- 1School of Earth, Atmosphere and Environment, Monash University, Clayton, VIC, Australia
- 2Department of Earth Sciences, Durham University, Durham, United Kingdom
- 3School of Earth and Ocean Sciences, Cardiff University, Cardiff, United Kingdom
The trace element and isotopic compositions of mid-ocean ridge basalts (MORB) provide an important cornerstone for all studies seeking to understand mantle evolution. Globally there is a significant over-enrichment in the incompatible trace element concentrations of MORB relative to levels which should be generated by fractional crystallization. Thermal and geochemical constraints suggest that MORB require generation in open system magma chambers. However, the petrology of lower oceanic crustal rocks suggests instead that these enrichments maybe formed through reactive porous flow (RPF). Stable isotope compositions are process dependent and therefore provide an excellent mechanism to compare these contrasting models. This study presents the first neodymium (Nd) stable isotope compositions of Indian MORB and well characterized gabbroic rocks from the lower oceanic crust sampled at the Southwest Indian Ridge (SWIR) (Hole 735B). Indian MORB is extremely homogenous with a mean δ146Nd of −0.025 ± 0.005‰ which is identical to the composition of Pacific MORB. Despite significant variability in the source composition of MORB globally (i.e., 143Nd/144Nd) their indistinguishable δ146Nd compositions suggests δ146Nd was homogenized through a consistent process (i.e., repeated melt addition in the open-system magma chambers across the global ridge network). In stark contrast, oceanic gabbros have δ146Nd ranging from −0.026 to −0.127‰, doubling the natural variability in Nd stable isotopes observed in terrestrial rocks. Clinopyroxene separates possess variable δ146Nd but are isotopically heavier than the gabbroic whole rocks at the same major element compositions. These large variations in δ146Nd cannot be generated solely by the fractionation or accumulation of magmatic minerals. Hole 735B preserves widespread evidence of RPF which could induce kinetic isotope fractionation during crystal growth. However, the maximum kinetic isotope fractionations that can be generated in clinopyroxene are only ca. 0.02‰, therefore several cycles of dissolution and reprecipitation of isotopic signatures at grain boundaries are required to explain the range of δ146Nd observed in the gabbros. The large disconnect between the average composition of the oceanic crust (δ146Nd = −0.067‰) and MORB, combined with limited evidence of melt extraction to the upper crust at Hole 735B, led to the conclusion that melts involved in RPF have not contributed in a substantial way to the Nd isotope composition of erupted MORB.
Introduction
The oceanic crust covers the majority of Earth’s surface (∼60%) and therefore accurate knowledge of its composition is imperative for constraining the composition of the silicate Earth and the long-term geochemical fluxes in recycling systems. Oceanic crust is produced along the global ocean ridge system which stretches over 60,000 km and produces 75% of Earth’s volcanism (Crisp, 1984). The composition of mid-ocean ridge basalts (MORB), therefore can be used to provide important insights into the composition of Earth’s most extensive crustal reservoir while also placing constraints on the composition of the upper mantle (e.g., Langmuir and Hanson, 1980; Hofmann, 1997; Workman and Hart, 2005; Gale et al., 2013). The magmas parental to these basalts were traditionally considered to have evolved by simple fractional crystallization, producing an oceanic crust consisting of 1–1.5 km of basalt and dolerite underlain by 4–5 km of gabbro (e.g., Dick et al., 1984; Klein, 2003). Although the chemical and isotope compositions of MORB are relatively homogenous compared to other magma types (e.g., ocean island basalts), MORB glasses can display significant elemental variations (White, 1985; Warren et al., 2009; Jenner and O’Neill, 2012). This compositional scatter is the result of the interplay between the contrasting effects of: (i) source composition (Rehkämper and Hofmann, 1997; Nielsen et al., 2018); (ii) differences in the extent of melting reflecting variations in mantle potential temperature (Klein and Langmuir, 1987; McKenzie and Bickle, 1988) or spreading rate (Regelous et al., 2016); and (iii) fractional crystallization processes which can be influenced by both the pressure of crystallization and magma composition (Michael and Cornell, 1998; Herzberg, 2004). However, a significant over-enrichment in the incompatible trace elements concentrations (e.g., Th, La) of magmas is observed compared to that which is predicted by simple fractional crystallization alone (White and Bryan, 1977; Hekinian and Walker, 1987; O’Neill and Jenner, 2012). Two distinct petrogenetic models have been advocated as the cause of these enrichments: (1) differentiation in an open system replenished-tapped-crystallizing (RTX) magma chambers (O’Hara, 1968; O’Neill and Jenner, 2012; Coogan and O’Hara, 2015) and (2) melt rock interaction resulting in the chromatographic separation of elements in the lower crust (Kamenetsky et al., 1998; Lissenberg and Dick, 2008; Lissenberg et al., 2013; Lissenberg and MacLeod, 2016). There is compelling geochemical and geophysical evidence that magma chambers at mid-ocean ridges operate as steady state open systems (Detrick et al., 1987; Johnson and Dick, 1992; Sinton and Detrick, 1992; White, 1993), supportive of an RTX model. Plutonic rocks from the oceanic crust are considered the crystallization products of MORB differentiation (Coogan, 2014), however, whether MORB do in fact represent the erupted complement of the preserved oceanic gabbros remains debatable. At high temperature stable isotope fractionation can provide important insights in the nature and conditions of the processes involved in magma genesis (e.g., Dauphas et al., 2014; Millet et al., 2016; McCoy-West et al., 2018). This study presents high-precision double spike Nd stable isotope data for oceanic gabbros from Hole 735B and MORB from the Indian Ocean to test the various models of MORB genesis.
Materials and Methods
Analyses were performed in the Arthur Holmes Geochemistry Labs at the Durham University. Basaltic glass, clinopyroxene or whole rock powders were weighed out to obtain 200 ng of natural Nd and then spiked with a 145Nd−150Nd double spike (McCoy-West et al., 2017, 2020), and then dissolved using a conventional HF-HNO3 hotplate digestion. Neodymium was separated using well-established chromatographic techniques. Briefly, the rare earth elements (REE) were separated from the sample matrix using BioRad AG50W-x8 cation exchange resin. A second elongated column filled with Eichrom Ln-Spec resin was then used to separate Nd from direct Sm isobaric interferences using dilute HCl. For a more detailed description of the methodology refer to McCoy-West et al. (2017) and McCoy-West et al. (2020).
Neodymium isotope measurements were performed using a Thermo-Fisher Triton Plus thermal ionisation mass spectrometer (TIMS). Neodymium was measured as a metal ion in static collection mode using a double Re filament assembly. Each analysis usually comprised 400 cycles of data acquisition (8 s per integration). Stable Nd isotope ratios are expressed using conventional delta notation, where δ146Nd which is the per mil deviation in the measured 146Nd/144Nd relative to the widely measured reference standard JNdi-1:
Double spike deconvolution was undertaken using the Wolfram Mathematica program and is based on the algebraic resolution method (Millet and Dauphas, 2014). As the radiogenic isotope 143Nd is not used during double spike deconvolution, the spike proportion, and the geological and analytical fractionation factors resolved during deconvolution can be used to calculate the 143Nd/144Nd ratios of the samples (see McCoy-West et al., 2020, for equations). It has been demonstrated that these 143Nd/144Nd values agree within analytical uncertainty of previously published values for conventionally processed samples (see Figure 1 in McCoy-West et al., 2017) providing confidence of the accuracy of the δ146Nd obtained from the same measurement. As a secondary check of data quality, it is also now possible to independently deconvolve the δ148/144Nd of the samples to confirm the mass dependence of analyses (Figure 1). The data presented here have been normalized to JNdi-1 = 0, to correct for small offsets in the measured composition of JNdi-1 during different analytical periods due to differences in faraday collector efficiency (McCoy-West et al., 2020). Propagated uncertainties were calculated using the 95% standard error on the average of JNdi-1 for the correction period. Applying a secondary normalization such as this is common practice for radiogenic TIMS data (e.g., Bennett et al., 2007; McCoy-West et al., 2013) and stable isotope data generated by MC-ICP-MS (e.g., Goldberg et al., 2013; Millet and Dauphas, 2014; Neely et al., 2018; McCoy-West et al., 2019) in order to deal with small amounts of non-exponential mass bias that is not accounted for by internal corrections methods. The long-term reproducibility of the δ146Nd measurements is considered to better than ± 0.015‰ (McCoy-West et al., 2017, 2020).
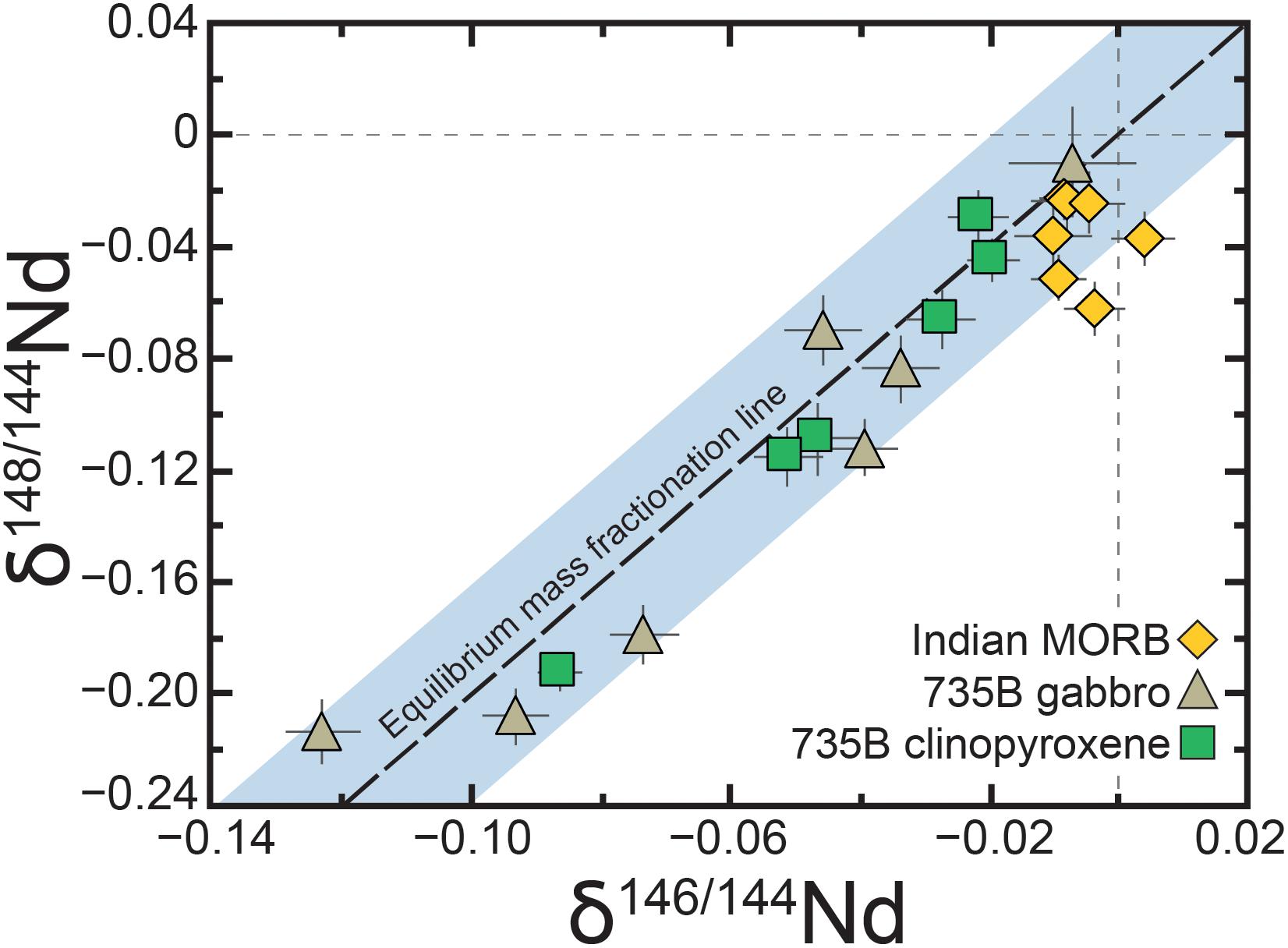
Figure 1. Plot showing the mass dependent covariation between δ146Nd and δ148Nd for samples measured in this study. Shaded field represents an uncertainty in δ148Nd of ± 0.038‰, which is based on the long-term uncertainty in δ146Nd (±0.015‰; McCoy-West et al., 2020) but has been increased proportionally based on the relative mass difference between the isotopes and the less precise counting statistics due to the smaller ion beam on 148Nd.
Samples and Results
Samples
This study focuses on samples from Ocean Drilling Program Hole 735B in the Atlantis II Transform Fault area located at 32°S 57°E on the Southwest Indian Ridge (SWIR) to the southeast of Madagascar (Figure 2). This region contains 12 Ma gabbroic ocean crust (John et al., 2004; Baines et al., 2009) that is exposed on a 5-km transverse ridge (Atlantis Bank) which formed as the result of detachment faulting from 13 to 10 Ma (Dick et al., 1991). The spreading rate in this region is ∼1.6 cm/year which is extremely slow (Baines et al., 2007). Hole 735B is located ∼95 km south of the present-day ridge axis and drilled 1508 m into the gabbroic rocks unroofed at the Atlantis Bank core complex, recovering a plutonic section dominated by series of generally coarse-grained olivine gabbros cross-cut by more evolved rocks (gabbronorites, oxide gabbros) and subordinate troctolite (Dick et al., 1991; Coogan et al., 2001; Natland and Dick, 2001). More felsic leucocratic igneous rocks are rare, making up ≤1% of the Hole 735B core and are generally present as highly altered, small crosscutting veins (Dick et al., 2000). Petrologic evidence and fluid inclusion studies suggest the ocean crust at Hole 735B is 4 ± 1 km thick, with 60–70% of the total gabbro column sampled (Vanko and Stakes, 1991; Muller et al., 1997).
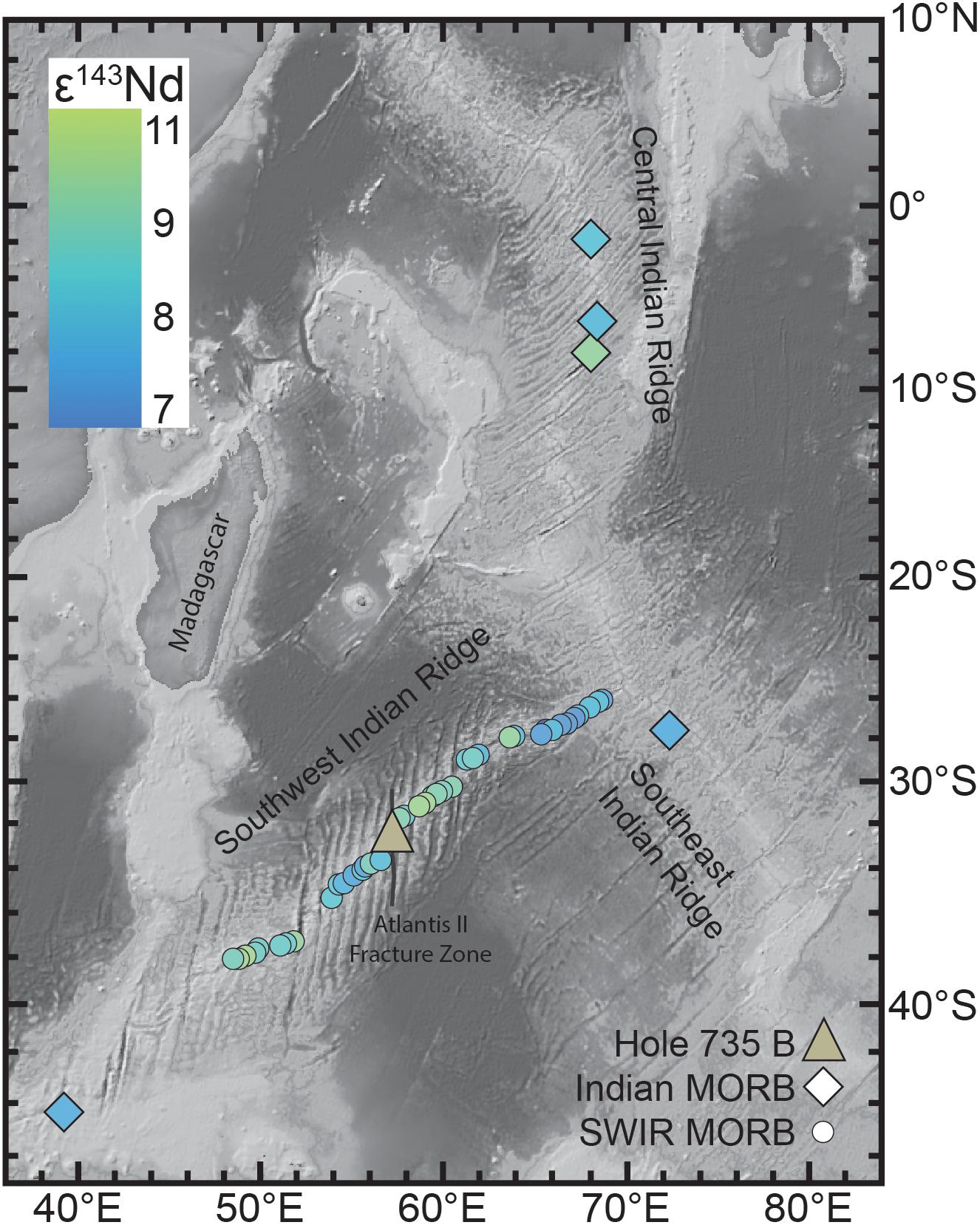
Figure 2. Map showing the major spreading ridges in the Indian Ocean and the locations of Hole 735B and the MORB glasses analyzed in this study. Symbols for MORB have been color coded based on their radiogenic Nd isotope compositions (diamonds samples herein; circles previously published data for the Southwest Indian Ridge (Meyzen et al., 2005). Bathymetry is from Ryan et al. (2009).
To supplement the gabbroic rocks analyses of MORB glass from the Indian Ocean where undertaken. These samples are widely distributed coming from all the major ridge segments in the Indian Ocean (SWIR, Southeast Indian Ridge and Central Indian Ridge; Figure 2) and have been previously been analyzed for Sr-Nd-Hf-Pb and Os radiogenic isotopes (Salters, 1996; Schiano et al., 1997; Escrig et al., 2004).
Results
Indian Ocean MORB
Measurements of six MORB glasses from the Indian Ocean show a restricted range in Nd stable isotopes with δ146Nd ranging from −0.015 to −0.029‰ (δ146Nd = 14 ppm; Figure 3 and Table 1). The average composition of the Indian Ocean MORB is δ146Nd = −0.025 ± 0.010‰ (n = 7; 95% SE = ± 0.005‰) and identical within uncertainty to that of MORB from the Garrett Fracture Zone (Pacific Ocean) reported previously with δ146Nd = −0.022 ± 0.019‰ (n = 4; 95% SE = ± 0.025‰; McCoy-West et al., 2017). Radiogenic neodymium isotope composition of MORB vary from ε143Nd = +7.8 to +9.8, consistent with previous analyses from this region (Hamelin et al., 1986; Meyzen et al., 2005) and show no covariation with δ146Nd (Figure 4). Average global MORB is calculated as δ146Nd = −0.024 ± 0.013‰ (n = 11; 95% SE = ± 0.004‰) and is similar to the composition of terrestrial magmatic rocks and within the uncertainty of the composition of chondritic meteorites (Figure 3).
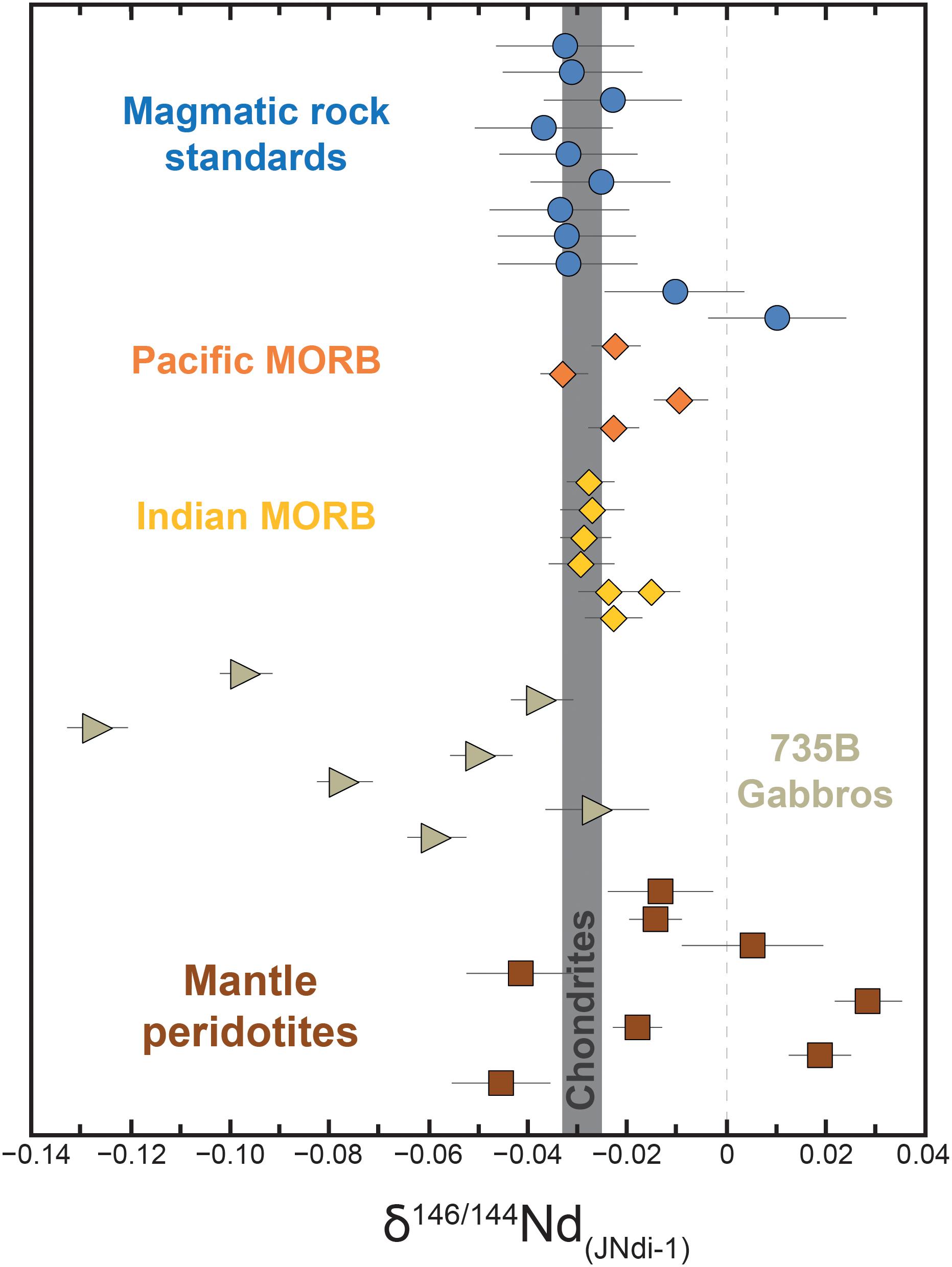
Figure 3. Comparison of δ146Nd in a range of terrestrial rocks. Indian MORB and Hole 735B gabbros are new measurements, all other data is from McCoy-West et al. (2017). Most data points are plotted with propagated two standard errors, except the magmatic rock standards which are plotted with the long-term reproducibility (±0.015‰) due to averaging multiply measurements/digestions. The gray band represents the chondritic average ±95% standard error on the mean (–0.029 ± 0.004‰; n = 38; McCoy-West et al., 2017).
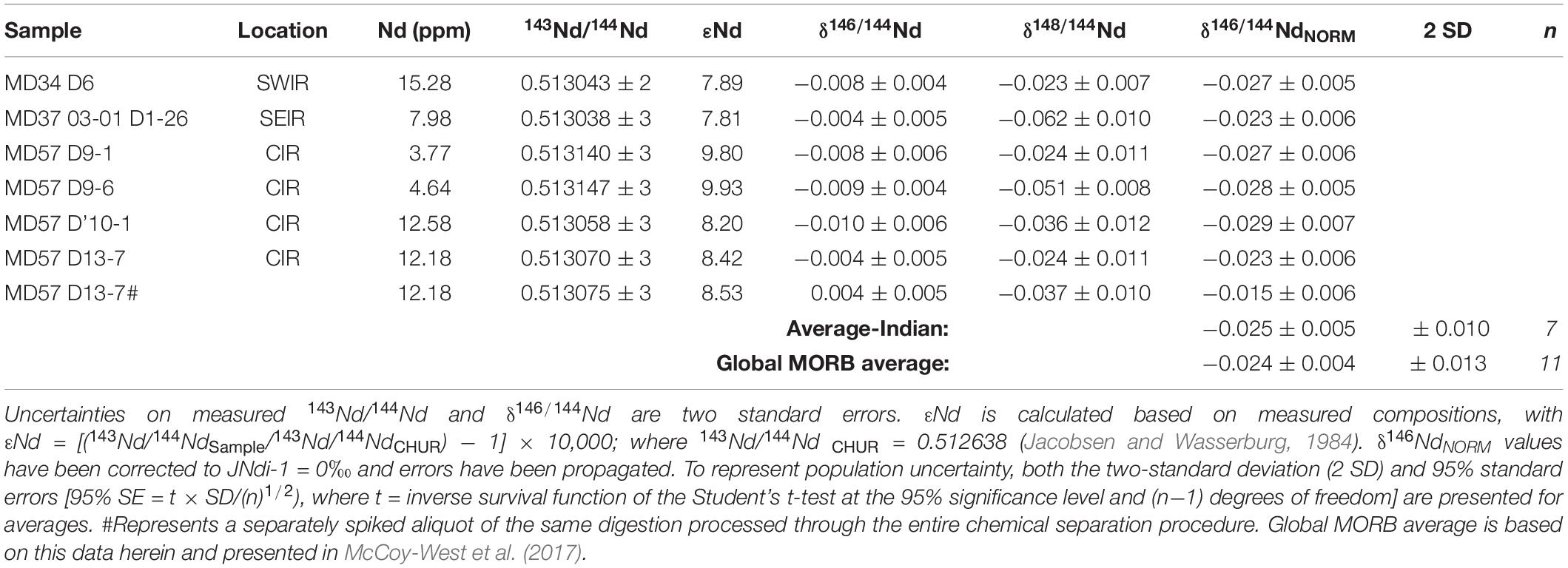
Table 1. Neodymium concentrations and isotopic compositions of glass from Indian mid-ocean ridge basalts.
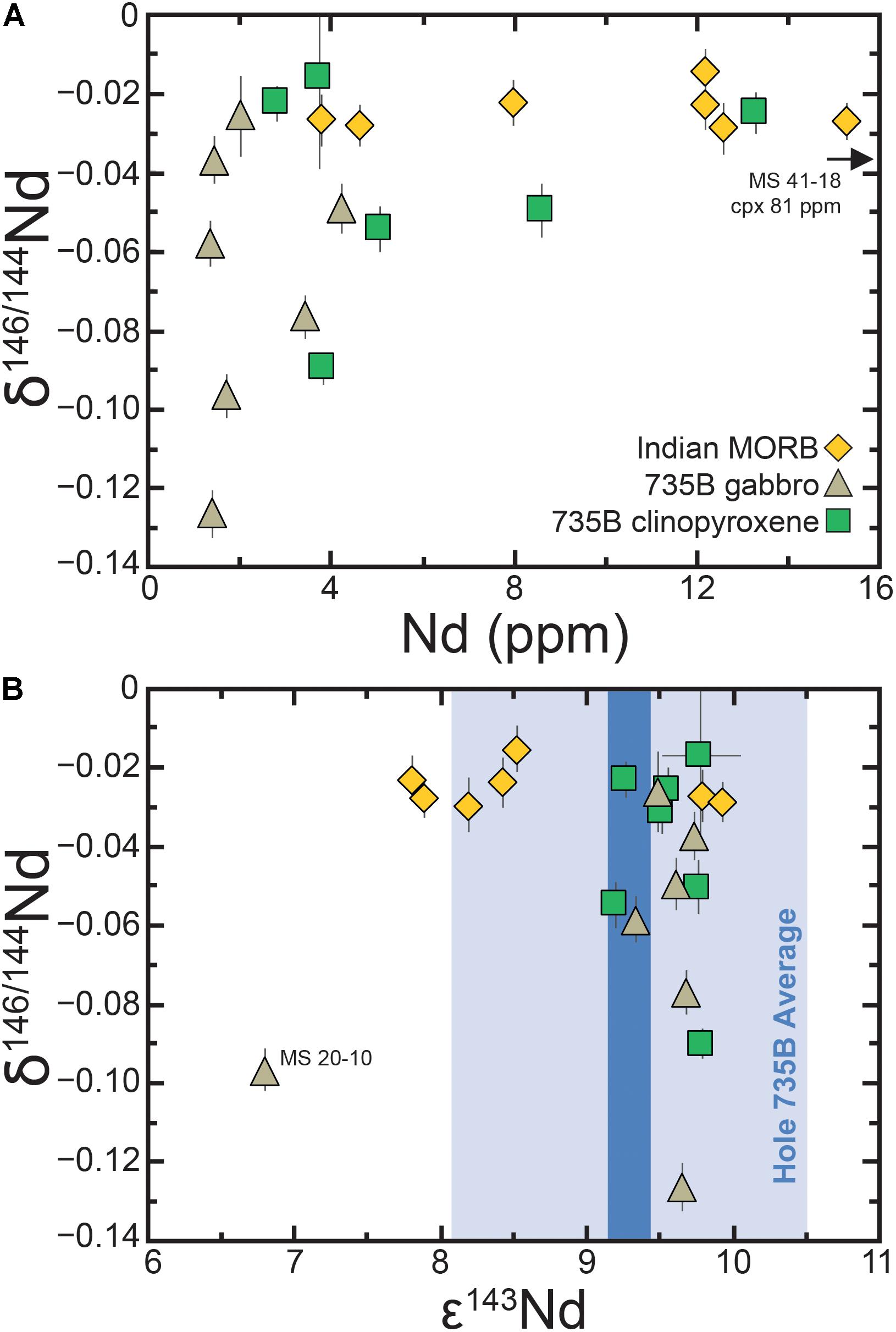
Figure 4. Comparison of the Nd contents and isotopic compositions of Indian MORB and Hole 735B gabbro whole rocks and clinopyroxene separates. Graphs of δ146Nd versus Nd concentration (A) and ε143Nd (B), respectively. Uncertainties on δ146Nd are propagated two standard errors. The blue shaded area represents the average Nd isotope composition of the Hole 735B (Kempton et al., 1991; Hart et al., 1999; Holm, 2002; herein). The light blue represents the average ±2 standard deviations: (ε143Nd = 9.29 ± 1.21; 143Nd/144Nd = 0.513114 ± 62; n = 72) with the dark blue band representing the 95% standard error of the mean (ε143Nd = ± 0.14; 143Nd/144Nd = ± 8).
735B Gabbro Whole Rock
The gabbro’s from Hole 735B possess highly variable δ146Nd with values ranging from −0.026 to −0.126‰ (n = 7; δ146Nd = 100 ppm; Figure 3 and Table 2), which extend to the lightest Nd stable isotope compositions so far observed. This large variation in δ146Nd is not correlated with the Nd concentration (1.4–4.2 ppm) or ε143Nd (ca. +9.5; excluding MS20-10 ε143Nd = + 6.8) with the samples possessing relatively similar radiogenic isotope compositions (Figure 4). Whole rock geochemistry is correlated with δ146Nd (Figure 5): strong (r2 > 0.6) positive correlations are observed with SiO2, Al2O3, Na2O, and CaO; with negative correlations observed against MgO, FeOT, and MnO. However, occasionally some samples appear as outliers, specifically sample BN-1(F) has a very light δ146Nd of −0.126‰ which is distinct from the strong trend formed by the rest of the gabbros (Figure 5C). Despite these strong correlations between δ146Nd and major element contents, no correlation is observed with indicators of magmatic differentiation (e.g., Mg#; Figure 6A), with the gabbros possessing an extremely narrow range of Mg# from 0.73 to 0.79.
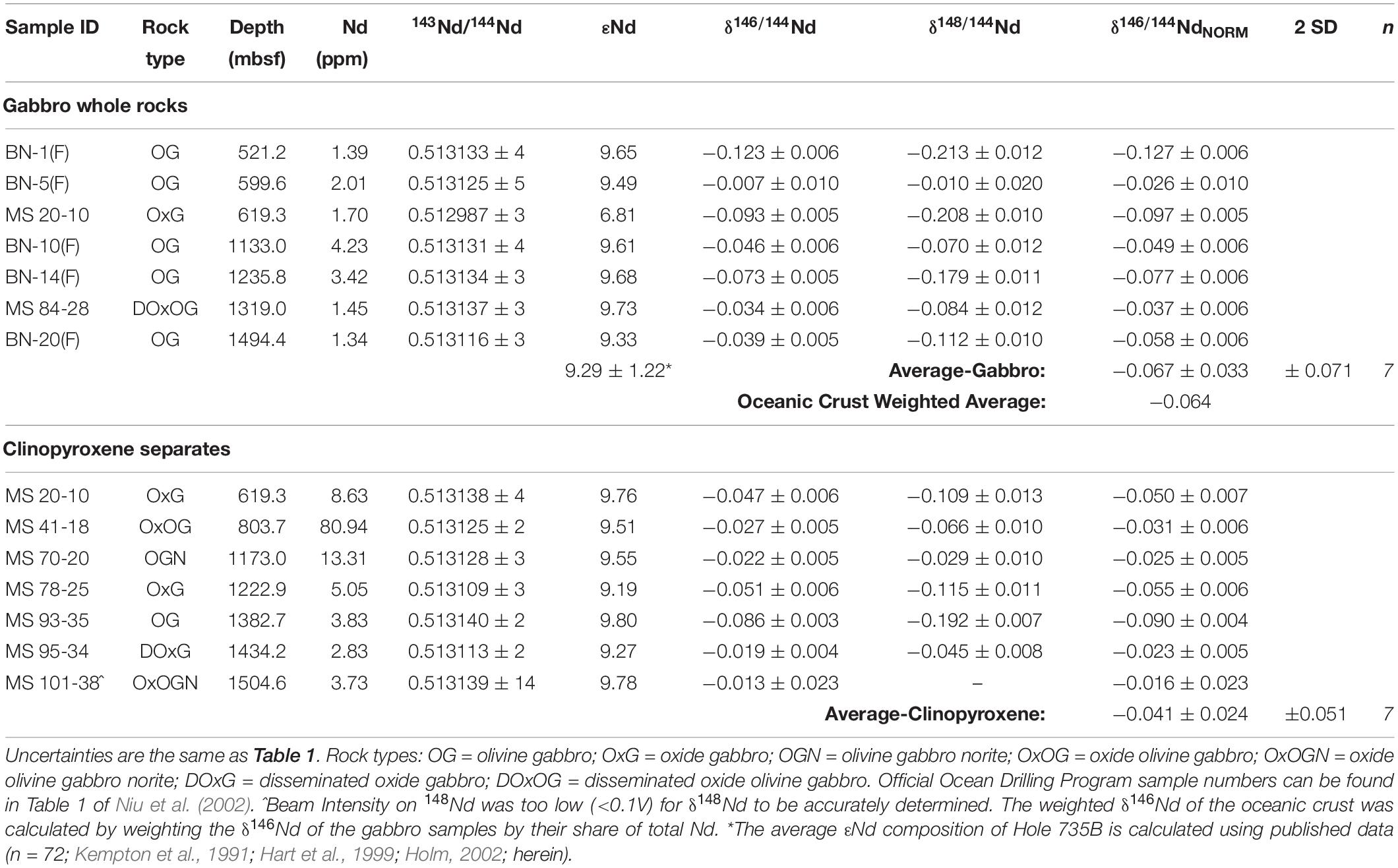
Table 2. Neodymium concentrations and isotopic compositions of gabbros and clinopyroxene separates from Hole 735B on the southwest Indian Ridge.
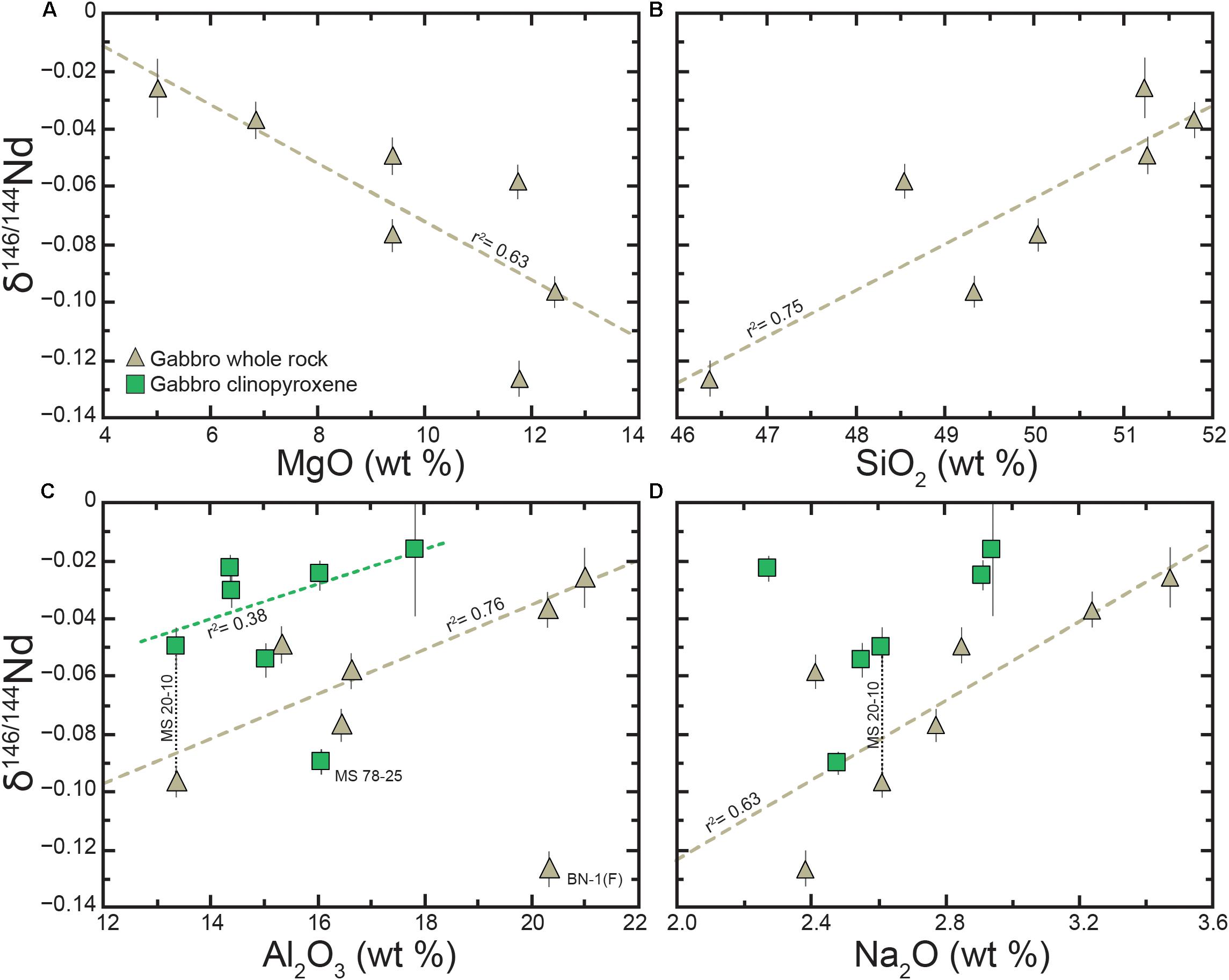
Figure 5. Graphs of Nd stable isotope composition of gabbroic rocks and clinopyroxene separates from Hole 735B versus whole rock major element contents. (A) MgO, (B) SiO2, (C) Al2O3, (D) Na2O. Major element data is from Niu et al. (2002) and Bach et al. (2001). In (C) samples which are significant outliers gabbro BN-1(F) and clinopyroxene MS78-25 and are excluded from the regressions.
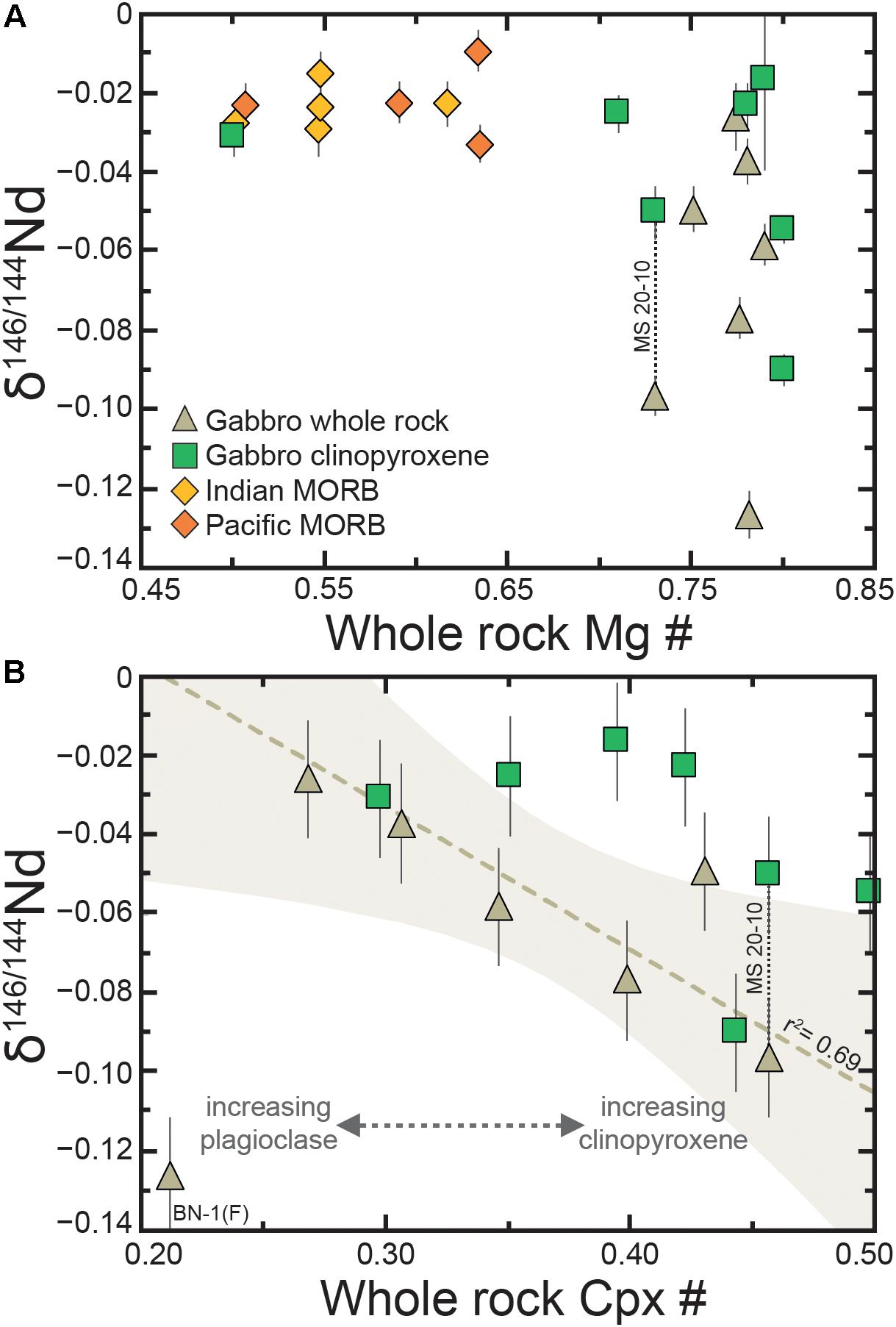
Figure 6. Graphs of δ146Nd against whole rock Mg# (A) and whole rock cpx# (B), respectively. (A) Note the distinctly different compositions of MORB and Hole 735B gabbros (Mg# = molar Mg/[Mg + Fe2+]). (B) Clinopyroxene-number (cpx# = cpx/[cpx + plag]) estimates the relative mass proportions of clinopyroxene and plagioclase in a sample based on the CaO/Al2O3 ratio and is calculated using the formula in Niu et al. (2002). A strong negative correlation is observed between δ146Nd and cpx#. Regression line and uncertainty envelope (shaded area) is calculated through the data excluding sample BN-1(F) using Isoplot (Ludwig, 2008) assuming a 2.5% uncertainty on the cpx# and the long-term uncertainty on δ146Nd (±0.015). The clinopyroxene separates are variably offset, generally to heavier values, relative to the whole rock relationship consistent with the operation of a disequilibrium process.
735B Gabbro Clinopyroxene
Clinopyroxene separates from Hole 735B possess between 2.8 and 13.3 ppm Nd (Figure 4A), excluding one separate from MS 41-18 that has a usually high Nd concentration of 80.9 ppm (Table 2). Comparable to the whole rocks the clinopyroxene separates also possess extremely variable δ146Nd with values ranging from −0.016 to −0.090‰ (n = 7; δ146Nd = 84 ppm; Table 2) with a constant radiogenic isotope composition (ε143Nd = +9.0 to +9.6; Figure 4B). For the one gabbro MS20-10, where both the whole rock and clinopyroxene composition are available the clinopyroxene is resolvably heavier (Δ146Ndclinopyroxene–whole rock = + 0.047‰). Qualitatively this finding holds when comparing the other samples using their whole rock Al2O3 and Na2O contents with the majority of clinopyroxene separates forming a secondary trend at heavier δ146Nd than the whole rock samples at similar major element compositions (Figures 5C,D).
Discussion
Spatial Variations in the Nd Isotope Composition of the Oceanic Crust
Radiogenic Nd Isotope Variability
One of the fundamental strengths of radiogenic isotopes is they remain unfractionated by magmatic process (e.g., fractional crystallization; partial melting), but rather provide a time-integrated tracer of parent-daughter fractionation (e.g., Sm from Nd). The heterogenous isotopic compositions of MORB globally has been used to map distinct mantle domains and trace long-term recycling of isotopically enriched components in the depleted MORB mantle (Zindler and Hart, 1986; Salters and Stracke, 2004; Hofmann, 2007). Indian MORB has less radiogenic Nd and more radiogenic Sr at the same Pb isotope composition than Atlantic, and Pacific MORB (see Figure 2 in Stracke et al., 2005). Despite this distinction significant variations in ε143Nd are observed across the Indian Ocean between spreading ridges (Hamelin et al., 1986; Robinson et al., 2001; Coogan et al., 2004; Meyzen et al., 2005), and even within individual segments of the SWIR (Figure 2). The previous characterization of Hole 735B (Kempton et al., 1991; Hart et al., 1999; Holm, 2002) means it provides an ideal test case to examine this isotopic heterogeneity with depth (Figure 7). Previous analyses of gabbro from Hole 735B have ε143Nd values spanning a significant range from +7.7 to +10.6, which is nearly the entire range observed in Indian MORB. The average composition of Hole 735B drill core is calculated as +9.29 ± 0.14 (95% SE) and is within uncertainty of basalts collected adjacent to the overlying Atlantis II fracture zone (ε143Nd = +9.13 ± 0.19), suggesting that the major sources of gabbroic rocks and overlying MORB are broadly similar. The clinopyroxene and whole rock samples analyzed herein are generally indistinguishable from the Hole 735B average, except sample MS20-10 (ε143Nd = +6.8) which is the least radiogenic so far measured but remains well within the range of heterogeneity observed along the SWIR (Figure 7). The preservation of extreme isotopic heterogeneity in oceanic mantle between constituent phases and across small length is increasingly being recognized as a common phenomenon (Burton et al., 2012; Lambart et al., 2019). This is not an unexpected results given the depleted mantle represents a mixture of materials at different stages in their evolution, and is therefore unlikely to be a isotopically homogeneous (e.g., Salters and Stracke, 2004).
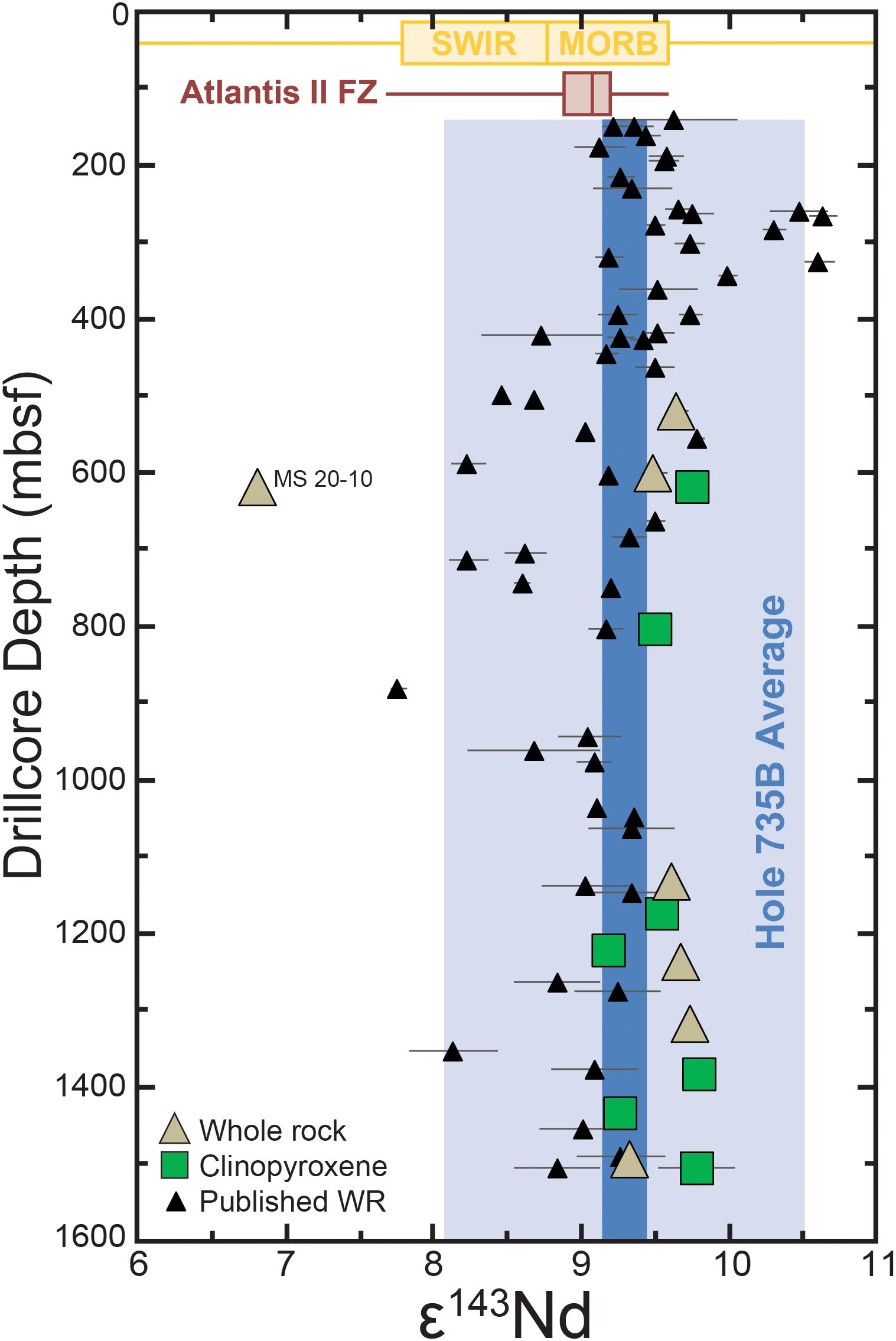
Figure 7. Downhole variation in radiogenic Nd isotopes (ε143Nd) in Hole 735B. Previously published compositions for 735B gabbros (Kempton et al., 1991; Hart et al., 1999; Holm, 2002) are not shown above 100 m. Box and whisker plots are shown for basalts immediately adjacent to the Atlantis II fracture zone (Robinson et al., 2001; Coogan et al., 2004) and Southwest Indian Ridge MORB (Robinson et al., 2001; Coogan et al., 2004; Meyzen et al., 2005). The blue shaded field is the average composition of Hole 735B as shown in Figure 4.
Stable Nd Variations in Hole 735B
Stable isotope ratios are time-independent and solely fractionated by the processes operating. At equilibrium, the magnitude of isotopic fractionation is proportional to the relative mass difference between the isotopes and inversely proportional to temperature (1/T2) (Bigeleisen and Mayer, 1947; Urey, 1947). Due to the well characterized nature of Hole 735B it provides a unique opportunity to study stable isotope fractionations in the lower oceanic crust. The oceanic gabbros have δ146Nd values extending to extremely light compositions (−0.026 to −0.127‰; Figure 3). In Hole 753B at long wavelengths (>200 m) there is generally a good covariation between the chemical compositions of the major silicate phases, consistent with the in situ crystallization of four distinct olivine gabbro bodies (Dick et al., 2002). Three major mineralogical discontinuities have been identified in the lower portion of Hole 735B (picked on the basis of plagioclase anorthite content: see Figure 16 in Dick et al., 2002). These bodies are also clearly delineated by whole rock major element compositions, with the gabbroic rocks in each body generally become slightly more evolved up section (lower Mg#; Figure 8A). Two of the lightest gabbro samples in Hole 735B occur above 650 mbsf (Figure 8B), however, when also considering the composition of the clinopyroxene separates no clear relationship between δ146Nd and depth is observed. Unfortunately, the current dataset remains too limited to constrain the variability of δ146Nd with depth, either throughout the entire drill core or relative to the individual position of the samples within the discrete gabbro bodies (Figure 8B).
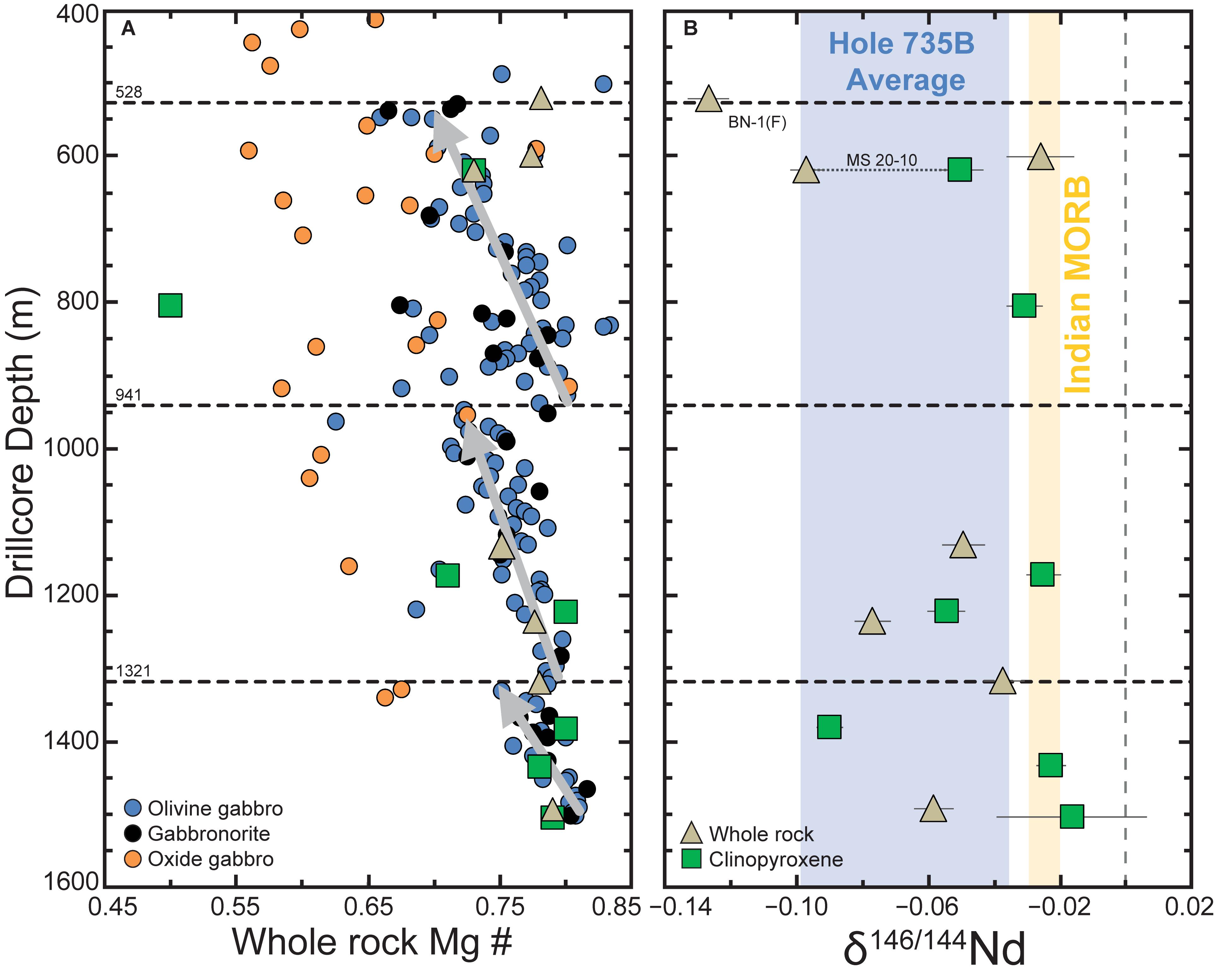
Figure 8. Compositional variation in gabbroic rocks from Hole 735B relative to sampling depth. (A) Plot of whole rock Mg# relative to drill core depth. Major element data for the samples herein is from Niu et al. (2002) and Bach et al. (2001) with comparative data taken from Dick et al. (1999), for clarity only the three major rock types are plotted. Dotted lines at 528, 941, and 1321 m represent major mineralogical discontinues identified in Dick et al. (2002) thought to delineate separate gabbro bodies. (B) Graph of δ146Nd in gabbroic rocks and clinopyroxene separates relative to sample depth. The yellow shaded area shows the average for Indian MORB (–0.025 ± 0.005‰; 95% SE) with the blue field the average composition of Hole 735B (–0.067 ± 0.033‰).
Resolving the Causes of Nd Stable Isotope Variation in Hole 735B
The lower oceanic crust is an extremely complex magmatic environment. Indeed, the crustal section at Hole 735B preserves 952 discrete intervals on basis of clear textural and mineralogic differences (Dick et al., 2002). The complexity of slow spreading ridges means that a range different process maybe contributing to the natural variations observed in δ146Nd. Ultimately, the whole rock compositions of the preserved gabbros integrate the effects of differences in mineral proportions, mineral compositions and any secondary modification through alteration.
Possible Low Temperature Fractionation?
Significant fractionation of both boron and iron isotopes have been shown to be caused by alteration in the oceanic crust (Spivack and Edmond, 1987; Rouxel et al., 2003). Boron is highly enriched in seawater and fluids and Fe is a major element in most mineral phases which is also sensitive to redox changes. In contrast, Nd a light (L)REE is generally moderately to highly incompatible in most silicate phases, possess a single valence state (3+), and is extremely fluid immobile (Bau, 1991). These characteristics make Nd generally very robust to secondary modification. Oxygen isotope evidence shows that abundant seawater penetration appears to be limited to the upper part of the crust preserved at Hole 735B (≥500 mbsf; Kempton et al., 1991; Hart et al., 1999; Gao et al., 2006). Given that the samples analyzed here are below this depth and the extremely low concentration of Nd in seawater (ca. 3–4 ppt), it is reasonable to conclude that seawater interaction had no effect on the δ146Nd values measured here. Bach et al. (2001) undertook a detailed study of the effects of alteration in Hole 735B with an overall decrease in the intensity of hydrothermal alteration observed with increasing depth. Alteration becomes rarer below 600 mbsf with the lowermost part of the section (500–1500 mbsf) showing the effects of a complex low-temperature (ca. 250°C) alteration history probably related to the tectonic uplift of the basement. This low-temperature alteration is localized to fractured regions where intense alteration of the host rocks can be observed adjacent to secondary mineral (smectite, chlorite, calcite, zeolite, sulfide, and Fe-oxyhydroxides) filled veins (Bach et al., 2001). Bienvenu et al. (1990) showed that during intense alteration of basalt the REE can become mobilized during the breakdown of secondary minerals. Here, we have focused on the freshest possible samples to eliminate alteration as a factor in interpreting geochemical signals. The majority of the gabbros possess a restricted range of Nd concentration (1.3–2.0 ppm; Table 2) but span the entire range δ146Nd (Figure 4A), with no correlation observed that could be attributed to Nd loss. Neodymium stable isotope compositions have also been compared to other common indicators of alteration intensity such as loss on ignition (Figure 9A) or enrichment of fluid mobile elements (e.g., Rb; Figure 9B) and remain uncorrelated with these parameters. Further evidence of the inability of alteration to fractionate δ146Nd comes from analyses of chondritic meteorites, which contain a range of exotic phases including REE enriched sulfides that are highly susceptible to alteration, that had experienced variable terrestrial alteration but showed no systematic change in δ146Nd values with increasing weathering intensity (see Figure 6 in McCoy-West et al., 2017). Therefore, we are confident the variations in δ146Nd observed here are generated by magmatic processes during formation of the oceanic crust.
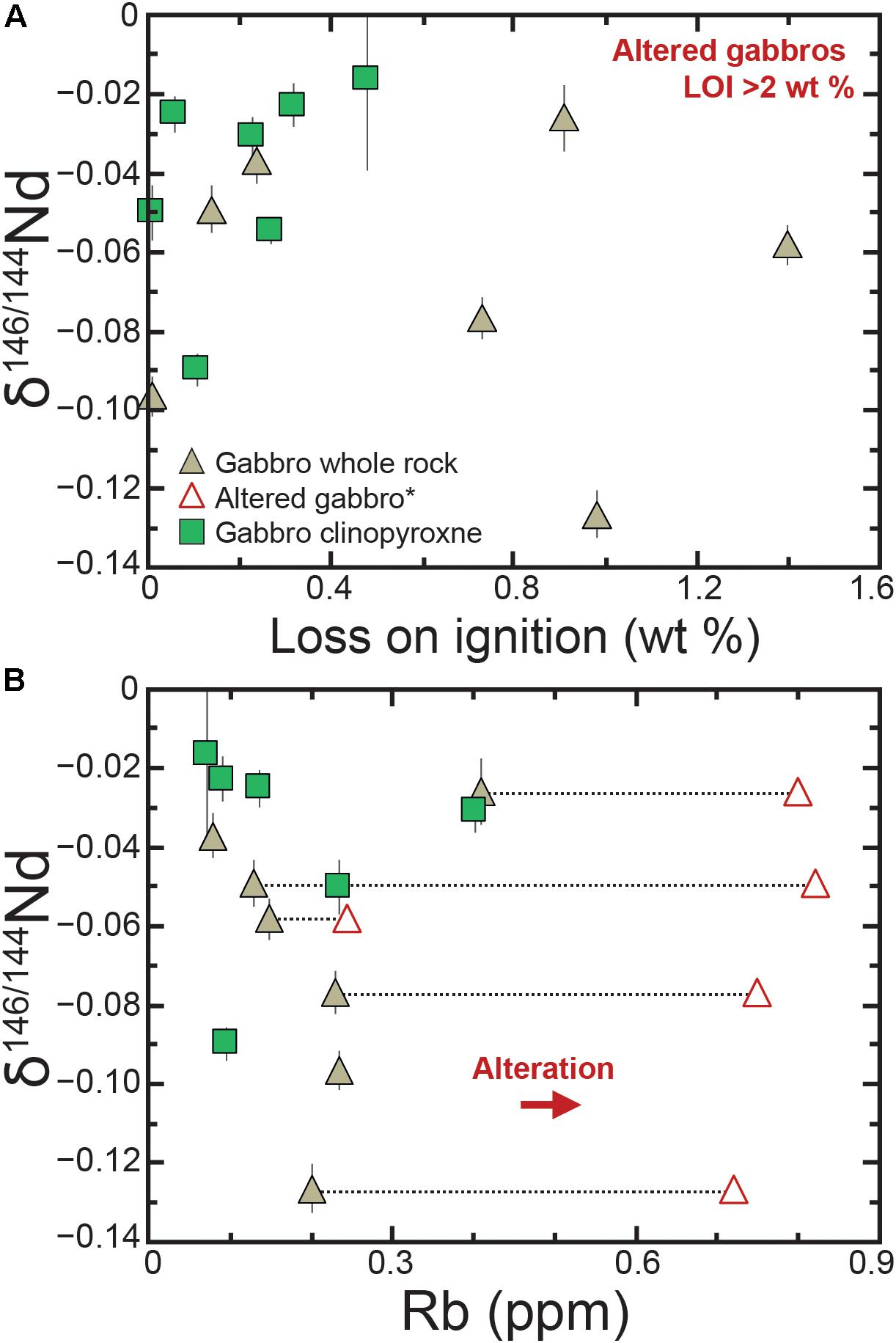
Figure 9. Graphs of δ146Nd against loss on ignition (A) and whole rock rubidium concentration (B), respectively. Clinopyroxenes separates are plotted at the compositional values of their whole rock. Comparative data comes from Bach et al. (2001) and Niu et al. (2002). (B) Altered samples are plotted at the δ146Nd of their equivalent unaltered sample. Significant enrichment of large ion lithophile elements, such as Rb occurs during alteration as the result of clay formation (e.g., smectite; Staudigel et al., 1981).
Fractionation or Accumulation of Magmatic Crystals
The gabbros at Hole 735B are dominated by three major phases: (1) plagioclase is present in every rock type examined, is the most abundant phase (50–65% in representative gabbros; Dick et al., 1999), and typically occurs in near cotectic proportions with clinopyroxene and olivine (Bloomer et al., 1991); (2) clinopyroxene is next most prevalent phase, with the majority of gabbros containing from 15 to 45% (Dick et al., 1999), its abundance varies inversely with the amount of plagioclase (Dick et al., 2002; Niu et al., 2002); and (3) olivine is a subordinate component and generally only constitutes 5–10% of the olivine gabbros (Dick et al., 1999). Clinopyroxene is the major host of Nd in the gabbroic rocks of Hole 735B. Due to distortions of the crystal lattice partition coefficients are dependent on the temperature, pressure and composition of a mineral during crystallization (Wood and Blundy, 1997). Clinopyroxene is characterized by three crystallographically unique polyhedra, with the LREE mainly occupying the eightfold coordinated M2 site (Ross et al., 2013; Bédard, 2014). Higher Al2O3 (IVAl) contents significantly increase the compatibility of REE in clinopyroxene, due to the high Al2O3 contents (IVAl up to 0.4) in typical Hole735B gabbros DNd values of 0.4–0.6 are likely (Hill et al., 2000). Due to the high modal abundance of plagioclase it could also host a significant amount of Nd. Plagioclase is a framework of linked (Si-Al)-O tetrahedra, with large interstices filled with Ca and Na ions. The REE occur in sixfold coordination in plagioclase (Cherniak, 2003), were they can replace Na following a coupled substitution due to their similar atomic radii (Nd = 0.983 Å; Na = 1.02 Å; Shannon, 1976). The partitioning of Nd into plagioclase is dependent on the anorthite content (An = Ca/[Ca + Na]; max An60 in 735B) with DNd values of 0.1–0.15 probable (Bindeman and Davis, 2000; Tepley et al., 2010; Sun et al., 2017). The REE are very incompatible in olivine (DNd = 0.0002; Prinzhofer and Allégre, 1985) and therefore olivine is not considered a likely host of Nd and will not be discussed further.
Geochemical evidence shows that the gabbroic rocks preserved at Hole 735B are not simple quenched melts and instead they represent crystal cumulates. Their whole rock compositions have Mg# that are too high, TiO2 contents that are too low and too wide a range in CaO/Al2O3 to be in equilibrium with melts (Niu et al., 2002). Gabbro whole rock compositions (e.g., CaO, Sc) are instead largely a function of the relative modal proportions of clinopyroxene and plagioclase (see Figure 6 in Niu et al., 2002). Niu et al. (2002) demonstrated that it is possible to calculate the proportion of clinopyroxene using the CaO/Al2O3 (RC/A; Cpx# = [1.3835 × RC/A – 0.5163]/[1.2571 × RC/A + 0.4587]) as it is insensitive to dilution by more mafic components (i.e., olivine). A strong correlation is observed between δ146Nd in Hole 735B gabbros and the proportion of clinopyroxene (Figure 6B), with increasing amounts of clinopyroxene correlated with lighter isotopic compositions. Stable isotope compositions are process dependent and therefore the accumulation or fractionation of a significant amount of crystal cargo with a unique isotopic composition can cause resolvable changes in the isotopic composition of a cogenetic rock suite (e.g., Sossi et al., 2012; Millet et al., 2016; McCoy-West et al., 2018). Stable isotope theory suggests that at equilibrium with a fixed valance state heavy isotopes will prefer the site with the stiffest bonds (i.e., lower coordination number; Schauble, 2004). The coordination of the REE in silicate glass is highly composition dependent with sixfold to ninefold coordination observed (e.g., Ponader and Brown, 1989). Existing experimental work suggests that Nd (and the LREE) are predominantly sevenfold coordinated in silicate and other oxide glasses, although sixfold coordination can also be present (Mann and DeShazer, 1970; Robinson, 1971; Weber and Brawer, 1982; Rao et al., 1983). Given that Nd sits in the cubical coordinated M2 site in clinopyroxene (VIIINd) an isotopic fractionation would be predicted with clinopyroxene preferentially taking lighter Nd isotopes. This prediction is at odds with the observed subparallel trends of δ146Nd versus major element contents which suggest isotopically heavier clinopyroxene (Figure 5) and the measured clinopyroxene-whole pair in gabbro MS20-10 (Figures 6,8). Two solutions could account for this discrepancy either the theoretical prediction does not hold in this instance or alternatively a disequilibrium reaction is occurring.
To explain the trend seen in Figure 6B would require that the gabbros with highest clinopyroxene contents represent magmas that have previously fractionated a significant amount of isotopically heavy clinopyroxene and then migrated and crystallized with a significantly lighter whole rock composition. To explore this process, we undertook Rayleigh fractionation modeling to show the effect of crystallizing isotopically heavy clinopyroxene (Figure 10): based on the measured offset in sample MS 20-10 (Δ146Ndclinopyroxene–whole rock = +0.047‰). Even with an extreme amount of fractionation of solely clinopyroxene (up to 40%), which is rather unrealistic and not consistent with major element compositions of the gabbros, the lightest melt produced only has a δ146Nd of −0.048‰ which is significantly less than the range preserved in Hole 735B (Figure 10). Plagioclase probably also has a role to play in the evolution of the melts in Hole 735B. Given the lack of direct measurements of this phase, we can merely make an educated assumption based on stable isotope principles. Based on Nd coordination in plagioclase (VINd) and silicate melts (VINd or VIINd) it would be predicted that plagioclase should have δ146Nd identical or slightly heavier than the melt. If the two phases occur in subequal proportions (as shown in Figure 6B) due to the difference in incompatibly, plagioclase will account for only about 1/5th of the total Nd of the rock. Therefore, even if Nd occurs in sevenfold coordination in melts and assuming a large Δ146Ndplagioclase–whole rock of +0.1‰, δ146Nd will change more rapidly but does not differ significantly from the pure clinopyroxene model already discussed (Figure 10). Ultimately, accumulation or fractionation of a mixture of clinopyroxene and plagioclase in subequal proportions is not capable of explaining the wide range of δ146Nd observed in Hole 735B gabbros instead disequilibrium processes are probably required.
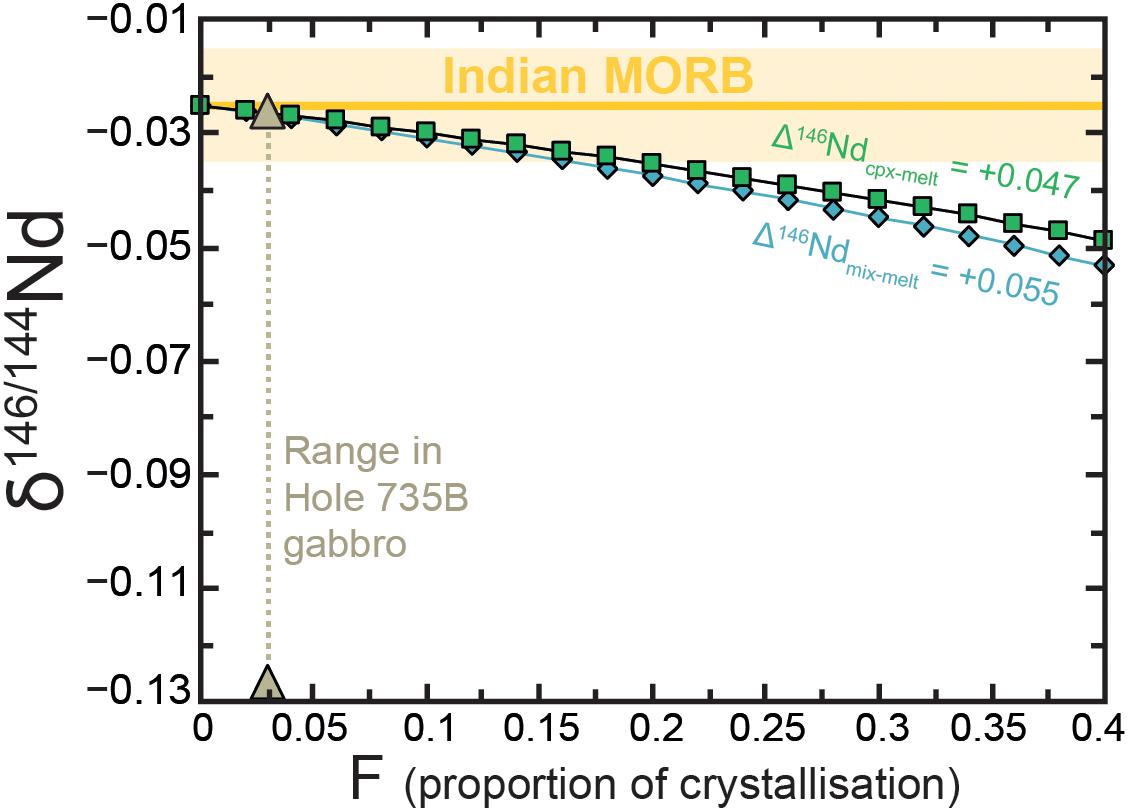
Figure 10. Rayleigh fractionation model showing the evolution of δ146Nd from fractional crystallization. Modeling assumes an initial δ146Nd of –0.025‰ based on Indian MORB, although this value is also within uncertainty of most global magmas and chondrites (see Figure 3). A clinopyroxene crystallization models (squares) is presented for Δ146Ndclinopyroxene–whole rock of + 0.047‰ (as observed in sample MS 20-10). A mixed assemblage model (diamonds) comprising 50% clinopyroxene and 50% plagioclase is also shown. This model uses the proportion of total Nd in the two phases (83.3% cpx; 16.7% plag) calculated assuming Dcpx = 0.5 and Dplag = 0.1 and assuming δ146Ndcpx = 0.021‰ and δ146Ndplag = 0.075‰. Even following significant amounts of crystallization (F = 0.4; 40%) it is not possible to reproduce the wide range of variation in δ146Nd observed in the Hole 735B gabbros.
Evidence for Reactive Porous Flow
The complicated histories preserved in plutonic rocks, collected from the three main ocean basins, have been used to propose that RPF is a nearly ubiquitous feature of the lower oceanic crust (e.g., Lissenberg and MacLeod, 2016). Complex zoning profiles, frozen mineral reaction textures, and mineral compositions and proportions that do not imitate models of simple fractional crystallization suggest that additional processes must have been involved in the formation of oceanic gabbros (Meyer et al., 1989; Coogan et al., 2000; Dick et al., 2002; Gao et al., 2007; Lissenberg and Dick, 2008; Lissenberg et al., 2013). RPF has been widely recognized through the over-enrichment of very to moderately incompatible trace elements in clinopyroxene (e.g., Ce/Y; see Figure 5 in Lissenberg and MacLeod, 2016), and poor correlations between mineral compositions and fractionation indices (e.g., elevated TiO2 contents in clinopyroxene; see Figure 5 in Lissenberg and Dick, 2008). Lissenberg and Dick (2008) presented a frozen example of RPF from the Kane Megamullion (mid-Atlantic Ridge), where ca. 5 cm wide channels of coarse-grained gabbro cross-cuts a medium grained troctolite host rock. Percolation of clinopyroxene-saturated melt through the troctolite (Eq. 2), led to the dissolution of olivine, plagioclase (partial) and Cr-spinel in the troctolite and crystallization of high Mg# clinopyroxene and lower-anorthite plagioclase.
Strong petrographic evidence is preserved that RPF has occurred widely in gabbros collected from the Atlantis Bank including: (1) textural evidence for the reaction of clinopyroxene with melt is preserved (i.e., complexly intergrown and ragged clinopyroxene grain boundaries); (2) troctolite-to-olivine gabbro reactions have been observed in the Hole 735B gabbros (see Figure 8 in Lissenberg and MacLeod, 2016); (3) significant core to rim enrichments of incompatible trace elements are preserved in clinopyroxene (Meyer et al., 1989; Kvassnes, 2004; Gao et al., 2007; Lissenberg and MacLeod, 2016); and (4) the composition of major silicate phases is poorly correlated with the evolution of the main gabbro bodies, instead a wide range in minor element concentrations (both compatible and incompatible) are seen in olivine and pyroxene at a given Mg#, consistent with widespread permeable flow of late-stage melt through these intrusions (Dick et al., 2002). Clinopyroxene with TiO2 contents >0.3 wt% cannot be generated by fractional crystallization of a primitive MORB magma and instead have been interpreted to reflect RPF (Lissenberg and Dick, 2008; Lissenberg and MacLeod, 2016). A negative correlation is observed between δ146Nd in clinopyroxene and the TiO2 of the clinopyroxene for samples from Hole 735B (Figure 11), consistent with increasing amounts of RPF producing isotopically light clinopyroxene. Furthermore, when the clinopyroxene separates are plotted on the Cpx# versus δ146Nd graph (Figure 6B), they are generally heavier, but variably offset from the well-defined whole rock trend consistent with a disequilibrium process where each individual sample is affected uniquely.
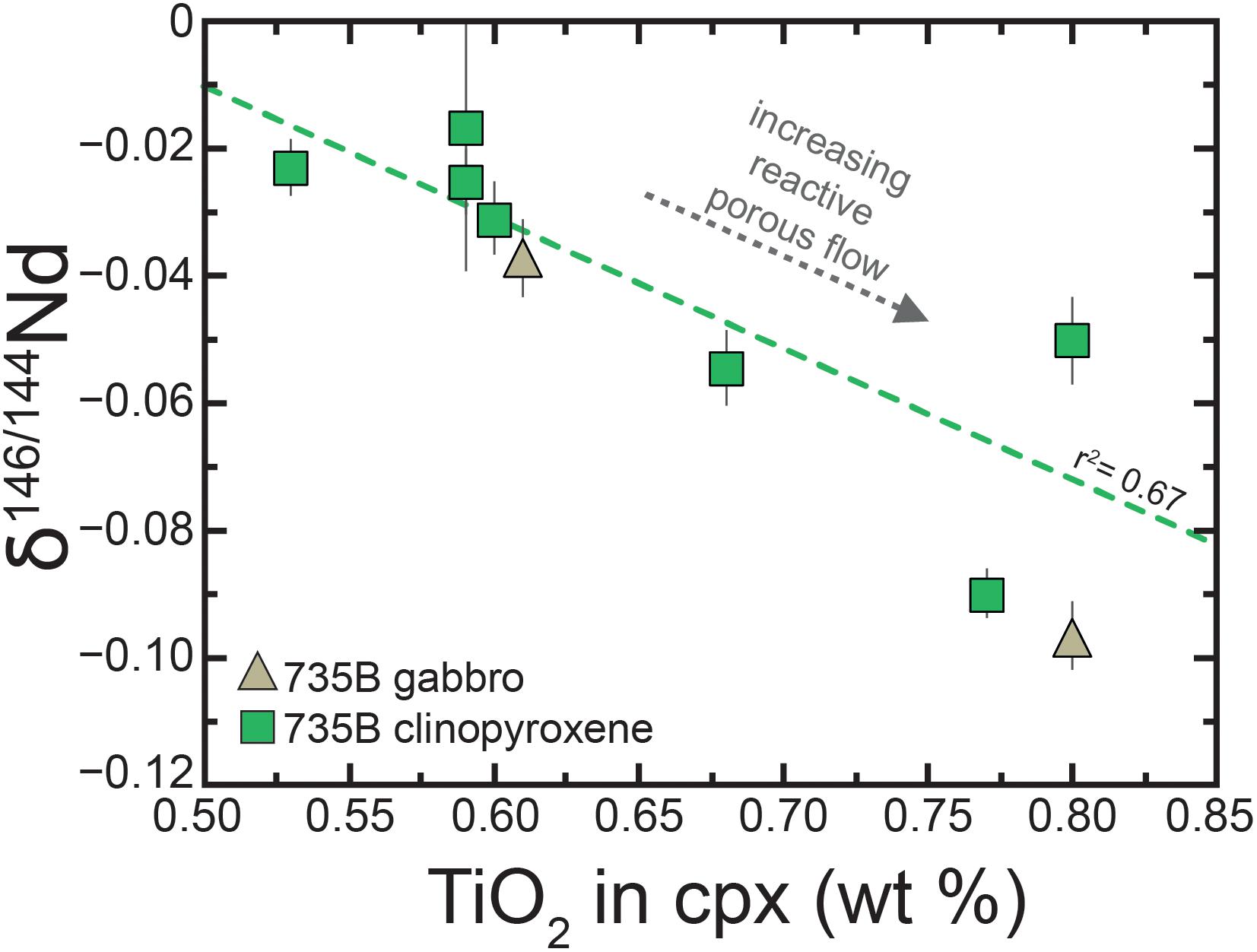
Figure 11. Relationship between the TiO2 content of clinopyroxene and δ146Nd in Hole 735B. Clinopyroxene major element data is only available for two of the whole rock gabbro samples (Niu et al., 2002). TiO2 contents >0.3 wt% are considered to result from reactive porous flow. A strong negative correlation is observed between δ146Nd and the TiO2 of the clinopyroxene, consistent with increased amounts of reactive porous flow producing isotopically lighter clinopyroxene.
Given the multi-faceted evidence for RPF in Hole 735B next we explore the effects of this process on δ146Nd values. The preservation of highly fractionated isotopic signatures in minerals is a widespread phenomenon and can arise because of diffusion during crystal growth or through entrainment of crystals that are not in equilibrium with a melt (e.g., Lundstrom et al., 2005; Weyer and Ionov, 2007; Sio et al., 2013; McCoy-West et al., 2018). When kinetic isotope fractionation occurs light isotopes will preferentially diffuse faster than heavier ones (e.g., Richter et al., 2009), although diffusion in a medium is also dependent on temperature, pressure, chemical composition and the spatial anisotropy of the medium (Watkins et al., 2017). In silicate melts, the ratio of the diffusivities of two isotopes (Drel) of the same element have been shown to depend on their masses Richter et al. (2003):
where DH and DL are the diffusivities of the heavy and light isotopes, respectively, β is a dimensionless empirical parameter allowing comparison between different elements, and mH and mL are their masses (in this instance 145.91313 and 143.9101, respectively). Given there is currently no theoretical basis for predicting the value of the β exponent it must be determined experimentally (Watson and Müller, 2009). Unfortunately experiments for Nd do not exist, however, the experimental determined values of β in silicate melts for heavier elements possess an extremely restricted range of 0.03–0.07 (Ca, Mg, Fe; Watkins et al., 2017). When modeling diffusion during crystal growth two assumptions are made: (1) the surface of the mineral is in equilibrium with the fluid at the solid-fluid interface and (2) that diffusion in the solid is negligible (which is valid given Nd diffusion in clinopyroxene and plagioclase is extremely slow; Van Orman et al., 2001; Cherniak, 2003). Therefore, any kinetic effect isotope fractionation occurs due to diffusion in the melt. Albarede and Bottinga (1972) introduced the concept of diffusive boundary layers showing that in fast growing crystals the uptake of impurities can diverge significantly from equilibrium, with the key parameter being the ratio of the growth rate to the diffusivity of the impurity (R/D; Figure 12). The kinetic uptake of REE impurities is more efficient when grain sizes are larger, or when a high melt flux moves through a region (Van Orman et al., 2002) making gabbros in the lower oceanic crust an ideal location for this process to occur. Watson and Müller (2009) developed a simple expression that calculates the maximum kinetic isotope fractionation in a growing crystal that would occur prior to the system reaching steady-state:
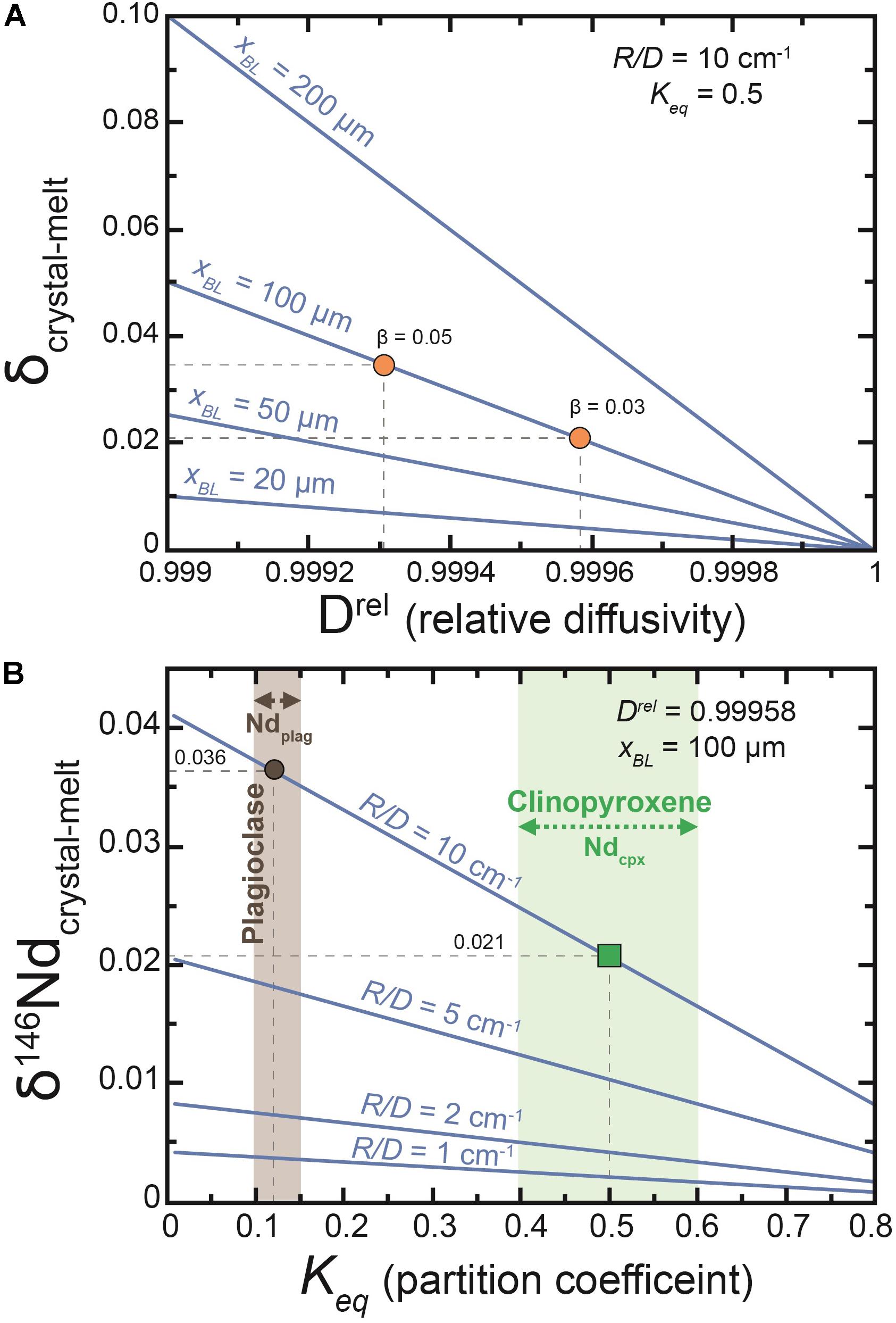
Figure 12. Diffusion modeling showing the maximum kinetic isotope fractionation predicted in a growing crystal. (A) The magnitude of isotope fractionation is proportional to both the thickness of the diffusive boundary layer (xBL) and the relative diffusivity of the two isotopes of interest (Drel = DH/DL). Circles show the calculated Drel for Nd based on assuming a β factor for the system of 0.03 and 0.05. (B) The magnitude of isotopic fractionation is proportional to the rate of crystal growth (R) relative to the diffusivity in the medium (D). By making geological reasonable assumptions it is possible to estimate the likely kinetic isotope fractionation in the major Nd hosts of the gabbros (clinopyroxene and plagioclase).
where δ is the deviation from equilibrium in parts per thousand. R is the growth rate of the crystal (in cms–1), Keq is the equilibrium partition coefficient between the crystal and the growth medium, and xBL is thickness of the boundary layer. The magnitude of isotope fractionation is proportional to both the thickness of the diffusive boundary layer and the relative diffusivity (Drel) of the two isotopes of interest which is dependent on the β factor for the system (Figure 12A). By assuming the diffusive boundary layer is 100 μm thick, the maximum observed in silicate systems (Kerr, 1995), and using the minimum experimentally determined β factor (i.e., βNd = βFe = 0.03; Richter et al., 2009) we can explore the size of fractionations in δ146Nd which can be ascribed to RPF (Figure 12B). This modeling shows that for a given crystal growth rate, larger isotopic fractionations are predicted when Nd is more incompatible in a phase, and minimal fractionation is predicted at R/D < 1. Assuming an R/D of 10, which is at the upper end of values for phenocryst growth (Orlando et al., 2008; Maaløe, 2011), kinetic isotope fractionations of ca. 0.021 and 0.036‰ are predicted in clinopyroxene and plagioclase, respectively. A strong negative correlation is observed between δ146Nd and the modal proportion of clinopyroxene in Hole 735B gabbros (Figure 6B). For kinetic process to explain this correlation would require that the extremely isotopically light gabbros represent quenched melts that have lost a proportion of their heavy isotopes during RPF in a different region of the lower crust (melt migration is observed at least on the short length scales in Hole 735B). Natland and Dick (2001) suggested that these gabbroic cumulates formed in a dense crystal mush dominated by fractures, channelized flow and intergranular porous flow which is the ideal environment to generate kinetic isotope fractionations. The mechanism responsible for generating variations in δ146Nd is clearly not straightforward, with the sample with the lightest δ146Nd clearly not adhering to this correlation [BN-1(F) = −0.127‰]. However, this sample was collected at 521 mbsf, within a region that contains several distinct lithostragraphic units, and within 10 m of a major boundary between distinct two gabbro bodies (Figure 8). This region probably experienced more concentrated melt flow aiding in the dissolution and reprecipitation of isotopic signatures at grain boundaries. Given the small magnitude of isotopic fractionation predicted in Figure 12, for RPF alone to account for the δ146Nd variations observed in Hole 735B gabbros one of the following is required: (1) crystal growth rates during this process are higher than previous recognized; (2) the β value for Nd is higher than the minimum experimentally determined value used for the predictions; or (3) several cycles of RPF are required to generated the extreme isotope compositions of some the gabbros. Further experimental work is required to better understand this process. Given the unrivaled complexity of the oceanic curst at slow spreading ridges it is fitting that no single process can conclusively explain the variability observed instead a combination of several processes is probably required.
Reconciling the Disconnect Between the Composition of the Oceanic Crust and Overlying MORB
The Homogenous δ146Nd of MORB
The large variations observed in δ146Nd in the gabbroic rocks of Hole 735B are distinctly different than erupted Indian MORB which possesses a very restricted range of δ146Nd from −0.015 to −0.029‰ (Figure 3). The average composition of Indian MORB is δ146Nd = −0.025 ± 0.010‰ (Figure 8), which is identical within uncertainty to the composition of Pacific MORB (Figure 3) and the global MORB average of −0.024 ± 0.013‰ (Table 1). In contrast, available mantle peridotite data display a significantly wider range of δ146Nd with values ranging from −0.045 to + 0.029‰ (McCoy-West et al., 2017). These peridotites have significantly lower Nd concentrations (0.10–0.81 ppm; McCoy-West et al., 2020) than erupted MORB and comprise samples from a variety of tectonic settings, therefore, several processes may be responsible for their variable δ146Nd including, but not limited to, partial melting, melt metasomatism, and mineralogical variations. In the MORB dataset no correlations are observed between δ146Nd and Nd radiogenic isotope compositions (Figure 4B), showing that mantle source heterogeneity and the process of formation are effectively decoupled. Given that δ146Nd is process dependent this means any initial variations in the δ146Nd of the source material need not be transferred to the final MORB, with the indistinguishable δ146Nd of global MORB suggesting that these magmas have been homogenized through the same global process. Partial melting concentrates Nd into the melt phase (modeling suggests that with only 10% melting >80% of the original Nd budget will be in the melt fraction; McCoy-West et al., 2015) helping effectively eliminate small scale heterogeneities from the source region. Further processing in the extensive magma chamber network observed at mid-ocean ridge systems globally, would be an ideal place to form such a homogenous reservoir. These magma chambers are required to be open systems to explain the trace element over-enrichment of MORB (O’Hara, 1977; Jenner and O’Neill, 2012), and therefore are constantly replenished with new melt providing an ideal mechanism to homogenize the δ146Nd of the magmas. Amongst the novel stable isotopes systems Nd appears unique with small heterogeneities greater than analytical precision observed for many other isotopic systems including Fe, Mo, Ba (Teng et al., 2013; Bezard et al., 2016; Nielsen et al., 2018). It will be interesting if the homogeneity of the MORB reservoir is confirmed when new data becomes available.
Can the Melts in the Oceanic Crust Generate MORB in the SWIR?
The discussion above shows that to produce the extremely light values observed in Hole 735B gabbros requires some level of RPF. Advocates of RPF also suggest that this mechanism is responsible for the geochemical variability observed in erupted MORB glasses (e.g., Lissenberg and Dick, 2008; Lissenberg and MacLeod, 2016). Meaning that MORB magmas and gabbros in the oceanic crust should directly complement each other. There are several lines of evidence that suggest this is not the case for the samples from the SWIR: (1) there is a significant disconnect between the major element composition of MORB magmas and the lower oceanic crust with MORB glasses being significantly more evolved than the bulk composition of Hole 735B, whereas they should be the complimentary melts of the preserved oceanic crust (i.e., Mg#; Figure 6A). Furthermore, the average composition of the crust at Hole 735B is not sufficiently primitive in terms of Mg# or highly compatible trace element concentrations (e.g., Ni) for the bulk crust to be in equilibrium with the mantle (Coogan et al., 2001); (2) Hole 735B is largely comprised of cumulate gabbros (Dick et al., 2000; Natland and Dick, 2001). Despite significant Sr and Eu anomalies (from plagioclase accumulation) being observed in individual samples (1–4 m length scale), the average composition of the complete upper 500 m section of the Hole 735B displays no significant Sr or Eu anomalies (≤1%), this implies that there has been local separation of melt and solids, but no large scale removal of melts into the upper crust (Hart et al., 1999); and (3) stable Nd isotope compositions also suggest a significant disconnect between the lower oceanic crust and MORB compositions. The calculated average composition of the gabbro whole rocks is δ146Nd = −0.067 ± 0.033‰ (95% SE), with the weighted average based on the proportion of Nd in each sample being almost an identical −0.064‰ (Table 2). This large isotopic discrepancy makes it very unlikely that significant melt has escaped the lower oceanic crust, and for the SWIR at least oceanic gabbros that have experienced RPF have had little impact on the compositions of erupted MORB.
Conclusions
Stable isotope compositions are process dependent and therefore provide an excellent mechanism to compare contrasting petrogenetic models. Here, we present the first neodymium stable isotope compositions of Indian Ocean MORB glass and gabbro whole rock and clinopyroxene separates from ODP Hole 735B on the SWIR which show:
(1) Mid-ocean ridge basalts are extremely homogenous with the average δ146Nd of Indian MORB of −0.025 ± 0.005‰, identical within uncertainty to the global average of −0.024 ± 0.004‰. Despite significant variability in the source composition of MORB magmas globally (i.e., 143Nd/144Nd) their indistinguishable δ146Nd suggests that any mantle source heterogeneities in δ146Nd are overprinted during the process of their formation. The extensive magma chamber at mid-ocean ridge systems globally, that are constantly replenished with new Nd-enriched melt, provide the ideal location to form such a homogenous reservoir.
(2) In Hole 735B the δ146Nd of gabbroic rocks is highly variable ranging from −0.026 to −0.127‰. Clinopyroxene separates also possess variable δ146Nd (δ146Nd = 74 ppm) but are variably offset to heavier values than the gabbro whole rocks at the same major element composition, with a Δ146Ndclinopyroxene–whole rock of +0.047‰ observed in one paired sample.
(3) Rayleigh fractionation modeling shows fractionation of isotopically heavy clinopyroxene ± plagioclase has a limited effect on δ146Nd even after significant fractional crystallization (F = 40%). Therefore, the large variations in δ146Nd observed in the oceanic gabbros cannot be generated solely by the fractionation or accumulation of magmatic minerals.
(4) Strong evidence exists to support RPF in Hole 735B, a process which will induce kinetic isotope fractionation during crystal growth. However, diffusion modeling based on geologically reasonable parameters, shows that minimal kinetic isotope fractionation ca. 0.02‰ will be induced in clinopyroxene. Thus, to produce the extremely light δ146Nd values observed in the gabbros would require multiple cycles of dissolution and reprecipitation of isotopic signatures at grain boundaries.
(5) In Hole 735B there is a large offset, significantly greater than analytical uncertainty, between the average composition of the lower oceanic crust (δ146Nd = −0.067‰) and erupted Indian MORB (δ146Nd = −0.025‰). Combined with the geochemical evidence (e.g., Sr and Eu anomalies) for no large-scale removal of melts into the upper crust we conclude that melts involved in RPF have no significant impact on the Nd stable isotope composition of MORB erupted at the SWIR. Whether this conclusion is a global phenomenon remains to be tested.
Data Availability Statement
All datasets generated for this study are included in the article/supplementary material.
Author Contributions
AM-W and KB conceived the study. AM-W and M-AM developed the double spike. AM-W undertook the chemistry and mass spectrometry, and wrote the manuscript with input from all the authors.
Funding
This project was funded by a National Environmental Research Council (United Kingdom) Grant (NE/N003926/1) to KB. While at Monash AM-W was supported by ARC grant FL160100168 to Peter Cawood.
Conflict of Interest
The authors declare that the research was conducted in the absence of any commercial or financial relationships that could be construed as a potential conflict of interest.
Acknowledgments
Yaoling Niu is thanked for generously providing the samples. AM-W thanks Peter Cawood for the freedom to complete this work. Geoff Nowell for the knowledge of mass spectrometry he imparted during my time at Durham. Priyadarshini Chowdhury for discussions about diffusion.
References
Albarede, F., and Bottinga, Y. (1972). Kinetic disequilibrium in trace element partitioning between phenocrysts and host lava. Geochim. Cosmochim. Acta 36, 141–156. doi: 10.1016/0016-7037(72)90003-90008
Bach, W., Alt, J. C., Niu, Y., Humphris, S. E., Erzinger, J., and Dick, H. J. B. (2001). The geochemical consequences of late-stage low-grade alteration of lower ocean crust at the SW Indian Ridge: results from ODP Hole 735B (Leg 176). Geochim. Cosmochim. Acta 65, 3267–3287. doi: 10.1016/S0016-7037(01)00677-679
Baines, A. G., Cheadle, M. J., John, B. E., Grimes, C. B., Schwartz, J. J., and Wooden, J. L. (2009). SHRIMP Pb/U zircon ages constrain gabbroic crustal accretion at Atlantis Bank on the ultraslow-spreading Southwest Indian Ridge. Earth Planet. Sci. Lett. 287, 540–550. doi: 10.1016/j.epsl.2009.09.002
Baines, A. G., Cheadle, M. J., Dick, H. J. B., Scheirer, A. H., John, B. E., Kusznir, N. J., et al. (2007). Evolution of the Southwest Indian Ridge from 55°45’E to 62°E: changes in plate-boundary geometry since 26 Ma. Geochemi.Geophys.Geosyst. 8, doi: 10.1029/2006GC001559
Bau, M. (1991). Rare-earth element mobility during hydrothermal and metamorphic fluid-rock interaction and the significance of the oxidation state of europium. Chem. Geol. 93, 219–230. doi: 10.1016/0009-2541(91)90115-90118
Bédard, J. H. (2014). Parameterizations of calcic clinopyroxene—Melt trace element partition coefficients. Geochem.Geophys., Geosyst. 15, 303–336. doi: 10.1002/2013GC005112
Bennett, V. C., Brandon, A. D., and Nutman, A. P. (2007). Coupled 142Nd-143Nd isotopic evidence for Hadean mantle dynamics. Science 318:1907. doi: 10.1126/science.1145928
Bezard, R., Fischer-Gödde, M., Hamelin, C., Brennecka, G. A., and Kleine, T. (2016). The effects of magmatic processes and crustal recycling on the molybdenum stable isotopic composition of Mid-Ocean Ridge Basalts. Earth Planet. Sci. Lett. 453, 171–181. doi: 10.1016/j.epsl.2016.07.056
Bienvenu, P., Bougault, H., Joron, J. L., Treuil, M., and Dmitriev, L. (1990). MORB alteration: rare-earth element/non-rare-earth hygromagmaphile element fractionation. Chem Geol. 82, 1–14. doi: 10.1016/0009-2541(90)90070-N
Bigeleisen, J., and Mayer, M. G. (1947). Calculation of equilibrium constants for isotopic exchange reactions. J. Chem. Phys. 15, 261–267. doi: 10.1063/1.1746492
Bindeman, I. N., and Davis, A. M. (2000). Trace element partitioning between plagioclase and melt: investigation of dopant influence on partition behavior. Geochim. Cosmochim Acta 64, 2863–2878. doi: 10.1016/S0016-7037(00)0038-386
Bloomer, S. H., Meyer, P. S., Dick, H. J. B., Ozawa, K., and Natland, J. H. (1991). “Textural and mineralogic variations in gabbroic rocks from Hole 735B,” in Proceedings. ODP, Sci. Results, eds R. Von Herzen and P. T. Robinson, (College Station, TX: Ocean Drilling Program).
Burton, K. W., Cenki-Tok, B., Mokadem, F., Harvey, J., Gannoun, A., Alard, O., et al. (2012). Unradiogenic lead in Earth’s upper mantle. Nat. Geosci. 5, 570–573. doi: 10.1038/ncomms5474
Cherniak, D. J. (2003). REE diffusion in feldspar. Chem. Geol. 193, 25–41. doi: 10.1016/S0009-2541(02)00246-242
Coogan, L. A. (2014). “4.14 - the lower oceanic crust,” in Treatise on Geochemistry, 2nd end, eds H. D. Holland and K. K. Turekian, (Oxford: Elsevier), 497–541. doi: 10.1016/b978-0-08-095975-7.00316-8
Coogan, L. A., MacLeod, C. J., Dick, H. J. B., Edwards, S. J., Kvassnes, A., Natland, J. H., et al. (2001). Whole-rock geochemistry of gabbros from the Southwest Indian Ridge: constraints on geochemical fractionations between the upper and lower oceanic crust and magma chamber processes at (very) slow-spreading ridges. Chem. Geol. 178, 1–22. doi: 10.1016/S0009-2541(00)00424-421
Coogan, L. A., and O’Hara, M. J. (2015). MORB differentiation: in situ crystallization in replenished-tapped magma chambers. Geochim. Cosmochim. Acta 158, 147–161. doi: 10.1016/j.gca.2015.03.010
Coogan, L. A., Thompson, G. M., MacLeod, C. J., Dick, H. J. B., Edwards, S. J., Hosford Scheirer, A., et al. (2004). A combined basalt and peridotite perspective on 14 million years of melt generation at the Atlantis Bank segment of the Southwest Indian Ridge: evidence for temporal changes in mantle dynamics? Chemi. Geol. 207, 13–30. doi: 10.1016/j.chemgeo.2004.01.016
Coogan, L. A., Saunders, A. D., Kempton, P. D., and Norry, M. J. (2000). Evidence from oceanic gabbros for porous melt migration within a crystal mush beneath the Mid-Atlantic Ridge. Geochem. Geophys., Geosyst. 1, doi: 10.1029/2000GC000072
Crisp, J. A. (1984). Rates of magma emplacement and volcanic output. J. Volcanol. Geotherm. Rese. 20, 177–211. doi: 10.1016/0377-0273(84)90039-90038
Dauphas, N., Roskosz, M., Alp, E. E., Neuville, D. R., Hu, M. Y., Sio, C. K., et al. (2014). Magma redox and structural controls on iron isotope variations in Earth’s mantle and crust. Earth Planet. Sci. Lett. 398, 127–140. doi: 10.1016/j.epsl.2014.04.033
Detrick, R. S., Buhl, P., Vera, E., Mutter, J., Orcutt, J., Madsen, J., et al. (1987). Multi-channel seismic imaging of a crustal magma chamber along the East Pacific Rise. Nature 326, 35–41. doi: 10.1038/326035a0
Dick, H. J. B., Fisher, R. L., and Bryan, W. B. (1984). Mineralogic variability of the uppermost mantle along mid-ocean ridges. Earth Planet. Sci. Lett. 69, 88–106. doi: 10.1016/0012-821X(84)90076-90071
Dick, H. J. B., Natland, J. H., Alt, J. C., Bach, W., Bideau, D., Gee, J. S., et al. (2000). A long in situ section of the lower ocean crust: results of ODP Leg 176 drilling at the Southwest Indian Ridge. Earth Planet. Sci. Lett. 179, 31–51. doi: 10.1016/S0012-821X(00)00102-103
Dick, H. J. B., Natland, J. H., and Miller, D. J. (1999). Proceedings. ODP, Initial Reports. College Station, TX: Ocean Drilling Program.
Dick, H. J. B., Ozawa, K., Meyer, P. S., Niu, Y., Robinson, P. T., Constantin, M., et al. (2002). “Primary silicate mineral chemistry of a 1.5-km section of very slow spreading lower ocean crust: ODP Hole 735B, Southwest Indian Ridge,” in Proceedings. ODP, Scientific Results, eds J. H. Natland, H. J. B. Dick, D. J. Miller, and V. Herzen, (College Station, TX: Ocean Drilling Program), 1–60.
Dick, H. J. B., Schouten, H., Meyer, P. S., Gallo, D. G., Bergh, H., Tyce, R., et al. (1991). “Tectonic evolution of the Atlantis II fracture zone,” in Proceedings. ODP, Sci. Results, eds R. Von Herzen and P. T. Robinson, (College Station, TX: Ocean Drilling Program).
Escrig, S., Capmas, F., Dupré, B., and Allègre, C. J. (2004). Osmium isotopic constraints on the nature of the DUPAL anomaly from Indian mid-ocean-ridge basalts. Nature 431, 59–63. doi: 10.1038/nature02904
Gale, A., Dalton, C. A., Langmuir, C. H., Su, Y., and Schilling, J.-G. (2013). The mean composition of ocean ridge basalts. Geochem.Geophys.Geosyst. 14, 489–518. doi: 10.1029/2012GC004334
Gao, Y., Hoefs, J., Hellebrand, E., von der Handt, A., and Snow, J. E. (2007). Trace element zoning in pyroxenes from ODP Hole 735B gabbros: diffusive exchange or synkinematic crystal fractionation? Contrib. Mineral. Petrol. 153, 429–442. doi: 10.1007/s00410-006-0158-154
Gao, Y., Hoefs, J., Przybilla, R., and Snow, J. E. (2006). A complete oxygen isotope profile through the lower oceanic crust. ODP Hole 735B. Chem. Geol. 233, 217–234. doi: 10.1016/j.chemgeo.2006.03.005
Goldberg, T., Gordon, G., Izon, G., Archer, C., Pearce, C. R., McManus, J., et al. (2013). Resolution of inter-laboratory discrepancies in Mo isotope data: an intercalibration. J. Anal. At. Spectrom. 28, 724–735. doi: 10.1039/C3JA30375F
Hamelin, B., Dupré, B., and Allègre, C. J. (1986). PbSrNd isotopic data of Indian Ocean ridges: new evidence of large-scale mapping of mantle heterogeneities. Earth Planet. Sci. Lett. 76, 288–298. doi: 10.1016/0012-821X(86)90080-90084
Hart, S. R., Blusztajn, J., Dick, H. J. B., Meyer, P. S., and Muehlenbachs, K. (1999). The fingerprint of seawater circulation in a 500-meter section of ocean crust gabbros. Geochim. Cosmochim. Acta 63, 4059–4080. doi: 10.1016/S0016-7037(99)00309-309
Hekinian, R., and Walker, D. (1987). Diversity and spatial zonation of volcanic rocks from the East Pacific Rise near 21° N. Contrib. Mineral. Petrol. 96, 265–280. doi: 10.1007/BF00371248
Herzberg, C. (2004). Geodynamic information in peridotite petrology. J Petrol 45, 2507–2530. doi: 10.1093/petrology/egh039
Hill, E., Wood, B. J., and Blundy, J. D. (2000). The effect of Ca-Tschermaks component on trace element partitioning between clinopyroxene and silicate melt. Lithos 53, 203–215. doi: 10.1016/S0024-4937(00)00025-26
Hofmann, A. W. (1997). Mantle geochemistry: the message from oceanic volcanism. Nature 385:219. doi: 10.1038/385219a0
Hofmann, A. W. (2007). “2.03 - Sampling mantle heterogeneity through oceanic basalts: isotopes and trace elements,” in Treatise on Geochemistry, eds H. D. Holland and K. K. Turekian, (Oxford: Pergamon), 1–44. doi: 10.1016/b0-08-043751-6/02123-x
Holm, P. M. (2002). Sr, Nd and Pb isotopic composition of in situ lower crust at the Southwest Indian Ridge: results from ODP Leg 176. Chem. Geol. 184, 195–216. doi: 10.1016/S0009-2541(01)00364-363
Jacobsen, S. B., and Wasserburg, G. J. (1984). Sm-Nd isotopic evolution of chondrites and achondrites. II. Earth Planet. Sci. Lett. 67, 137–150. doi: 10.1016/0012-821X(84)90109-2
Jenner, F. E., and O’Neill, H. S. C. (2012). Major and trace analysis of basaltic glasses by laser-ablation ICP-MS. Geochem.Geophys.Geosyst. 13:Q03003. doi: 10.1029/2011gc003890
John, B. E., Foster, D. A., Murphy, J. M., Cheadle, M. J., Baines, A. G., Fanning, C. M., et al. (2004). Determining the cooling history of in situ lower oceanic crust—Atlantis Bank. SW Indian Ridge. Earth Planet. Sci. Lett. 222, 145–160. doi: 10.1016/j.epsl.2004.02.014
Johnson, K. T. M., and Dick, H. J. B. (1992). Open system melting and temporal and spatial variation of peridotite and basalt at the Atlantis II Fracture Zone. J. Geophys. Res.: Solid Earth 97, 9219–9241. doi: 10.1029/92jb00701
Kamenetsky, V. S., Eggins, S. M., Crawford, A. J., Green, D. H., Gasparon, M., and Falloon, T. J. (1998). Calcic melt inclusions in primitive olivine at 43°N MAR: evidence for melt-rock reaction/melting involving clinopyroxene-rich lithologies during MORB generation. Earth Planet. Sci. Lett 160, 115–132. doi: 10.1016/s0012-821x(98)00090-9
Kempton, P. D., Hawkesworth, C. J., and Fowler, M. (1991). “Geochemistry and isotopic composition of gabbros from layer 3 of the Indian Ocean crust, Hole 735B,” in Proceedings ODP, Science Results, eds R. Von Herzen and P. T. Robinson, (College Station, TX: Ocean Drilling Program), 127–143.
Kerr, R. C. (1995). Convective crystal dissolution. Contrib. Mineral. Petrol. 121, 237–246. doi: 10.1007/bf02688239
Klein, E. M. (2003). “3.13 - Geochemistry of the Igneous Oceanic Crust,” in Treatise on Geochemistry, eds H. D. Holland and K. K. Turekian, (Oxford: Pergamon), 433–463. doi: 10.1016/b0-08-043751-6/03030-9
Klein, E. M., and Langmuir, C. H. (1987). Global correlations of ocean ridge basalt chemistry with axial depth and crustal thickness. J. Geophys. Res.: Solid Earth 92, 8089–8115. doi: 10.1029/JB092iB08p08089
Kvassnes, A. (2004). The Evolution of Oceanic Gabbros: In-situ and Ancient Examples.Cambridge, MA: MIT — Woods Hole Joint Program in Oceanography.
Lambart, S., Koornneef, J. M., Millet, M.-A., Davies, G. R., Cook, M., and Lissenberg, C. J. (2019). Highly heterogeneous depleted mantle recorded in the lower oceanic crust. Nat. Geosci.. 12, 482–486. doi: 10.1038/s41561-019-0368-369
Langmuir, C. H., and Hanson, G. N. (1980). An evaluation of major element heterogeneity in the mantle sources of basalts. Philos. Trans. R. Soc. Lon.. Ser. A Math. Phys. Sci. 297, 383–407. doi: 10.1098/rsta.1980.0223
Lissenberg, C. J., and Dick, H. J. B. (2008). Melt–rock reaction in the lower oceanic crust and its implications for the genesis of mid-ocean ridge basalt. Earth Planet. Sci. Lett. 271, 311–325. doi: 10.1016/j.epsl.2008.04.023
Lissenberg, C. J., and MacLeod, C. J. (2016). A reactive porous flow control on mid-ocean ridge magmatic evolution. J. Petrol. 57, 2195–2220. doi: 10.1093/petrology/egw074
Lissenberg, C. J., MacLeod, C. J., Howard, K. A., and Godard, M. (2013). Pervasive reactive melt migration through fast-spreading lower oceanic crust (Hess Deep, equatorial Pacific Ocean). Earth Planet. Sci. Lett. 361, 436–447. doi: 10.1016/j.epsl.2012.11.012
Lundstrom, C. C., Chaussidon, M., Hsui, A. T., Kelemen, P., and Zimmerman, M. (2005). Observations of Li isotopic variations in the trinity ophiolite: evidence for isotopic fractionation by diffusion during mantle melting. Geochim. Cosmochim. Acta 69, 735–751. doi: 10.1016/j.gca.2004.08.004
Maaløe, S. (2011). Olivine phenocryst growth in hawaiian tholeiites: evidence for supercooling. J Petrol. 52, 1579–1589. doi: 10.1093/petrology/egr015
Mann, M. M., and DeShazer, L. G. (1970). Energy levels and spectral broadening of neodymium ions in laser glass. J. f Appl. Phys. 41, 2951–2957. doi: 10.1063/1.1659342
McCoy-West, A. J., Millet, M.-A., Nowell, G. M., Nebel, O., and Burton, K. W. (2020). Simultaneous measurement of neodymium stable and radiogenic isotopes from a single aliquot using a double spike. J. Ana. At. Spectrom. 35, 388–402. doi: 10.1039/C9JA00308H
McCoy-West, A. J., Bennett, V. C., O’Neill, H. S. C., Hermann, J., and Puchtel, I. S. (2015). The interplay between melting, refertilization and carbonatite metasomatism in off-cratonic lithospheric mantle under Zealandia: an integrated major, trace and platinum group element study. J. Petrol. 56, 563–604. doi: 10.1093/petrology/egv011
McCoy-West, A. J., Bennett, V. C., Puchtel, I. S., and Walker, R. J. (2013). Extreme persistence of cratonic lithosphere in the Southwest Pacific: paleoproterozoic os isotopic signatures of Zealandia. Geology 41, 231–234. doi: 10.1130/G33626.1
McCoy-West, A. J., Chowdhury, P., Burton, K. W., Sossi, P., Nowell, G. M., Fitton, J. G., et al. (2019). Extensive crustal extraction in Earth’s early history inferred from molybdenum isotopes. Nat. Geosci. 12, 946–951. doi: 10.1038/s41561-019-0451-452
McCoy-West, A. J., Godfrey Fitton, J., Pons, M.-L., Inglis, E. C., and Williams, H. M. (2018). The Fe and Zn isotope composition of deep mantle source regions: insights from Baffin Island picrites. Geoch.et Cosmochim. Acta 238, 542–562. doi: 10.1016/j.gca.2018.07.021
McCoy-West, A. J., Millet, M.-A., and Burton, K. W. (2017). The neodymium stable isotope composition of the silicate Earth and chondrites. Earth Planet. Sci. Lett. 480, 121–132. doi: 10.1016/j.epsl.2017.10.004
McKenzie, D., and Bickle, M. J. (1988). The volume and composition of melt generated by extension of the lithosphere. J. Petrol. 29, 625–679. doi: 10.1038/nature12292
Meyer, P. S., Dick, H. J. B., and Thompson, G. (1989). Cumulate gabbros from the Southwest Indian Ridge, 54°S-7° 16′ E: implications for magmatic processes at a slow spreading ridge. Contrib. Mineral. Petrol. 103, 44–63. doi: 10.1007/BF00371364
Meyzen, C. M., Ludden, J. N., Humler, E., Luais, B., Toplis, M. J., Mével, C., et al. (2005). New insights into the origin and distribution of the DUPAL isotope anomaly in the Indian Ocean mantle from MORB of the Southwest Indian Ridge. Geochem.Geophys. Geosyst. 6, doi: 10.1029/2005GC000979
Michael, P. J., and Cornell, W. C. (1998). Influence of spreading rate and magma supply on crystallization and assimilation beneath mid-ocean ridges: evidence from chlorine and major element chemistry of mid-ocean ridge basalts. J. Geophys. Res. 103, 18325–18356. doi: 10.1029/98JB00791
Millet, M.-A., and Dauphas, N. (2014). Ultra-precise titanium stable isotope measurements by double-spike high resolution MC-ICP-MS. J. Anal. At. Spectrom 29, 1444–1458. doi: 10.1039/C4JA00096J
Millet, M.-A., Dauphas, N., Greber, N. D., Burton, K. W., Dale, C. W., Debret, B., et al. (2016). Titanium stable isotope investigation of magmatic processes on the Earth and Moon. Earth Planet. Sci. Lett. 449, 197–205. doi: 10.1016/j.epsl.2016.05.039
Muller, M. R., Robinson, C. J., Minshull, T. A., White, R. S., and Bickle, M. J. (1997). Thin crust beneath ocean drilling program borehole 735B at the Southwest Indian Ridge? Earth Planet. Sci. Lett. 148, 93–107. doi: 10.1016/S0012-821X(97)00030-37
Natland, J. H., and Dick, H. J. B. (2001). Formation of the lower ocean crust and the crystallization of gabbroic cumulates at a very slowly spreading ridge. J. Volcanol. Geothermal Res. 110, 191–233. doi: 10.1016/S0377-0273(01)00211-216
Neely, R. A., Gislason, S. R., Ólafsson, M., McCoy-West, A. J., Pearce, C. R., and Burton, K. W. (2018). Molybdenum isotope behaviour in groundwaters and terrestrial hydrothermal systems. Iceland. Earth Planet. Sci. Lett. 486, 108–118. doi: 10.1016/j.epsl.2017.11.053
Nielsen, S. G., Horner, T. J., Pryer, H. V., Blusztajn, J., Shu, Y., Kurz, M. D., et al. (2018). Barium isotope evidence for pervasive sediment recycling in the upper mantle. Sci. Adv. 4, eaas8675. doi: 10.1126/sciadv.aas8675
Niu, Y., Gilmore, T., Mackie, S., Greig, A., and Bach, W. (2002). “Mineral chemistry, whole-rock compositions, and petrogenesis of Leg 176 gabbros: Data and discussion,” in Proceedings. ODP, Scientific Results, eds J. H. Natland, H. J. B. Dick, D. J. Miller, and R. P. Von Herzen, (College Station, TX: Ocean Drilling Program), 1–60.
O’Hara, M. J. (1977). Geochemical evolution during fractional crystallisation of a periodically refilled magma chamber. Nature 266:503. doi: 10.1038/266503a0
O’Neill, H. S. C., and Jenner, F. E. (2012). The global pattern of trace-element distributions in ocean floor basalts. Nature 491, 698. doi: 10.1038/nature11678
Orlando, A., D’Orazio, M., Armienti, P., and Borrini, D. (2008). Experimental determination of plagioclase and clinopyroxene crystal growth rates in an anhydrous trachybasalt from Mt Etna (Italy). Eur. J. Mineral. 20, 653–664. doi: 10.1127/0935-1221/2008/0020-1841
Ponader, C. W., and Brown, G. E. (1989). Rare earth elements in silicate glassmelt systems: i. effects of composition on the coordination environments of La, Gd, and Yb. Geochim. Cosmochim. Acta 53, 2893–2903. doi: 10.1016/0016-7037(89)90166-X
Prinzhofer, A., and Allégre, C. J. (1985). Residual peridotites and the mechanisms of partial melting. Earth Planet. Sci. Lett. 74, 251–265. doi: 10.1016/0012-821x(85)90025-1
Rao, K. J., Wong, J., and Weber, M. J. (1983). Bonding and structure of Nd3+ in BeF2 glass by XANES and EXAFS spectroscopy. J. Chem. Phys. 78, 6228–6237. doi: 10.1063/1.444587
Regelous, M., Weinzierl, C. G., and Haase, K. M. (2016). Controls on melting at spreading ridges from correlated abyssal peridotite – mid-ocean ridge basalt compositions. Earth Planet.ry Sci. Lett. 449, 1–11. doi: 10.1016/j.epsl.2016.05.017
Rehkämper, M., and Hofmann, A. W. (1997). Recycled ocean crust and sediment in Indian Ocean MORB. Earth Planet. Sci. Lett. 147, 93–106. doi: 10.1016/S0012-821X(97)00009-5
Richter, F. M., Davis, A. M., DePaolo, D. J., and Watson, E. B. (2003). Isotope fractionation by chemical diffusion between molten basalt and rhyolite. Geochim. Cosmochim. Acta 67, 3905–3923. doi: 10.1016/S0016-7037(03)00174-171
Richter, F. M., Watson, E. B., Mendybaev, R., Dauphas, N., Georg, B., Watkins, J., et al. (2009). Isotopic fractionation of the major elements of molten basalt by chemical and thermal diffusion. Geochim. Cosmochim. Acta 73, 4250–4263. doi: 10.1016/j.gca.2009.04.011
Robinson, C. C. (1971). Evidence of sixfold coordination of Nd3+ in barium rubidium silicate glass. J. Chem. Phys. 54, 3572–3578. doi: 10.1063/1.1675383
Robinson, C. J., Bickle, M. J., Minshull, T. A., White, R. S., and Nichols, A. R. L. (2001). Low degree melting under the Southwest Indian Ridge: the roles of mantle temperature, conductive cooling and wet melting. Earth Planet. Sci. Lett. 188, 383–398. doi: 10.1016/S0012-821X(01)00329-326
Ross, K., Kamber, B. S., and McDonald, A. M. (2013). An empirical test of the crystal lattice strain model for rare-earth element partitioning into clinopyroxene. Chem. Geol. 340, 139–150. doi: 10.1016/j.chemgeo.2012.11.006
Rouxel, O., Dobbek, N., Ludden, J., and Fouquet, Y. (2003). Iron isotope fractionation during oceanic crust alteration. Chem. Geol. 202, 155–182. doi: 10.1016/j.chemgeo.2003.08.011
Ryan, W. B. F., Carbotte, S. M., Coplan, J. O., O’Hara, S., Melkonian, A., Arko, R., et al. (2009). Global multi-Resolution topography synthesis. Geochem. Geophys., Geosyst. 10:Q03014. doi: 10.1029/2008gc002332
Salters, V. J. M. (1996). The generation of mid-ocean ridge basalts from the Hf and Nd isotope perspective. Earth Planet. Sci. Lett. 141, 109–123. doi: 10.1016/0012-821X(96)00070-72
Salters, V. J. M., and Stracke, A. (2004). Composition of the depleted mantle. Geochem.Geophys.Geosyst. 5:Q05B07. doi: 10.1029/2003GC000597
Schauble, E. A. (2004). Applying Stable Isotope Fractionation Theory to New Systems. Rev. Mineral.Geochem. 55, 65–111. doi: 10.2138/gsrmg.55.1.65
Schiano, P., Birck, J.-L., and Allègre, C. J. (1997). Osmium-strontium-neodymium-lead isotopic covariations in mid-ocean ridge basalt glasses and the heterogeneity of the upper mantle. Earth Planet. Sci. Lett. 150, 363–379. doi: 10.1016/S0012-821X(97)00098-98
Shannon, R. (1976). Revised effective ionic radii and systematic studies of interatomic distances in halides and chalcogenides. Acta Crystallogr. Section A 32, 751–767. doi: 10.1107/S0567739476001551
Sinton, J. M., and Detrick, R. S. (1992). Mid-ocean ridge magma chambers. J. Geophys. Res.: Solid Earth 97, 197–216. doi: 10.1029/91JB02508
Sio, C. K. I., Dauphas, N., Teng, F.-Z., Chaussidon, M., Helz, R. T., and Roskosz, M. (2013). Discerning crystal growth from diffusion profiles in zoned olivine by in situ Mg–Fe isotopic analyses. Geochim. Cosmochim. Acta 123(Suppl. C), 302–321. doi: 10.1016/j.gca.2013.06.008
Sossi, P. A., Foden, J. D., and Halverson, G. P. (2012). Redox-controlled iron isotope fractionation during magmatic differentiation: an example from the Red Hill intrusion. S. Tasmania. Contrib. Mineral. Petrol. 164, 757–772. doi: 10.1007/s00410-012-0769-x
Spivack, A. J., and Edmond, J. M. (1987). Boron isotope exchange between seawater and the oceanic crust. Geochim. Cosmochim. Acta 51, 1033–1043. doi: 10.1016/0016-7037(87)90198-90190
Staudigel, H., Hart, S. R., and Richardson, S. H. (1981). Alteration of the oceanic crust: processes and timing. Earth Planet. Sci. Lett. 52, 311–327. doi: 10.1016/0012-821X(81)90186-90182
Stracke, A., Hofmann, A. W., and Hart, S. R. (2005). FOZO, HIMU, and the rest of the mantle zoo. Geochem. Geophys. Geosyst. 6:Q05007. doi: 10.1029/2004GC000824
Sun, C., Graff, M., and Liang, Y. (2017). Trace element partitioning between plagioclase and silicate melt: the importance of temperature and plagioclase composition, with implications for terrestrial and lunar magmatism. Geochim. Cosmochim. Acta 206, 273–295. doi: 10.1016/j.gca.2017.03.003
Teng, F.-Z., Dauphas, N., Huang, S., and Marty, B. (2013). Iron isotopic systematics of oceanic basalts. Geochim. Cosmochim. Acta 107, 12–26. doi: 10.1016/j.gca.2012.12.027
Tepley, F. J., Lundstrom, C. C., McDonough, W. F., and Thompson, A. (2010). Trace element partitioning between high-An plagioclase and basaltic to basaltic andesite melt at 1 atmosphere pressure. Lithos 118, 82–94. doi: 10.1016/j.lithos.2010.04.001
Urey, H. C. (1947). The thermodynamic properties of isotopic substances. J. t Chem. Soc. 562–581. doi: 10.1039/JR9470000562
Van Orman, J. A., Grove, T. L., and Shimizu, N. (2001). Rare earth element diffusion in diopside: influence of temperature, pressure, and ionic radius, and an elastic model for diffusion in silicates. Contrib. Mineral. Petrol. 141, 687–703. doi: 10.1007/s004100100269
Van Orman, J. A., Grove, T. L., and Shimizu, N. (2002). Diffusive fractionation of trace elements during production and transport of melt in Earth’s upper mantle. Earth Planet. Sci. Lett. 198, 93–112. doi: 10.1016/S0012-821X(02)00492-2
Vanko, D. A., and Stakes, D. S. (1991). “Fluids in oceanic layer 3: evidence from veined rocks, Hole 735B, Southwest Indian Ridge,” in Proceedings. ODP, Sci. Results, eds R. Von Herzen and P. T. Robinson, (College Station, TX: Ocean Drilling Program), 181–215.
Warren, J. M., Shimizu, N., Sakaguchi, C., Dick, H. J. B., and Nakamura, E. (2009). An assessment of upper mantle heterogeneity based on abyssal peridotite isotopic compositions. J. Geophys. Res.: Solid Earth 114, doi: 10.1029/2008JB006186
Watkins, J. M., DePaolo, D. J., and Watson, E. B. (2017). “Chapter 4. kinetic fractionation of non-traditional stable isotopes by diffusion and crystal growth reactions,” in Non-Traditional Stable Isotopes, eds F.-Z. Teng, N. Dauphas, and J. M. Watkins, (Chantilly, VA: The Mineralogical Society of America), 85–126.
Watson, E. B., and Müller, T. (2009). Non-equilibrium isotopic and elemental fractionation during diffusion-controlled crystal growth under static and dynamic conditions. Chem. Geol. 267, 111–124. doi: 10.1016/j.chemgeo.2008.10.036
Weber, M. J., and Brawer, S. A. (1982). Comparison of optical spectra and computer-simulated structure of rare-earth-doped fluoroberyllate glasses. J. Non Cryst. Solids 52, 321–336. doi: 10.1016/0022-3093(82)90307-90306
Weyer, S., and Ionov, D. A. (2007). Partial melting and melt percolation in the mantle: the message from Fe isotopes. Earth and Planetary Science Letters 259, 119–133. doi: 10.1016/j.epsl.2007.04.033
White, W. M. (1985). Sources of oceanic basalts; radiogenic isotopic evidence. Geology 13, 115–118. doi: 10.1073/pnas.1719570115
White, W. M. (1993). 238U/204Pb in MORB and open system evolution of the depleted mantle. Earth Planet. Sci. Lett. 115, 211–226. doi: 10.1016/0012-821X(93)90223-V
White, W. M., and Bryan, W. B. (1977). Sr-isotope. K, Rb, Cs, Sr, Ba, and rare-earth geochemistry of basalts from the FAMOUS area. GSA Bull. 88, 571–576.
Wood, B. J., and Blundy, J. D. (1997). A predictive model for rare earth element partitioning between clinopyroxene and anhydrous silicate melt. Contrib. Mineral. Petrol. 129, 166–181. doi: 10.1007/s004100050330
Workman, R. K., and Hart, S. R. (2005). Major and trace element composition of the depleted MORB mantle (DMM). Earth Planet. Sci. Lett. 231, 53–72. doi: 10.1016/j.epsl.2004.12.005
Keywords: MORB, IODP, non-traditional stable isotopes, Hole 735B, reactive porous flow, kinetic isotope diffusion, Rayleigh fractionation
Citation: McCoy-West AJ, Millet M-A and Burton KW (2020) The Neodymium Stable Isotope Composition of the Oceanic Crust: Reconciling the Mismatch Between Erupted Mid-Ocean Ridge Basalts and Lower Crustal Gabbros. Front. Earth Sci. 8:25. doi: 10.3389/feart.2020.00025
Received: 12 September 2019; Accepted: 24 January 2020;
Published: 19 February 2020.
Edited by:
Fang-Zhen Teng, University of Washington, United StatesReviewed by:
Gangjian Wei, Guangzhou Institute of Geochemistry (CAS), ChinaShui Jiong Wang, China University of Geosciences, China
Copyright © 2020 McCoy-West, Millet and Burton. This is an open-access article distributed under the terms of the Creative Commons Attribution License (CC BY). The use, distribution or reproduction in other forums is permitted, provided the original author(s) and the copyright owner(s) are credited and that the original publication in this journal is cited, in accordance with accepted academic practice. No use, distribution or reproduction is permitted which does not comply with these terms.
*Correspondence: Alex J. McCoy-West, YWxleC5tY2NveXdlc3RAbW9uYXNoLmVkdQ==; YWxleC5tY2NveXdlc3RAZ21haWwuY29t