- 1School of Earth Sciences, Faculty of Science, University of Bristol, Bristol, United Kingdom
- 2Earth and Life Institute, Université catholique de Louvain, Louvain-la-Neuve, Belgium
- 3Alfred Wegener Institute, Helmholtz Centre for Polar and Marine Research, Bremerhaven, Germany
- 4Facultad de Cs. Exactas y Naturales, Universidad de Buenos Aires, Buenos Aires, Argentina
- 5Byrd Polar and Climate Research Center, College of Arts and Sciences, The Ohio State University, Columbus, OH, United States
- 6School of Earth Sciences, The Ohio State University, Columbus, OH, United States
- 7School of Geographical Sciences, Faculty of Science, University of Bristol, Bristol, United Kingdom
- 8School of Earth and Ocean Sciences, College of Physical Sciences and Engineering, Cardiff University, Cardiff, United Kingdom
- 9University of Cologne, Cologne, Germany
- 10Institute of Arctic and Alpine Research, University of Colorado Boulder, Boulder, CO, United States
Glaciers and ice sheets export significant amounts of silicon (Si) to downstream ecosystems, impacting local and potentially global biogeochemical cycles. Recent studies have shown Si in Arctic glacial meltwaters to have an isotopically distinct signature when compared to non-glacial rivers. This is likely linked to subglacial weathering processes and mechanochemical reactions. However, there are currently no silicon isotope (δ30Si) data available from meltwater streams in Antarctica, limiting the current inferences on global glacial silicon isotopic composition and its drivers. To address this gap, we present dissolved silicon (DSi), δ30SiDSi, and major ion data from meltwater streams draining a polythermal glacier in the region of the West Antarctic Peninsula (WAP; King George Island) and a cold-based glacier in East Antarctica [Commonwealth Stream, McMurdo Dry Valleys (MDV)]. These data, alongside other global datasets, improve our understanding of how contrasting glacier thermal regime can impact upon Si cycling and therefore the δ30SiDSi composition. We find a similar δ30SiDSi composition between the two sites, with the streams on King George Island varying between -0.23 and +1.23‰ and the Commonwealth stream varying from -0.40 to +1.14‰. However, meltwater streams in King George Island have higher DSi concentrations, and the two glacial systems exhibit opposite DSi – δ30SiDSi trends. These contrasts likely result from differences in weathering processes, specifically the role of subglacial processes (King George Island) and, supraglacial processes followed by in-stream weathering in hyporheic zones (Commonwealth Stream). These findings are important when considering likely changes in nutrient fluxes from Antarctic glaciers under climatic warming scenarios and consequent shifts in glacial thermal regimes.
Introduction
There is growing evidence that links nutrient fluxes to the ocean with the export of glacial meltwater and icebergs, especially from the Greenland Ice Sheet (Hood et al., 2009; Gerringa et al., 2012; Hawkings et al., 2014, 2015, 2017; Lawson et al., 2014; Hendry et al., 2019). However, predictions of how nutrient fluxes may change under climatic warming scenarios are poorly constrained, due to the complexity of these systems, and the lack of data available from large glaciers (Hopwood et al., 2018). The vast majority of data currently available are only from the Arctic or sub-Arctic. Recent studies of the West Antarctic Peninsula (WAP) have shown that glacial meltwater may provide iron to coastal waters (Annett et al., 2015; Lyons et al., 2015; Hodson et al., 2017; Henkel et al., 2018; Höfer et al., 2019). Furthermore, upwelling marine waters can be modified due to benthic fluxes of iron and silica in shallow marine shelf sediments, which are in turn affected by the inputs of particles from glacial sources both in the Arctic and Antarctic (Henley et al., 2018; Sherrell et al., 2018; Hendry et al., 2019), highlighting a potentially significant role of glacial nutrients derived from particle dissolution.
The WAP has experienced some of the most rapid warming of the Southern Hemisphere and ice shelves have undergone retreat over the past 50 years (Turner et al., 2005). Under climatic warming scenarios, ice free areas on Antarctica could expand by 25% by 2100 (Lee et al., 2017), highlighting the future potential for further glacial retreat. This clearly has implications for the delivery of glacially derived nutrients to downstream ecosystems and may impact wider biogeochemical cycles. But in order to improve our predictions, we need to gain a better understanding of the underlying biogeochemical processes, including subglacial and periglacial weathering processes.
Stable silicon isotope data have been used previously to improve understanding of weathering processes in glacial and permafrost covered environments (Georg et al., 2007; Opfergelt et al., 2013; Hatton et al., 2019a). Previous studies have found that glacial rivers export isotopically distinct silicon compared to non-glacial rivers (Hatton et al., 2019b). It is hypothesized that the subglacial weathering processes, fueled by high physical erosion, drive the export of isotopically light glacial silicon due to large amounts of fine grained material enriched in 28Si, high mineral surface areas and elevated rock to water ratios. This has implications for understanding of the global silicon cycle over glacial to interglacial timescales when considering oceanic diatom utilization of silicon (Hawkings et al., 2018). However, the current silicon isotope dataset for glacial rivers is limited to Arctic and sub-Arctic regions, and it remains unclear whether this understanding is transferrable to Antarctica. Using silicon concentration data from subglacial Lake Whillans, the potential flux of DSi from the Antarctic ice sheet via melt and icebergs is equivalent to the Greenland Ice Sheet (Hawkings et al., 2017), highlighting the potential importance of better constraining Antarctic silicon sources, especially as glacial retreat occurs.
To address the role of Antarctic glaciers in silicon cycling, we investigate the dissolved silicon isotope composition (δ30SiDSi) of riverine waters fed by two contrasting glacial environments; Fourcade Glacier, a polythermal glacier on King George Island, and Commonwealth Glacier, a cold-based glacier in McMurdo Dry Valleys (MDV). Analysis of streams fed by glaciers with contrasting thermal regimes and stream properties allows us to consider the impact differing conditions may have upon Si export and how we may use the δ30SiDSi composition of proglacial streams to infer different weathering processes within different glacial environments. This will allow a greater understanding of global glacial silicon cycling.
Currently, studies of δ30SiDSi composition from glacial environments do not include any cold-based systems. We can use the δ30SiDSi composition of Commonwealth Stream, MDV, East Antarctica to assess the current state of these environments and how these systems behave differently to warm-based glaciers, so we can begin to determine a global glacial Si cycle, its drivers, and potential changes in the future. The MDV are home to a collection of largely cold-based glaciers, providing the ideal glacial thermal regime endmember in conceptual models of silicon cycling in cold regions. Commonwealth Stream is fed mainly by the supraglacial melt from Commonwealth Glacier. A cold-based glacier is defined by the basal ice being below the temperature of the basal melting point (Waller, 2001). Such low temperatures result in a lack in active subglacial biogeochemical weathering, which is the process that has been suggested to drive the distinct silicon isotope signature of warm-based glaciers (Hatton et al., 2019b). Despite this, there is evidence that cold-based glaciers can erode, transport, and deposit sediments (Cuffey et al., 2000; Hambrey and Fitzsimons, 2010). Although streams in the MDV are not impacted by subglacial chemical weathering, they are influenced by weathering processes within cryoconite holes via supraglacial melt (Fortner et al., 2005; Bagshaw et al., 2013; Fountain, 2014). In addition, most streams have extensive hyporheic zones, where chemical weathering and dissolution occurs (Gooseff et al., 2002; Welch et al., 2010; Olund et al., 2018). Previous studies have found these streams to be a potential source of Fe and P to coastal phytoplankton communities (Lyons et al., 2015; Olund et al., 2018). Despite the cold and dry conditions in the Dry Valleys, analysis of stream hyporheic zones has shown primary silicate weathering rates to be comparable to temperate environments (Gooseff et al., 2002). This concurs with other studies challenging the assumption that high latitude systems have lower weathering rates (Huh et al., 1998; Wadham et al., 2010; Urra et al., 2019).
We contrast the study of silicon isotope geochemistry in the MDV with analysis of silicon isotopes in streams influenced by the polythermal Fourcade Glacier on Potter Peninsula on King George Island (Falk et al., 2018). King George Island (also known as Isla 25 de Mayo) is the largest of the South Shetland Archipelago and 92% of the island is ice-covered (Leal et al., 2008). The more frequent occurrence of positive Southern Annual Mode phases is responsible for warmer air temperatures at the Southern Shetland Islands, increasing surface melt and glacier retreat (Jerosch et al., 2018). Therefore, the study of δ30SiDSi composition from glacial streams on King George Island captures the status quo of a rapidly changing environment, by sampling streams in contrasting hydrogeological basins (fed by glacial melt or not), building a basis for the assessment of changes related to deglaciation. It was estimated that 44% of the coastline on King George Island was ice-free in 2008 (Rückamp et al., 2011), a figure likely to have increased over the past decade. This glacial retreat has impacted the downstream fjord ecosystem, with contrasting effects on marine biota by decreased light penetration due to increased suspended matter discharge and increased nutrient availability (Schloss et al., 1999, 2014; Ardelan et al., 2010; Monien et al., 2017; Jerosch et al., 2018). The impact the retreat may have on the silicon cycle has not been studied, but such knowledge could enable us to decipher how other glaciers may behave in the future under climatic warming scenarios where glacial retreat is increasing.
Materials and Methods
Sampling Locations
Commonwealth Stream is located in the MDV, and the bedrock is made up from the Beacon Supergroup sandstone, Ferrar Dolerite, crystalline basement igneous and metamorphic rocks, and the McMurdo Volcanics (Hall and Denton, 2000). Supraglacial meltwater from Commonwealth Glacier feeds into Commonwealth stream, which flows for 4–10 weeks of the year. The stream is 4.2 km long, has an average gradient of 0.044 m/m, and discharges directly into the McMurdo Sound (Wlostowski et al., 2016). The stream channel is bounded by permafrost and there is also a hyporheic zone present, which expands to up to 60 cm depth throughout the summer (McKnight et al., 1999; Olund et al., 2018). The daily and seasonal discharge varies greatly due to solar incidence, temperature, and precipitation (McKnight et al., 1999; Olund et al., 2018), as highlighted in Supplementary Figure S1. Commonwealth Stream has been gauged for 24 years by the MCM-LTER program, with flow data collected every 15 min using Campbell CR10 data loggers (McKnight et al., 1999; Wlostowski et al., 2016). Water samples from Commonwealth Stream were collected in the austral summers between 2014 and 2017 (Supplementary Table S1). Most of the samples were collected at the discharge gauging station, approximately 0.7 km from the glacier terminus (Figure 1, sampling location 1, hereafter referred to as Gauging Station). A partial diurnal set of samples was also collected on 1 January 2016 close to the river mouth, approximately 3.4 km downstream from the Gauging Station and 100 m from where the stream discharges into the McMurdo Sound (as described in Olund et al., 2018, Figure 1, sampling location 2, subsequently referred to as the Mouth Station).
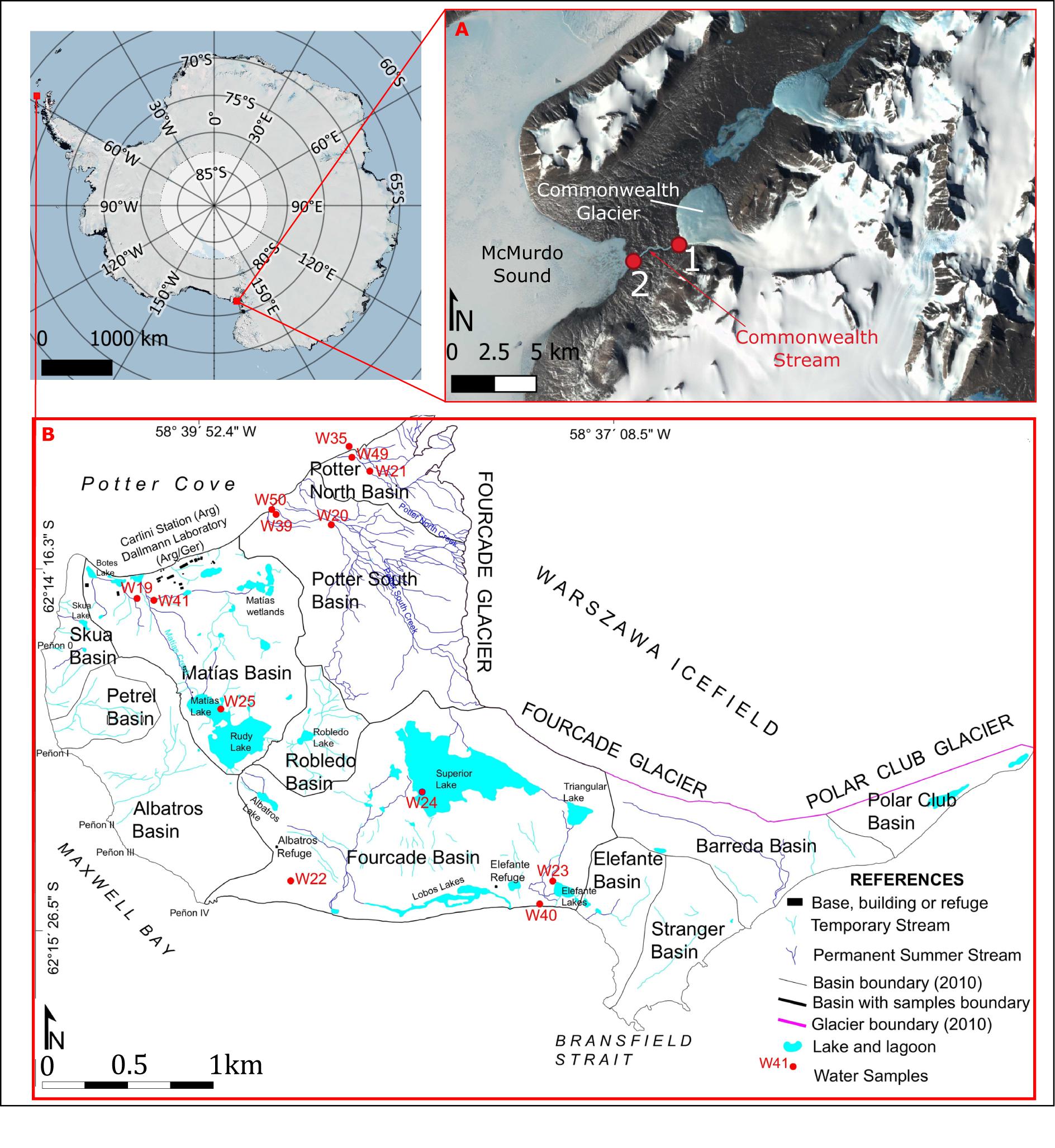
Figure 1. Sampling location map. Sample locations shown in red, with labeling corresponding to sample points described in Section “Materials and Methods.” (A) Two sampling locations (red circles) on Commonwealth Stream (drawn in blue). 1 shows the location of the majority of samples and the discharge gauge station (Commonwealth Gauge) and 2 shows the location of the partial diurnal samples collected at the stream mouth (Commonwealth Mouth). Figure created using Quantarctica and QGIS, produced by the Norwegian Polar Institute (Matsuoka et al., 2018). (B) Sampling location of streams on Potter Peninsula, King George Island (red circles), with the estimated drainage network and the hydrogeological basins. Due to the transient nature of the streams and it is very likely that not all the streams present in the basins are marked. Base map by Lusky et al. (2001), which was geohydrologically corrected over successive field campaigns.
King George Island is the largest island of the mostly ice capped South Shetland Islands, located 130 km from the north-western tip of the Antarctic peninsula (Jerosch et al., 2018). Streams were sampled on Potter Peninsula, which is on the southern-most tip of King George Island. Water samples were collected from a range of streams on Potter Peninsula in February 2012 and January–March 2013 (Figure 1 and Supplementary Table S1). Each sample was collected from a different stream, with estimated stream locations on marked on Figure 1 (see photos in Henkel et al., 2018). The transient nature of the streams on Potter Peninsula means we cannot accurately mark all the stream paths, especially as samples were collected over two melt seasons. In the summer, streams on Potter Peninsula are fed by a combination of precipitation, glacial melt, groundwaters, and permafrost thaw. It was not within the scope of this study to quantify the different water sources to each stream, but the Peninsula can be separated into hydrogeological basins (Figure 1, further details in provided in the Supplementary Material and Supplementary Figure S2), which we used to consider whether or not the streams are fed by glacial meltwater (Silva-Busso, 2009). Glacial meltwater originates from Fourcade Glacier, a polythermal glacier, which drains from the Warzawa Ice Field, which then feeds into Potter Cove and Bransfield Strait. Fourcade Glacier was classified as marine terminating (into Potter Cover), until 2016, but rapid retreat (mean rate of 20 m a–1 between 1956 and 2008, Rückamp et al., 2011), has led to it being land-based (Jerosch et al., 2018). We sampled streams in the three basins; Potter South, Potter North, and Fourcade Basin (Figure 1), which are fed, at least in part by Fourcade Glacier. The drainage network in Potter North and Potter South are composed of ephemeral, short, shallow, and unstable channels that develop a sub-dendritic design on top of modern glacial deposits of homogenous lithology. Melt processes can explain about 98–99% of the water supply to streams in the basins on the glaciated parts of the catchment, based upon a proposed hydrological discharge model (Falk et al., 2018). There are no existing systematic studies of Fourcade Basin to date; however, some geohydrological observations can be made to characterize the basin. Fourcade Glacier is the main contribution of water to the basin, but large lakes have a storage effect before the water discharges into the Brandsfield Strait.
We also sampled streams in Matías Basin, where water is sourced from rainfall and permafrost, but no glacial meltwaters feed the streams (Ermolin and Silva-Busso, 2008). The basin is interpreted as a hummocky moraine related to Warszawa glacial retreat. It is an area of the discontinuous permafrost, with closed talik formation in the surface drainage area and open talik below Lake Matías. The groundwater is considered as an active and integrated supra-permafrost aquifer system throughout the southern summer (September to May inclusive), which flows toward Potter Cove (Silva-Busso, 2009).
The dynamic nature of the streams on the Potter Peninsula meant it was not possible to quantify discharge of the sampled streams. However, annual discharge into Potter Cove from Fourcade Glacier is estimated as 25 ± 6 hm3 year–1 (Falk et al., 2018), leading to an estimate of annual specific discharge of 1.1 ± 0.26 m year–1. This is lower than the specific discharge of Greenlandic Glaciers previously studied for δ30SiDSi composition (Leverett Glacier and Kiattuut Sermiat Glacier, Hatton et al., 2019a), but Leverett Glacier has the most similar specific discharge (1.8–3.7 m year–1, Hawkings et al., 2016), thus was chosen as the site to compare results from this study. The streams on Potter Peninsula overlay modern glacial deposits which have high lithological homogeneity (Falk et al., 2018). The bedrock of Potter Peninsula mainly consists of the King George Island Supergroup, which is made up of lavas and volcanoclastic rocks of basaltic and andesitic characteristics (Birkenmajer, 1980; Kraus, 2005; Ye et al., 2018).
It was not within the scope of this study to examine streams from more than one area of King George Island; however, major ion analysis of streams on Potter Peninsula shows similar geochemical compositions to rivers previously studied on King George Island (Ye et al., 2018). Meltwater streams on Fildes Peninsula have Si concentrations of 34 ± 20 μM (Ye et al., 2018), which compares well with the Si concentrations reported in this study [29.2 (11.1–58.0) μM], suggesting we can apply the δ30SiDSi composition of Potter Peninsula to other glacially fed streams on King George Island.
Sampling Protocol and Analysis
Water samples from Commonwealth Stream were filtered through 0.4 μm Whatman Nuclepore polycarbonate membrane filters within 24 h of collection (Olund et al., 2018). Samples were kept refrigerated and in the dark until analysis. Samples from King George Island were sequentially filtered through 5 and 0.45 μm Millipore Durapore PFDF filters which were pre-cleaned with 3% Elma Clean 70 (an alkaline detergent), deionized water, aqua regia (distilled HCl and HNO3), and ultra-pure water. The filtration was performed on the day of sampling. The filtrate was acidified with double distilled HCl to ∼pH 2 and sample bottles were kept in the dark until processing and analysis. All sample bottles were cleaned with 3% Elma 70 (7 days), deionized water (three rinses), 3 M HNO3 (p.a.) (21 days), deionized water (1 day), 0.4 M HCl distilled. (7 days), 0.01 M HCl double distilled (7 days), and MilliQ (1 day) before use.
Dissolved silicon (DSi) was measured spectrophotometrically using flow injection analysis (FIA) on a LaChat 8500 series (QuikChem Method 31-114-27-1-D) at the University of Bristol and using a Skalar San++ Automated Wet Chemistry Analyzer at The Ohio State University, as detailed previously by Hawkings et al. (2017) and Olund et al. (2018).
Major ions for Commonwealth Stream samples were determined using a Dionex 120 at the Crary Laboratory at McMurdo Station, as detailed by Welch et al. (2010). Major ions for King George Island were determined by ion chromatography, using a Thermo ScientificTM DionexTM capillary ICS-5000 fitted with anion and cation columns at the University of Bristol (Hawkings et al., 2015). We used the divalent/monovalent ion ratio (D:M) as an indication of carbonate and silicate weathering, with elevated proportions of monovalent ions linked to enhanced silicate mineral weathering (Wadham et al., 2010).
As both sampling locations at Commonwealth Stream are within close proximity to the ocean, atmospheric inputs were corrected for using a normalization to chloride (Stallard and Edmond, 1981; Grosbois et al., 2000). The riverine portion of the major ions for samples from Commonwealth Stream was calculated by Eq. 1:
where X[Measured] and Cl[Measured] are the original molar concentrations of the element of interest and Cl, respectively, and (X/Cl)[Mar] is the molar ratio of the element of interest to Cl in seawater, according to Millero et al. (2008). Samples collected from Potter Peninsula were acidified on collection with HCl, so a correction based on Cl was not possible. Therefore, we applied the average atmospheric input correction that was previously used for samples from Fildes Peninsula, King George Island (Ye et al., 2018). When applying the atmospheric input correction, the D:M ratio for Commonwealth Stream samples were impacted more strongly than those from Potter Peninsula and some samples resulted in negative Na+ values (Supplementary Table S2). This overcorrection likely arose from the assumption that all Cl– in the samples derived from atmospheric input. Crompton et al. (2015) described elevated Cl– in subglacial waters, which could be associated with Na dissolution and/or precipitation into secondary weathering products during basal freeze-on, and higher Cl– concentrations of the supraglacial waters reaching the bed, due to preferential retention of Cl– in the snow and ice pack. However, we chose to maintain this correction to ensure our dataset is comparable with other studies in close proximity.
Silicon isotope analysis was completed at the Bristol Isotope Laboratories (University of Bristol) using Thermo Scientific Neptune Plus High Resolution MC-ICP-MS. Detailed methods have been published previously (Hawkings et al., 2018; Hatton et al., 2019a) but, briefly, a standard-sample bracketing procedure was employed, with Mg-doping to correct for instrumental mass bias (Cardinal et al., 2003). Samples were also doped with 100 μL 0.1 M sulfuric acid (Romil-UpA) to ensure matrix match between sample and standard and reduce the mass bias effects of anion loading (Hughes et al., 2011). Most samples were measured in triplicate, with typical reproducibility of ±0.07‰ (0.01–0.15‰, 2SD). Long-term reproducibility of δ30Si of reference standards LMG and Diatomite were -3.44 ± 0.07 (2SD, n = 138) and +1.22 ± 0.07 (2SD, n = 99) respectively. A comparison of δ30Si and δ29Si showed a mass-dependent fractionation line with gradient 0.5130 (Supplementary Figure S3, Reynolds et al., 2007).
Some samples from Potter Peninsula were collected in duplicate with one sample being additionally filtered using Vivaflow 200 cross flow cassettes which were pre-cleaned with EDTA and ultrapure water. Ultra-filtration was performed to determine potential differences between the methodologically defined dissolved fraction that may still contain colloids and nanoparticles and the truly dissolved fraction, as highlighted in Patagonian glacial rivers (Pryer et al., Submitted). δ30SiDSi composition analysis was completed on both sets of water samples to assess potential effects of colloidal phases on the δ30SiDSi composition in the 0.45 μm filtered samples. Supplementary Table S3 shows that δ30SiDSi compositions of 0.45 μm and ultra-filtered samples are similar. It was therefore decided to use the 0.45 μm samples throughout this study for consistency.
Stream bed sediments from Commonwealth Stream were analyzed using scanning electron microscopy (SEM), to evaluate mineral alteration. Samples were collected close to the Gauging Station, stored at room temperature, and allowed to air dry. Prior to analysis, samples were placed on carbon tape on an aluminum stub and then coated with gold/palladium with a Denton Desk V precious metal coater. SEM was completed with a FEI Quanta FEG 250 Field Emission SEM equipped with a Bruker EDX detector. Most images were collected at 15 kV using backscattered electron detector (BSE), with grayscale images providing some compositional information.
Results
Both Commonwealth Stream and the streams of the Potter Peninsula have a similar average and range of δ30SiDSi composition, +0.42‰ (-0.40 to +1.14‰) and +0.54‰ (-0.23 to +1.23‰) respectively (Table 1 and Figure 2). These values are isotopically light in comparison with recent global data compilations of non-glacial rivers [+1.37‰ (-0.14 to +4.66‰), Frings et al., 2016; Hatton et al., 2019b], and they correspond well to the average δ30SiDSi composition of glacial rivers [+0.16‰ (-0.58 to +1.01‰), Hatton et al., 2019b]. However, when considering each location in more detail, we see differences in the δ30SiDSi composition dependent on the predominance of specific weathering processes.
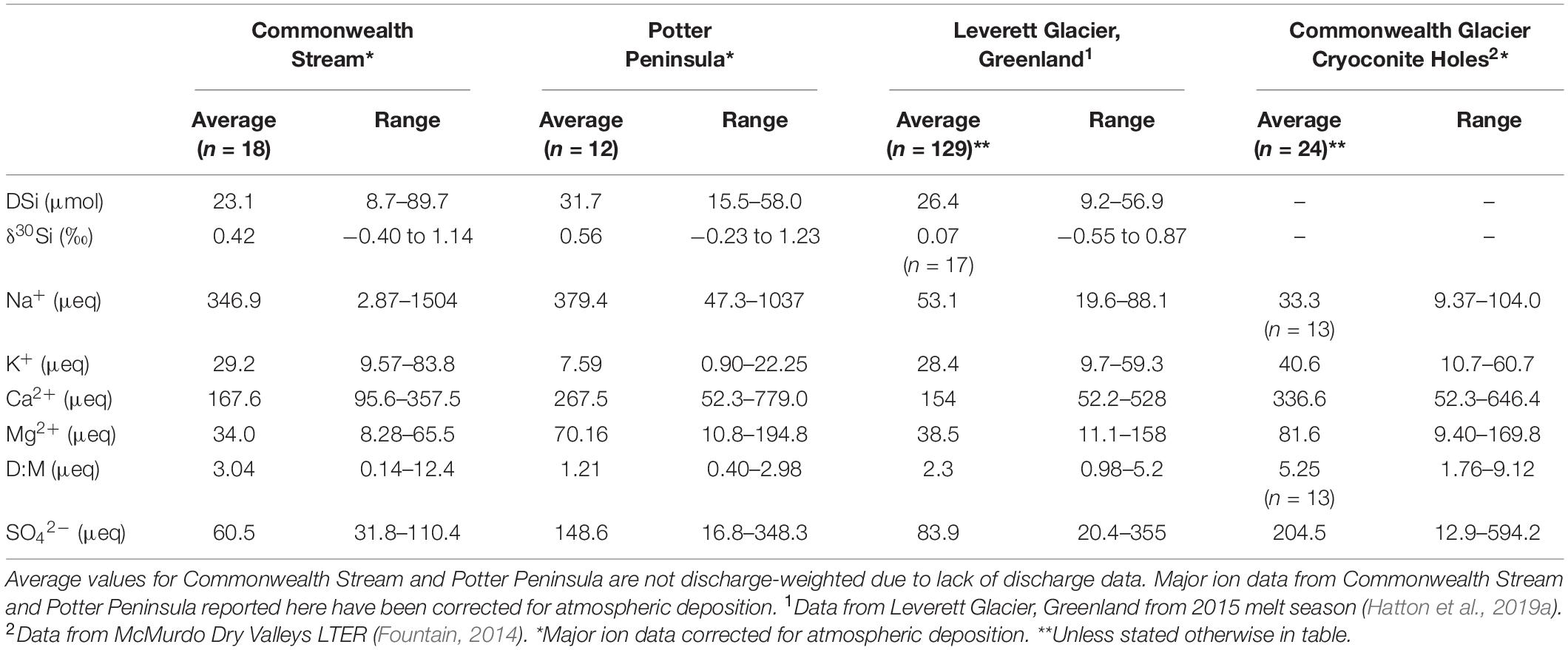
Table 1. Summary of geochemical data for Commonwealth Stream and Potter Peninsula (this study) and Leverett Glacier Greenland (Hatton et al., 2019a) for comparison to a well-categorized polythermal glacial system.
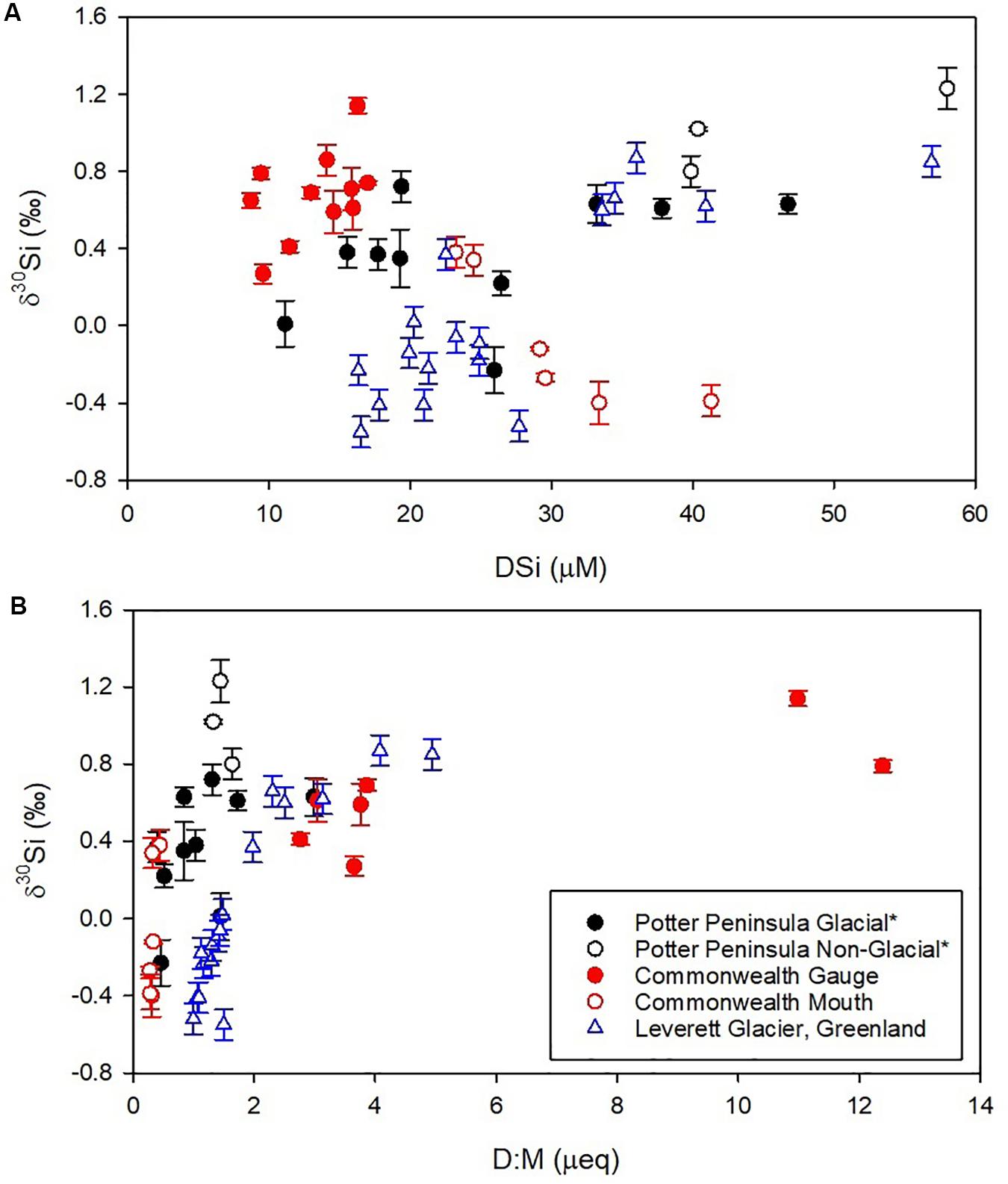
Figure 2. Silicon isotope (δ30Si) composition of Potter Peninsula, King George Island (black), Commonwealth Stream (red), Antarctica and Leverett Glacier, Greenland (blue). (A) δ30Si composition versus dissolved silicon (DSi) concentration and (B) δ30Si composition versus divalent/monovalent ratio (D:M) as a proxy for carbonate to silicate weathering. D:M ratios are calculated from major ion data corrected for atmospheric deposition for Potter Peninsula and Commonwealth Stream (demoted by *). Major ions from Leverett Glacier have not been corrected for atmospheric deposition, in line with standard practice of published data from Leverett Glacier. Error bars for Potter Peninsula and Commonwealth Stream are calculated from the 2SD of the external error of triplicated measurements, with an average of 0.07‰. Error bars for Leverett Glacier are 2SD external error of replicated standards (0.08‰, Hatton et al., 2019a).
There is a significant difference between the δ30SiDSi composition measured at the Gauging Station and Mouth Station in Commonwealth Stream, with the lightest δ30SiDSi composition at the Mouth Station. When separating the gauge and mouth samples, we see a much narrower δ30SiDSi composition range at both locations. The average gauge δ30SiDSi composition was +0.67‰ (+0.27 to +1.14‰) for the 3-year study period, and the average mouth δ30SiDSi composition was -0.08‰ (-0.40 to +0.38‰), for the 1 day of sample collection in January 2016. Sampling location is also an important factor on Potter Peninsula: samples collected from streams with no glacial influence in Matías Basin have consistently higher δ30SiDSi compositions (+0.8 to +1.23‰) than streams in Potter South, Potter North, and Fourcade Basin (-0.23 to +0.72‰), which are mainly influenced by glacial meltwaters.
The relationship between δ30SiDSi composition and DSi is contrasting between the Commonwealth Stream and Potter Peninsula (Figure 2), with higher DSi concentrations resulting in lower δ30SiDSi values in Commonwealth Stream (R2 = 0.665, p-value < 0.0001) but higher δ30SiDSi composition in Potter Peninsula (R2 = 0.545, p-value < 0.001). Figure 2 shows Potter Peninsula and Leverett Glacier, Greenland have similar relationships between δ30SiDSi composition, DSi, and D:M ratio. In contrast, Commonwealth Stream follows the typical relationship between δ30SiDSi composition and DSi in non-glacial rivers (Frings et al., 2016). We have chosen to use Leverett Glacier to consider how Potter Peninsula streams behave when compared to Arctic glaciers because Leverett Glacier is one of the most well-studied Arctic glaciers. The δ30SiDSi record from Leverett Glacier is also the most complete time-series, spanning almost the entire 2015 melt season, which was considered a relatively “typical” melt season in terms of discharge in recent years, with annual specific discharge in the same order of magnitude as Fourcade Glacier (see section “Materials and Methods”). While δ30SiDSi data exist from other glacial rivers in Arctic and sub-Arctic regions (see Hatton et al., 2019b for a complete dataset to date), these are mainly spot samples, without wider context. The data from Leverett Glacier provide the most comprehensive study of δ30SiDSi composition thus far, considering changes in dissolved and amorphous δ30Si over a melt season within the context of wider hydrogeochemical parameters, due to the large numbers of studies that have focused upon Leverett Glacier over several years. The relationships between δ30SiDSi and DSi or D:M compiled in Hatton et al. (2019b) are not statistically significant, but this is likely a reflection of the complexities in comparing a snapshot of numerous glacial systems with differing subglacial geologies, hydrological drainage developments, and/or water residence times. Thus we compare our data with Leverett Glacier in an attempt to ensure the comparisons are straightforward and inferences about the systems can be made.
Figure 2 also shows that there are systematically lower D:M ratios with lower δ30SiDSi composition in both systems, although relationships are not statistically significant, likely due to complexity of the environments. This concurs with the data from Arctic and sub-Arctic glaciers (Hatton et al., 2019b). There is also a narrower range (at lower values) of D:M ratios at Potter Peninsula, which may be expected as the bedrock is made up basaltic volcanic rocks, compared to a more heterogenous bedrock lithology in the MDV. However, this is unlikely to impact our interpretations of δ30SiDSi composition. This is because bedrock has little impact on riverine δ30SiDSi composition, due to the relatively homogeneous δ30SiDSi composition of bulk bedrock and the upper continental crust (Bulk Silicate Earth = -0.29 ± 0.08‰) compared to the large fractionation induced by weathering and biological processes (Savage et al., 2010; Frings et al., 2016).
Discussion
The similarity in the range of δ30SiDSi composition for both Potter Cove and Commonwealth Streams is surprising given the differences in glacier thermal regime and retreat history. However, a closer analysis of other geochemical parameters suggests these streams drain catchments in which there are contrasting geochemical weathering processes, which in turn influence the δ30SiDSi composition in each catchment. This is highlighted by the opposing relationships between DSi and δ30SiDSi composition in the two catchments. The negative relationship between DSi and δ30SiDSi composition in Commonwealth Stream is consistent with that found in the majority of global rivers and reflects the removal of Si from solution into secondary weathering products or biogenic silica (Sutton et al., 2018). In comparison, DSi and δ30SiDSi composition in Potter Peninsula follow a positive relationship, which is also reported in Greenlandic glacial rivers, and likely reflects an addition of Si from dissolution processes (Hatton et al., 2019a). This suggests that the absence of an active subglacial environment in the Dry Valley’s stream is a primary influence upon its chemical composition and renders the δ30SiDSi composition more similar to other non-glacial rivers. We can also use the D:M ratio of each catchment to infer differing processes between the two catchments, with changes in δ30SiDSi composition and the D:M ratio at Potter Peninsula driven by subglacial weathering, compared to in-stream weathering reactions in Commonwealth Stream in absence of subglacial weathering. In the following sections, we explore the key differences in ion ratios and δ30SiDSi composition in MDVs and Potter Peninsula.
In-Stream Weathering Impacts δ30SiDSi Composition in McMurdo Dry Valleys
Previous studies have found that the chemistry of streams in MDV is dominated by in-stream weathering processes, involving the dissolution of minerals from the stream bed and the hyporheic zone (Gooseff et al., 2002; McKnight et al., 2004; Welch et al., 2010). Interestingly, the major ion composition of stream waters at the Gauging Station was very similar to that of cryoconite holes on Commonwealth Glacier, although the Mouth Station samples differed significantly (Figure 3). Cryoconite holes are small, water filled holes (covered with an ice lid in MDV), which are created by the solar heating of aeolian debris on the surface of the glacier (Bagshaw et al., 2013; Fortner and Lyons, 2018). There is evidence that supraglacial melt impacts upon the streams and lakes downstream, particularly during high flow conditions (Lyons et al., 2003; Fortner et al., 2005; Bagshaw et al., 2007, 2013). Fortner et al. (2005) estimated that >80% of the discharge into Lake Hoare is of supraglacial origin from Canada Glacier, and Fountain et al. (2004) estimated that cryoconite holes contribute approximately 15% of the total water flux, highlighting the potential importance of the supraglacial melt system on stream chemistry. The interaction between meltwater and sediment in the cryoconite is the primary opportunity for solute acquisition before the water enters the ephemeral stream system. The similarity in major ion composition between cryoconite holes and stream water at the Gauging Station supports the assertion that the network of cryoconite holes is a critical geochemical component in the cold-based glacier system.
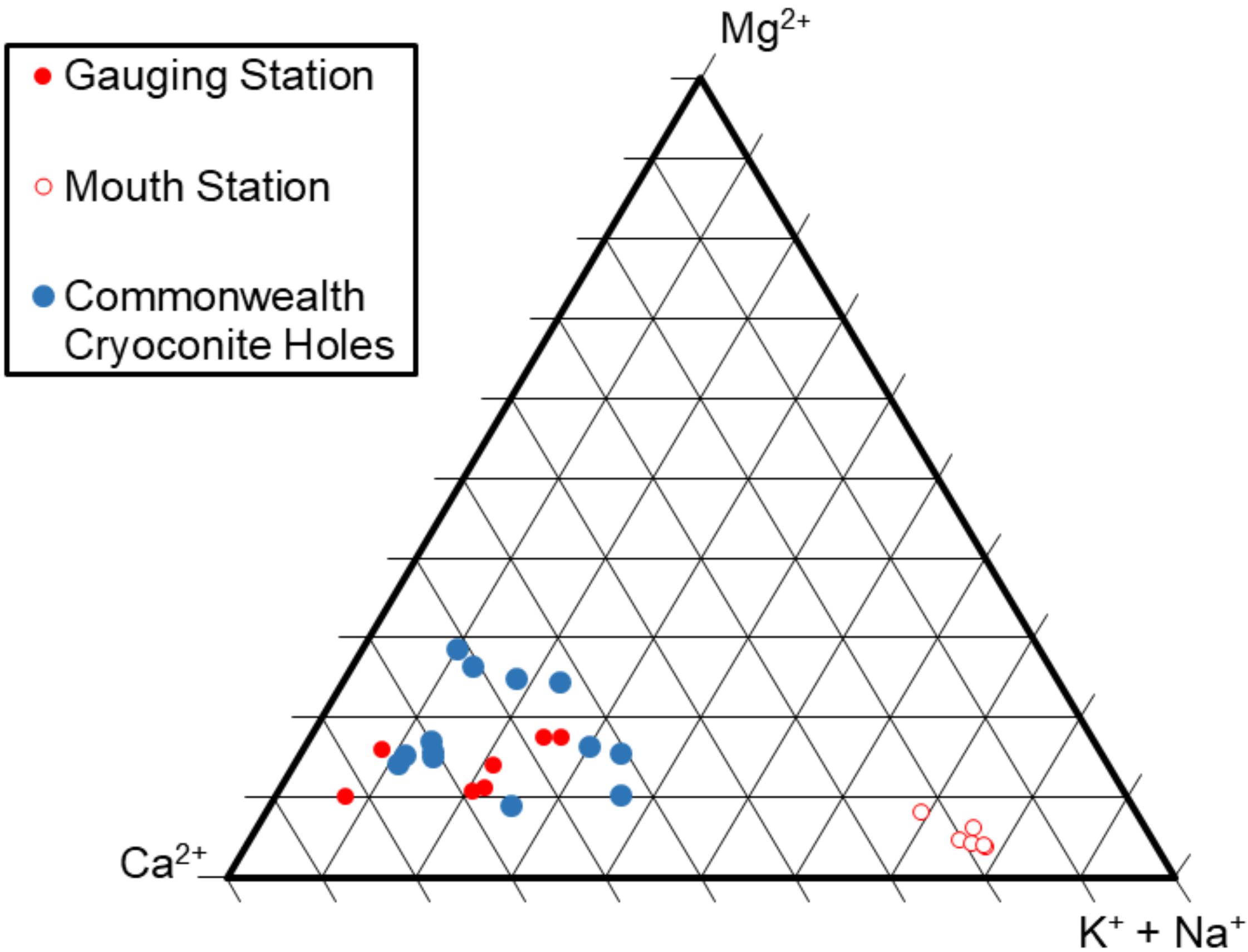
Figure 3. Ternary diagram comparing major ion composition of Commonwealth Stream and Commonwealth Cryoconite holes. All major ion data have been corrected for atmospheric deposition. Commonwealth Stream have been separated by gauge (closed red circles) and mouth (open red circles) samples. Data from Cryoconite holes on Commonwealth Glacier are from McMurdo Dry Valleys LTER (Fountain, 2014).
The δ30SiDSi composition at the Gauging Station is higher than other glacial rivers, indicating that the lack of high physical erosion rates and mechanochemical reactions beneath cold-based glaciers results in a riverine δ30SiDSi composition most similar to non-glacial rivers. The δ30SiDSi composition may also be impacted by diatom-containing microbial mats, which have been observed upstream of the Gauging Station in Commonwealth Stream. Diatoms utilize and potentially drawdown DSi, which would result in isotopic fractionation and residual waters downstream to be isotopically heavier. There is evidence of a relationship between high discharge events and particulate organic matter (Cullis et al., 2014), but there is a suggestion that the microbial mats are generally resilient under normal flow events. Thus, we would not expect large amounts of biogenic Si dissolution to occur from these microbial mats except potentially at very high flow events (above 100 L s–1). However, even at these high flow events at the Gauging Station, we see no impact upon δ30SiDSi composition (Figure 4), suggesting minimal fractionation inducing processes, such as precipitation or re-dissolution associated with changes in flow conditions. This is consistent with previous work in MDV that indicates that concentration-discharge relationships are chemostatic (Wlostowski et al., 2018), as high chemical weathering rates (e.g., Gooseff et al., 2002) result in short equilibrium timescales (Wlostowski et al., 2018).
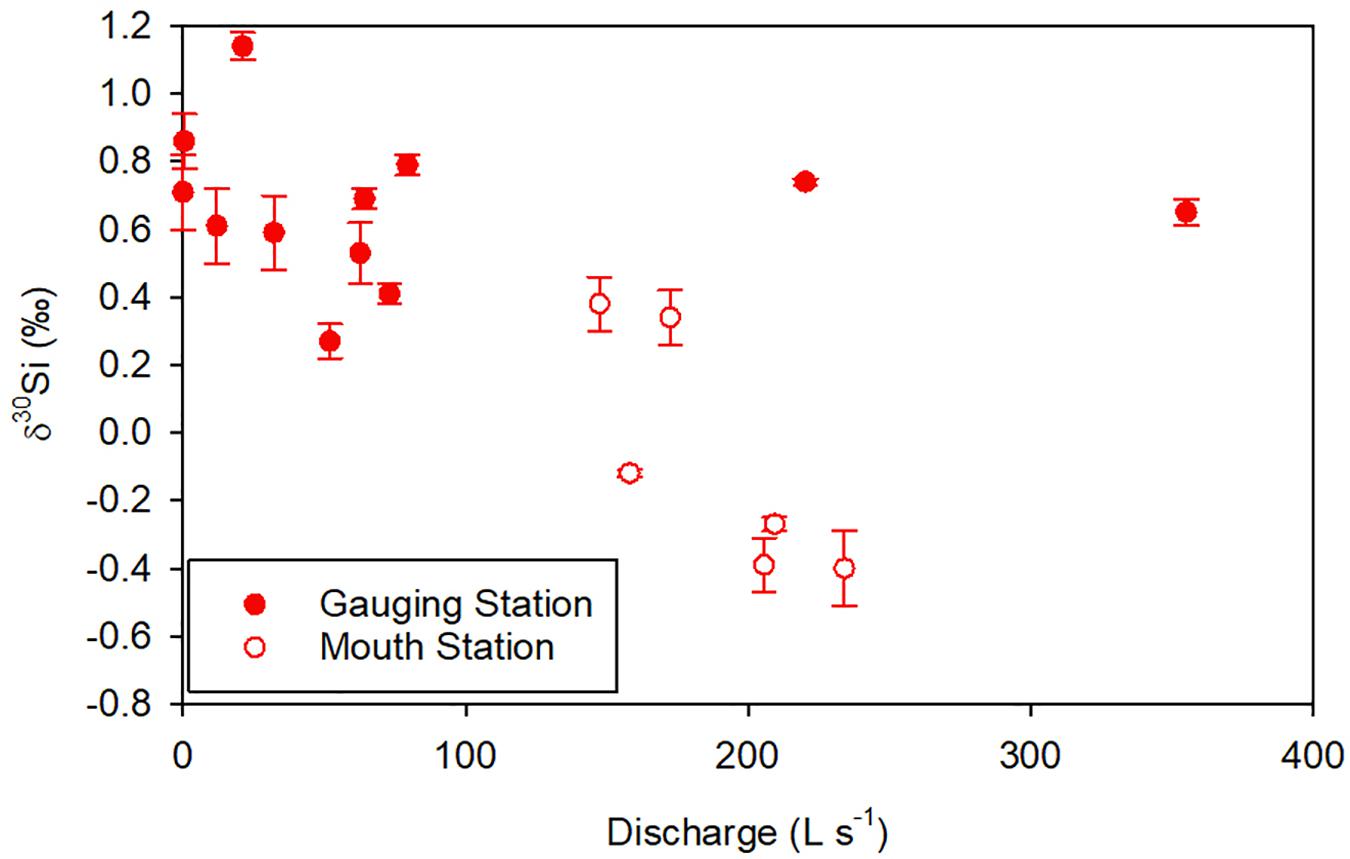
Figure 4. Impact of sampling location on silicon isotopic (δ30Si) composition in Commonwealth Stream. Samples collected further downstream at the mouth (open circles) have lower δ30Si composition at the same discharge as those collected upstream at the gauging station (closed circles). Error bars are calculated from the 2SD of the external error of triplicated measurements, with an average of 0.07‰.
In comparison, the δ30SiDSi composition at the Mouth Station is isotopically light, and the significant deviation from the cryoconite major ion proportions at this location demonstrates that in-stream weathering adds additional solute as the water progresses further through the Dry Valley hydrological system. However, it must be noted that we only have samples from the Mouth Station from one day in the 2016 melt season, at relatively high flow conditions and following a large discharge event. The flow conditions within the streams have a major impact on the geochemistry further downstream, since they control the interaction with the hyporheic zone (Gooseff et al., 2002). Hyporheic zones are the sediment and pore space adjacent to the streams, where water exchange occurs, and are zones of chemical weathering and sources of inorganic solutes (Gooseff et al., 2002). Interactions between the hyporheic zone and the stream increase downstream under all flow conditions, particularly in longer streams and interactions also increase as the season progresses as the temperature warms and the active layer depth increases. Under high flow conditions, the extent of the hyporheic may be further expanded due to the warmer conditions associated with high flow, resulting in further deepening of active layer. The similarity between stream major ion composition at the Mouth Station and hyporheic zone composition supports that the stream waters are influenced by the hyporheic zone during our sampling at the Mouth Station.
We hypothesize that the high flow and interactions with the hyporheic zone may also have influenced the δ30SiDSi composition at the Mouth Station, via enhanced silicate dissolution. For example, there is a relationship between discharge and δ30Si composition at the Mouth Station. The stream was sampled over a 4-h period while discharge was increasing (Supplementary Figure S1) and higher discharge resulted in lower δ30Si composition (Figure 3). The discharge during the 2015/16 flow season was generally much higher than preceding and subsequent flow seasons, with a peak flow 2 weeks prior to sample collection at the Mouth Station of 900 L s–1 (Supplementary Figure S1), which is nine times greater than the threshold for a “flood event” (Cullis et al., 2014). We would expect the active layer to have thawed more by the time we sampled at the Mouth Station, as the active layer thaws more later in the season (Conovitz et al., 2006) and this large flood event will have promoted greater active layer thaw due to the associated higher temperatures. We therefore predict geomorphic disturbances of the stream bed may have occurred, which could have altered flow paths and may have resulted in water exchange in areas of the hyporheic zone that have been undergoing weathering for longer in relative isolation. The creation of new flow paths could therefore promote the dissolution of weathering products, which would result in isotopically lighter δ30SiDSi composition of the stream waters. There is also evidence of increased suspended particulate matter (SPM) in the MDV streams in recent years, with fine sediments coming from the stream banks (Gooseff et al., 2016; Leslie et al., 2017) and expansion of the wetted perimeter during high flow may have led to the dissolution of channel-margin salt crusts (Wlostowski et al., 2018). While the streams are usually very clear and lack suspended sediments (Gooseff et al., 2016), it may have been that during this high flow event a higher proportion of fine sediment was entrained into the stream. Previous studies of Commonwealth Stream have shown an increase in dissolved Si concentrations downstream (Olund et al., 2018), indicating a dissolution of primary minerals and SEM images of stream sediments provide evidence of primary mineral alteration (Supplementary Figure S4). This is consistent with the δ30SiDSi composition data, since primary mineral dissolution will result in lower δ30SiDSi composition and this dissolution would be promoted in an undersaturated system. Hyporheic waters have been shown to be undersaturated with respect to albite, chlorite, illite, K-feldspar, quartz, and SiO2 (Gooseff et al., 2002), which further supports the notion that silicate dissolution continues to add solute to river waters downstream. SEM images (Supplementary Figure S4) also indicate the presence of secondary weathering products, potentially smectite or zeolite, which would be expected, considering findings from suspended sediments from other streams in MDV. Sediments from both Huey and Green Creeks have considerable amorphous weathering products, with a higher proportion of clay materials compared to primary minerals (Gooseff et al., 2002). Dissolution of these secondary weathering products would also result in an influx of isotopically light silicon, which could have lowered the δ30Si composition of the stream.
The difference in δ30SiDSi composition between the Gauging Station and the Mouth Station, and the consistent δ30SiDSi composition at the Gauging Station despite changes in discharge suggests that downstream transport impacts stream chemistry and δ30SiDSi composition, likely due to mineral dissolution. However, we must also consider that the high flow conditions during sampling at the Mouth Station may have an impact, which may not wholly reflect the downstream conditions and δ30SiDSi composition exported into the McMurdo Sound under present climatic conditions. It would be advisable to consider the average δ30SiDSi composition from the Gauging Station as more likely to be representative of streams within the MDV, as most streams are relatively short resulting in less interactions with the hyporheic zones and thus less mineral dissolution, which is consistent with findings from Hirst et al. (Submitted to this Special Issue). The heavier δ30SiDSi compositions of Crescent Stream indicate that the precipitation of secondary weathering products, rather than mineral dissolution is driving the stream δ30SiDSi composition, with additional influence of different stream bed and hyporheic zone weathering conditions. These could include variations in (i) the starting primary mineral compositions, (ii) extent of secondary mineral formation, (iii) hyporheic zone width and thus area over which weathering can occur, (iv) stream flow and thus stream erosive capacity, and (v) extent of diatom uptake and dissolution in stream microbial mats. The discrepancy between Si concentrations and isotopic compositions between the Mouth and Gauging stations are related to downstream weathering processes and/or high flow conditions. The data from the Mouth Station may represent a high-flow endmember which could be useful when considering future changes to the MDV. In combination, these observations highlight the potential for different hyporheic zone processes to mediate the δ30SiDSi composition in MDV streams. These findings contribute to our understanding of Si weathering fluxes in MDV streams and provide a baseline for predicting future Si weathering fluxes in response to future climate change. There is evidence that the MDV is responding to climatic warming, with annual discharge between 2001 and 2016 being higher than the 8 years measured prior (Olund et al., 2018, MCM-LTER record1). Increasing temperatures are predicted to increase glacial melt and potentially increase the prevalence of “warm years” that experience higher than average temperatures and associated melt (Fountain et al., 2016). This may also lead to deepening of active layers, and increases of hyporheic zones and melt of buried ice (Fountain et al., 2014). These changes are likely to impact rock–water interaction times, weathering regimes and biogeochemical reactions within the streams (Olund et al., 2018), and δ30SiDSi data from the Mouth Station of Commonwealth Stream suggest that this could lead to stream waters with isotopically lighter δ30SiDSi composition.
Glacial Meltwaters of Potter Peninsula Impact Stream δ30SiDSi Composition
In contrast to MDV, which may see warming and associated changes in the future but are currently extremely cold, dry environments, the Fourcade Glacier on King George Island, has undergone rapid and significant retreat over the past decades. We have noted that the overall δ30SiDSi composition of Commonwealth Stream and Potter Peninsula is relatively similar; however, the DSi concentrations and major ion chemistry highlight differences in weathering processes. The geochemical relationships in surficial meltwater streams of Potter Peninsula reflect those of rivers draining glaciers with active subglacial environments, thus indicating that the difference in thermal regime between Commonwealth Glacier and Fourcade Glacier impacts upon silicon cycling.
It is important to note that streams on Potter Peninsula are fed from a mixture of sources; glacial melt, permafrost thaw, precipitation, and snow melt. It was not within the scope of this study to estimate the proportion of glacially derived waters into these streams due to complex and heterogenous nature of the mixed stream inputs (Silva-Busso et al., 2004), so we use hydrological basins defined by Silva-Busso (2009) to consider the likelihood of streams being impacted by glacial meltwater (Supplementary Figure S2).
Streams sampled in Matías Basin are considered as the non-glacial endmember, as this basin has no input from Fourcade glacial melt, as evidenced by the strong correlation between precipitation and discharge. Instead, streams in Matías Basin are likely dominated by precipitation, snowmelt, and seasonal permafrost melt, with the rapid development of the permafrost active layer in the summer. Below 60 m.a.s.l, permafrost on Potter Peninsula is discontinuous and there is evidence of approximately 30 m of thawed talik in the sediments of Matías Basin, covering basaltic bedrock. Streams in Matías Basin have consistently higher δ30SiDSi composition than all other streams on the Peninsula, consistent with previous observations of streams impacted by permafrost, where amorphous Si precipitation and clay formation result in fractionation (Pokrovsky et al., 2013; Sun et al., 2018; Hirst et al., 2020).
Streams in Potter North, Potter South, and Fourcade Basins have inputs from glacial meltwater; therefore, we group these streams together as “glacial” for discussion purposes (see Supplementary Material for further information). Glacial melt is routed via the subglacial environment, and then drains directly into surficial streams or enters streams via groundwater flow. However, we recognize that the proportion of glacial meltwater varies between streams and over time due to climatic factors and we are unable to quantify that in this study. Field observations indicate that subglacial meltwater contributes to the streams within Potter South, Potter North, and Fourcade Basin (Silva-Busso, 2009; Monien et al., 2017). Previously studied rivers draining warm or polythermal glaciers exhibit a positive relationship between δ30SiDSi composition and DSi concentration (Opfergelt et al., 2013; Sutton et al., 2018; Hatton et al., 2019a), which is opposite to the general trend seen in non-glacial rivers (Frings et al., 2016; Sutton et al., 2018). This positive relationship has been attributed to subglacial weathering processes and changes in discharge over the melt season. Subglacial weathering and changes in discharge are likely what is driving the δ30SiDSi composition of Potter Peninsula glacial streams. However, without discharge data from Potter Peninsula, we have to infer similar processes are occurring, based upon the similar relationship between DSi and δ30SiDSi as at Leverett Glacier. It has been hypothesized that the high physical erosion rates in the subglacial environment result in freshly ground mineral surfaces which are enriched in isotopically light Si (Ziegler et al., 2005; Hatton et al., 2019b). During times of high discharge, the melt waters access amorphous silica (ASi) surface layers, leading to re-dissolution and driving an increase in DSi concentration and a lower δ30SiDSi composition. However, the proportion of ASi that needs to undergo dissolution is minimal, thus the absolute DSi concentration decreases, due to the dilution effect of overall higher discharge (Hatton et al., 2019a).
The similar patterns between δ30SiDSi and DSi and major ion composition seen in Potter Peninsula glacial streams compared to Leverett Glacier suggest that the subglacial weathering processes under Fourcade Glacier may be comparable to those in under Arctic and sub-Arctic polythermal glaciers. Monien et al. (2017) describe higher SPM concentrations in streams in Potter South (2011 average of 283 ± 99 mg L–1) compared to Matías Basin (consistently < 38 mg L–1). This suggests that the glacially linked erosion processes could result in weathering product dissolution and therefore influence the δ30SiDSi composition of these streams. However, it is important to consider that the high SPM in the streams of Potter South (and other glacial basins) are likely resulting from the proglacial environment in addition to the active subglacial system. For example, sites that are more recently ice free (such as those in Potter South) have higher proportions of freshly ground material (Monien et al., 2017). While measurements of proglacial till at Leverett Glacier, Greenland, showed very low ASi concentrations (Hatton et al., 2019a), the relatively recent retreat of Fourcade Glacier could mean that these sediments have not undergone as much weathering in the proglacial environment and the dissolution processes that occur when the streams flow in summer are similar to subglacial dissolution of ASi.
When considering the drivers of δ30SiDSi composition in the glacial streams on Potter Peninsula, we must also acknowledge that the streams in this study were not sampled immediately at the glacier terminus and thus other water sources may contribute to the streams. Therefore, the variation in meltwater proportion to the streams on Potter Peninsula may help to explain the range of δ30SiDSi values for streams, with higher proportions of glacial meltwater and/or less influence from permafrost thaw resulting in lower δ30SiDSi composition. Lentic systems also exist with the basins, which host active phytoplankton communities. This may help to explain why, although isotopically light, the δ30SiDSi composition of the stream waters from Fourcade Glacier is not as light as at Leverett Glacier, where no phytoplankton activity is present and the proglacial river measured is almost entirely sourced from the subglacial environment.
Interestingly, the lightest δ30SiDSi value measured on Potter Peninsula (-0.23‰) was from a stream within the Fourcade Basin, downstream of a lake. The presence of microbial mats in the lakes of Potter Peninsula (Vinocur and Pizarro, 2000) may result in biological uptake of Si and isotopic fractionation, leading to higher δ30SiDSi composition in outflow streams. However, it may be that a large proportion of the stream is fed by groundwater flow, originating subglacially. This could help to explain the isotopically light DSi via re-dissolution of mineral weathering products, similar to the processes hypothesized in subglacial environments with elevated rock–water interaction times (Hatton et al., 2019a), especially considering the present of fluvial-glacial sediments in the region. However, we are unable to quantify this with the present dataset, and would recommend an integrated study of these streams in the future, quantifying both water sources and flow paths to better understand the drivers of stream geochemistry in the region.
The Si fluxes from King George Island may not be as significant as Si export from Arctic glaciers for the downstream ecosystem, as surrounding coastal waters are already elevated in DSi. For example, subsurface seawater of adjacent seas of King George Island has an average Si concentration of 60 μM (Ye et al., 2018). However, this environment does provide an interesting study location, with streams potentially impacted by contrasting weathering regimes depending on the influence of glacial meltwaters. The glacial streams provide further understanding of silicon cycling below polythermal glaciers and allow glacial δ30SiDSi composition to be informed by glaciers beyond the Arctic and sub-Arctic. That the δ30SiDSi composition of streams fed by Fourcade Glacier is within the range of other well-established glacial rivers may indicate that isotopically light δ30SiDSi composition of (polythermal) glacial meltwaters is a bi-polar phenomenon and will assist in informing global Si models.
However, the complexity of the streams within Potter Peninsula also highlights the importance of understanding the hydrogeochemistry of these environments when considering potential nutrient fluxes into the marine environment. For example, the potential differences in the streams not fed by glacial meltwaters in Matías Basin, which are a greater distance from the glacier front may present an interesting consideration of the importance of characterizing these rapidly changing environments, so that we can better estimate the likely changes to the glacial Si cycle in the future. As Fourcade Glacier retreats further, more areas of the Peninsula will be exposed resulting in higher proportions of recently eroded sediments to be transported. However, streams closer to the coast may be increasingly dominated by permafrost-weathering processes as meltwater streams have less of an impact further from the retreating glacier front. It is therefore likely that the δ30SiDSi composition of these streams will be impacted in the future under climatic warming and improving of our understanding of the drivers of Si cycling in these environments is key to being able to better predict these changes.
Conclusion
Silicon export and corresponding δ30SiDSi composition from glacially environments has recently been of interest due to its usefulness in understanding subglacial weathering processes and potential implications for downstream ecosystems. We have presented the first δ30SiDSi composition data for Antarctic streams, helping to extend the data availability of δ30SiDSi composition in glaciated areas. We have shown that the δ30SiDSi composition of streams from two contrasting environments on the Antarctic continent is very similar, yet both systems are driven by differing processes. In-stream weathering processes dominate the geochemistry of Commonwealth Stream in MDV, as highlighted by previous studies of the area, and there is a negative relationship between DSi and δ30Si composition, resembling non-glacial rivers. These in-stream processes include the dissolution of primary minerals, resulting in lower δ30SiDSi composition at the stream mouth compared to further upstream toward to glacier. In comparison, glacially fed streams on Potter Peninsula behave very similarly to previously studied Arctic and sub-Arctic glacial rivers, and there is a positive relationship between DSi and δ30Si composition. It is therefore likely that dissolution of weathering products from subglacial or recently exposed sediments impact the δ30SiDSi composition of glacial streams on Potter Peninsula. We expect that these weathering processes, dominated by high physical erosion rates, are ubiquitous to wet-based glaciers and we should expect low δ30SiDSi composition from the glacial rivers compared to non-glacial rivers.
The MDV and WAP are expected to undergo major changes due to climatic warming, which could impact on weathering processes, sediment loads, and export to the downstream ecosystems. Therefore, understanding the processes occurring at present is fundamental to being able to predict what changes may occur and inform future predictions.
Data Availability Statement
The datasets generated for this study are available from https:// doi.pangaea.de/10.1594/PANGAEA.914103.
Author Contributions
KH and JH conceived the project. SH, SW, MS, WL, AS-B, and DM conducted fieldwork, sample collection, and hydrological monitoring. JH and SW completed laboratory analysis. All authors contributed to the data discussion and writing of the manuscript.
Funding
JH and KH were funded by the European Research Council starting grant ICY-LAB (agreement number 678371) and Royal Society Enhancement Award (RGF\EA\181036). Field campaigns to King George Island were funded via the German Research Foundation (DFG grant numbers STA 936/5-1 and KA 2769/3-1). CH and SO were funded by the European Research Council starting grant WeThaw (agreement number 714617) and National Funds for Scientific Research FNRS (Grant Nos. 26043653 and FC69480). WL and SW were funded by NSF grant to the MCM LTER project, OPP-ANT 1115249.
Conflict of Interest
The authors declare that the research was conducted in the absence of any commercial or financial relationships that could be construed as a potential conflict of interest.
Acknowledgments
The authors thank Jochen Scheld (University of Cologne), Jan Hartmann (MARUM), the staff of the Argentinian research station Carlini, and the adjoint Dallmann laboratory of AWI for field support on King George Island. The authors also thank the McMurdo Dry Valleys LTER team, particularly the Stream Team for assistance and use of the flow data. The authors are also grateful for laboratory support from Dr. Christopher D. Coath (Bristol Isotope Group) for isotopic data and Subsurface Energy Materials Characterization and Analysis Laboratory (SEMCAL), School of Earth Sciences, The Ohio State University for SEM images. The authors also thank the editor, Ramanathan Alagappan, and reviewers for their time and thorough reviews to improve the manuscript.
Supplementary Material
The Supplementary Material for this article can be found online at: https://www.frontiersin.org/articles/10.3389/feart.2020.00286/full#supplementary-material
Footnotes
References
Annett, A. L., Skiba, M., Henley, S. F., Venables, H. J., Meredith, M. P., Statham, P. J., et al. (2015). Comparative roles of upwelling and glacial iron sources in Ryder Bay, coastal western Antarctic Peninsula. Mar. Chem. 176, 21–33. doi: 10.1016/j.marchem.2015.06.017
Ardelan, M. V., Holm-Hansen, O., Hewes, C. D., Reiss, C. S., Silva, N. S., Dulaiova, H., et al. (2010). Natural iron enrichment around the Antarctic Peninsula in the Southern Ocean. Biogeosciences 7, 11–25. doi: 10.5194/bg-7-11-2010
Bagshaw, E. A., Tranter, M., Fountain, A. G., Welch, K., Basagic, H. J., and Lyons, W. B. (2013). Do cryoconite holes have the potential to be significant sources of C, N, and P to downstream depauperate ecosystems of Taylor valley, Antarctica? Arctic Antarctic Alpine Res. 45, 440–454. doi: 10.1657/1938-4246-45.4.440
Bagshaw, E. A., Tranter, M., Fountain, A. G., Welch, K. A., Basagic, H., and Lyons, W. B. (2007). Biogeochemical evolution of cryoconite holes on Canada Glacier, Taylor Valley, Antarctica. J. Geophys. Res. 112:442.
Birkenmajer, K. (1980). Geology of admiralty bay, King George Island (South Shetland Islands) - an outline. Pol. Polar Res. 1, 29–54.
Cardinal, D., Alleman, L. Y., De Jong, J., Ziegler, K., and Andre, L. (2003). Isotopic composition of silicon measured by multicollector plasma source mass spectrometry in dry plasma mode. J. Analyt. Atom. Spectrom. 18, 213–218. doi: 10.1039/b210109b
Conovitz, P. A., Macdonald, L. H., and Mcknight, D. M. (2006). Spatial and temporal active layer dynamics along three glacial meltwater streams in the McMurdo Dry Valleys, Antarctica. Arctic Antarctic Alpine Res. 38, 42–53. doi: 10.1657/1523-0430(2006)038[0042:satald]2.0.co;2
Crompton, J. W., Flower, G. E., Kirste, D., Hagedorn, B., and And Sharp, M. J. (2015). Clay mineral precipitation and low silica in glacier meltwaters explored through reaction-path modelling. J. Glaciol. 61, 1061–1078. doi: 10.3189/2015jog15j051
Cuffey, K. M., Conway, H., Gades, A. M., Hallet, B., Lorrain, R., Severinghaus, J. P., et al. (2000). Entrainment at cold glacier beds. Geology 28, 351–354. doi: 10.1130/0091-7613(2000)028<0351:eacgb>2.3.co;2
Cullis, J. D. S., Stanish, L. F., and Mcknight, D. M. (2014). Diel flow pulses drive particulate organic matter transport from microbial mats in a glacial meltwater stream in the McMurdo Dry Valleys. Science 50, 86–97. doi: 10.1002/2013wr014061
Ermolin, E., and Silva-Busso, A. (2008). “Interaction between permafrost and groundwater on the potter Peninsula, King george Island, (Isla 25 de Mayo), Antarctica,” in The Antarctic Ecosystem Of Potter Cove, King-George Island (Isla 25 de MAYO). Synopsis of Research Performed 1999-2006 at the Dallmann Laboratory and Jubany Station Alfred Wegener Institute, eds C. Wiencke, G. A. Ferreyra, D. Abele, and S. Marenssi, (Villa Lynch: Instituto Antartico Argentino).
Falk, U., López, D. A., and Silva-Busso, A. (2018). Multi-year analysis of distributed glacier mass balance modelling and equilibrium line altitude on King George Island, Antarctic Peninsula. Cryosphere 12, 1211–1232. doi: 10.5194/tc-12-1211-2018
Fortner, S. K., and Lyons, W. B. (2018). Dissolved trace and minor elements in cryoconite holes and supraglacial streams, Canada glacier, Antarctica. Front. Earth Sci. 6:31. doi: 10.3389/feart.2018.00031
Fortner, S. K., Tranter, M., Fountain, A., Lyons, W. B., and Welch, K. A. J. A. G. (2005). The geochemistry of supraglacial streams of canada glacier, Taylor Valley (Antarctica), and their evolution into Proglacial waters. Cryosphere 11, 391–412. doi: 10.1007/s10498-004-7373-2
Fountain, A. G. (2014). McMurdo Dry Valleys Lter: geochemistry data of cryoconite holes collected from Canada, commonwealth, howard, hughes and Taylor glaciers in Nov 2001 and Jan 2002. Environ. Data Initiat. doi: 10.6073/pasta/ba862a096fbc7fca4063c44ad3af3c0f
Fountain, A. G., Levy, J. S., Gooseff, M. N., and Van Horn, D. (2014). The McMurdo Dry Valleys: a landscape on the threshold of change. Geomorphology 225, 25–35. doi: 10.1016/j.geomorph.2014.03.044
Fountain, A. G., Saba, G., Adams, B., Doran, P., Fraser, W., Gooseff, M., et al. (2016). The impact of a large-scale climate event on antarctic ecosystem processes. Bioscience 66, 848–863. doi: 10.1093/biosci/biw110
Fountain, A. G., Tranter, M., Nylen, T. H., Lewis, K. J., and Mueller, D. R. (2004). Evolution of cryoconite holes and their contribution to meltwater runoff from glaciers in the McMurdo Dry Valleys, Antarctica. J. Glaciol. 50, 35–45. doi: 10.3189/172756504781830312
Frings, P. J., Clymans, W., Fontorbe, G., De La Rocha, C., and Conley, D. J. (2016). The continental Si cycle and its impact on the ocean Si isotope budget. Chem. Geol. 425, 12–36. doi: 10.1016/j.chemgeo.2016.01.020
Georg, R. B., Reynolds, B. C., West, A. J., Burton, K. W., and Halliday, A. N. (2007). Silicon isotope variations accompanying basalt weathering in Iceland. Earth Planet. Sci. Lett. 261, 476–490. doi: 10.1016/j.epsl.2007.07.004
Gerringa, L. J. A., Alderkamp, A. C., Laan, P., Thuroczy, C. E., De Baar, H. J. W., Mills, M. M., et al. (2012). Iron from melting glaciers fuels the phytoplankton blooms in Amundsen Sea (Southern Ocean): iron biogeochemistry. Deep Sea Res. Part Top. Stud. Oceanogr. 71–76, 16–31. doi: 10.1016/j.dsr2.2012.03.007
Gooseff, M. N., Mcknight, D. M., Lyons, W. B., and Blum, A. E. (2002). Weathering reactions and hyporheic exchange controls on stream water chemistry in a glacial meltwater stream in the McMurdo Dry Valleys. Chem. Geol. 38, 15–17.
Gooseff, M. N., Van Horn, D., Sudman, Z., Mcknight, D. M., Welch, K. A., and Lyons, W. B. (2016). Stream biogeochemical and suspended sediment responses to permafrost degradation in stream banks in Taylor Valley, Antarctica. Biogeosciences 13, 1723–1732. doi: 10.5194/bg-13-1723-2016
Grosbois, C., Négrel, P., Fouillac, C., and Grimaud, D. (2000). Dissolved load of the loire river: chemical and isotopic characterization. Chem. Geol. 170, 179–201. doi: 10.1016/s0009-2541(99)00247-8
Hall, B. L., and Denton, G. H. (2000). Radiocarbon chronology of ross sea drift, eastern taylor valley, antarctica: evidence for a grounded ice sheet in the ross sea at the last glacial maximum. Geograf. Ann. 82, 305–336. doi: 10.1111/j.0435-3676.2000.00127.x
Hambrey, M. J., and Fitzsimons, S. J. (2010). Development of sediment-landform associations at cold glacier margins, Dry Valleys, Antarctica. Sedimentology 57, 857–882. doi: 10.1111/j.1365-3091.2009.01123.x
Hatton, J. E., Hendry, K. R., Hawkings, J. R., Wadham, J. L., Kohler, T. J., Stibal, M., et al. (2019a). Investigation of subglacial weathering under the Greenland Ice Sheet using silicon isotopes. Geochim. Cosmochim. Acta 247, 191–206. doi: 10.1016/j.gca.2018.12.033
Hatton, J. E., Hendry, K. R., Hawkings, J. R., Wadham, J. L., Opfergelt, S., Kohler, T. J., et al. (2019b). Silicon isotopes in arctic and sub-arctic glacial meltwaters: the role of subglacial weathering in the silicon cycle. R. Soc. Proc. A 475:98.
Hawkings, J., Wadham, J., Tranter, M., Telling, J., Bagshaw, E., Beaton, A., et al. (2016). The Greenland Ice Sheet as a hot spot of phosphorus weathering and export in the Arctic. Glob. Biogeochem. Cycles 30, 191–210. doi: 10.1002/2015gb005237
Hawkings, J. R., Hatton, J. E., Hendry, K. R., De Souza, G. F., Wadham, J. L., Ivanovic, R., et al. (2018). The silicon cycle impacted by past ice sheets. Nat. Commun. 9:3210.
Hawkings, J. R., Wadham, J. L., Benning, L. G., Hendry, K. R., Tranter, M., Tedstone, A., et al. (2017). Ice sheets as a missing source of silica to the polar oceans. Nat. Commun. 8:14198.
Hawkings, J. R., Wadham, J. L., Tranter, M., Lawson, E., Sole, A., Cowton, T., et al. (2015). The effect of warming climate on nutrient and solute export from the Greenland Ice Sheet. Geochem. Perspect. Lett. 1, 94–104. doi: 10.7185/geochemlet.1510
Hawkings, J. R., Wadham, J. L., Tranter, M., Raiswell, R., Benning, L. G., Statham, P. J., et al. (2014). Ice sheets as a significant source of highly reactive nanoparticulate iron to the oceans. Nat. Commun. 5:3929.
Hendry, K. R., Huvenne, V. A. I., Robinson, L. F., Annett, A., Badger, M., Jacobel, A. W., et al. (2019). The biogeochemical impact of glacial meltwater from Southwest Greenland. Prog. Oceanogr. 176:102126. doi: 10.1016/j.pocean.2019.102126
Henkel, S., Kasten, S., Hartmann, J. F., Silva-Busso, A., and Staubwasser, M. (2018). Iron cycling and stable Fe isotope fractionation in Antarctic shelf sediments, King George Island. Geochim. Cosmochim. Acta 237, 320–338. doi: 10.1016/j.gca.2018.06.042
Henley, S. F., Jones, E. M., Venables, H. J., Meredith, M. P., Firing, Y. L., Dittrich, R., et al. (2018). Macronutrient and carbon supply, uptake and cycling across the Antarctic Peninsula shelf during summer. J. Oceanogr. 376:20170168. doi: 10.1098/rsta.2017.0168
Hirst, C., Opfergelt, S., Gaspard, F., Hendry, K. R., Hatton, J. E., Welch, S., et al. (2020). Silicon isotopes reveal a non-glacial source of silicon to Crescent Stream, McMurdo Dry Valleys, Antarctica. Front. Earth Sci. 8:299. doi: 10.3389/feart.2020.00229
Hodson, A., Nowak, A., Sabacka, M., Jungblut, A., Navarro, F., Pearce, D., et al. (2017). Climatically sensitive transfer of iron to maritime Antarctic ecosystems by surface runoff. Nat. Commun. 8:14499.
Höfer, J., Giesecke, R., Hopwood, M. J., Carrera, V., Alarcón, E., and González, H. E. (2019). The role of water column stability and wind mixing in the production/export dynamics of two bays in the Western Antarctic Peninsula. Prog. Oceanogr. 174, 105–116. doi: 10.1016/j.pocean.2019.01.005
Hood, E., Fellman, J., Spencer, R. G. M., Hernes, P. J., Edwards, R., D’amore, D., et al. (2009). Glaciers as a source of ancient and labile organic matter to the marine environment. Nature 462, 1044–U100.
Hopwood, M. J., Carroll, D., Browning, T. J., Meire, L., Mortensen, J., Krisch, S., et al. (2018). Non-linear response of summertime marine productivity to increased meltwater discharge around Greenland. Nature 9:3256.
Hughes, H. J., Delvigne, C., Korntheuer, M., De Jong, J., André, L., and Cardinal, D. (2011). Controlling the mass bias introduced by anionic and organic matrices in silicon isotopic measurements by Mc-Icp-Ms. J. Analyt. Atom. Spectrom. 26:1892. doi: 10.1039/c1ja10110b
Huh, Y., Chan, L.-H., Zhang, L., and Edmond, J. M. (1998). Lithium and its isotopes in major world rivers: implications for weathering and the oceanic budget. Geochim. Cosmochim. Acta 62, 2039–2051. doi: 10.1016/s0016-7037(98)00126-4
Jerosch, K., Pehlke, H., Monien, P., Scharf, F., Weber, L., Kuhn, G., et al. (2018). Benthic meltwater fjord habitats formed by rapid glacier recession on King George Island, Antarctica. Philos. Trans. R. Soc. A 376:20170178. doi: 10.1098/rsta.2017.0178
Kraus, S. (2005). Magmatic Dyke Systems Of The South Shetland Islands Volcanic Arc (West Antarctica): Reflections Of The Geodynamic History. Ph.D. Munich University Library, Munich.
Lawson, E. C., Wadham, J. L., Tranter, M., Stibal, M., Lis, G. P., Butler, C. E. H., et al. (2014). Greenland ice sheet exports labile organic carbon to the Arctic oceans. Biogeosciences 11, 4015–4028. doi: 10.5194/bg-11-4015-2014
Leal, M. A., Joppert, M., Licínio, M. V., Evangelista, H., Maldonado, J., Dalia, K. C., et al. (2008). Atmospheric impacts due to anthropogenic activities in remote areas: the case study of admiralty bay/king george island/Antarctic peninsula. Water Air Soil Pollut. 188, 67–80. doi: 10.1007/s11270-007-9525-7
Lee, J. R., Raymond, B., Bracegirdle, T. J., Chadès, I., Fuller, R. A., Shaw, J. D., et al. (2017). Climate change drives expansion of Antarctic ice-free habitat. Nature 547, 49–54. doi: 10.1038/nature22996
Leslie, D. L., Welch, K. A., and Lyons, W. B. (2017). A temporal stable isotopic (δ18O, δD, d-excess) comparison in glacier meltwater streams, Taylor Valley, Antarctica. Cell 31, 3069–3083. doi: 10.1002/hyp.11245
Lusky, J., Vallverdú, E., Gómez, I., Del Valle, R., and Felske, H. (2001). Map of Potter Peninsula. King George (25 de Mayo) Island, South Shetland Islands, Antarctica. Münich: Instituto Antártico Argentino-Institut für Allgemeine und Angewandte.
Lyons, W. B., Dailey, K. R., Welch, K. A., Deuerling, K. M., Welch, S. A., and Mcknight, D. M. (2015). Antarctic streams as a potential source of iron for the Southern Ocean. Geology 43, 1003–1006. doi: 10.1130/g36989.1
Lyons, W. B., Welch, K. A., Fountain, A. G., Dana, G. L., Vaughn, B. H., and Mcknight, D. M. (2003). Surface glaciochemistry of Taylor Valley, southern Victoria Land, Antarctica and its relationship to stream chemistry. Hydrol. Process. 17, 115–130. doi: 10.1002/hyp.1205
Matsuoka, K., Skoglund, A., and Roth, G. (2018). Quantarctica. Tromsø, NO: Norwegain Polar Institute.
McKnight, D., Runkel, R., Tate, C., Duff, J., and Moorhead, D. (2004). Inorganic N and P dynamics of Antarctic glacial meltwater streams as controlled by hyporheic exchange and benthic autotrophic communities. J. North Am. Benthol. Soc. 23, 171–188. doi: 10.1899/0887-3593(2004)023<0171:inapdo>2.0.co;2
McKnight, D. M., Niyogi, D. K., Alger, A. S., Bomblies, A., Conovitz, P. A., and Tate, C. M. (1999). Dry valley streams in antarctica: ecosystems waiting for water. Bioscience 49, 985–995.
Millero, F. J., Feistel, R., Wright, D. G., and Mcdougall, T. J. (2008). The composition of standard seawater and the definition of the Reference-composition salinity scale. Deep Sea Res. Part I Oceanogr. Res. Pap. 55, 50–72. doi: 10.1016/j.dsr.2007.10.001
Monien, D., Monien, P., Brünjes, R., Widmer, T., Kappenberg, A., Silva Busso, A. A., et al. (2017). Meltwater as a source of potentially bioavailable iron to Antarctica waters. Antarct. Sci. 29, 277–291. doi: 10.1017/s095410201600064x
Olund, S., Lyons, W. B., Welch, S. A., and Welch, K. A. (2018). Fe and nutrients in coastal antarctic streams: implications for primary production in the ross Sea. JGR Sci. 123, 3507–3522. doi: 10.1029/2017jg004352
Opfergelt, S., Burton, K. W., Pogge Von Strandmann, P. A. E., Gislason, S. R., and Halliday, A. N. (2013). Riverine silicon isotope variations in glaciated basaltic terrains: implications for the Si delivery to the ocean over glacial-interglacial intervals. Earth Planet. Sci. Lett. 369–370, 211–219. doi: 10.1016/j.epsl.2013.03.025
Pokrovsky, O. S., Reynolds, B. C., Prokushkin, A. S., Schott, J., and Viers, J. (2013). Silicon isotope variations in Central Siberian rivers during basalt weathering in permafrost-dominated larch forests. Chem. Geol. 355, 103–116. doi: 10.1016/j.chemgeo.2013.07.016
Reynolds, B. C., Aggarwal, J., Andre, L., Baxter, D., Beucher, C., Brzezinski, M. A., et al. (2007). An inter-laboratory comparison of Si isotope reference materials. J. Analyt.cal Atomic Spectrom. 22, 561–568. doi: 10.1039/b616755a
Rückamp, M., Braun, M., Suckro, S., and Blindow, N. (2011). Observed glacial changes on the King George Island ice cap, Antarctica, in the last decade. Glob. Planet. Chang. 79, 99–109. doi: 10.1016/j.gloplacha.2011.06.009
Savage, P. S., Georg, R. B., Armytage, R. M. G., Williams, H. M., and Halliday, A. N. (2010). Silicon isotope homogeneity in the mantle. Earth Planet. Sci. Lett. 295, 139–146. doi: 10.1016/j.epsl.2010.03.035
Schloss, I. R., Ferreyra, G. A., Mercuri, G., and Kowalke, J. (1999). Particle flux in an Antarctic shallow coastal environment: a sediment trap study. Sci. Mar. 63, 99–111. doi: 10.3989/scimar.1999.63s199
Schloss, I. R., Wasilowska, A., Dumont, D., Almandoz, G. O., Hernando, M. P., Michaud-Tremblay, C.-A., et al. (2014). On the phytoplankton bloom in coastal waters of southern King George Island (Antarctica) in January 2010: an exceptional feature? Limnol. Oceanogr. 59, 195–210. doi: 10.4319/lo.2014.59.1.0195
Sherrell, R. M., Annett, A. L., Fitzsimmons, J. N., Roccanova, V. J., and Meredith, M. P. (2018). A “shallow bathtub ring” of local sedimentary iron input maintains the palmer deep biological hotspot on the West Antarctic Peninsula shelf. Sci. Mar. 376:20170171. doi: 10.1098/rsta.2017.0171
Silva-Busso, A. (2009). “Aguas superficiales Y subterràneas en el área Norte da la PenÍnsula Antaràrtica,” in El agua en el Norte de la Península Antártica. INA, DNA-IAA, Fundaci n de Historia Natural F lix de Azara Cap. 4 ed. A. Silva-Busso. Buenos Aires. 47–82.
Silva-Busso, A., Fresina, M., and Vodopivez, C. (2004). Congress Xxxiii Aih - Ahsud Groundwater Flow Understanding. Hidrodinámica e Hidroquímica Subterránea En La Cuenca Del Arroyo Matías, Península Potter, Islas Shetland Antártic. Zacatecas: Asociación Geológica Española.
Stallard, R. F., and Edmond, J. M. (1981). Geochemistry of the amazon: 1. precipitation chemistry and the marine contribution to the dissolved load at the time of peak discharge. J. Geophys. Res. Oceans 86, 9844–9858.
Sun, X., Mörth, C.-M., Porcelli, D., Kutscher, L., Hirst, C., Murphy, M. J., et al. (2018). Stable silicon isotopic compositions of the Lena River and its tributaries: implications for silicon delivery to the Arctic Ocean. Geochim. Cosmochim. Acta 241, 120–133. doi: 10.1016/j.gca.2018.08.044
Sutton, J. N., André, L., Cardinal, D., Conley, D. J., De Souza, G. F., Dean, J., et al. (2018). A review of the stable isotope bio-geochemistry of the global silicon cycle and its associated trace elements. Front. Earth Sci. 5:112. doi: 10.3389/feart.2017.00112
Turner, J., Colwell, S. R., Marshall, G. J., Lachlan-Cope, T. A., Carleton, A. M., Jones, P. D., et al. (2005). Antarctic climate change during the last 50 years. Intern. J. Climatol. 25, 279–294. doi: 10.1002/joc.1130
Urra, A., Wadham, J., Hawkings, J., Telling, J., Hatton, J., Hasholt, B., et al. (2019). Weathering dynamics under contrasting greenland ice sheet catchments. Front. Earth Sci. 7:299. doi: 10.3389/feart.2019.00299
Vinocur, A., and Pizarro, H. (2000). Microbial mats of twenty-six lakes from potter Peninsula, King George Island, Antarctica. Hydrobiologia 437, 171–185.
Wadham, J. L., Tranter, M., Skidmore, M., Hodson, A. J., Priscu, J., Lyons, W. B., et al. (2010). Biogeochemical weathering under ice: size matters. Glob. Biogeochem. Cycles 24:3688.
Waller, R. I. (2001). The influence of basal processes on the dynamic behaviour of cold-based glaciers. Q. Intern. 86, 117–128. doi: 10.1016/s1040-6182(01)00054-4
Welch, K. A., Lyons, W. B., Whisner, C., Gardner, C. B., Gooseff, M. N., Mcknight, D. M., et al. (2010). Spatial variations in the geochemistry of glacial meltwater streams in the Taylor Valley, Antarctica. Antarct. Sci. 22, 662–672. doi: 10.1017/s0954102010000702
Wlostowski, A. N., Gooseff, M. N., Mcknight, D. M., Jaros, C., and Lyons, W. B. (2016). Patterns of hydrologic connectivity in the McMurdo Dry Valleys, Antarctica: a synthesis of 20years of hydrologic data. Hydrol. Proc. 30, 2958–2975. doi: 10.1002/hyp.10818
Wlostowski, A. N., Gooseff, M. N., Mcknight, D. M., and Lyons, W. B. (2018). Transit times and rapid chemical equilibrium explain chemostasis in glacial meltwater streams in the McMurdo Dry Valleys, Antarctica. Hydrol. Proc. 13:322.
Ye, L., Zhang, R., Sun, Q., Jin, J., and Zhang, J. (2018). Hydrochemistry of the meltwater streams on Fildes Peninsula, King George Island, Antarctica. J. Sci. Limnol. 36, 2181–2193. doi: 10.1007/s00343-019-7193-2
Keywords: silicon isotope geochemistry, Antarctica, stream weathering, subglacial weathering, silicon cycle
Citation: Hatton JE, Hendry KR, Hirst C, Opfergelt S, Henkel S, Silva-Busso A, Welch SA, Wadham JL, Lyons WB, Bagshaw E, Staubwasser M and McKnight DM (2020) Silicon Isotopic Composition of Dry and Wet-Based Glaciers in Antarctica. Front. Earth Sci. 8:286. doi: 10.3389/feart.2020.00286
Received: 13 March 2020; Accepted: 19 June 2020;
Published: 14 July 2020.
Edited by:
Ramanathan Alagappan, Jawaharlal Nehru University, IndiaReviewed by:
Andrew Jonathan Hodson, The University Centre in Svalbard, NorwayJon Telling, Newcastle University, United Kingdom
Copyright © 2020 Hatton, Hendry, Hirst, Opfergelt, Henkel, Silva-Busso, Welch, Wadham, Lyons, Bagshaw, Staubwasser and McKnight. This is an open-access article distributed under the terms of the Creative Commons Attribution License (CC BY). The use, distribution or reproduction in other forums is permitted, provided the original author(s) and the copyright owner(s) are credited and that the original publication in this journal is cited, in accordance with accepted academic practice. No use, distribution or reproduction is permitted which does not comply with these terms.
*Correspondence: Jade E. Hatton, ai5lLmhhdHRvbkBicmlzdG9sLmFjLnVr