- Natural Sciences Unit, Finnish Museum of Natural History, University of Helsinki, Helsinki, Finland
The Earth’s early basaltic crust converted episodically into felsic TTG (tonalite-trondhjemite-granodiorite) crust by unknown tectonic processes. To contribute to the debate on the possible tectonic settings of TTGs, this article illustrates and explains migmatite structures of Meso- to Neoarchean TTG-amphibolite terrains in Arctic Fennoscandia. The Lake Inari and Rommaeno complexes in northern Finland and West Troms Complex in northern Norway consist of folded and banded TTG gneisses with abundant amphibolite enclaves. The terrains show migmatite structures generated by in situ and in-source melting of amphibolites and repeated metatexite-diatexite transitions that form infinite and boundless interconnected networks over vast areas. The aim of this article is to show that the TTGs of these terrains represent coalesced in situ and in-source neosomes of amphibolite protoliths and are not similar to granitoids sensu stricto generated by modern-style plate tectonics. The structures of the TTG-amphibolite associations of Arctic Fennoscandia suggest intracrustal differentiation by syn-anatectic partial melting of amphibolites in deep parts of a thick mafic crust.
Introduction
In the Archean, the Earth’s early basaltic crust was episodically converted into a thickening continental tonalite-trondhjemite-granodiorite (TTG) crust. Despite of many decades of TTG investigations, the researchers have not yet agreed upon the tectonics behind the conversion, and several scenarios such as stagnant lid, plume, and arc tectonics are under debate (Nagel et al., 2012; Johnson et al., 2014, 2017; Palin et al., 2016; Hawkesworth et al., 2017; Bédard, 2018). A dear child has many names: TTGs, sometimes with abundant amphibolite enclaves, are termed as “gray gneisses,” “banded gneisses,” TTG migmatites, TTG gneisses, TTG suites, TTG plutons, or just TTGs. Nevertheless, what is the real essence of these rocks that form the majority of the Earth’s earliest crust?
Sodic and often migmatitic TTGs show strikingly different appearance and geochemical features from the diversified, potassic multi-source granitoid batholiths that predominate the present-day continents. According to previous research, the formation of continents started in early Archean, at 4.0 Ga, by conversion of Earth’s early oceanic basalts into felsic TTG crust, a process that continued almost throughout the entire Archean, mainly up to 2.7 Ga (see review of Halla, 2018 and references therein). The Middle and Late Archean brought a weighty novelty in the geochemistry of felsic igneous rocks: the emergence of multi-source high-K calc-alkaline granitoid batholiths at 3.0–2.5 Ga, the origin of which has been related to crust–mantle interactions at the onset of modern-type plate tectonics (Laurent et al., 2014; Halla et al., 2017; Joshi et al., 2017).
One view has been that the TTGs are intrusive granitoids formed by partial melting of mafic oceanic or lower crust at different depths, subsequent melt migration, and magma emplacement into upper crust, i.e., they have been regarded as granitoid intrusions whose formation mechanisms were more or less like in the present subduction environments. Later polymetamorphism has provided explanation for the deformed and migmatized appearance of TTG gneisses. However, the appearance and geochemical compositions of TTG-amphibolite terrains are very different from those of the modern granitoids, even deformed and metamorphosed ones. Instead of clear pluton emplacement structures, the TTG-amphibolite terrains of Arctic Fennoscandia show field evidence for syn-anatectic in situ and in source melting, metatexite and diatexite transitions, and melt migration pathways. There are only minor local intrusion structures within the terrains.
This article emphasizes the importance of understanding the essence of such a heterogeneous system that the TTG-amphibolite terrains represent as well as the implications of their complexity to geochemical interpretations. The amphibolites often form an essential part of the TTG crust and thus are important factors in understanding the formation of the early crust. To throw light on the petrogenesis of the TTG-amphibolite associations, this work presents field observations from Meso- to Neoarchean TTG-amphibolite complexes in Arctic Fennoscandia.
The aim of this article is to show that closed-system in situ and in-source melting of amphibolites may have led to the formation of the migmatite structures of these terrains, without contributions from significant amounts of magmas from other reservoirs. In the case of in situ melting, the melt remains at the site of formation, whereas during in-source melting the melt migrates away from the formation site but remains in the source layer. When the melt migrates slightly farther away from the source area, it generates leucocratic veins or dikes. Granite dikes, sills, or plutons form when the melt migrates completely out of its source region and inject rocks that are not genetically related.
Archean Ttg-Amphibolite Terrains of Arctic Fennoscandia
Large areas of alternating amphibolites and TTGs occur in the northern Fennoscandia in structurally analogous Meso- to Neoarchean migmatite terrains that outcrop sporadically from northern Norway and Finland to the Russian border and beyond (Figure 1). The Neoarchean Lake Inari TTG-amphibolite complex (2.8 Ga) of the Kola Province is located in Inari municipality and extends across the border to Russia. FIRE seismic reflection line (OpenFIRE website) transects the northern shore of the lake. On the northern part of the Lake Inari, there are many small, flat islands consisting solely of amphibolite as well as abundant amphibolite rafts on island shorelines.
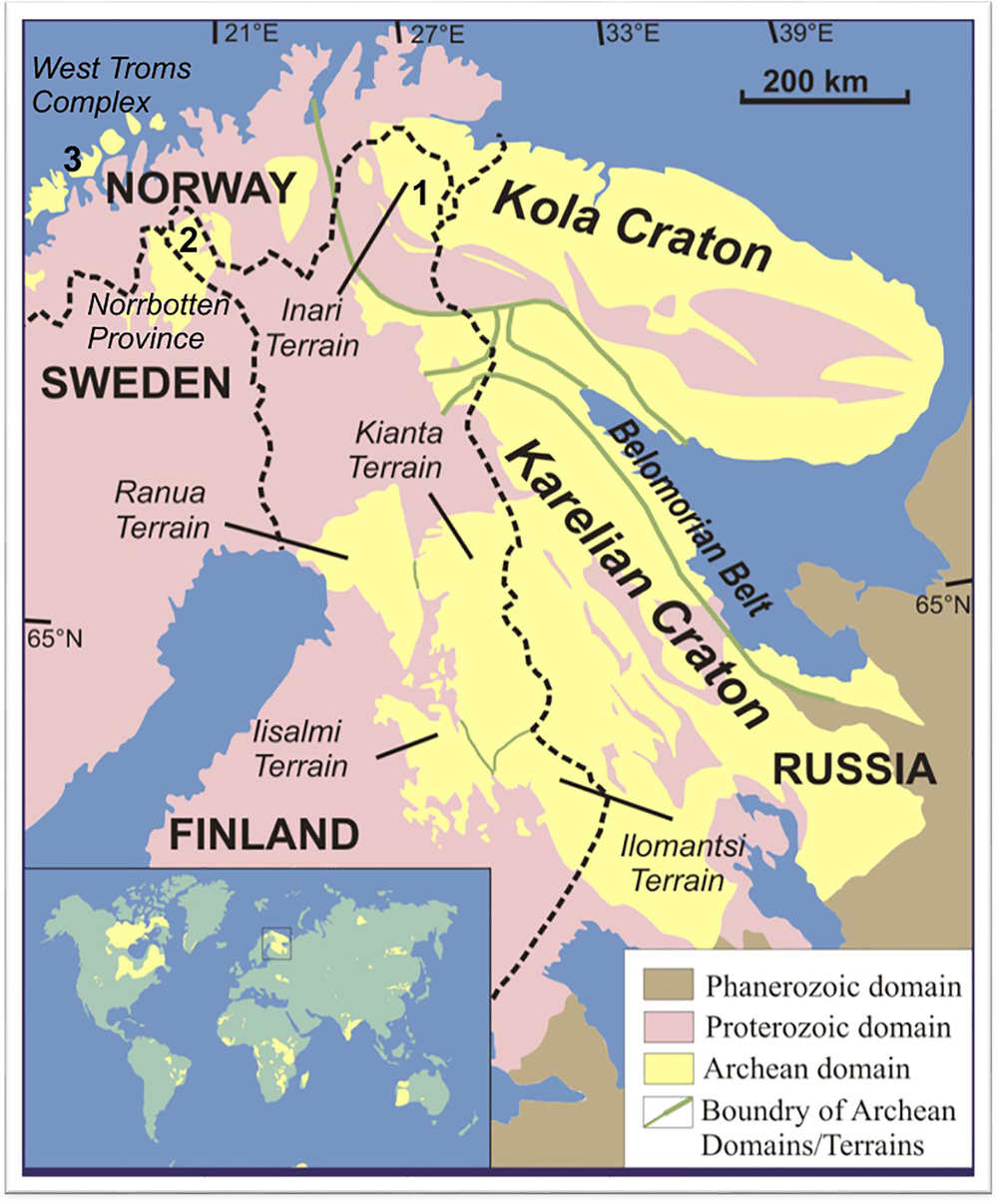
Figure 1. Simplified map of the Fennoscandian Shield showing the locations of the TTG-amphibolite terrains of Arctic Fennoscandia. (1) Lake Inari Complex of the Kola Province, (2) Rommaeno Complex of the Norrbotten Province, and (3) the West Troms Complex (West Troms Basement Complex).
The Mesoarchean Rommaeno TTG-amphibolite complex (3.2 Ga) of the Norrbotten Province is located in Enontekiö municipality, in the Käsivarsi area in Finnish Lapland, and outcrops in Sweden and Norway (Karinen et al., 2015). These mostly unexplored areas are located in the logistically challenging remote wilderness of Finnish Lapland; therefore, detailed descriptions of the total extensions of the terrains will remain to the future.
The 3.0–2.8 Ga West Troms Complex is located in NW Norway (for further details, see Laurent et al., 2019). Another structurally analogous terrain in northern Europe is the Lewisian complex (3.0–2.8 Ga) of the North Atlantic Craton in Outer Hebrides of NW Scotland (Johnson et al., 2013). All these terrains consist of migmatitic, folded, and banded TTG gneisses and abundant layered or dike-like amphibolite rafts and enclaves. Since the complexes in Finland and Norway fall in three different provinces and are located above the Arctic Circle, they are collected under the term Arctic Fennoscandian TTG-amphibolite terrains.
Structures
This article uses the migmatite terminology to describe the TTG-amphibolite terrains in Arctic Fennoscandia. The terminology and interpretations of the migmatite structures of the terrains follow the outlines of Sawyer (1998, 2008), White et al. (2005); Weinberg and Mark (2008), Pawley et al. (2015), and Maxeiner et al. (2017).
Migmatites (Sederholm, 1907) are component rocks that consist of paleosome—the pre-existing rock—and neosome, a new component crystallized from partial melts of the original protolith. Melt proportion divides migmatites into metatexites and diatexites. Low-fraction melts form metatexites and high-fraction melts diatexites (Brown, 1973). Migmatites can take many forms depending on the pressure, which further divides them into several morphological types in terms of melt fraction and syn-anatectic strain (Figure 2). Figures 3–13 present typical morphologies of amphibolite metatexites and diatexites and their transition zones at different melt fraction and strain regimes from the TTG-amphibolite terrains of Arctic Fennoscandia and the comparable Lewisian complex. The formation of migmatites may occur at different combinations of melt fraction and strain, i.e., at different melt fraction-strain regimes.
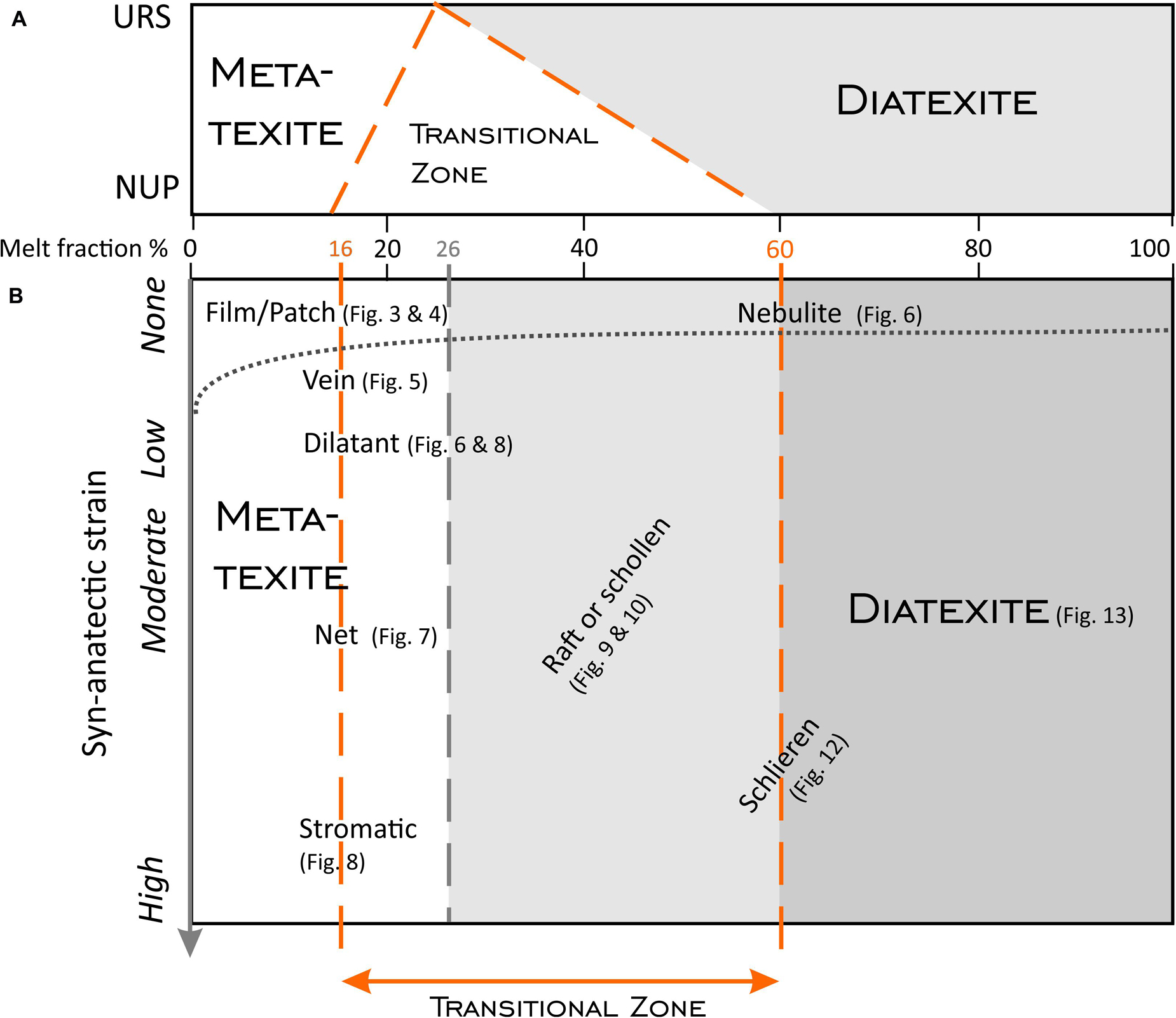
Figure 2. Morphological division of migmatites. (A) Twofold division of migmatites into metatexites and diatexites based on melt fraction percentage and properties of solid grains (URS, uniform rigid spheres, NUP, non-uniform particles) in a partially melted rock. (B) Further division of the migmatite morphology by melt fraction and syn-anatectic strain. The gray dashed vertical line indicates an abrupt transition at 26% melt fraction (URS model) and orange dashed vertical lines indicate the transitional zone between 16 and 60% melt fraction (NUP model). Modified from Sawyer (2008).
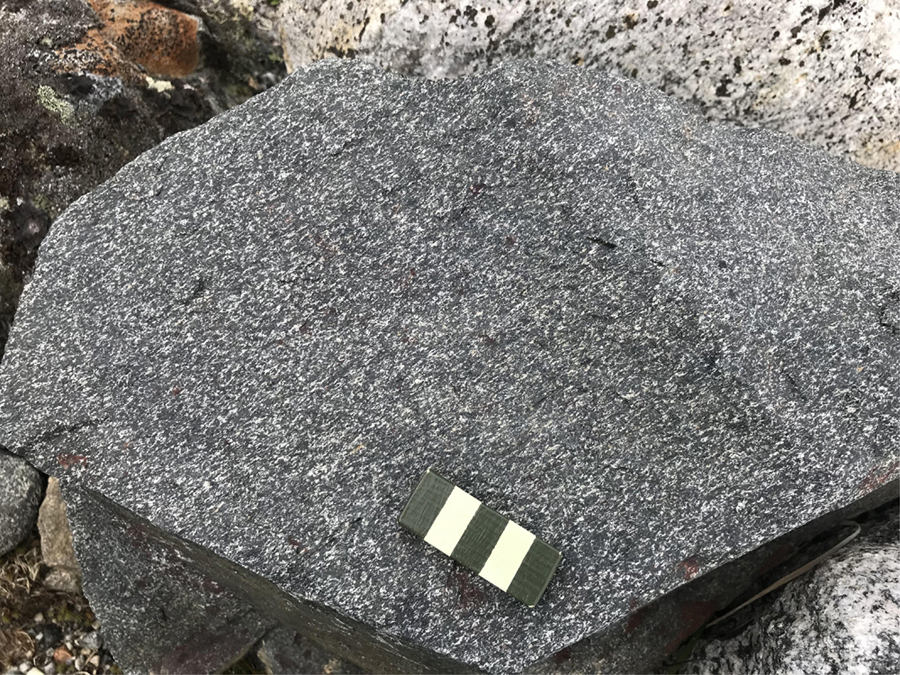
Figure 3. “Film” metatexite, Lammasoaivi, NW Finland, Rommaeno Complex, Norrbotten Province. Fine-grained, weathered quartz-feldspar films of crystallized melt indicate the onset of very low-degree partial melting of amphibolite at low-strain conditions. Length of scale 5 cm.
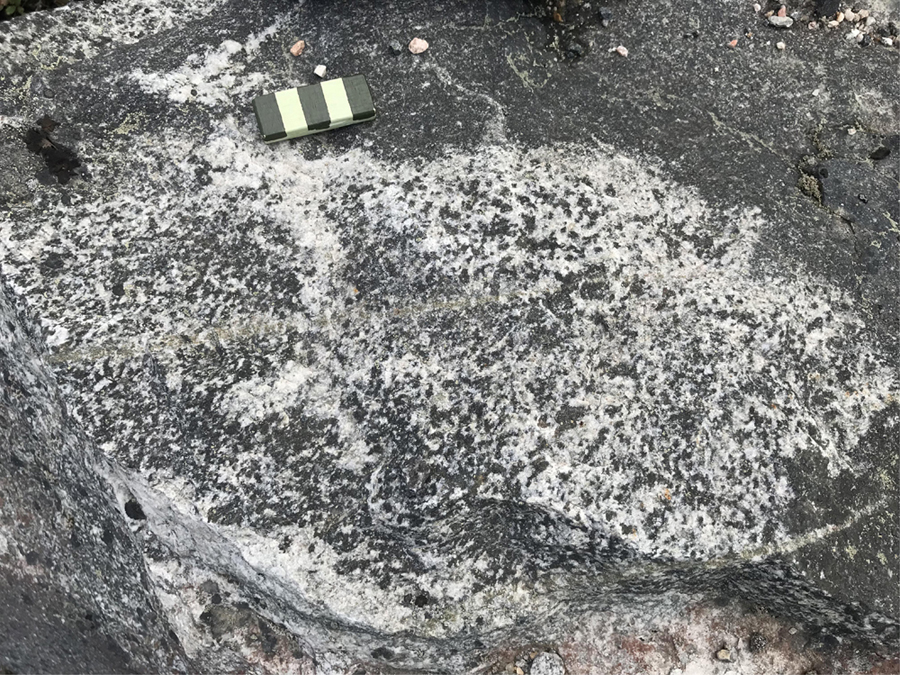
Figure 4. Patch metatexite, Kilpisjärvi, NW Finland, Rommaeno Complex, Norrbotten Province. Neosome occurs as discrete coarse-grained, unsegregated patches representing slightly higher degrees of melting at low syn-anatectic strain. Length of scale 5 cm.
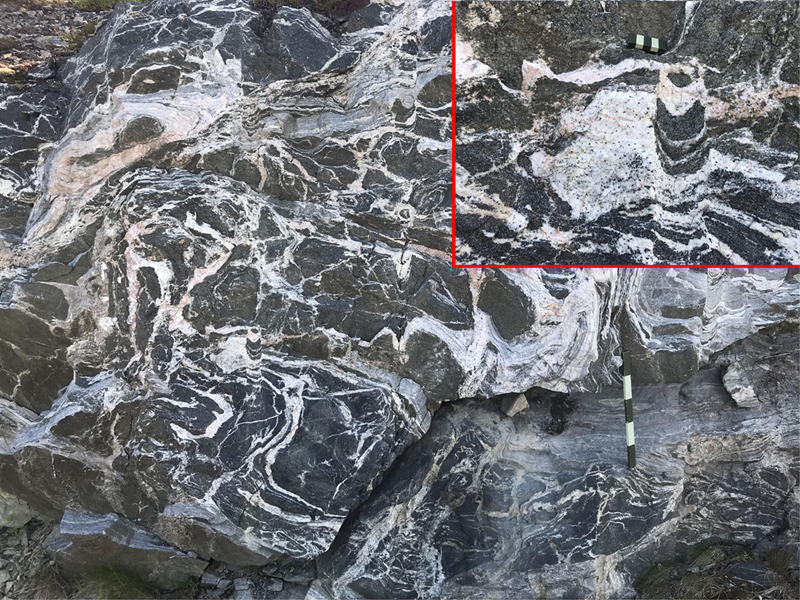
Figure 5. Vein metatexite Kvaløya, northern Norway, West Troms Basement Complex. Discordant vein-like and patchy leucosomes and dikelets form an interconnected network for melt migration. Length of scale 50 cm. Inlet: peritectic hornblende formed by the melt-producing reaction occur as small black grains in leucosome. Length of scale 5 cm.
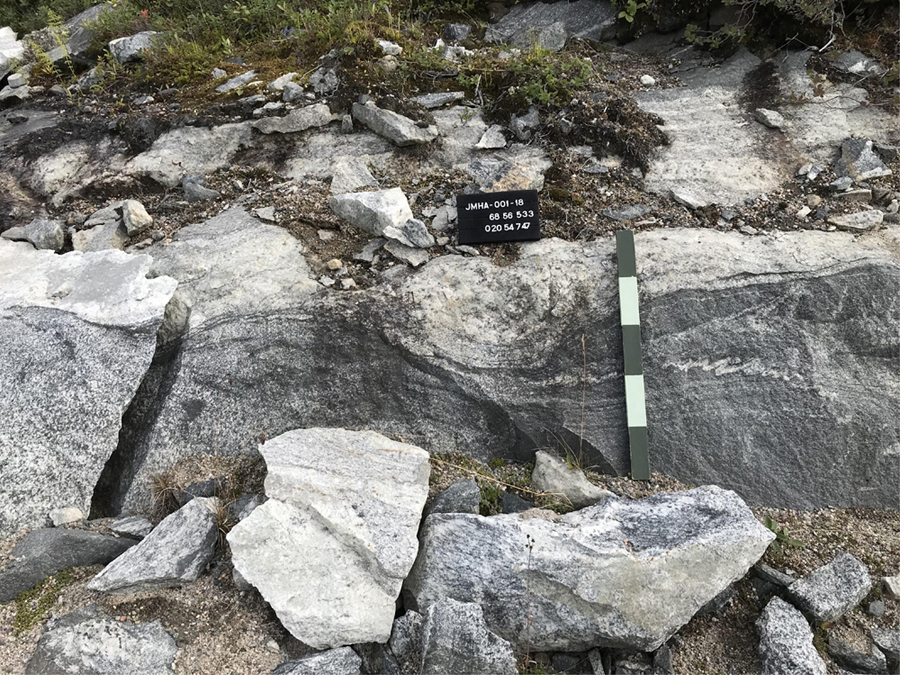
Figure 6. Transition from amphibolite (right boudin) to nebulite (left boudin) in a coherent boudinage structure. Laassaniemi, NW Finland, Rommaeno Complex, Norrbotten Province. Length of scale 50 cm.
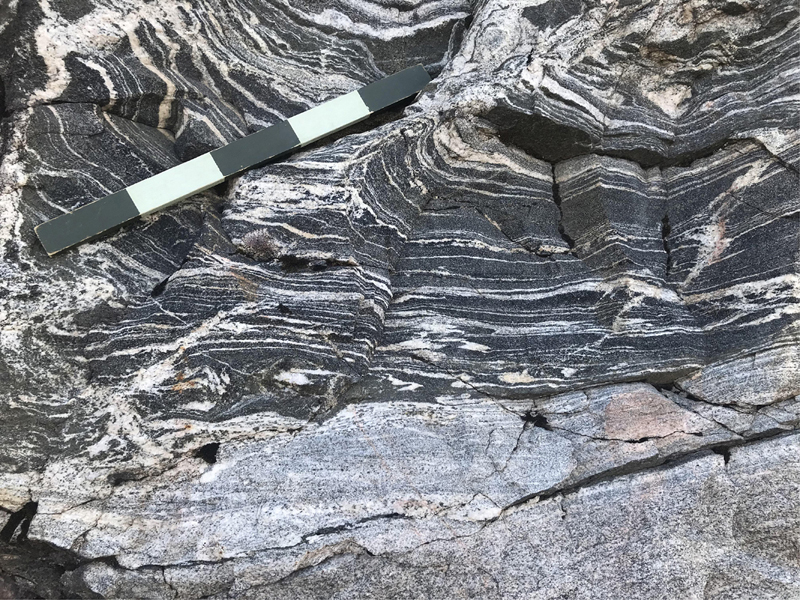
Figure 7. Net-structured metatexite (upper part of the photo), Kvaløya, northern Norway, West Troms Basement Complex (same outcrop as in Figure 5). Layer-parallel leucosomes connecting to discordant veinlets indicate melting during folding and represent the remains of outward melt channels. The lack of melanocratic borders in crosscutting leucosomes suggest that they did not attract melt from their hosts but are conduits for the migration of melt out of the source region. Length of scale 50 cm.
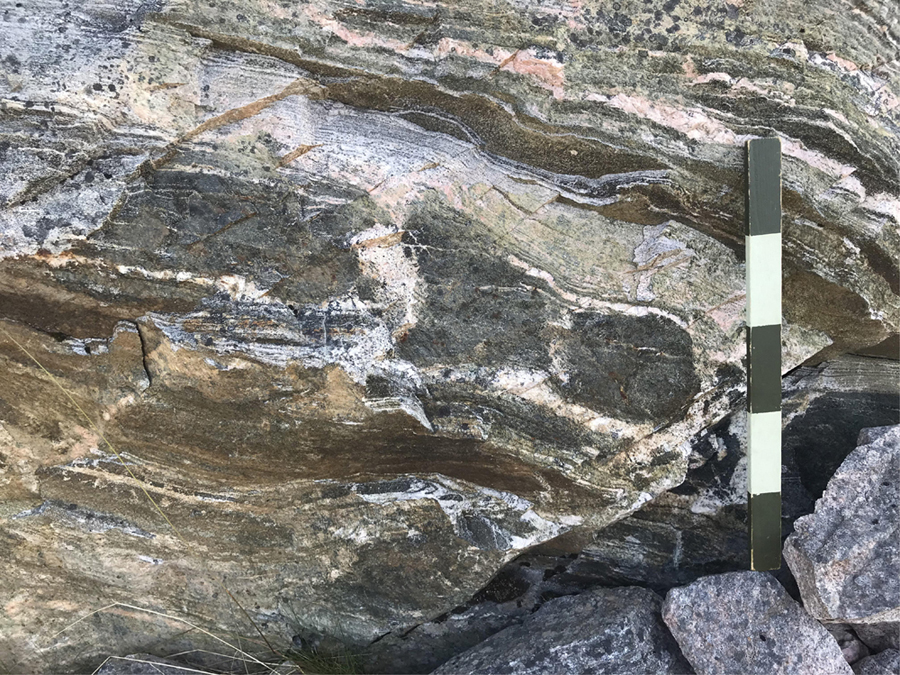
Figure 8. Dilation structures and layered (stromatic) metatexite, Kvaløya, northern Norway, West Troms Basement Complex. Length of scale 50 cm.
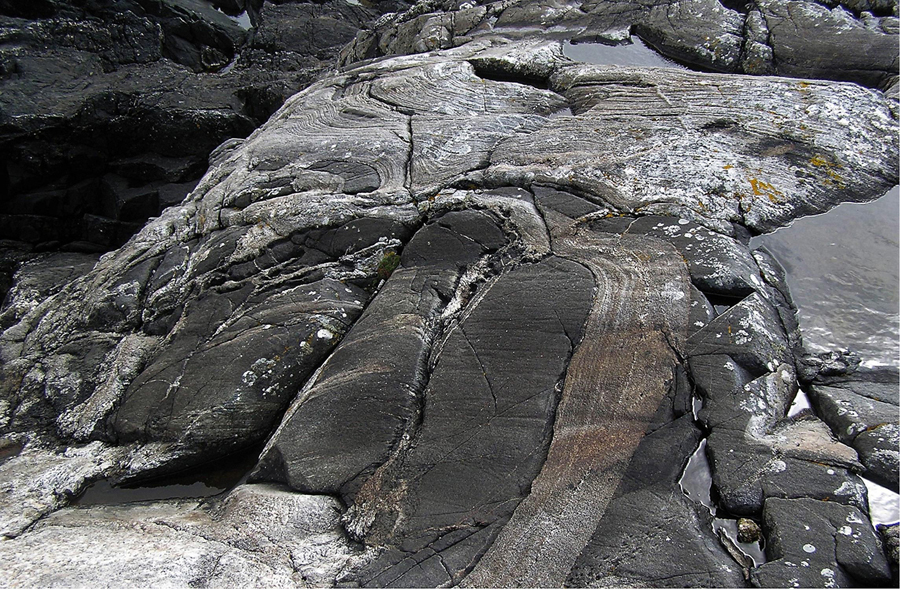
Figure 9. Raft diatexite, Lewisian Complex of the North Atlantic Craton, Outer Hebrides, NW Scotland. At the start of the metatexite-diatexite transition, leuco- or mesocratic flow bands of diatexite disaggregates the original rock and isolates meter-scale blocks of amphibolite that often show rounded ends. Smaller rafts (schollen) are visible on the left. Photo: Pekka Kivimäki.
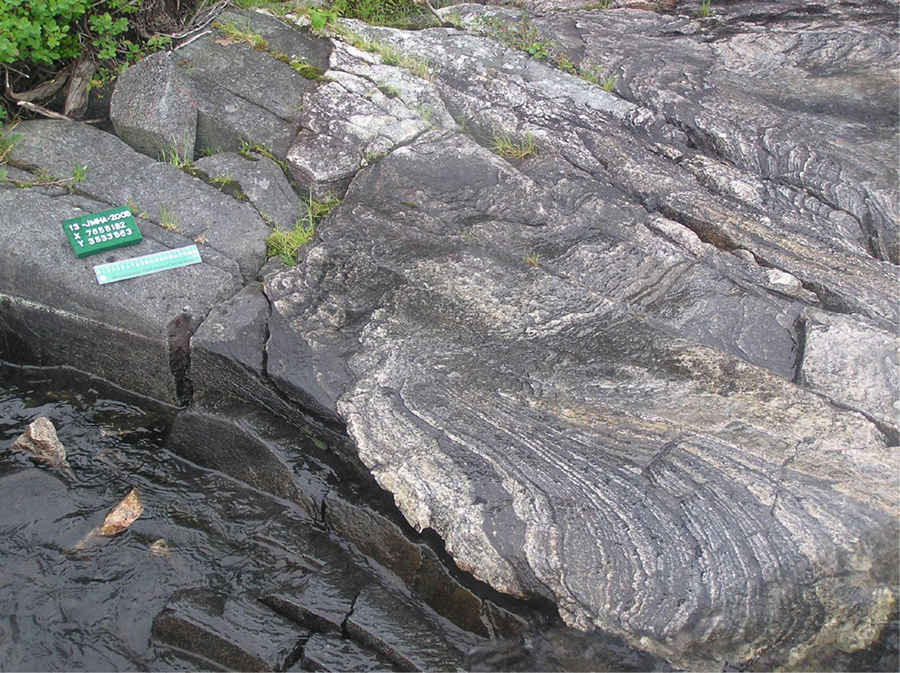
Figure 10. Weathering enhances the appearance of a flow band wrapping around the amphibolite raft. Lake Inari, Kola Province, NE Finland. Length of scale 20 cm. Photo: Pekka Kivimäki.
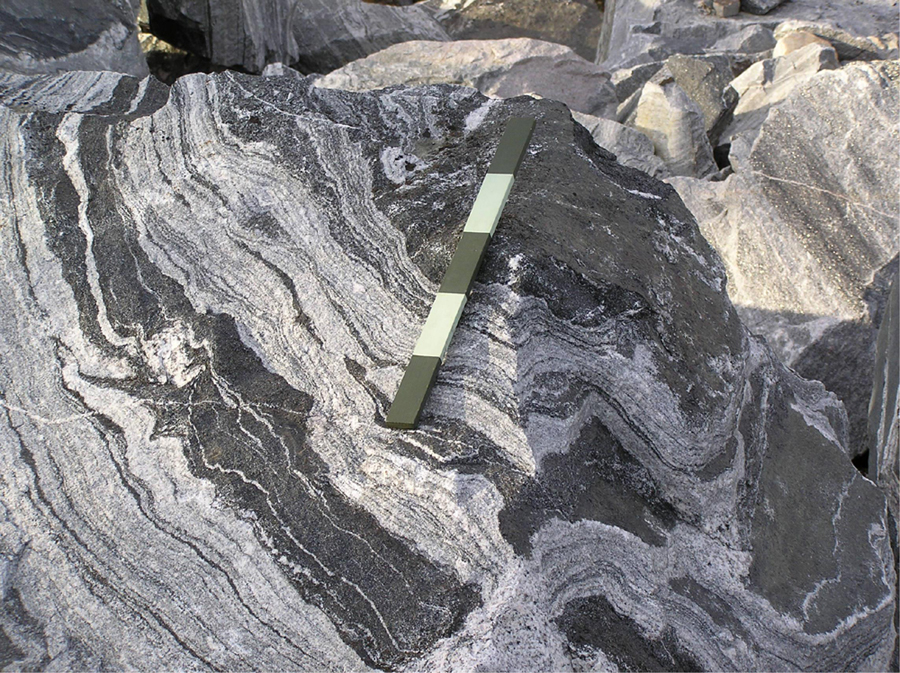
Figure 11. Folded raft diatexite, Näätämö, Lake Inari Complex of the Kola Craton, NE Finland. Deformation and folding separates and stretches rafts of amphibolite. Photo: Pekka Kivimäki.
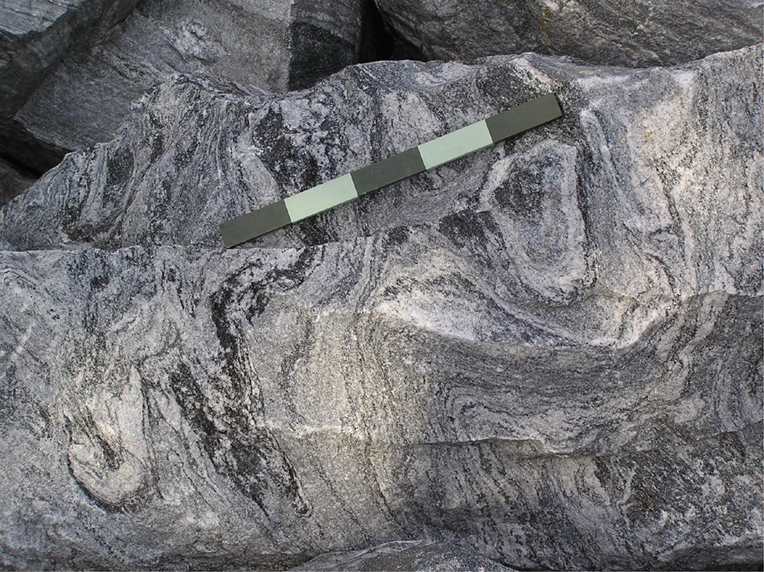
Figure 12. Schlieren diatexite, Näätämö, Lake Inari Complex of the Kola Craton, NE Finland. Same outcrop as in Figure 11. Length of scale 50 cm. Photo: Pekka Kivimäki.
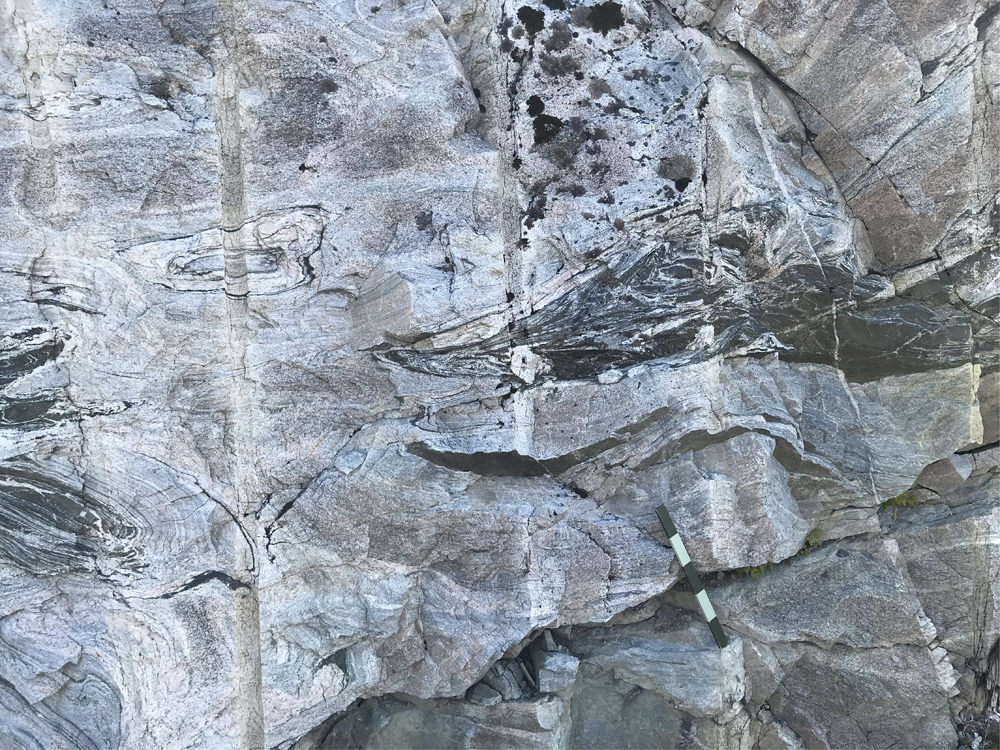
Figure 13. Vestiges of metatexite in diatexite, Kvaløya, northern Norway, West Troms Basement Complex (same outcrop as in Figure 5). Note the almost completely melted small raft on the left. Length of scale 50 cm.
Low-Melt Fraction Metatexites
Low-Strain Regime
An amphibolite metatexite forms when a mafic metaigneous rock starts to melt in situ at the site and crystallizes into a neosome, with such a low melt fraction that the rock retains its structural coherence and, at least some, original features. According to a model presented in Figure 2, there is a transitional zone between metatexite and diatexite migmatites that may possibly extend from 16 to 60% melt fraction depending on the properties of grains.
An amphibolite sample in Figure 3 shows the first signs of the onset of melting in the low-melt fraction and low-strain regime. Fine-grained quartz-feldspar aggregates along the grain boundaries have crystallized from very low melt fractions and formed a “film” metatexite. Slightly higher fractions of melt generated patch metatexites with scattered and generally more or less rounded neosome patches with diffuse boundaries, as shown in Figure 4. In a neosome patch, the leucosome and melanosome have not separated, and the neosome is commonly mesocratic. Advancing incipient in situ melting of amphibolites produces small, widely scattered diffuse patches, veinlets, and networks of thin veins or streaks of leucosome, as in the vein metatexite in Figure 5. A typical product of water-present amphibolite melting is a leucocratic rock with peritectic hornblende, a solid-state mineral formed by a melt-producing reaction (Figure 5, inlet). In the field, peritectic hornblende grains are helpful in recognizing partial melting in deformed rocks.
Film and patch metatexite amphibolites are indicators of incipient in situ melting at low syn-anatectic strain. Nebulitic metatexites or diatexites form when melt fraction increases in low-strain conditions generating an unsegregated, homogeneous portion of the rock showing a spotty or patchy appearance (Figure 6).
Increasing-Strain Regime
Increasing syn-anatectic strain causes the melt fraction to migrate to dilatant structures in the migmatite creating a morphology termed dilation-structured metatexite. In this structure, leucosomes are located in low-pressure (dilatant) structural sites such as spaces between boudins, in pressure shadows, or fractures (Figure 6). It is an important point to note that the grain size depends on the pressure; thick leucosomes located in pressure shadows and interboudin partitions are coarse-grained and homogeneous (e.g., Sawyer, 2008, p. 82). In net-structured metatexites, leucosomes occur as systematic sets building a net-like pattern, which is commonly a result of extensional shear (Figure 7). The highest strain produces layered (stromatic) metatexites (Figure 8) with layer-parallel leucosomes.
Metatexite-Diatexite Transition in Increasing-Melt Fraction and High-Strain Regime
Diatexite forms when a large proportion of a rock melts. According to models presented in Figure 2, the metatexite-diatexite transition may occur abruptly at melt fraction of 16% (URS, uniform rigid spheres model) or transitionally between 26 to 60% (NUP, non-uniform particles model) and at wide ranges of temperatures and pressures, especially if external water is present (e.g., Weinberg and Hasalová, 2015).
The diatexite melts can crystallize at the site, or migrate and inject into other rocks in the source area and form in-source leucosomes. In both cases, structural cohesion of the rock is largely lost. The metatexite-diatexite transition, which can be abrupt or gradational, initiates when increasing amounts of melts start to break up the amphibolites. The original amphibolite gradually disrupts into isolated rafts by increasing fractions of neosome (Figure 9), which can be either homogeneous and coarse-grained or foliated and fine-grained, depending on the strain. A rock containing a high melt fraction is very weak. Due to shear stresses, magma flows easily and a foliation develops generating flow bands around the rafts (Figure 10). In the metatexite-diatexite transition zone, the size and number of amphibolite rafts decrease toward the diatexite zone. The rafts become more rounded, rotated, and dispersed “schollen” within the neosome. Magma migration can assist the folding and stretching of metatexites and create disharmonic folds and truncated layering (Figure 11).
With increasing strain, a flowing diatexite melt tears off schlieren (streaks or swirls) from the disaggregating protolith (Figure 12). Schlieren diatexite zones approaching the transition to massive diatexites are texturally very complex, because also the amphibolite rafts start to melt and fuse into the diatexite (Figure 13). In other words, massive diatexites are a mixture of both in situ metatexite melts and migrated diatexite melts, which, of course, has strong implications to geochemical sampling and interpretations.
Diatexites
With increasing amounts of melting, diatexites that are texturally more homogeneous start to form. They have a massive appearance with more or less abundant vestiges of disrupted amphibolite metatexites showing further melting (Figure 13). Amphibolite metatexites digested by diatexite melts may have an aureole of in situ leucosome. In massive diatexites, in situ melts from metatexites and diatexite melts that migrated from elsewhere have mixed in a complex way. The most advanced stage of migmatization forms large volumes of flow-banded diatexite with schlieren and occasional vestiges of amphibolite paleosome, metatexite, and residuum.
Discussion
Metatexite-Diatexite Transitions in the Field
The breakup of amphibolite or metatexite reflects melt fraction, melt migration, and strain and occurs through a certain sequence of metatexite-diatexite transition structures. Firstly, relatively large blocks of the original amphibolite protolith or metatexite start to disaggregate (Figure 9). In the second stage, abundant diminishing blocks of amphibolites and metatexites (Figure 9, smaller rafts on the left side of the large raft) form within leucocratic neosomes showing flow banding (Figure 10). In the third stage, folding (Figure 11), streaking, and swirling of the metatexite-diatexite mixture forms schlieren diatexites (Figure 12). Finally, a massive diatexite matrix forms, showing occasional vestiges of amphibolite paleosome, metatexite, or residuum (Figure 13).
In the TTG-amphibolite terrains, metatexite-diatexite transitions seem to form an endless and boundless interconnected network, in which one transition zone starts when another one ends, in a rough scale of few hundred meters. This indicates that a huge amount of Archean basalts have been converted into TTGs through partial melting of amphibolites. From the complex structures, it is evident that also the geochemistry is complex. The metatexite-diatexite interplay must have a profound effect on the geochemistry of the TTGs, placing critical demands, especially, on the geochemical sampling and description of the samples.
Melting of Amphibolite
Amphibolites can melt either by dehydration (water absent) or water-fluxed (water present) melting. Dehydration melting of amphibolites occur at higher P-T conditions, whereas the presence of water lowers the melting temperature and the melting can occur in a wide range of P-T conditions (Weinberg and Hasalová, 2015 and references therein). Both dehydration and water-fluxed melting reactions can form solid-state peritectic minerals, which are anhydrous (e.g., garnet and pyroxenes) in the former case and commonly hydrous (e.g., amphibole) in the latter. Dehydration melting may produce leucosomes with peritectic anhydrous minerals, sharp boundaries to the host rock, and melanosome rims (melanosome is the solid residual after the extracted melt fraction). Water-fluxed melting reactions may produce hydrous peritectic minerals, diffuse boundaries with host rock, and commonly the melanosome is absent (Weinberg and Hasalová, 2015). The migmatite structures in the Arctic Fennoscandian TTG-amphibolite terrains resemble mainly the latter, because hornblende is the common peritectic mineral in the leucosomes and melanosomes are often absent.
Weinberg and Hasalová (2015) have provided a comprehensive review on water-fluxed melting of continental crust including compilations of P-T conditions and reactions for dehydration and water-fluxed melting. In the field, water-fluxed melting can be recognized by the occurrence of peritectic hornblende (e.g., T 675–850°C, P 4–11 kbar; biotite + plagioclase + quartz + H2O = hornblende ± K-feldspar + melt; according to the compilation by Weinberg and Hasalová, 2015). Amphibolite dehydration melting typically produces anhydrous peritectic minerals such as garnet and pyroxenes (e.g., T 800–900°C, P 9–12 kbar; hornblende + plagioclase + quartz = clinopyroxene + garnet + melt; combined from Nehring et al., 2008 and Zhang et al., 2013).
Migration of anatectic magmas is the main process of crustal differentiation. Melts move along pressure gradients toward lower pressures along local high-strain zones, fracture systems and permeable melt channelways (Weinberg and Mark, 2008). According to Weinberg and Hasalová (2015), water-fluxed melting can produce voluminous melting even at amphibolite facies conditions. Therefore, an attractive hypothesis for the genesis of TTG-amphibolite terrains would be the migration of water-rich fluids and melts from granulite-facies dehydration melting reactions to upper amphibolite facies and subsequent water-fluxed melting of the amphibolites.
Implications to Geochemistry
The most prominent geochemical feature of TTGs is the variation from low Y and HREE to high Y and HREE end-members. The low- and high-HREE TTG end-members of Halla et al. (2009) are equivalent to the low- and high-pressure TTGs of Moyen (2011). Both Y and HREE are garnet-controlled elements; therefore melting of amphibolites in different P-T conditions provides an explanation for the HREE variation in TTGs.
Melts formed by dehydration reactions in granulite facies conditions may migrate along pressure gradients through fractures and shear zones and along permeable melt channelways (Weinberg and Mark, 2008), leaving behind garnet retaining the HREE. Thus, HREE-depleted diatexite melts deriving from granulite facies could carry the low-HREE signature to the upper amphibolite facies. The arriving melts and fluids would trigger water-fluxed melting in the amphibolite facies yielding high-HREE end members, because the garnet is not stable in water-fluxed melting conditions.
Preliminary geochemical results for parallel sets of TTG and amphibolite samples from the Lake Inari Complex confirm the bimodality of the tonalite–basalt association as well as the intermingled combination of low- and high-HREE TTGs. Supplementary Table S1 presents major and trace element data for representative samples from the Lake Inari Complex as well as the Fennoscandian TTG averages from Halla et al. (2009). There are two geochemical end-members of TTGs within the Lake Inari area, one with low HREE and lower Mg content (Supplementary Table S1, Sample JMHA-3.1, Figure 14) and the other with high HREE and elevated Mg content (Supplementary Table S1, Sample JMHA-8.1, Figure 15). Both these types seem to be coeval and intermingling, perhaps because of mixing of local metatexite melts and diatexite melts from garnet-bearing granulite facies layers. The amphibolites are basalts in TAS classifications and show flattish REE patterns.
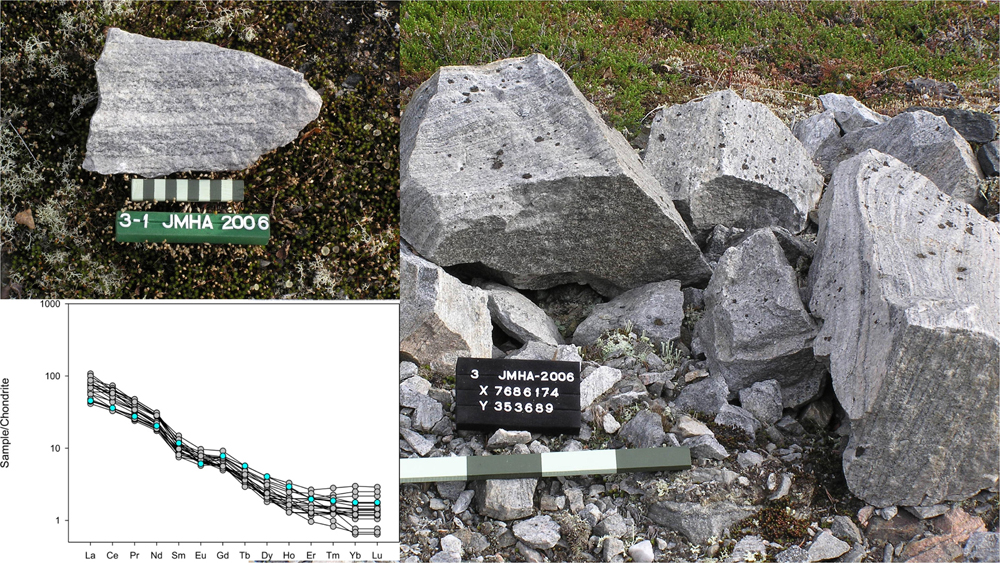
Figure 14. Left: REE patterns of low-HREE TTGs from the Lake Inari Complex (Supplementary Table S1). The pattern indicated by blue symbols is from a diatexite sample found in the same Näätämö area as metatexite sample in Figure 15. Length of scale: hand specimen 10 cm, outcrop 40 cm.
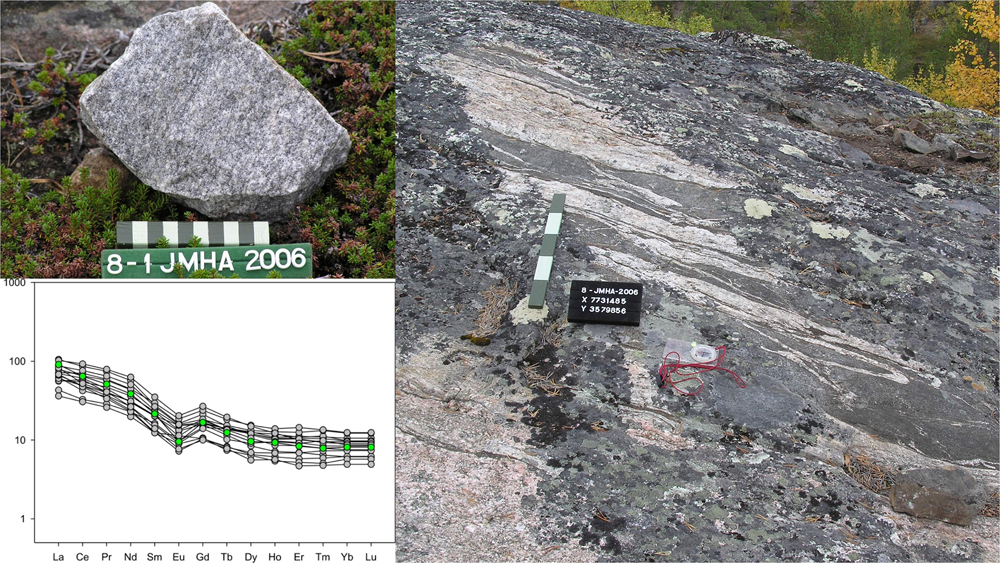
Figure 15. REE patterns of high-HREE TTGs from the Lake Inari Complex (Supplementary Table S1). The pattern indicated by green symbols is from a metatexite sample found in the same Näätämö area as diatexite in Figure 14. Length of scale: hand specimen 10 cm, outcrop 50 cm.
Although large amounts of apparently homogeneous diatexite of TTG composition may resemble granitoids sensu stricto, mixing of metatexite and diatexite melts and contamination by mafic enclaves complicates their geochemistry. Therefore, careful descriptions of samples by using appropriate migmatite terminology are necessary when reporting geochemical and isotope geochemical data. Using diagrams designed for granites generated by modern-type plate tectonics to interpret complex mixtures of diatexites may lead to wrong conclusions.
Massive diatexites are gradational to allochtonous tonalite plutons controlled by melt migration and fractures or folds. During migmatization, an extensive permeable network of melt-bearing channels develop, and melt may accumulate as autochtonous leucocratic bodies or migrate to form allochtonous granitoids. Accumulated metatexite melts produce leucocratic, residuum-free bodies with differentiated geochemical signatures. The diatexite melting produces less differentiated, residuum-rich granites. The TTG-amphibolite terrains of Arctic Fennoscandia commonly include some leucocratic pods by accumulation of low-degree metatexite melts.
Constraints for the Tectonic Setting
TTG-amphibolite terrains of Arctic Fennoscandia represent vast networks of infinite metatexite-diatexite transitions, in which both in situ and in-source melts have mixed. The structures of the felsic parts contain flow bands of melts migrated from nearby (in-source leucosomes), leucosomes in metatexites (in situ leucosomes) and ghost-like remnants of amphibolites that may represent the paleosome, metatexite, or residuum.
Since the melts tend to migrate from high-pressure areas to low-pressure sites, the HREE variation in TTGs may reflect contributions and mixing of melts from both granulite and upper amphibolite facies, for example at the lower part of a thick basaltic crust. Fluids related to the granulite facies dehydration reactions may have triggered extensive melting in the amphibolite facies. The presented migmatite structures show that increasing melt fractions and syn-anatectic deformation have contributed to the formation of the metatexite-diatexite terrains in Arctic Fennoscandia.
It is clear that structures alone cannot distinguish between geodynamic models for the origin of the TTG-amphibolite associations in Arctic Fennoscandia. However, any tectonic model presented should explain why/how (1) the crust thickened enough for granulite facies conditions to exist, (2) the partial melting of the crust started, (3) water entered to trigger amphibolite facies partial melting, and (4) the syn-anatectic deformation occurred.
A comprehensive discussion on subduction versus plateau origin is beyond of the scope of this article and waiting for further results from the Arctic Fennoscandian TTG-amphibolite terrains. However, some recent work such as amphibolite dehydration-melting experiments (Zhang et al., 2013) and phase equilibrium modeling (Palin et al., 2016) indicate that amphibole dehydration melting could have occurred at the base of a 40 km thick oceanic plateau or otherwise overthickened basaltic crust in P-T conditions that would be suitable for the formation of low-HREE TTGs. The delamination of the dense lower crust could have then destroyed the garnet-rich restite of amphibolite melting.
Recently discovered B isotope evidence support a plateau origin over subduction for 3.8–2.8 Ga TTGs (Smit et al., 2019). The results indicate that seawater did not contribute to the mafic source of TTGs. Therefore, it might be possible that the external water derived from granulite-facies dehydration melting reactions triggered the amphibolite-facies water-fluxed melting producing high-HREE TTGs. The TTG-amphibolite terrains may have formed by migmatization in the lower part of a thick basaltic oceanic plateau, but further microstructural, geochemical and geochronological studies of the terrains are needed to sustain the origin of the TTG-amphibolite terrains of Arctic Fennoscandia.
Conclusion
1. The migmatite structures of the Meso- to Neoarchean TTG (tonalite-trondhjemite-granodiorite)-amphibolite terrains of Arctic Fennoscandia form an infinite and boundless network of amphibolite metatexite-diatexite transitions controlled by melt proportions and syn-anatectic strain.
2. The closed system of interconnected metatexite-diatexite transitions have formed by in situ and in-source partial melting of basalts in the lower parts of a thick mafic crust.
3. Heterogeneous mixing of amphibolite-facies water-fluxed melts with dehydration melts migrated from granulite-facies provides a worthwhile hypothesis for the HREE variation in TTGs.
4. Arctic Fennoscandian TTGs represent coalesced in situ and in-source neosomes of amphibolite protoliths generated at closed-system intracrustal settings. They are not similar to granitoids generated by open-system processes at modern-style plate tectonic settings.
5. The structures of the TTG-amphibolite associations of Arctic Fennoscandia suggest intracrustal differentiation by syn-anatectic partial melting of amphibolites in deep parts of a thick mafic crust.
Data Availability Statement
All datasets generated for this study are included in the article/Supplementary Material.
Author Contributions
The author confirms being the sole contributor of this work and has approved it for publication.
Funding
Open access publication fees are paid by the Helsinki University Library.
Conflict of Interest
The author declares that the research was conducted in the absence of any commercial or financial relationships that could be construed as a potential conflict of interest.
Acknowledgments
K.H. Renlund Foundation and the Pentti Tuomikoski Fund are acknowledged for field work and travel support for this project. The Kilpisjärvi Biological Station is thanked for excellent accommodation and care. The helpful and constructive reviews of TN and MZ are highly appreciated.
Supplementary Material
The Supplementary Material for this article can be found online at: https://www.frontiersin.org/articles/10.3389/feart.2020.00252/full#supplementary-material
References
Bédard, J. H. (2018). Stagnant lids and mantle overturns: implications for Archaean tectonics, magma genesis, crustal growth, mantle evolution, and the start or the plate tectonics. Geosci. Front. 9, 19–49. doi: 10.1016/j.gsf.2017.01.005
Halla, J. (2018). Highlights on geochemical changes in archaean granitoids and their implications for early earth geodynamics. Geosciences 8:353. doi: 10.3390/geosciences8090353
Halla, J., van Hunen, J., Heilimo, E., and Hölttä, P. (2009). Geochemical and numerical constraints on Neoarchean plate tectonics. Precamb. Res. 174, 155–162. doi: 10.1016/j.precamres.2009.07.008
Halla, J., Whitehouse, M. J., Ahmad, T., and Bagai, Z. (2017). “Archaean granitoids: an overview and significance from a tectonic perspective,” in Crust–Mantle Interactions and Granitoid Diversification: Insights from Archaean Cratons, eds J. Halla, M. J. Whitehouse, T. Ahmad, and Z. Bagai (London: Geological Society), 1–18. doi: 10.1144/sp449.10
Hawkesworth, C. J., Cawood, P. A., Dhuime, B., and Kemp, T. I. S. (2017). Earth’s continental lithosphere through time. Annu. Rev. Earth Planet. Sci. 45, 169–198. doi: 10.1146/annurev-earth-063016-020525
Johnson, T. E., Brown, M., Gardiner, N. J., Kirkland, C. L., and Smithies, H. (2017). Earth’s first stable continents did not form by subduction. Nature 543, 239–243.
Johnson, T. E., Brown, M., Kaus, B. J. P., and VanTongeren, J. (2014). Delamination and recycling of Archaean crust caused by gravitational instabilities. Nat. Geosci. 7, 47–52. doi: 10.1038/ngeo2019
Johnson, T. E., Fischer, S., and White, R. W. (2013). Field and petrographic evidence for partial melting of TTG gneisses from the central region of the mainland Lewisian complex, NW Scotland. J. Geol. Soc. London 170, 319–326. doi: 10.1144/jgs2012-096
Joshi, K. B., Bhattacharjee, J., Rai, G., Halla, J., Ahmad, T., Kurhila, M., et al. (2017). “The diversification of granitoids and plate tectonic implications at the archaean proterozoic boundary in the bundelkhand craton, Central India,” in Archaean Cratons: Crust–Mantle Interactions and Granitoid Diversi?cation: Insights from Archaean Cratons, eds J. Halla, M. Whitehouse, T. Ahmad, and Z. Bagai (London: Geological Society), 123–157. doi: 10.1144/sp449.8
Karinen, T., Lepistö, S., Konnunaho, J., Lauri, L. S., Manninen, T., and Huhma, H. (2015). Unit Description Report, Enontekiö, Käsivarsi. Finnish: Yksikkökuvausraportti, Enontekiö, Käsivarsi. Rovaniemi: Geological Survey of Finland.
Laurent, O., Martin, H., Moyen, J. F., and Doucelance, R. (2014). The diversity and evolution of late-archean granitoids: evidence for the onset of “modern-style” plate tectonics between 3.0 and 2.5 Ga. Lithos 205, 208–235. doi: 10.1016/j.lithos.2014.06.012
Laurent, O., Vander Auwera, J., Bingen, B., Bolle, O., and Gerdes, A. (2019). Building up the first continents: mesoarchean to paleoproterozoic crustal evolution in West Troms, Norway, inferred from granitoid petrology, geochemistry and zircon U-Pb/Lu-Hf isotopes. Precamb. Res. 321, 303–327. doi: 10.1016/j.precamres.2018.12.020
Maxeiner, R. O., Ashton, K., Card, C. D., Morelli, R. M., and Knox, B. (2017). A Field Guide to Naming Migmatites and Their Textures, with Saskatchewan Examples. Report number: miscellaneous Report 2017-4.2, Affiliation: economy - Government of Saskatchewan. Regina, SK: Saskatchewan Ministry of Economy.
Moyen, J. F. (2011). The composite archaean grey gneisses: petrological significance, and evidence for a non-unique tectonic setting for archaean crustal growth. Lithos 123, 21–36. doi: 10.1016/j.lithos.2010.09.015
Nagel, T. J., Hoffmann, E. J., and Münker, C. (2012). Generation of eoarchean tonalite-trondhjemite-granodiorite series from thickened mafic arc crust. Geology 40, 375–378. doi: 10.1130/g32729.1
Nehring, F., Foley, S. F., Hölttä, P., and van den Kerkhof, A. M. (2008). Internal differentiation of the archean continental crust: fluid-controlled partial melting of granulites and TTG-Amphibolite associations in central finland. J. Petrol. 50, 3–35. doi: 10.1093/petrology/egn070
Palin, R. M., White, R. W., and Green, E. C. R. (2016). Partial melting of metabasic rocks and the generation of tonalitic-trondhjemitic-granodioritic (TTG) crust in the Archaean: constraints from phase equilibrium modelling. Precamb. Res. 287, 73–90. doi: 10.1016/j.precamres.2016.11.001
Pawley, M., Reid, A., Dutch, R., and Preiss, W. (2015). Demystifying migmatites: an introduction for the field-based geologist. Appl. Earth Sci. 124, 147–174. doi: 10.1179/1743275815y.0000000014
Sawyer, E. W. (1998). Formation and evolution of granite magmas during crustal reworking: the significance of diatexites. J. Petrol. 39, 1147–1167. doi: 10.1093/petroj/39.6.1147
Sawyer, E. W. (2008). Atlas of Migmatites, The Canadian Mineralogist, Special Publictation 9. Ottawa, ON: NRC Research Press.
Smit, M. A., Scherstén, A., Næraa, T., Emo, R. B., Scherer, E. E., Sprung, P., et al. (2019). Formation of archean continental crust constrained by boron isotopes. Geochem. Perspect. Lett. 12, 23–26. doi: 10.7185/geochemlet.1930
Weinberg, R. F., and Hasalová, P. (2015). Water-fluxed melting of the continental crust: a review. Lithos 212-215, 158–188. doi: 10.1016/j.lithos.2014.08.021
Weinberg, R. F., and Mark, G. (2008). Magma migration, folding, and disaggregation of migmatites in the Karakoram Shear Zone, Ladakh, NW India. GSA Bull. 120, 994–1009. doi: 10.1130/b26227.1
White, R. W., Pomroy, N. E., and Powell, R. (2005). An in situ metatexite-diatexite transition in upper amphibolite facies rocks from Broken Hill, Australia. J. Metamorph. Geol. 23, 579–602. doi: 10.1111/j.1525-1314.2005.00597.x
Zhang, C., Holtz, F., Koepke, J., Wolff, P. E., Ma, C., and Bédard, J. H. (2013). Constraints from experimental melting of amphibolite on the depth of formation of garnet-rich resites, and implications for models of Early archaean crustal growth. Precamb. Res. 231, 206–217. doi: 10.1016/j.precamres.2013.03.004
Keywords: Archean, TTG, amphibolite, metatexite, diatexite
Citation: Halla J (2020) The TTG-Amphibolite Terrains of Arctic Fennoscandia: Infinite Networks of Amphibolite Metatexite-Diatexite Transitions. Front. Earth Sci. 8:252. doi: 10.3389/feart.2020.00252
Received: 07 March 2020; Accepted: 08 June 2020;
Published: 30 June 2020.
Edited by:
Kristoffer Szilas, University of Copenhagen, DenmarkCopyright © 2020 Halla. This is an open-access article distributed under the terms of the Creative Commons Attribution License (CC BY). The use, distribution or reproduction in other forums is permitted, provided the original author(s) and the copyright owner(s) are credited and that the original publication in this journal is cited, in accordance with accepted academic practice. No use, distribution or reproduction is permitted which does not comply with these terms.
*Correspondence: Jaana Halla, amFhbmEuaGFsbGFAaGVsc2lua2kuZmk=; amFhbmFoYWxsYUBnbWFpbC5jb20=