leaf waxes from C3 plants, and dicotyledons produce more enriched leaf waxes than monocotyledons (Sachse et al., 2012; Kahmen et al., 2013b; Hepp et al., 2020). Li et al. (2019) reported very similar ε2H n–C29/p values as for Mongolia from the semi-arid and arid regions in China (−127 ± 10‰ compared to −129 ± 14‰), yet ε2H n–C31/p is much less negative than in Mongolia (−133 ± 13‰ compared to −146 ± 14‰). We suggest that this reflects the fact that C4 grasses are more dominant in China than along our transect that is dominated by C3 grasses (Pyankov et al., 2000). Our ε2H n–C31/p values are also very much comparable to those reported for C3 grass sites along a transect in Europe (Hepp et al., 2020), and our ε2H n–C29/p values are in very good agreement with their ε2H n–alkane/p values for sites dominated by deciduous trees, which ones more highlights the dampening effect of C3 grasses compared to dicotyledons.
The ε18O ara/p values for Mongolia (44 ± 2‰) are very similar to values reported by Strobel et al. (2020) for relatively arid regions in South Africa. There, the more humid regions have a significantly lower ε18O ara/p (∼37‰), quite similar to the C3 grass sites in Europe (Hepp et al., 2020). The deciduous tree sites in Europe, however, are again characterized by more enriched δ18Osugar values (ε18O sugar/p = ∼43‰). All this indicates that δ18O is more sensitive to evapotranspirative enrichment than δ2H, so that climate can more strongly modulate δ18Osugar, and again that grasses show the signal dampening much more pronounced than dicotyledons.
This study investigated compound-specific δ2Hn–alkane and δ18Oara values in topsoils collected along a transect through semi-arid and arid Mongolia in order to evaluate to which degree they reflect variations in the isotopic signature of precipitation and/or they are affected by climate and evapotranspirative enrichment. We therefore tested for correlations of δ2Hn–alkane and δ18Oara with δ2Hp–WM and δ18Op–WM, respectively, as well as εapp with climate. We can conclude the following:
• Leaf wax-derived n-alkanes and the hemicellulose-derived sugar arabinose are significantly more enriched in 2H and 18O in the more arid southern and eastern parts of the transect. This reflects the changes in the isotopic composition of precipitation along the transect, and the correlations with δ2Hp–WM and δ18Op–WM have RMSE of 13.4‰ for δ2Hn–alkane and 2.2‰ for δ18Oara.
• The apparent fractionation remains mostly constant at −129 ± 14‰, −146 ± 14‰, and at + 44 ± 2‰ for ε2H n–C29/p, ε2H n–C31/p and ε18O ara/p, respectively. There are no significant differences along the transect, nor strong correlations with climate.
Compound-specific δ2Hn–alkane and δ18Oara analyzes on terrestrial biomarkers, preserved e.g., in lake sediments, have great potential for reconstructing past changes in the isotopic composition of precipitation and thus for paleoclimate and -hydrological reconstructions in Mongolia.
The datasets presented in this study can be found in the article/Supplementary Material.
JS, MB, PS, MZ, and RZ designed the study. MB and RZ collected the samples along transect I in 2016. JS and RZ collected the samples along transect II in 2017. JS carried out the major part of the laboratory analyzes in the laboratory of RZ and BG, assisted by LB, PS, and MB. EB, DA, and WZ organized the sample logistics in 2016 and 2017. JS wrote the manuscript with contributions of all coauthors. All authors contributed to the article and approved the submitted version.
This research was supported by the SNF (Grant No. PP00P2 – 150590).
The authors declare that the research was conducted in the absence of any commercial or financial relationships that could be construed as a potential conflict of interest.
We thank the Swiss National Science Foundation (SNF) for funding. PS acknowledges the support by a fellowship from the state of Thuringia (Landesgraduiertenstipendium). DA gratefully acknowledges the support of the framework of Goszadanie no. AAAA-A17-117011810038-7. Furthermore, we greatly acknowledge M. Benesch (Martin Luther University Halle-Wittenberg) for compound-specific δ18O measurements and N. Ueberschaar (MS platform, Friedrich-Schiller-University Jena) for providing laboratory facilities. I. K. Schäfer (Agilent) and J. Wintel (Elementar) are acknowledged for support during δ2H measurements. We want to acknowledge H. Maennicke, C. Heinrich, T. Bromm, S. Polifka, M. Lerch (all Martin Luther University Halle-Wittenberg), M. Wagner, F. Freitag, I. Paetz, and N. Blaubach (all Friedrich-Schiller-University Jena) for laboratory support. We thank our logistic partners in Mongolia, especially G. Gereltsogt from Remote Mongolian Adventures and all field trip participants 2016 and 2017. We thank ML and M-AC for their valuable and helpful comments on this manuscript.
The Supplementary Material for this article can be found online at: https://www.frontiersin.org/articles/10.3389/feart.2020.00343/full#supplementary-material
Aichner, B., Feakins, S. J., Lee, J. E., Herzschuh, U., and Liu, X. (2015). High-resolution leaf wax carbon and hydrogen isotopic record of the late Holocene paleoclimate in arid Central Asia. Clim. Past 11, 619–633. doi: 10.5194/cp-11-619-2015
Amelung, W., Cheshire, M. V., and Guggenberger, G. (1996). Determination of neutral and acidic sugars in soil by capillary gas-liquid chromatography after trifluoroacetic acid hydrolysis. Soil Biol. Biochem. 28, 1631–1639. doi: 10.1016/S0038-0717(96)00248-9
Barbour, M. M., Roden, J. S., Farquhar, G. D., and Ehleringer, J. R. (2004). Expressing leaf water and cellulose oxygen isotope ratios as enrichment above source water reveals evidence of a Péclet effect. Oecologia 138, 426–435. doi: 10.1007/s00442-003-1449-3
Berke, M. A., Tipple, B. J., Hambach, B., and Ehleringer, J. R. (2015). Life form-specific gradients in compound-specific hydrogen isotope ratios of modern leaf waxes along a North American Monsoonal transect. Oecologia 179, 981–997. doi: 10.1007/s00442-015-3432-1
Bliedtner, M., Schäfer, I. K., Zech, R., and von Suchodoletz, H. (2018). Leaf wax n-alkanes in modern plants and topsoils from eastern Georgia (Caucasus) – implications for reconstructing regional paleovegetation. Biogeosciences 15, 3927–3936. doi: 10.5194/bg-15-3927-2018
Bliedtner, M., Zech, R., Zech, J., Schäfer, I., and Suchodoletz, H. (2020). A first Holocene leaf wax isotope-based paleoclimate record from the semi-humid to semi-arid south-eastern Caucasian lowlands. J. Quatern. Sci. 35, 625–633. doi: 10.1002/jqs.3210
Bowen, G. J. (2019). The Online Isotopes in Precipitation Calculator, version 3.1. Available online at: http://www.waterisotopes.org (accessed January 31, 2020).
Bowen, G. J., Wassenaar, L. I., and Hobson, K. A. (2005). Global application of stable hydrogen and oxygen isotopes to wildlife forensics. Oecologia 143, 337–348. doi: 10.1007/s00442-004-1813-y
Cernusak, L. A., Barbour, M. M., Arndt, S. K., Cheesman, A. W., English, N. B., Feild, T. S., et al. (2016). Stable isotopes in leaf water of terrestrial plants. Plant Cell Environ. 39, 1087–1102. doi: 10.1111/pce.12703
Cormier, M.-A., Werner, R. A., Sauer, P. E., Gröcke, D. R., Leuenberger, M. C., Wieloch, T., et al. (2018). 2H-fractionations during the biosynthesis of carbohydrates and lipids imprint a metabolic signal on the δ2H values of plant organic compounds. New Phytol. 218, 479–491. doi: 10.1111/nph.15016
Daniels, W. C., Russell, J. M., Giblin, A. E., Welker, J. M., Klein, E. S., and Huang, Y. (2017). Hydrogen isotope fractionation in leaf waxes in the Alaskan Arctic tundra. Geochim. Cosmochim. Acta 213, 216–236. doi: 10.1016/j.gca.2017.06.028
Dansgaard, W. (1964). Stable isotopes in precipitation. Tellus 16, 436–468. doi: 10.1111/j.2153-3490.1964.tb00181.x
Dashkhuu, D., Kim, J. P., Chun, J. A., and Lee, W.-S. (2015). Long-term trends in daily temperature extremes over Mongolia. Weather Clim. Extremes 8, 26–33. doi: 10.1016/j.wace.2014.11.003
Douglas, P. M. J., Pagani, M., Brenner, M., Hodell, D. A., and Curtis, J. H. (2012). Aridity and vegetation composition are important determinants of leaf-wax δD values in southeastern Mexico and Central America. Geochim. Cosmochim. Acta 97, 24–45. doi: 10.1016/j.gca.2012.09.005
Eglinton, G., and Hamilton, R. J. (1967). Leaf epicuticular waxes. Science 156, 1322–1335. doi: 10.1126/science.156.3780.1322
Feakins, S. J., and Sessions, A. L. (2010). Controls on the D/H ratios of plant leaf waxes in an arid ecosystem. Geochim. Cosmochim. Acta 74, 2128–2141. doi: 10.1016/j.gca.2010.01.016
Fick, S. E., and Hijmans, R. J. (2017). WorldClim 2: new 1-km spatial resolution climate surfaces for global land areas. Int. J. Climatol. 37, 4302–4315. doi: 10.1002/joc.5086
Griepentrog, M., Wispelaere, L., de Bauters, M., Bodé, S., Hemp, A., Verschuren, D., et al. (2019). Influence of plant growth form, habitat and season on leaf-wax n-alkane hydrogen-isotopic signatures in equatorial East Africa. Geochim. Cosmochim. Acta 263, 122–139. doi: 10.1016/j.gca.2019.08.004
Helliker, B. R., and Ehleringer, J. R. (2000). Establishing a grassland signature in veins: 18O in the leaf water of C3 and C4 grasses. PNAS 97, 7894–7898. doi: 10.1073/pnas.97.14.7894
Helliker, B. R., and Ehleringer, J. R. (2002). Differential 18O enrichment of leaf cellulose in C3 versus C4 grasses. Funct. Plant Biol. 29:435. doi: 10.1071/PP01122
Hepp, J., Rabus, M., Anhäuser, T., Bromm, T., Laforsch, C., Sirocko, F., et al. (2016). A sugar biomarker proxy for assessing terrestrial versus aquatic sedimentary input. Organ. Geochem. 98, 98–104. doi: 10.1016/j.orggeochem.2016.05.012
Hepp, J., Schäfer, I. K., Lanny, V., Franke, J., Bliedtner, M., Rozanski, K., et al. (2020). Evaluation of bacterial glycerol dialkyl glycerol tetraether and 2H–18O biomarker proxies along a central European topsoil transect. Biogeosciences 17, 741–756. doi: 10.5194/bg-17-741-2020
Hepp, J., Tuthorn, M., Zech, R., Mügler, I., Schlütz, F., Zech, W., et al. (2015). Reconstructing lake evaporation history and the isotopic composition of precipitation by a coupled δ18O–δ2H biomarker approach. J. Hydrol. 529, 622–631. doi: 10.1016/j.jhydrol.2014.10.012
Hepp, J., Wüthrich, L., Bromm, T., Bliedtner, M., Schäfer, I. K., Glaser, B., et al. (2019). How dry was the younger dryas? Evidence from a coupled δ2H–δ18O biomarker paleohygrometer applied to the gemündener maar sediments, western eifel, Germany. Clim. Past 15, 713–733. doi: 10.5194/cp-15-713-2019
Herrmann, N., Boom, A., Carr, A. S., Chase, B. M., West, A. G., Zabel, M., et al. (2017). Hydrogen isotope fractionation of leaf wax n-alkanes in southern African soils. Organ. Geochem. 109, 1–13. doi: 10.1016/j.orggeochem.2017.03.008
Holloway-Phillips, M., Cernusak, L. A., Barbour, M., Song, X., Cheesman, A., Munksgaard, N., et al. (2016). Leaf vein fraction influences the Péclet effect and 18O enrichment in leaf water. Plant Cell Environ. 39, 2414–2427. doi: 10.1111/pce.12792
Hou, J., D’Andrea, W. J., and Huang, Y. (2008). Can sedimentary leaf waxes record D/H ratios of continental precipitation? Field, model, and experimental assessments. Geochim. Cosmochim. Acta 72, 3503–3517. doi: 10.1016/j.gca.2008.04.030
Hou, J., Tian, Q., and Wang, M. (2018). Variable apparent hydrogen isotopic fractionation between sedimentary n-alkanes and precipitation on the Tibetan Plateau. Organ. Geochem. 122, 78–86. doi: 10.1016/j.orggeochem.2018.05.011
IAEA/WMO (2015). Global Network of Isotopes in Precipitation: The GNIP Database. Available online at: https://nucleus.iaea.org/wiser (accessed January 31, 2020).
Jarvis, A., Reuter, H. I., Nelson, A., and Guevara, E. (2008). Hole-Filled Seamless SRTM Data V4, International Centre for Tropical Agriculture (CIAT). Available online at: http://srtm.csi.cgiar.org (accessed January 31, 2020).
Kahmen, A., Hoffmann, B., Schefuß, E., Arndt, S. K., Cernusak, L. A., West, J. B., et al. (2013a). Leaf water deuterium enrichment shapes leaf wax n-alkane δD values of angiosperm plants II: observational evidence and global implications. Geochim. Cosmochim. Acta 111, 50–63. doi: 10.1016/j.gca.2012.09.004
Kahmen, A., Schefuß, E., and Sachse, D. (2013b). Leaf water deuterium enrichment shapes leaf wax n-alkane δD values of angiosperm plants I: experimental evidence and mechanistic insights. Geochim. Cosmochim. Acta 111, 39–49. doi: 10.1016/j.gca.2012.09.003
Klinge, M., and Sauer, D. (2019). Spatial pattern of Late Glacial and Holocene climatic and environmental development in Western Mongolia – A critical review and synthesis. Quatern. Sci. Rev. 210, 26–50. doi: 10.1016/j.quascirev.2019.02.020
Lang, B., Ahlborn, J., Oyunbileg, M., Geiger, A., von Wehrden, H., Wesche, K., et al. (2020). Grazing effects on intraspecific trait variability vary with changing precipitation patterns in Mongolian rangelands. Ecol. Evol. 10, 678–691. doi: 10.1002/ece3.5895
Lehmann, M. M., Gamarra, B., Kahmen, A., Siegwolf, R. T. W., and Saurer, M. (2017). Oxygen isotope fractionations across individual leaf carbohydrates in grass and tree species. Plant Cell Environ. 40, 1658–1670. doi: 10.1111/pce.12974
Li, Y., Yang, S., Luo, P., and Xiong, S. (2019). Aridity-controlled hydrogen isotope fractionation between soil n-alkanes and precipitation in China. Organ. Geochem. 133, 53–64. doi: 10.1016/j.orggeochem.2019.04.009
Liu, H. T., Schäufele, R., Gong, X. Y., and Schnyder, H. (2017). The δ18O and δ2H of water in the leaf growth-and-differentiation zone of grasses is close to source water in both humid and dry atmospheres. New Phytol. 214, 1423–1431. doi: 10.1111/nph.14549
Liu, J., and An, Z. (2019). Variations in hydrogen isotopic fractionation in higher plants and sediments across different latitudes: implications for paleohydrological reconstruction. Sci. Total Environ. 650, 470–478. doi: 10.1016/j.scitotenv.2018.09.047
Liu, J., Liu, W., An, Z., and Yang, H. (2016). Different hydrogen isotope fractionations during lipid formation in higher plants: implications for paleohydrology reconstruction at a global scale. Sci. Rep. 6:19711. doi: 10.1038/srep19711
Liu, J., Song, X., Sun, X., Yuan, G., Liu, X., and Wang, S. (2009). Isotopic composition of precipitation over Arid Northwestern China and its implications for the water vapor origin. J. Geograph. Sci. 19, 164–174. doi: 10.1007/s11442-009-0164-3
Mekonnen, B., Zech, W., Glaser, B., Lemma, B., Bromm, T., Nemomissa, S., et al. (2019). Chemotaxonomic patterns of vegetation and soils along altitudinal transects of the Bale Mountains, Ethiopia, and implications for paleovegetation reconstructions – Part 1: stable isotopes and sugar biomarkers. E&G Quatern. Sci. J. 68, 177–188. doi: 10.5194/egqsj-68-177-2019
Newberry, S. L., Kahmen, A., Dennis, P., and Grant, A. (2015). n-Alkane biosynthetic hydrogen isotope fractionation is not constant throughout the growing season in the riparian tree Salix viminalis. Geochim. Cosmochim. Acta 165, 75–85. doi: 10.1016/j.gca.2015.05.001
Pyankov, V. I., Gunin, P. D., Tsoog, S., and Black, C. C. (2000). C4 plants in the vegetation of Mongolia: their natural occurrence and geographical distribution in relation to climate. Oecologia 123, 15–31. doi: 10.1007/s004420050985
Rach, O., Engels, S., Kahmen, A., Brauer, A., Martín-Puertas, C., van Geel, B., et al. (2017). Hydrological and ecological changes in western Europe between 3200 and 2000 years BP derived from lipid biomarker δD values in lake Meerfelder Maar sediments. Quatern. Sci. Rev. 172, 44–54. doi: 10.1016/j.quascirev.2017.07.019
Rao, M. P., Davi, N. K., D’Arrigo, R. D., Skees, J., Nachin, B., Leland, C., et al. (2015). Dzuds, droughts, and livestock mortality in Mongolia. Environ. Res. Lett. 10:74012. doi: 10.1088/1748-9326/10/7/074012
Ripullone, F., Matsuo, N., Stuart-Williams, H., Wong, S. C., Borghetti, M., Tani, M., et al. (2008). Environmental effects on oxygen isotope enrichment of leaf water in cotton leaves. Plant Physiol. 146, 729–736. doi: 10.1104/pp.107.105643
Sachse, D., Billault, I., Bowen, G. J., Chikaraishi, Y., Dawson, T. E., Feakins, S. J., et al. (2012). Molecular paleohydrology: interpreting the hydrogen-isotopic composition of lipid biomarkers from photosynthesizing organisms. Annu. Rev. Earth Planet. Sci. 40, 221–249. doi: 10.1146/annurev-earth-042711-105535
Sachse, D., Radke, J., and Gleixner, G. (2006). δD values of individual n-alkanes from terrestrial plants along a climatic gradient – Implications for the sedimentary biomarker record. Organ. Geochem. 37, 469–483. doi: 10.1016/j.orggeochem.2005.12.003
Sauer, P. E., Eglinton, T. I., Hayes, J. M., Schimmelmann, A., and Sessions, A. L. (2001). Compound-specific D/H ratios of lipid biomarkers from sediments as a proxy for environmental and climatic conditions. Geochim. Cosmochim. Acta 65, 213–222. doi: 10.1016/S0016-7037(00)00520-2
Schädel, C., Blöchl, A., Richter, A., and Hoch, G. (2010). Quantification and monosaccharide composition of hemicelluloses from different plant functional types. Plant Physiol. Biochem. 48, 1–8. doi: 10.1016/j.plaphy.2009.09.008
Schäfer, I. K., Bliedtner, M., Wolf, D., Kolb, T., Zech, J., Faust, D., et al. (2018). A δ13C and δ2H leaf wax record from the Late quaternary loess-paleosoil sequence El Paraíso, central spain. Palaeogeogr. Palaeoclimatol. Palaeoecol. 507, 52–59. doi: 10.1016/j.palaeo.2018.06.039
Schimmelmann, A., Sessions, A. L., and Mastalerz, M. (2006). Hydrogen isotopic (D/H) composition of organic matter during diagenesis and thermal maturation. Annu. Rev. Earth Planet. Sci. 34, 501–533. doi: 10.1146/annurev.earth.34.031405.125011
Schmidt, M. W. I., Torn, M. S., Abiven, S., Dittmar, T., Guggenberger, G., Janssens, I. A., et al. (2011). Persistence of soil organic matter as an ecosystem property. Nature 478, 49–56. doi: 10.1038/nature10386
Sessions, A. L. (2016). Factors controlling the deuterium contents of sedimentary hydrocarbons. Organ. Geochem. 96, 43–64. doi: 10.1016/j.orggeochem.2016.02.012
Sessions, A. L., Burgoyne, T. W., Schimmelmann, A., and Hayes, J. M. (1999). Fractionation of hydrogen isotopes in lipid biosynthesis. Organ. Geochem. 30, 1193–1200. doi: 10.1016/S0146-6380(99)00094-7
Strobel, P., Haberzettl, T., Bliedtner, M., Struck, J., Glaser, B., Zech, M., et al. (2020). The potential of δ2Hn–alkanes and δ18Osugar for paleoclimate reconstruction – A regional calibration study for South Africa. Sci. Total Environ. 716:137045. doi: 10.1016/j.scitotenv.2020.137045
Struck, J., Bliedtner, M., Strobel, P., Schumacher, J., Bazarradnaa, E., and Zech, R. (2020). Leaf wax n-alkane patterns and compound-specific δ13C of plants and topsoils from semi-arid and arid Mongolia. Biogeosciences 17, 567–580. doi: 10.5194/bg-17-567-2020
Thomas, E. K., Huang, Y., Clemens, S. C., Colman, S. M., Morrill, C., Wegener, P., et al. (2016). Changes in dominant moisture sources and the consequences for hydroclimate on the northeastern Tibetan Plateau during the past 32 kyr. Quatern. Sci. Rev. 131, 157–167. doi: 10.1016/j.quascirev.2015.11.003
Tipple, B. J., and Pagani, M. (2013). Environmental control on eastern broadleaf forest species’ leaf wax distributions and D/H ratios. Geochim. Cosmochim. Acta 111, 64–77. doi: 10.1016/j.gca.2012.10.042
Trabucco, A., and Zomer, R. (2019). Global Aridity Index and Potential Evapotranspiration (ET0) Climate Database version 2.
Tuthorn, M., Zech, M., Ruppenthal, M., Oelmann, Y., Kahmen, A., Valle, H. F. D., et al. (2014). Oxygen isotope ratios (18O/16O) of hemicellulose-derived sugar biomarkers in plants, soils and sediments as paleoclimate proxy II: insight from a climate transect study. Geochim. Cosmochim. Acta 126, 624–634. doi: 10.1016/j.gca.2013.11.002
Tuthorn, M., Zech, R., Ruppenthal, M., Oelmann, Y., Kahmen, A., del Valle, H. F., et al. (2015). Coupling δ2H and δ18O biomarker results yields information on relative humidity and isotopic composition of precipitation – a climate transect validation study. Biogeosciences 12, 3913–3924. doi: 10.5194/bg-12-3913-2015
Vogts, A., Badewien, T., Rullkötter, J., and Schefuß, E. (2016). Near-constant apparent hydrogen isotope fractionation between leaf wax n-alkanes and precipitation in tropical regions: evidence from a marine sediment transect off SW Africa. Organ. Geochem. 96, 18–27. doi: 10.1016/j.orggeochem.2016.03.003
Wang, W., and Feng, Z. (2013). Holocene moisture evolution across the Mongolian Plateau and its surrounding areas: a synthesis of climatic records. Earth Sci. Rev. 122, 38–57. doi: 10.1016/j.earscirev.2013.03.005
Yamanaka, T., Tsujimura, M., Oyunbaatar, D., and Davaa, G. (2007). Isotopic variation of precipitation over eastern Mongolia and its implication for the atmospheric water cycle. J. Hydrol. 333, 21–34. doi: 10.1016/j.jhydrol.2006.07.022
Zech, M., and Glaser, B. (2009). Compound-specific δ18O analyses of neutral sugars in soils using gas chromatography-pyrolysis-isotope ratio mass spectrometry: problems, possible solutions and a first application. Rapid Commun. Mass Spectr. 23, 3522–3532. doi: 10.1002/rcm.4278
Zech, M., Pedentchouk, N., Buggle, B., Leiber, K., Kalbitz, K., Markoviæ, S. B., et al. (2011). Effect of leaf litter degradation and seasonality on D/H isotope ratios of n-alkane biomarkers. Geochim. Cosmochim. Acta 75, 4917–4928. doi: 10.1016/j.gca.2011.06.006
Keywords: biomarkers, n-alkanes, sugars, compound-specific isotopes, apparent fractionation
Citation: Struck J, Bliedtner M, Strobel P, Bittner L, Bazarradnaa E, Andreeva D, Zech W, Glaser B, Zech M and Zech R (2020) Leaf Waxes and Hemicelluloses in Topsoils Reflect the δ2H and δ18O Isotopic Composition of Precipitation in Mongolia. Front. Earth Sci. 8:343. doi: 10.3389/feart.2020.00343
Received: 27 February 2020; Accepted: 22 July 2020;
Published: 10 September 2020.
Edited by:
Moritz Felix Lehmann, University of Basel, SwitzerlandReviewed by:
Marco Lehmann, Paul Scherrer Institut (PSI), SwitzerlandCopyright © 2020 Struck, Bliedtner, Strobel, Bittner, Bazarradnaa, Andreeva, Zech, Glaser, Zech and Zech. This is an open-access article distributed under the terms of the Creative Commons Attribution License (CC BY). The use, distribution or reproduction in other forums is permitted, provided the original author(s) and the copyright owner(s) are credited and that the original publication in this journal is cited, in accordance with accepted academic practice. No use, distribution or reproduction is permitted which does not comply with these terms.
*Correspondence: Julian Struck, anVsaWFuLnN0cnVja0B1bmktamVuYS5kZQ==
Disclaimer: All claims expressed in this article are solely those of the authors and do not necessarily represent those of their affiliated organizations, or those of the publisher, the editors and the reviewers. Any product that may be evaluated in this article or claim that may be made by its manufacturer is not guaranteed or endorsed by the publisher.
Research integrity at Frontiers
Learn more about the work of our research integrity team to safeguard the quality of each article we publish.