- 1Section of Earth Sciences, Department of Biological, Geological and Environmental Sciences, University of Catania, Catania, Italy
- 2Research School of Earth Sciences, The Australian National University, Canberra, ACT, Australia
Inherited zircon ages and geochemical features of late Cadomian and late Variscan granitoids from the north-eastern Peloritani Mountains (NE Sicily) and the western Aspromonte Massif (SW Calabria) shed new light on the sources and processes involved in poly-orogenic granitoid magmatism. The two groups of strongly peraluminous granitoids have similarities in trace element abundance patterns, and Sr and Nd isotopic compositions consistent with both being derived from crustal sources, possibly with a minor contribution from mantle-related components. Comparison of the granite compositions with those of experimental melts derived from various metaigneous and metasedimentary sources indicates that both groups of granitoids originated exclusively from different degrees of melting of similar greywacke-dominated turbidite. Abundant inherited zircon cores from representative samples of metamorphosed late Cadomian (545 ± 5 Ma) granite and late Variscan (300 ± 4 Ma) leucogranodiorite have the same range of U–Pb ages, from Early Paleoproterozoic to latest Neoproterozoic, with main age clusters at ∼0.55 and ∼0.63 Ga, and minor age clusters at ∼0.95 and ∼2.5 Ga. The pattern of detrital zircon ages from a paragneiss, host rock to the late Cadomian granite, is the same, indicating, in conjunction with the geochemistry, that both granites originated by partial melting of deeper crustal equivalents of those paragneisses. The same crustal sequence melted during successive orogenies under different thermal regimes and in different post-collisional tectonic settings, giving rise to granitoid associations with different ages and geochemical features largely reflecting the melting conditions. On the other hand, the zircon inheritance patterns and specific chemical features of S-type granitoids reflect the nature of their crustal magma sources, independently from the effects of the thermal regime or tectonic setting at the time of magmatism.
Introduction
Following several decades during which zircon geochronology has been used to reconstruct the history of our planet and the timescales of geological processes since the formation of the first solid crust (e.g., Froude et al., 1983; Bowring and Williams, 1999; Wilde et al., 2001), increasing attention is now being paid to combining zircon age information with the mineral’s chemistry and microstructures (e.g., Vance et al., 2003; Engi et al., 2017), opening the way to the use of zircon as a powerful petrogenetic tool in the study of magmatic, metamorphic, and sedimentary systems. Zircon from igneous rocks has the capacity to preserve its isotopic composition much better than the whole rock or other mineral geochronometers. Lu–Hf and O isotopic systems in igneous zircon are widely used geochemical tracers in studying the petrogenesis of magmatic rocks and crustal evolution (e.g., Payne et al., 2016 and references therein). The analysis of age patterns of detrital and inherited zircon in metasedimentary and igneous rocks, respectively, is commonly used in provenance studies aimed at reconstructing the geodynamic evolution of continents (e.g., Nance et al., 2014 and references therein). On the other hand, zircon inheritance patterns in igneous rocks have been employed relatively little as a petrogenetic tool, despite their considerable potential to provide key information on both magma sources and petrogenetic processes (e.g., Chen and Williams, 1990; Paterson et al., 1992; Williams, 1992, 2001; Zeck and Williams, 2002; Fiannacca et al., 2008, 2013, 2017; Jeon et al., 2012; Samperton et al., 2015; Díaz-Alvarado et al., 2016; Jeon and Williams, 2018; Schaltegger and Davies, 2018).
The medium to high-grade basement exposed in the Peloritani Mountains (north-eastern Sicily) and the adjacent Aspromonte Massif (southern Calabria) is characterized by the widespread occurrence of S-type granitoids emplaced during the post-collisional stages of both the Cadomian and Variscan orogenies. The late Cadomian (∼565–540 Ma) granitoids (Micheletti et al., 2007; Fiannacca et al., 2013) were subjected to Variscan amphibolite facies metamorphism, producing augen gneisses. The late Variscan (∼314–300 Ma) granitoids (Graessner et al., 2000; Fiannacca et al., 2008) intruded into the amphibolite facies basement – mostly paragneisses derived from metamorphism of flysch-like greywacke sequences, large augen gneiss bodies, and minor amounts of mica schist, amphibolite, and marble. Both the late Cadomian and late Variscan granitoids are characterized by large amounts of inherited zircon, indicating the presence of a substantial component of metasedimentary crust in the source of the granite magmas (Fiannacca et al., 2008, 2013). In this study we use new and literature whole rock geochemical data and published inherited zircon ages of the late Cadomian and late Variscan granitoids from the Aspromonte Massif and the Peloritani Mountains to demonstrate that the same metasedimentary sequence, deposited at the northern margin of Gondwana in the latest Precambrian, melted during successive orogenies (at ∼545 and ∼300 Ma). Comparison and discussion of existing and new results from both late Cadomian and late Variscan granitoids shows that poly-orogenic melting gave rise to granitoid associations with diagnostic zircon inheritance patterns and geochemical features, reflecting the nature of their crustal magma sources independently from the effects of the thermal regime or tectonic setting at the time of magmatism. We propose that combined studies of whole rock geochemistry and zircon inheritance patterns in crustal rocks can represent a potent method for linking S-type granites to their specific magma sources.
Geo-Petrological Background
The Calabria-Peloritani Orogen (CPO; Figure 1), located at the boundary between the European and African plates in the central Mediterranean region, is an outstanding example of a poly-metamorphic basement complex, comprising remnants of Variscan and older mountain belts, locally reworked during the Alpine-Apennine orogeny (Cirrincione et al., 2015 and references therein). Rocks metamorphosed during the Variscan orogenic cycle record deep-crustal conditions, with pressures up to 1.1 GPa in the Serre Massif, central Calabria (Acquafredda et al., 2008), and up to 0.9 GPa in the Peloritani Mountains (Fiannacca et al., 2012). In particular, peak conditions of 0.9 GPa at ∼530°C, and 0.8 GPa at ∼600°C were obtained by Fiannacca et al. (2012) and Ortolano et al. (2014), respectively, by thermodynamic modeling of garnet phyllites and schists from the Mandanici Unit and the overlying Aspromonte Unit. Other P-T estimates for the Variscan metamorphism obtained from the Aspromonte Unit in the Peloritani Mountains are ∼0.5 GPa at ∼550–680°C (Ioppolo and Puglisi, 1989; Rotolo and De Fazio, 2001); peak conditions estimated in the central Aspromonte Massif are similar, 0.4–0.5 GPa at 650–675°C (Ortolano et al., 2005). High-temperature metamorphism led to anatexis in large portions of the Aspromonte Unit, mostly producing widespread metasedimentary metatexites. No clear metamorphic gradient can be traced in the field, but migmatites tend to be more abundant in the northernmost part of the Peloritani Mountains and in the western Aspromonte Massif.
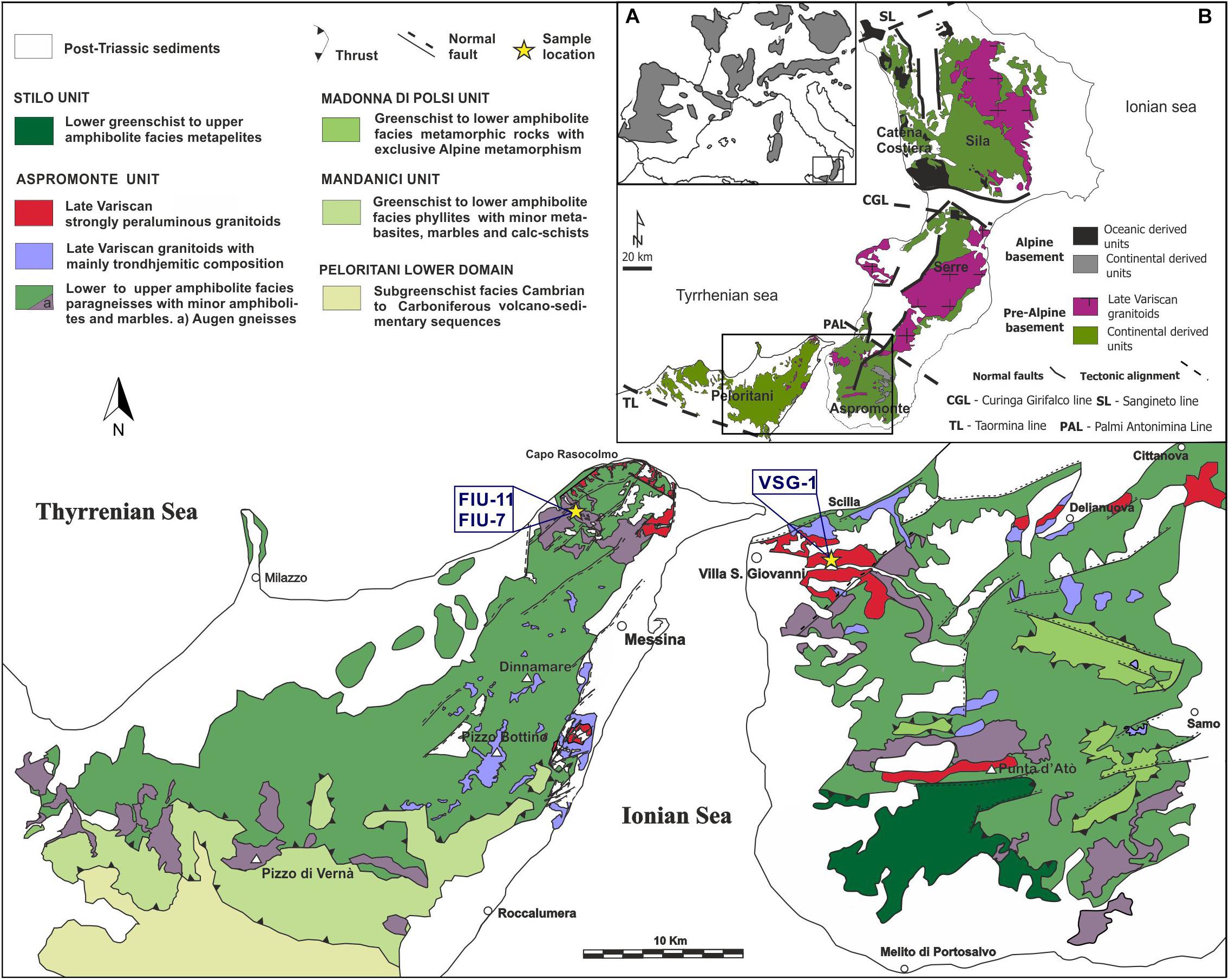
Figure 1. Geological sketch map of the western Aspromonte Massif and north-eastern Peloritani Mountains showing the main tectonic units and the distribution of the late Cadomian granitoids (now augen gneisses) and of the late-Variscan granitoids (modified after Fiannacca et al., 2019). (A) Distribution of pre-Alpine basement rocks in Western Europe (modified after von Raumer et al., 2013). (B) Distribution of Alpine and pre-Alpine basement rocks in the Calabria-Peloritani Orogen and main tectonic alignments (modified after Angì et al., 2010).
After the thickening-related baric peak, scattered small late Carboniferous (∼314–300 Ma) early post-collisional plutons of weakly to strongly peraluminous leucotonalite to leucogranite were emplaced (Rottura et al., 1993; Graessner et al., 2000; Fiannacca et al., 2005, 2008, 2019). These were followed by batholith-scale magmatism at 300–290 Ma (Rottura et al., 1990; Del Moro et al., 2000b; Langone et al., 2014; Fiannacca et al., 2015, 2017) and finally by late- to post-orogenic rhyolitic to andesitic dykes (Festa et al., 2010; Romano et al., 2011, 2012), heralding the early breakup of Pangea (Barca et al., 2010; Cirrincione et al., 2014, 2016).
The Peloritani Mountains and Aspromonte Massif, which comprise the southern termination of the CPO, consist of a stack of south-verging Alpine nappes formed mainly by pre-Alpine metamorphic rocks (Ogniben, 1960, 1973; Cirrincione et al., 2015 and references therein). The late Variscan granitoids in the area occur only as small plutons that intrude, with sharp discordant contacts, only the metamorphic rocks from the Aspromonte Unit – amphibolite facies paragneisses, migmatites, and augen gneisses, with minor amphibolites and marbles. In particular, the Villa S. Giovanni granitoids, in the Aspromonte Massif make up a large and homogeneous magmatic body, while the Capo Rasocolmo granitoids, on the opposite side of the Messina Strait, are exposed as a multitude of smaller bodies and dykes, meter to decameters in size, possibly representing the roof sector of the same pluton (D’Amico et al., 1973). The dominant host rocks in both areas are migmatitic paragneisses. Most of the granitoids have a dominant strongly peraluminous leucogranodioritic composition and probably reflect crustal partial melting associated with early post-collisional decompression (e.g., Rottura et al., 1990; Fiannacca et al., 2008). Alternatively, it has been argued that mixing with mantle-derived magmas is required to explain some of their geochemical and isotopic features (Rottura et al., 1993). Recent geochronological studies have shown that pre-Variscan igneous rocks produced during the latest stages of the Cadomian Orogeny are more widespread in the CPO than previously thought, and have highlighted the presence of a relatively large province of post-collisional, weakly to strongly peraluminous, late Neoproterozoic (Ediacaran) granitoids, mostly emplaced at ∼545 Ma (Micheletti et al., 2007, 2008; Williams et al., 2012; Fiannacca et al., 2013). The host rocks of the late Cadomian granites (now augen gneisses) in the northern Peloritani Mountains are mostly migmatitic paragneisses, both rock types exhibiting the same schistosity. Contacts between augen gneisses and paragneisses are also parallel, although the original field relationships are difficult to determine due to intense tectonic and metamorphic reworking during the subsequent Variscan and Alpine orogenies, the former turning the Cadomian granites into upper amphibolite facies augen gneisses.
Major element, trace element and Sr–Nd isotopic compositions, together with zircon inheritance age patterns, suggested that the granitoid protoliths of these augen gneisses were produced by varying degrees of mixing between magmas derived from supracrustal and mantle sources (Fiannacca et al., 2013 and references therein). In particular, the granitoids rich in inherited zircon would reflect a significant contribution from the partial melting of the paragneisses that are the dominant regional lithology in the Aspromonte Unit. The small difference between the assumed deposition age of the greywacke protolith of the paragneisses and the emplacement age of the granitoids has been interpreted to indicate rapid crustal recycling involving successive erosion, burial and metamorphism up to partial melting followed by extensive granitoid magmatism in less than ∼10 Ma (Fiannacca et al., 2013).
According to many authors (e.g., Figure 7 in Nance et al., 2010 and references therein), following late stage Cadomian magmatism at ∼580–560 Ma, collision of the Cadomian arc with the northern Gondwana margin at ∼545–540 Ma led to deposition of greywacke-dominated turbidite sequences in the intervening back-arc basin (e.g., Lausitz Group in Saxo-Thuringia; Linnemann et al., 2007, 2008). During closure of the back-arc basin, those greywackes were rapidly buried, deformed and then intruded, at ∼540 Ma, by large volumes of granitoid magma, probably derived from partial melting of the same turbidites. Nance et al. (2010) proposed that such latest Neoproterozoic processes of rapid crustal recycling were the result of a short-lived regime of high heat-flow connected to slab break-off, in a context of ridge-trench collision.
Furthermore, as suggested by Schulmann et al. (2002), thinned and thermally softened intra-continental back-arc domains are particularly susceptible to crustal thickening during collision and may achieve high temperatures as a consequence of thermo-rheological processes with no need to involve a mantle heat source. An analogous peri-Gondwanan back-arc setting between a magmatic arc and a north-eastern segment of the African craton has been envisaged for the northern Peloritanian terrane during the latest Precambrian (Williams et al., 2012; Fiannacca et al., 2013). The Ediacaran granitoids originating from this rapid crustal evolution were metamorphosed during the Variscan orogeny and transformed into the amphibolite facies augen gneisses that, together with the small late Variscan granitoid plutons, and the dominant paragneisses, form the bulk of the Aspromonte Unit.
The late Variscan granitoids were then produced, after the final collision and merging of Gondwana, the peri-Gondwanan terranes and Laurussia, to form Pangea at ∼300 Ma. Large-scale crustal melting and granitoid magmatism at 320–280 Ma throughout the southern Variscan terranes probably resulted from the transition from a compressional to a transpressional/transtensional regime, locally associated with crustal thinning and asthenospheric upwelling (von Raumer et al., 2013 and references therein).
Materials and Methods
With the aim of identifying possible relationships between the granites and their sources, U–Th–Pb data from inherited zircon from two representative inheritance-rich granitoid samples, including a late Variscan leucogranodiorite (VSG-1; Fiannacca et al., 2008) and a late Cadomian granite (sample FIU-11; Fiannacca et al., 2013), were compared with detrital zircon from a paragneiss (FIU-7; Williams et al., 2012) that is the host rock of the late Cadomian granite and represents the dominant rock type in the Aspromonte Unit of the Peloritani Mountains and Aspromonte Massif. In addition, whole rock major and trace element compositions of 15 samples of late Cadomian and late Variscan granites from the northern Peloritani Mountains and the western Aspromonte Massif are presented in combination with previously published data from the same rock types from the same areas published by previous authors (e.g., Puglisi and Rottura, 1973; Messina et al., 1974; Atzori and Lo Giudice, 1982; Rottura et al., 1993; Fiannacca et al., 2008, 2013 and references therein). The whole rock compositions, reported in Supplementary Table S1, were plotted using the GCDkit program (Janoušek et al., 2006).
Whole-rock major and selected trace element contents for the new samples were obtained by X-ray fluorescence (XRF) on pressed-powder pellets at the Department of Biological, Geological and Environmental Sciences of the University of Catania, Italy. Volatile contents were measured as weight loss on ignition (LOI) by standard gravimetric methods after drying the rock powders for several hours at 110°C, then for 6 h in a furnace at 950°C. XRF analyses were carried out on a Philips PW 2404 spectrometer equipped with an Rh target. The average relative standard deviations were less than 5%. The analyses were quantified using a calibration line based on 45 international rock standards. The accuracy is better than 3% for major elements and better than 5% for trace elements with concentrations well above the detection limits.
Detailed information about the SHRIMP (Sensitive High Resolution Ion Microprobe) zircon dating method and obtained results, together with full descriptions of the features of the zircon from the rocks studied can be found in Fiannacca et al. (2008, 2013) and Williams et al. (2012). Extensive geochemical characterization of the late Cadomian and late Variscan granites is reported in Fiannacca et al. (2013) and Rottura et al. (1993), respectively. In the following only the main general features and the data relevant to the present study are presented and discussed.
Results
Whole Rock Geochemistry
The late Cadomian and late Variscan granitoids dominantly consist of strongly peraluminous granodiorites and granites, with subsidiary tonalites in the Variscan rocks (Figure 2A). The peraluminousity index [A/CNK = molar Al2O3/(CaO + Na2O + K2O)] shows a weak negative correlation with SiO2 for the late Cadomian granites (a common feature of S-type granites), but defines no discernible trend for the late Variscan granitoids (Figure 2B). The late Cadomian granitoids are significantly more enriched in FeOt, MgO, and TiO2 (not shown), Y and Zr, and less enriched in SiO2, Al2O3, CaO, and Sr (Figure 3). TiO2, MgO, FeOt, and Al2O3 are negatively correlated and Rb is positively correlated with SiO2 for all the granitoids, while only the late Variscan samples show well defined negative correlations for CaO, Sr, Zr, La, and Th and a positive correlation for K2O. Yttrium exhibits a poorly defined negative correlation in both granitoid populations.
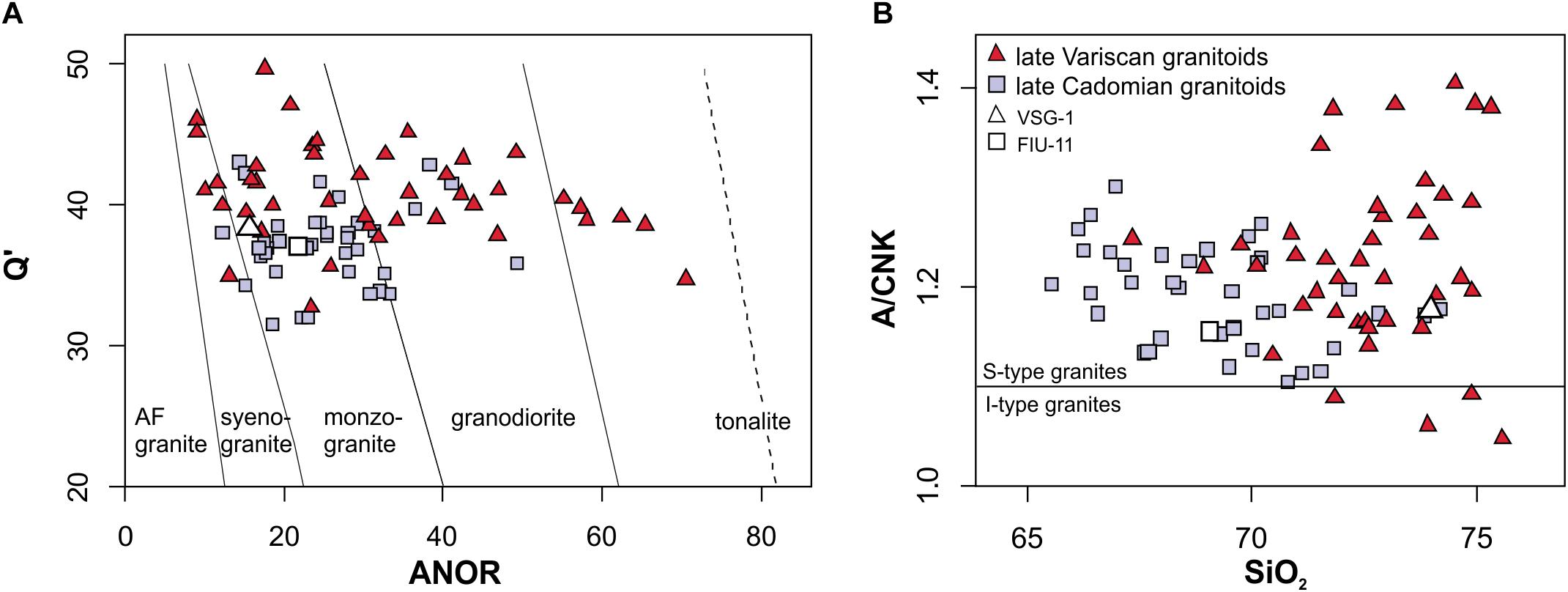
Figure 2. (A) CIPW normative Q’-ANOR classification diagram Q’ = 100∗Q/(Q + Or + Ab + An); ANOR = 100 × [An/(Or + An)] after Streckeisen and Le Maitre, 1979) and (B) A/CNK vs. SiO2 diagram for the studied granitoids. Unfilled symbols refer to the two dated samples from Fiannacca et al. (2008; triangle) and Fiannacca et al. (2013; square).
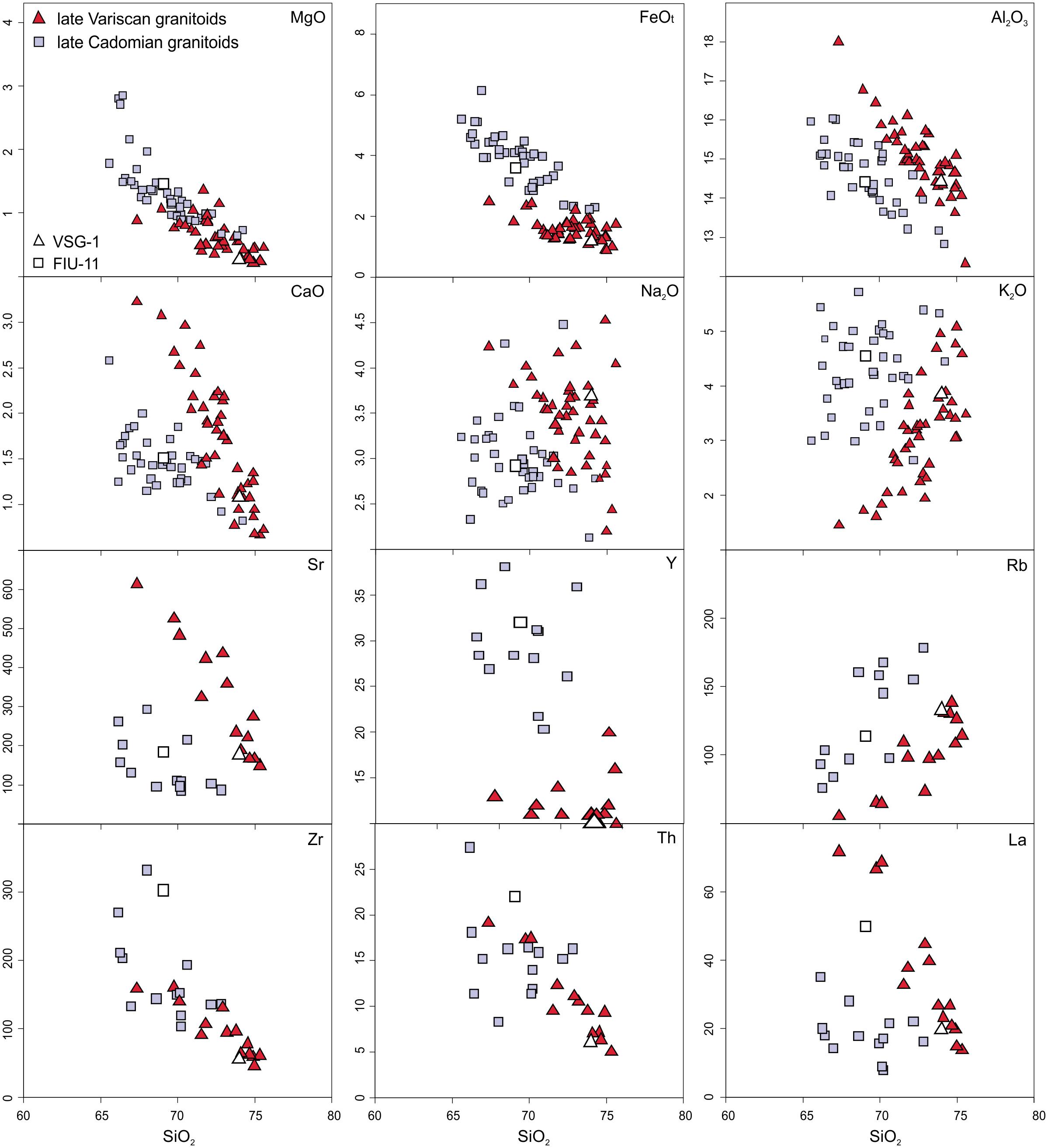
Figure 3. Variation diagrams for selected major and trace elements. Oxides in wt. %, trace elements in ppm.
The two dated granitoid samples have similar patterns in multi-element diagrams (Figure 4), except for the late Variscan leucogranodiorite VSG-1 displaying a lower trace element content than the late Cadomian granite (FIU-11), except for Nb, Sr, and P. The most striking feature of the plots, however, is the remarkable similarity in all trace elements, including the REE, between the late Cadomian granite and its host paragneiss (FIU-7). Sr and Nd isotopic compositions recalculated to the emplacement age of the late Variscan granitoids are shown in Figure 5.
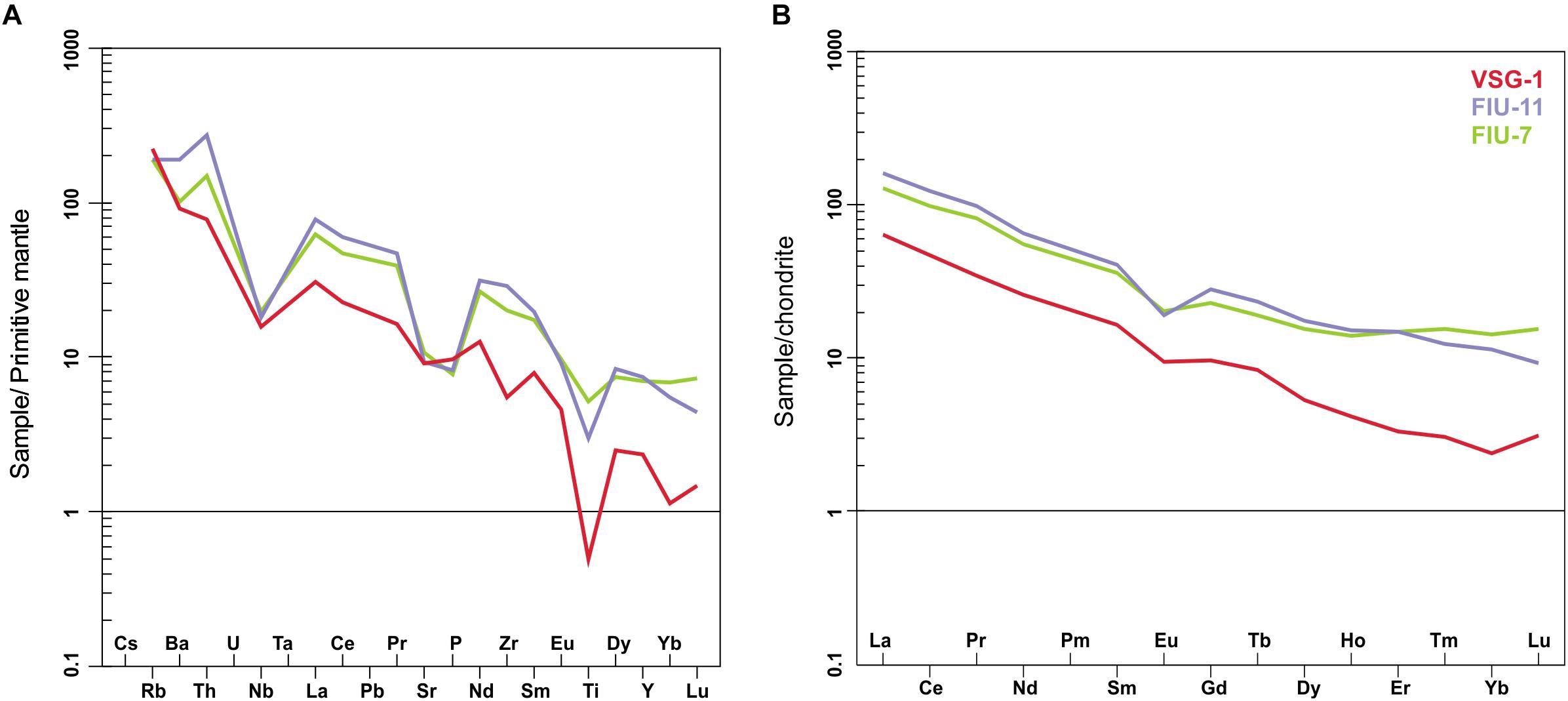
Figure 4. Primitive mantle-normalized trace element patterns (A) and chondrite-normalized REE patterns (B) for the late Variscan leucogranodiorite (VSG-1; Fiannacca et al., 2008), the late Cadomian granite FIU-11 (Fiannacca et al., 2013) and the paragneiss FIU-7 (Williams et al., 2012). Normalization values are from McDonough and Sun (1995) for primitive mantle and from Boynton (1984) for chondrite.
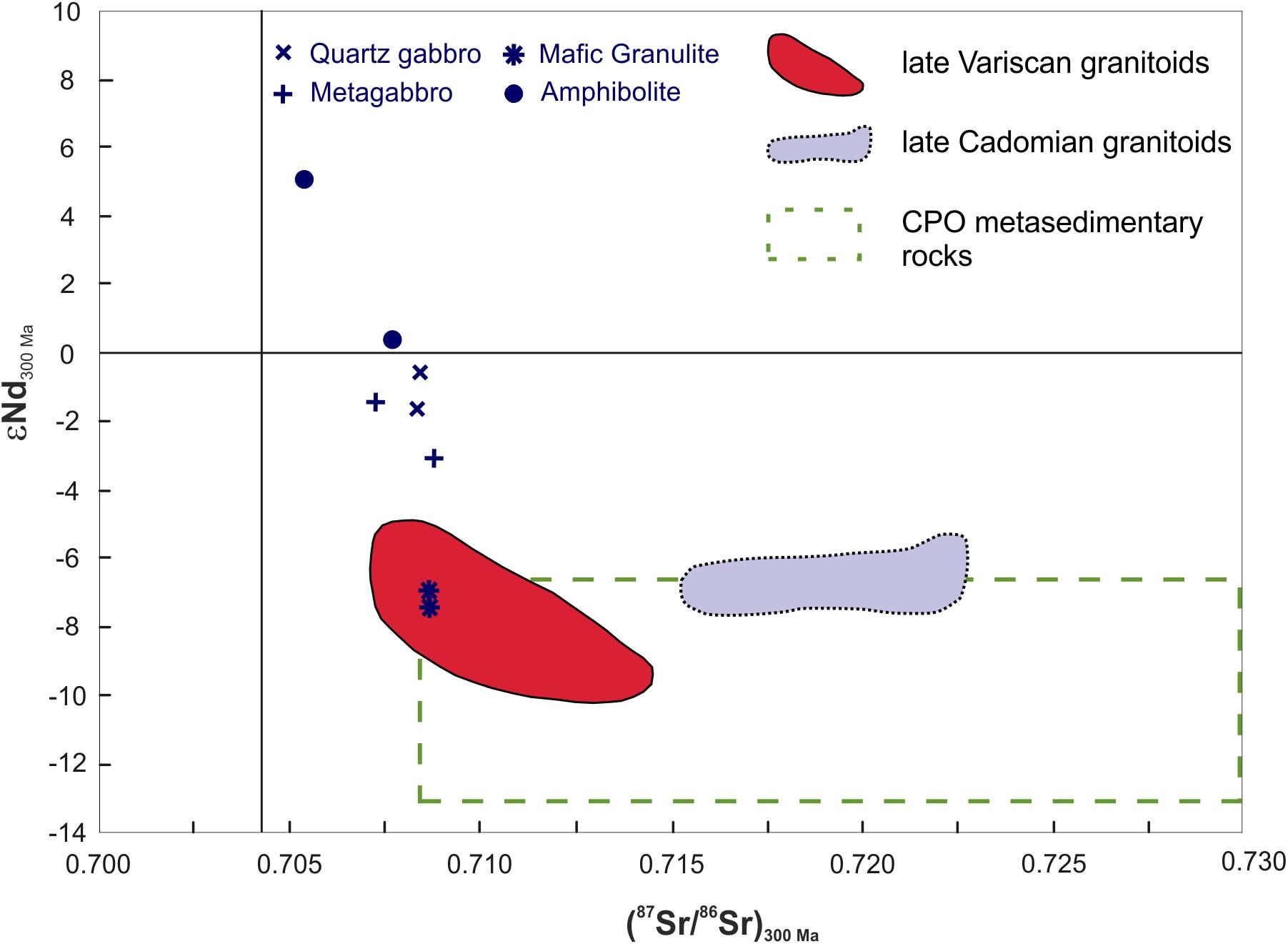
Figure 5. εNd vs. (87Sr/86Sr)300 Ma diagrams for the studied granitoid rocks. Field of CPO strongly peraluminous granitoids after Rottura et al. (1991, 1993). Field of the CPO late Cadomian granitoids after Fiannacca et al. (2013) and reference therein. Serre Massif metagabbros and mafic granulites after Caggianelli et al. (1991); Quartz gabbro after Rottura et al. (1989); Peloritani Mts amphibolites after Fiannacca (2000). Field of CPO metasedimentary rocks after Rottura et al. (1989); Caggianelli et al. (1991); Fiannacca et al. (2005), and Williams et al. (2012).
In particular, an age of 300 Ma has been considered for recalculation based on the U–Pb zircon emplacement age of 300.2 ± 3.8 Ma obtained for the Villa S. Giovanni leucogranodiorite VSG-1 by Fiannacca et al. (2008); U–Pb monazite emplacement ages of ∼303–302 Ma were also obtained by Graessner et al. (2000) for other strongly peraluminous late Variscan granitoids from the Aspromonte Massif.
In Figure 5, both the late Cadomian and the late Variscan granitoids show strongly negative εNd values and evolved initial Sr isotopic compositions. The initial Sr–Nd compositions of all the granitoids are close to those of the metasedimentary rocks from the CPO; on the other hand, some of the granitoids plot along possible mixing trends between metasediment-derived and mantle-derived magmas, leading some authors to propose a mantle contribution to the generation of the original granitoids (see discussions in Fiannacca et al., 2008, 2013).
Zircon Geochronology
Supplementary Figure S1 shows the main textural features of the zircon from samples VSG-1, FIU-11, and FIU-7, dated by Fiannacca et al. (2008, 2013) and Williams et al. (2012) – large inherited zircon cores of a range of ages are overgrown discordantly by melt-precipitated zircon, mostly with weak growth zoning.
An emplacement age for the late Variscan leucogranodiorite VSG-1 of 300 ± 4 Ma was obtained by SHRIMP U–Pb zircon dating of melt-precipitated igneous zircon occurring as core-free grains or overgrowths (Fiannacca et al., 2008). Much of the igneous zircon occurs as thick overgrowths on older cores, indicating that the cores represent detrital zircon inherited from the magma source, rather than accidental xenocrysts incorporated in the ascending magma. The inherited cores range in age from Early Paleoproterozoic to latest Neoproterozoic, with main clusters at ∼0.55 and 0.63 Ga and minor ones at ∼0.90 and ∼2.4 Ga.
The emplacement age of the late Cadomian granite protolith of the augen gneiss is 545 ± 5 Ma, based on SHRIMP U–Pb zircon dating of core-free grains and overgrowths (Fiannacca et al., 2013). Similarly to the zircon in the late Variscan leucogranodiorite VSG-1, the zircon cores in the augen gneiss are surrounded by thick igneous overgrowths – they are therefore also interpreted as inherited zircon. The age of the inherited cores ranges from Early Paleoproterozoic to latest Neoproterozoic, with main clusters at ∼0.55 and 0.63 Ga and minor ones at ∼0.95 and ∼2.5 Ga (Williams et al., 2012; Fiannacca et al., 2013). The interpreted deposition age of the greywacke protolith of the paragneiss is ∼545 Ma, bracketed by the combination of the age of the youngest detrital zircon population (545 ± 7 Ma), the emplacement ages of the virtually coeval granitoid magmas that intruded the metagreywackes (545 ± 5 Ma), and the age of the zircon overgrowths surrounding the detrital zircon cores in the paragneiss (536 ± 8 Ma) (Williams et al., 2012). All zircon grains consist of a large core, partially or totally overgrown by a thin metamorphic rim (<10 μm) marking the end of the Cadomian orogenic cycle in the region, at 535.9 ± 8.0 Ma. The age of the detrital zircon cores ranges from Early Paleoproterozoic to latest Neoproterozoic with main clusters at ∼0.55 and ∼0.63 Ga and minor ones at ∼0.8, 0.95, and ∼2.5 Ga.
Relative probability density plots of SHRIMP U–Pb ages for zircon cores from the different samples are reported in Figure 6. Data refer to 56 zircon detrital cores from paragneiss FIU-7, 24 inherited cores from late Cadomian granite FIU-11, and 11 inherited cores from late Variscan leucogranodiorite VSG-1. A feature to be highlighted is the close correspondence between the ages of the inherited zircon cores from both granitoid rocks and the ages of the detrital zircon in the paragneiss sample.
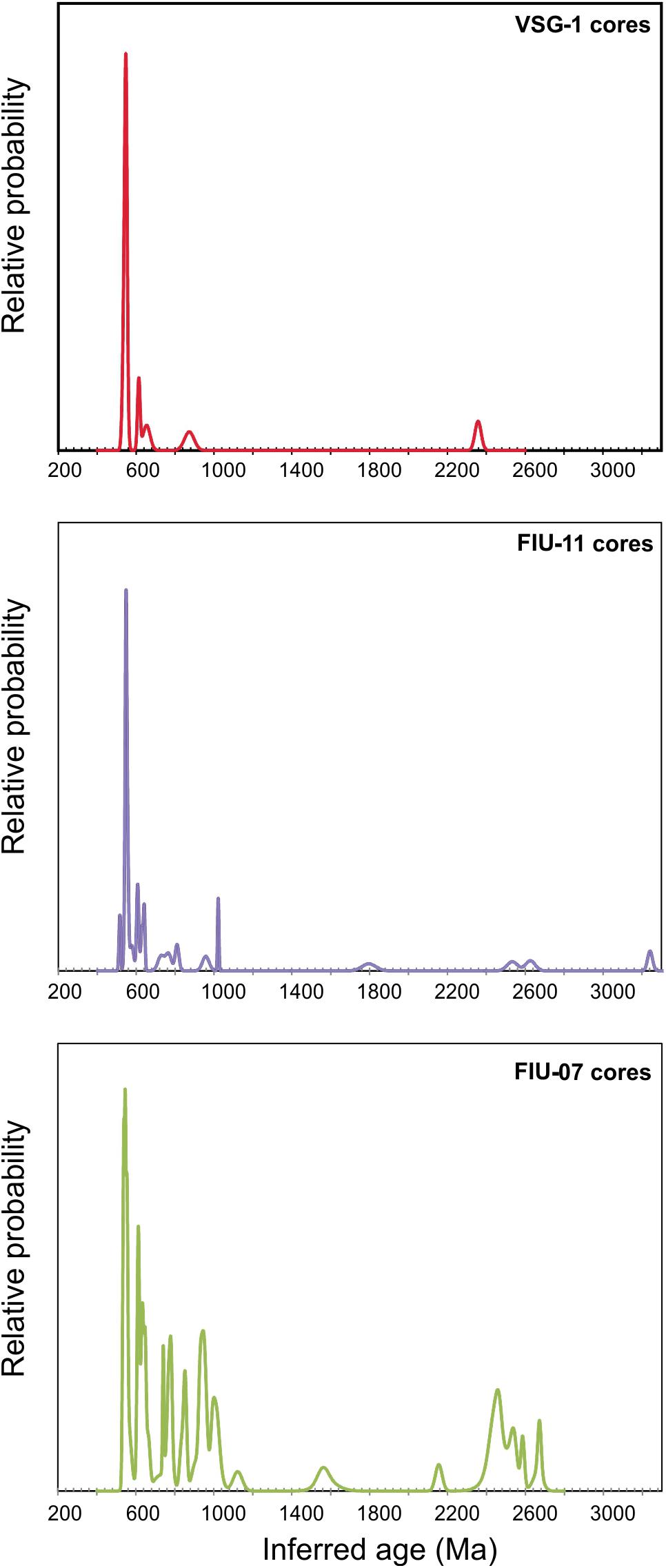
Figure 6. Relative probability density distributions of the ages for the zircon cores of the late Variscan leucogranodiorite (VSG-1), the late Cadomian granite (FIU-11) and the paragneiss (FIU-7) (modified after Fiannacca et al., 2008, 2013; Williams et al., 2012).
Discussion and Conclusion
The granitoid rocks emplaced into the amphibolite facies basement of the Aspromonte Unit during the latest stages of the Cadomian and Variscan orogenic cycles have strongly peraluminous compositions and similar patterns in primitive mantle- and chondrite-normalized multi-element diagrams, indicative of similar sources. In particular, the metamorphosed late Cadomian granite, now augen gneiss, has element abundance patterns almost identical to those of its host paragneiss, apart from slightly lower contents of Ti and Yb–Lu. These patterns are consistent with derivation of the augen gneiss protolith by high degrees of melting of a paragneiss-like source, with retention of these elements in residual mafic phases; indeed, trace element patterns nearly identical to those of a metagreywacke source, with only slight variations in individual trace element contents, have been observed in granitoids produced by at least 20–40% partial melting of metagreywackes (e.g., Otamendi and Patiño Douce, 2001). Very high melt proportions, of 80–90 vol. %, have been proposed by Fernández et al. (2008), in order to account for such strong similarity in trace element contents of granites and their assumed metasedimentary source rocks. The late Variscan sample also shows similar patterns, which are however, slightly more fractionated and characterized by lower abundances of nearly all the plotted trace elements. This may reflect the more evolved nature of the late Variscan granite sample, having a SiO2 content of 74 wt. %, compared to 69 wt. % for the late Cadomian granite. This interpretation would be supported by the correlations in the late Variscan granites revealed in the Harker diagrams (Figure 3), which show decreasing contents in elements such as Zr, Th, and La with increasing SiO2 contents, consistent with fractionation of accessory phases rich in those elements, such as zircon, monazite, and apatite. However, it is also possible that a lower degree of melting, as well as a higher extent of disequilibrium melting in generating the late Variscan granites could have played a role in producing slightly different patterns.
Recalculated Sr–Nd isotopic compositions for both granite suites indicate that melting of crustal rocks played a dominant role in their generation. The isotopic compositions are in fact close to those of the CPO metasediments, including the Peloritanian metagreywackes, although not matching the sediment compositions exactly in several cases. Their position in the εNd vs. (87Sr/86Sr)300 Ma diagram (Figure 5) is consistent with either involvement of mantle-related components in the genesis of the granitoids, or with disequilibrium melting of the metasedimentary rocks. It is possible, in fact, for granitoids to have lower (87Sr/86Sr)i and less negative εNd than their crustal sources as a consequence of isotopic disequilibrium during crustal partial melting (e.g., Del Moro et al., 2000a; Knesel and Davidson, 2002; Zeng et al., 2005; Farina and Stevens, 2011; Farina et al., 2014). This process has been documented in metasedimentary migmatites from the Serre Massif, in central Calabria, hosting leucosomes characterized by Sr–Nd isotopic compositions not matching those of the associated meso-melanosomes and some of which plotting even outside the metasedimentary field (Del Moro et al., 2000a).
Considering the possibility of mantle involvement, together with the respective tectonic settings of the two granite generations, a back-arc setting with a regime of high heat-flow associated to slab break-off, as proposed for the late Cadomian granites, appears more suitable for the production of hybrid magmas than the post-collisional setting of the late Variscan granites. In particular, according to Fiannacca et al. (2013) a mantle contribution could be envisaged for the late Cadomian granites from the western Peloritani Mountains because of their relatively low inherited zircon content, slightly less negative εNd values, metaluminous to weakly peraluminous compositions and associated lack of monazite and muscovite. On the other hand, this contribution would be negligible to totally absent in those samples with abundant zircon inheritance and with compositions fitting more closely derivation from a metasedimentary source, such as the late Cadomian granites from the northern Peloritani.
As for the late Variscan granites, mantle-derived magmas are mostly considered to have contributed only the heat necessary to partially melt the lower crustal metaigneous and metasedimentary source rocks of the ∼300–290 Ma Serre Batholith (e.g., Fiannacca et al., 2015, 2017), in a context of lithospheric thinning and basaltic magma underplating following the earlier stages of orogenic decompression. Decompression alone, with no heat input from basaltic magma, is considered to have produced the slightly older (304–300 Ma), small, strongly peraluminous plutons by partial melting of metasediments (e.g., Caggianelli et al., 2003; Fiannacca et al., 2008).
In the AB diagram of Villaseca et al. (1998) (Figure 7) both the late Cadomian and late Variscan granites plot within the field of S-type granites. The late Variscan granites mostly plot as highly “felsic peraluminous,” typical of melts generated by low-degree partial melting of various crustal rocks (e.g., Villaseca et al., 1998 and references therein). One group of samples plots along the trend defined experimentally by progressive melting of metagreywacke-metapelite, but still with low contents of mafic components (Fe + Mg + Ti ≤ 50) consistent with less than 20% melting. The late Cadomian granites plot almost exclusively in the field of the “highly peraluminous granitoids” along trends representative of progressive melting of metagreywacke sources, with melt fractions greater than 20%.
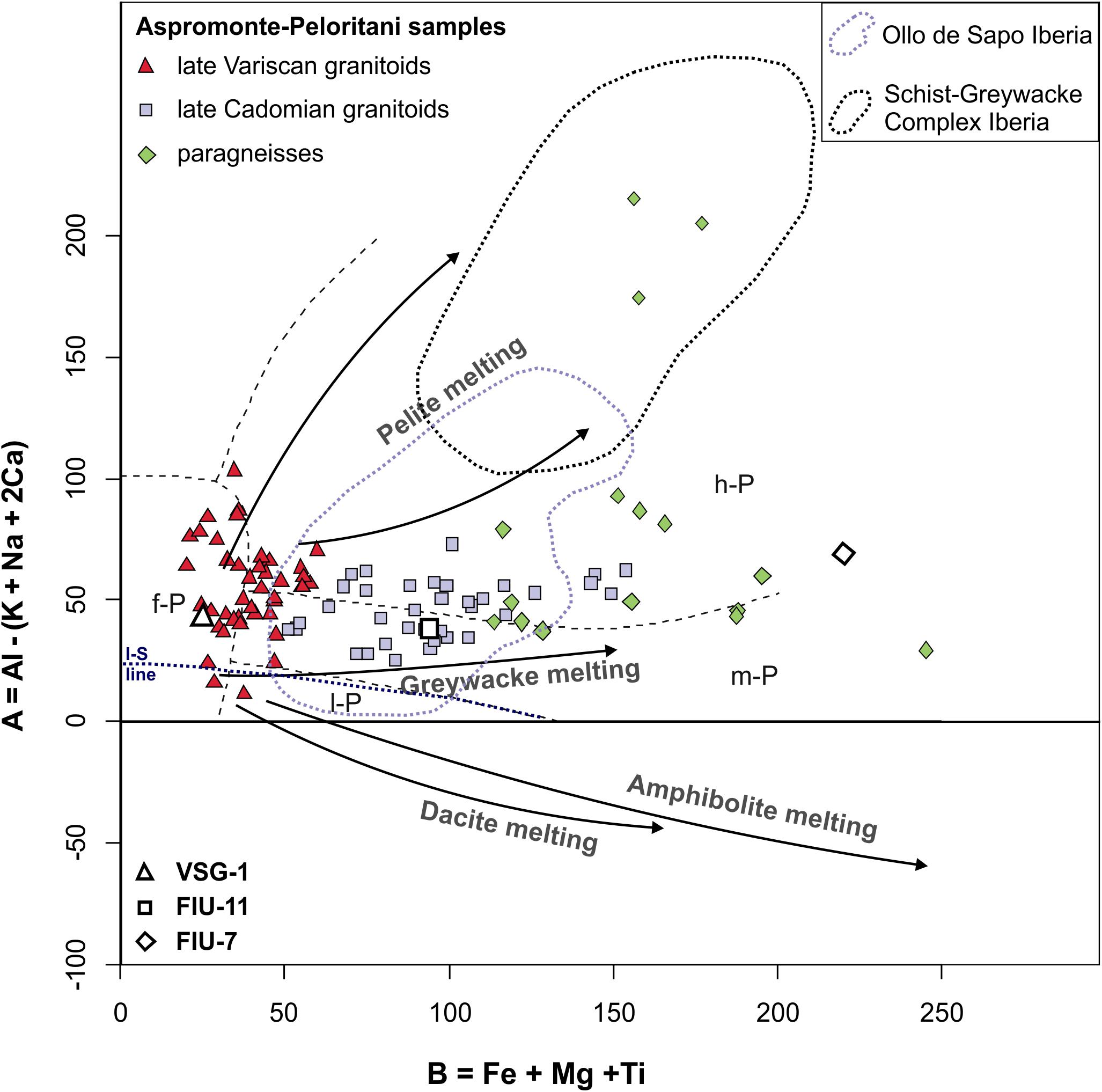
Figure 7. AB diagram (Villaseca et al., 1998; after Debon and Le Fort, 1983) for the studied rocks. The arrows represent the compositions of progressive melt fractions produced by experimental melting of different crustal rocks (Villaseca et al., 1998 and references therein). Peloritani paragneisses after Fiannacca (2000) and Atzori et al. (2003). Fields of Iberian Ollo de Sapo ferrosilic granitoids and Schist-Greywacke Complex after Atzori et al., 2003.
It is important to note that the observed trends show an increase in peraluminousity with increasing maficity. This is typical of S-type granites, but opposite to the case where the increase in maficity is due to the involvement of either metaluminous metabasic sources, or coeval basaltic magmas, in the production of the granitoid magmas. As a consequence, the more mafic granitoid compositions here are considered to reflect either a higher degree of partial melting, involving consumption of more of the mafic phases in the source, or the mixture of melt with higher proportions of restitic/peritectic phases entrained from the source (e.g., Chappell et al., 1987; Stevens et al., 2007). In this respect, it is relevant to note that it is the compositions of the more mafic granitoids that are closest to that of paragneiss FIU-7, and of the paragneisses from the north-eastern Peloritani in general, reflecting the view that the most mafic rocks of a peraluminous suite are those that best image their source (e.g., Chappell et al., 1987; Chappell and White, 1992).
Additional inferences can be drawn by plotting the compositions of the studied rocks in the CaO/Na2O vs. Al2O3/TiO2 diagram by Sylvester (1998). In Figure 8, the late Cadomian and late Variscan granites display exactly the same range of CaO/Na2O ratios, but different ranges of Al2O3/TiO2 ratios, with the late Cadomian granites characterized by significantly lower values. The CaO/Na2O is an index of source composition, with values decreasing from plagioclase-rich/clay-poor to plagioclase-poor/clay-rich sources. Values in the range of 0.3–0.9, as observed for the studied granitoids, are consistent with melting of meta-greywackes at pressures of 0.5–2.0 GPa. On the contrary, melting of plagioclase-poor pelites at similar pressures (0.7–1.3 GPa) produces granitoid magmas with CaO/Na2O < 0.3. On the other hand, the Al2O3/TiO2 ratio is an index of the melting temperature, with low and high Al2O3/TiO2 ratios characteristic of strongly peraluminous granites produced at higher and lower temperatures, respectively. Therefore, the late Cadomian granites formed by melting of the same meta-greywacke source as the late Variscan granites, but at higher temperatures. This interpretation is consistent with the high content in ferromagnesian components observed in Figure 7 and reinforces the possibility that the most mafic late Cadomian granitoids resulted from particularly high degrees of melting, as proposed for the Cambro-Ordovician ferrosilicic magmatism defined in the central Iberian Massif (Fernández et al., 2008; Castro et al., 2009; Díaz-Alvarado et al., 2016). The CPO late Cadomian granitoids show indeed many geochemical features of the high-silica ferrosilicic magmatic rocks, as defined by Castro et al. (2009), such as high FeO and MgO contents (FeOt > 2.5 wt. % and MgO > 0.8 wt. %) associated to low CaO contents (CaO < 2.0 wt. %) and peraluminous compositions; they also show a similar distribution in the A-B diagram (Figure 7), with B values (Fe + Mg + Ti) in the range 50–150. Compared to the rocks from the Iberian Massif, the studied late Cadomian granites are on average less peraluminous, but this difference turns out to be further evidence of the source-granite link that can be illustrated by the variation trends in the A–B diagram. In fact, the S-type meta-magmatic rocks from the Iberian Massif define a steep trend pointing to their strongly peraluminous sources – the metasediments from the Schists and Greywacke Complex, in the same way the late Cadomian granites from the Peloritani Mountains define a less steep trend, pointing exactly to the less peraluminous Peloritani paragneisses.
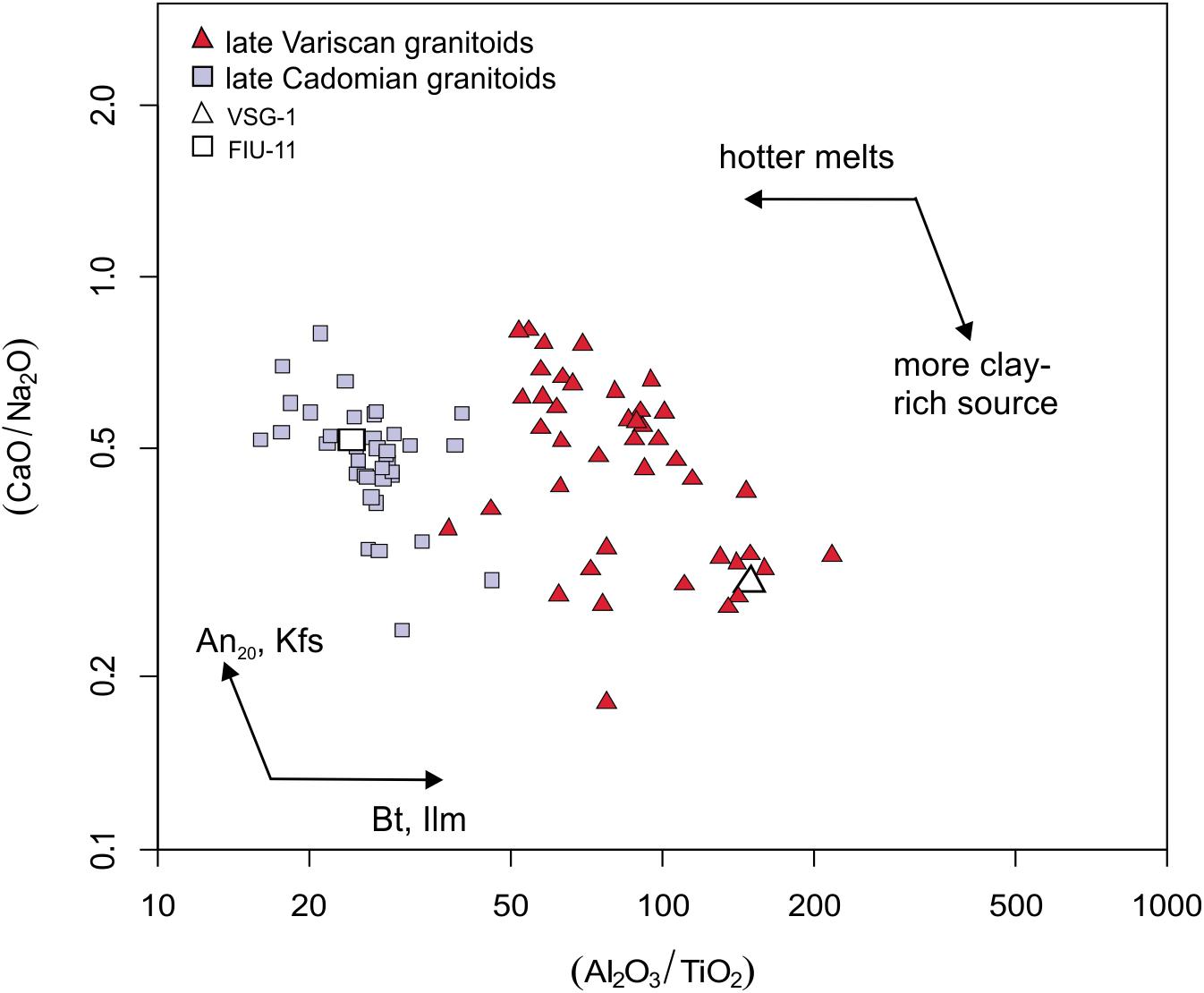
Figure 8. CaO/Na2O vs. Al2O3/TiO2 ratios for the studied granitoids. Vectors for fractional crystallization of oligoclase (An20), K-feldspar (Kfs), biotite (Bt) and ilmenite, and for illustrating compositional variations with increasing melting temperature and clay components in the source, are from Sylvester (1998 and references therein).
These observations apply better to granites generated by relatively high degrees of partial melting. Indeed, the source signal recorded by the late Variscan granites is less strong, as typical for low-temperature granitoids with near-minimum melt compositions, which tend to define vertical trends, or no defined trend, in the A–B diagrams (Villaseca et al., 1998).
On the other hand, according to Fernández et al. (2008) and Castro et al. (2009), the high Fe and Mg contents of ferrosilicic magmas require more than 80% melting of metasediments at temperatures higher than 1000°C; the same authors have proposed that these conditions were achieved in a cold diapir, consisting of subducted turbiditic sediments ascending across the hot mantle-wedge. Alternatively, highly mafic compositions can also reflect entrainment of large amounts of restitic/peritectic ferromagnesian minerals from the source, at lower temperature (e.g., Chappell et al., 1987; Stevens et al., 2007).
A final constraint on the source-granite link can be obtained by using the projection from biotite of the Shand (1943) devised by Moyen et al. (2017) as a powerful method for identifying the different sources involved in the generation of granitoid rocks, minimizing the effect of differentiation (Figure 9). In this diagram, all melts from a same source define a tight array, with a slope that depends exclusively on the nature of the source. As is evident in Figure 9, both the late Variscan and late Cadomian granitoids plot above the line Al = 3Ca + (Na + K), which separates the melts related to mafic igneous sources from the “truly” peraluminous melts related to felsic igneous rocks or meta-sediments. In particular, both studied granitoids define a single array, with a slope consistent with derivation from a common source composed of felsic igneous rocks or immature metasediments, such as metagreywackes. In this regard, it is no coincidence that, toward the mafic end, the array points exactly to the composition of metagreywacke sample FIU-7.
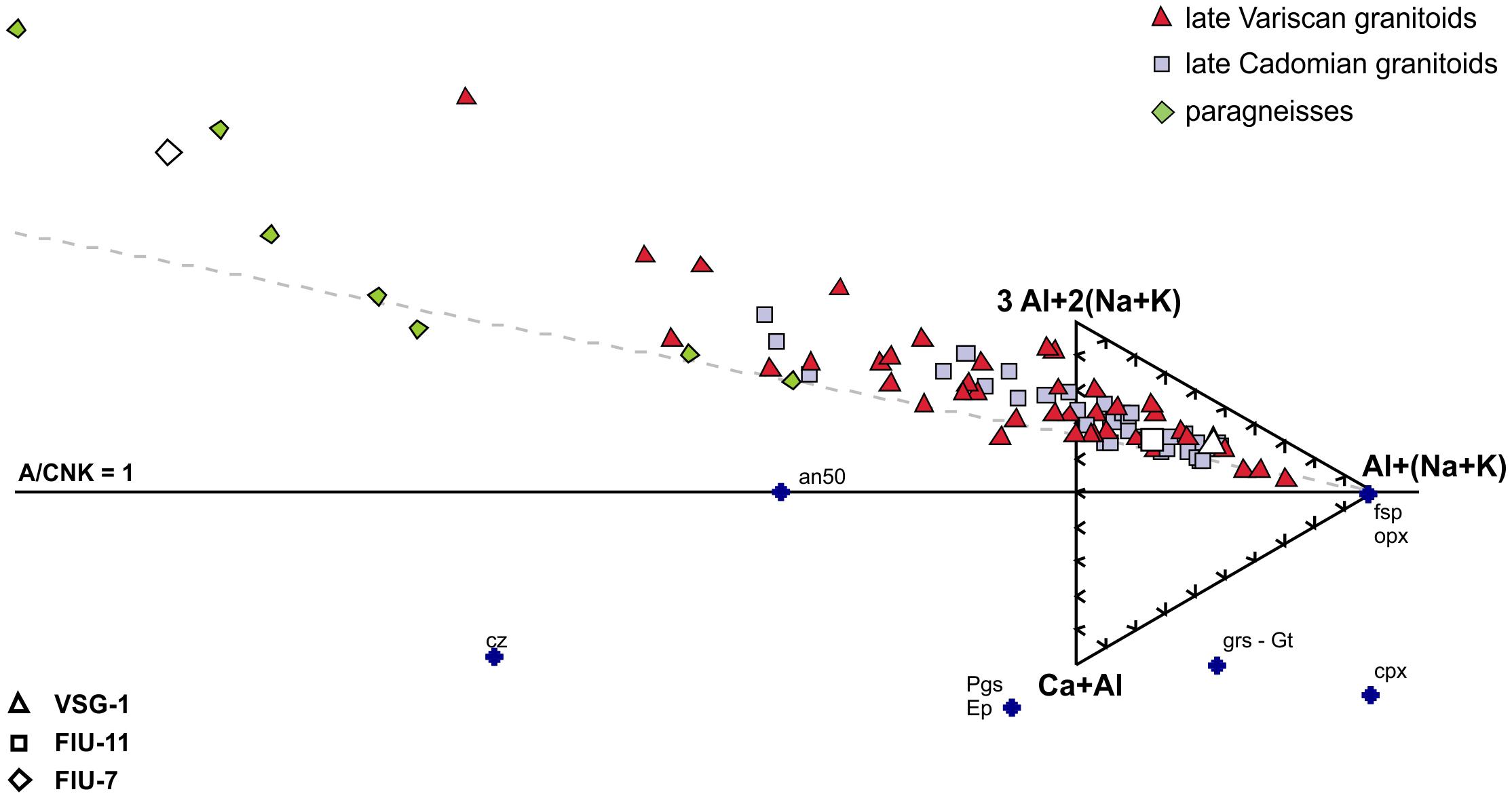
Figure 9. Composition of the studied rocks projected from biotite on the Ca + Al - 3Al + 2(Na + K) – Al + (Na + K) plane (after Moyen et al., 2017; reference data for the construction of the diagram were the compositions of more than 800 granitic melts (with SiO2 > 62 wt. %) produced by experimental melting of felsic to mafic igneous rocks and mature to immature sediments). Both granitoid populations plot immediately above the Al = 3 Ca + (Na + K) line, with a slope indicating their derivation from a felsic source (immature sediments and/or igneous rocks). Details about the construction and meaning of this diagram can be found in Moyen et al. (2017).
Despite minor compositional differences within the metasedimentary magma source, comparison with the information derived from the Villaseca et al. (1998) and Sylvester (1998) diagrams would indicate that, as stated by Moyen et al. (2017), the projection from biotite of the Shand diagram is better able to link all the granites to their source, independently of compositional variations induced by different degrees of fractionation or partial melting.
Geochemical data therefore suggest derivation of both the late Cadomian and late Variscan granitoids by partial melting of a metasedimentary source compositionally similar to the paragneisses that are the dominant rock type in the Aspromonte Unit of the southern CPO.
This inference is strongly reinforced by the zircon data. Dating of the inherited zircon cores in both the late Variscan leucogranodiorite VSG-1 and the metamorphosed late Cadomian granite revealed the same inheritance pattern, ranging in age from Early Paleoproterozoic to latest Neoproterozoic, with main clusters at ∼0.55 and ∼0.63 Ga, and minor groups at ∼0.95 and ∼2.5 Ga. These results suggest that the two granitoid populations were generated by partial melting of similar paragneisses from the same turbidite formation during two subsequent orogenic cycles. The detrital zircon from the paragneiss, host rock of the late Cadomian granite in the northeastern Peloritani, shows exactly the same age distribution of inherited zircons as in the two granites, although with slightly different proportions (Figure 6), providing strong evidence that both granites were produced by partial melting of deeper-seated paragneisses of the same provenance and composition, but with somewhat more of the 0.55 Ga component.
There are few in situ U–Pb zircon data available for the poly-orogenic basement of the CPO, but the zircon age distribution in the proposed metasedimentary magma source of the two granitoids studied here appears to be characteristic of the metasediments of the Aspromonte Unit. The review of the existing CPO U–Pb zircon data compiled by Fornelli et al. (2016) shows that a predominance of late Neoproterozoic zircon ages associated with significant clusters at ∼0.95 and ∼2.5 Ga is a feature of the Aspromonte Unit metagreywackes. Only sporadic late Neoproterozoic and Mesoproterozoic zircon dates were reported from the lower crustal metasediments (felsic granulites and metapelitic migmatites) exposed in the Serre Massif of central Calabria, with no clusters of Precambrian zircon dates like those in the rocks from the Aspromonte Unit. Furthermore, a preliminary study by Fiannacca et al. (2016) of the detrital zircon from a felsic granulite and the xenocrystic zircon from a sediment-contaminated metagabbro, both from the bottom of the Serre lower crustal sequence, suggested deposition of the original Serre sediments later than ∼450 Ma. This result appears consistent with the age of the inherited zircon in the late Variscan S-type granites of the Serre Batholith (Fiannacca et al., 2017), with the youngest cluster at ∼430–420 Ma pointing to deposition of the source sediment after that period.
Finally, the detrital zircon age distribution in a micaschist from the Mandatoriccio Complex in Sila (northern Calabria) shows strong similarities with that in the paragneisses of the Aspromonte, with main clusters between 0.7 and 0.5 Ga, some dates between 1.0 and 0.75 Ga and minor older ones (>1.6 Ga; Langone, 2008). Nevertheless, also in this case, the occurrence in the Mandatoriccio micaschists of large amounts of detrital zircon of Ordovician–Silurian age (451 ± 6 Ma) indicates that these sediments had not yet been deposited at the time the late Cadomian granitoids formed by partial melting of their metagreywacke source.
Analysis of the zircon data can also help assessing whether the high Fe and Mg contents of the studied late Cadomian granitoids reflect near-total melting, as proposed for the Ollo de Sapo Cambro-Ordovician ferrosilicic magmatism (e.g., Fernández et al., 2008; Castro et al., 2009), or a mobilized restite-melt system. High abundance of inherited zircon is considered a typical feature of low-temperature granitoids, formed at temperatures lower than 800–850°C (Chappell et al., 1998, 2004; Miller et al., 2003). Zircon saturation temperatures for the studied rocks range from 695 to 792°C for the late Variscan granitoids (average TZr = 746°C) and from 758 to 862°C for the late Cadomian granitoids (average TZr = 801°C). Again, the saturation temperatures of the late Cadomian granitoids show strong similarities with those calculated for the inheritance-rich Ollo de Sapo meta-magmatic rocks (770–860°C, average TZr = 826°C; Bea et al., 2007). Calculations by Bea et al. (2007) showed that, for an estimated magma temperature of 900°C, 100 μm-sized pre-magmatic zircon could survive only in case of fast melting, e.g., less than 10,000 years from beginning of melting at about 700°C, to the thermal peak of 900°C; then, any partially dissolved survived zircon could still survive upon cooling of a ∼300 m-thick granitoid magma body only if larger than 40 μm. This implies significant zircon dissolution in a magma with temperature about 70–80°C higher of the average TZr of the granitoids. Temperatures higher than 1000°C, as in the case of near-total melting, would necessarily lead to further considerable dissolution. These observations do not match the zircon features of the late Cadomian granitoids, whose inherited zircon cores are comparable in size with the detrital zircon cores in the paragneiss (Supplementary Figure S1). Furthermore, the late Cadomian granitoids were produced ∼50 Ma earlier than the Iberian Cambro-Ordovician ferrosilicic magmatic rocks, in a closing back-arc basin receiving contribution of detrital zircon from both the late Ediacaran magmatic arc and a north-African cratonic area (Fiannacca et al., 2013 and references therein). A provenance of the magma source sediment from the subduction channel, i.e., sediment deposited in the fore-arc region, as proposed in the cold diapir model of Fernández et al. (2008) and Castro et al. (2009), should in fact preclude the occurrence in the same magma source of zircon deriving from the Gondwana continent, beyond the back-arc basin. Therefore, our preferred interpretation for the high Fe and Mg contents of the studied late Cadomian granitoids is a significant entrainment of mafic minerals from the sedimentary magma source. These observations show that zircon inheritance studies in S-type granites provide important contributions to a better understanding of granite petrogenesis.
This study reveals that the same crustal sequence can melt during successive orogenies under different thermal regimes and in different post-collisional tectonic settings, giving rise to granitoid associations of different ages, with largely different geochemical features reflecting the specific melting conditions.
On the other hand, the zircon inheritance patterns and diagnostic chemical features of the granitoids are characteristic, not of the thermal regime or tectonic setting, but of the nature and composition of the crustal source rocks. Combining whole rock geochemistry with the zircon record in low-temperature granitoid rocks can therefore provide direct and reliable evidence of the source-granite petrogenetic link.
Data Availability
All datasets generated for this study are included in the manuscript and/or the Supplementary Files.
Author Contributions
PF conceived the study and wrote the manuscript with inputs from IW, RC, and AP. All authors contributed to manuscript revision, read, and approved the submitted version.
Funding
This project has received support from University of Catania through grant PDR 2016-2108 – 22722132115.
Conflict of Interest Statement
The authors declare that the research was conducted in the absence of any commercial or financial relationships that could be construed as a potential conflict of interest.
Acknowledgments
We thank Herve Rezeau, Juan Díaz-Alvarado, and Carlos Villaseca for their constructive comments and Julien Leuthold for editorial handling.
Supplementary Material
The Supplementary Material for this article can be found online at: https://www.frontiersin.org/articles/10.3389/feart.2019.00119/full#supplementary-material
References
Acquafredda, P., Fornelli, A., Piccarreta, G., and Pascazio, A. (2008). Multi-stage dehydration-decompression in the metagabbros from the lower crustal rocks of the Serre (southern Calabria, Italy). Geol. Mag. 145, 397–411. doi: 10.1017/S001675680700430X
Angì, G., Cirrincione, R., Fazio, E., Fiannacca, P., Ortolano, G., and Pezzino, A. (2010). Metamorphic evolution of preserved Hercynian crustal section in the Serre Massif (Calabria–Peloritani Orogen, southern Italy). Lithos 115, 237–262. doi: 10.1016/j.lithos.2009.12.008
Atzori, P., Cirrincione, R., Hartmut, K., Mazzoleni, P., Pezzino, A., Puglisi, G., et al. (2003). “The abundance of 53 elements and petrovolumetric models of the crust in north-eastern Peloritani Mountains (site 8),” in The Abundance of 55 Elements and Petrovolumetric Models of the Crust in 9 Types Areas from the Crystalline Basements of Italy, with some Geophysical and Petrophysical Data, Vol. 32, ed. F. P. Sassi (Rome: Accademia Nazionale delle Scienze detta dei XL), 319–371.
Atzori, P., and Lo Giudice, A. (1982). Gli gneiss occhiadini del messinese: caratterizzazione geopetrografica e petrochimica. Per. Mineral. 51, 51–74.
Barca, D., Cirrincione, R., De Vuono, E., Fiannacca, P., Ietto, F., and Lo Giudice, A. (2010). The Triassic rift system in the northern Calabrian-Peloritani Orogen: evidence from basaltic dyke magmatism in the San Donato Unit. Per. Mineral. 79, 61–72. doi: 10.2451/2010PM0010
Bea, F., Montero, P., González-Lodeiro, F., Talavera, C. (2007). Zircon inheritance reveals exceptionally fast crustal magma generation processes in Central Iberia during the Cambro-Ordovician. J. Petrol. 48, 2327–2339. doi: 10.1093/petrology/egm061
Bowring, S., and Williams, I. (1999). Priscoan (4.00–4.03 Ga) orthogneisses from northwestern Canada. Contrib. Mineral. Petrol. 134, 3–16. doi: 10.1007/s004100050465
Boynton, W. V. (1984). “Geochemistry of rare earth elements: meteorite studies,” in Rare Earth Element Geochemistry, ed. P. Henderson (New York, NY: Elsevier), 63–114. doi: 10.1016/B978-0-444-42148-7.50008-3
Caggianelli, A., Del Moro, A., Di Battista, P., Prosser, G., and Rottura, A. (2003). Leucogranite genesis connected with low-pressure high-temperature metamorphism in the Sila basement (Calabria, Italy). Schweiz. Mineral. Petrogr. Mitt. 83, 301–316.
Caggianelli, A., Del Moro, A., Paglionico, A., Piccarreta, G., Pinarelli, L., and Rottura, A. (1991). Lower crustal granite genesis connected with chemical fractionation in the continental crust of Calabria (Southern Italy). Eur. J. Mineral. 3, 159–180. doi: 10.1127/ejm/3/1/0159
Castro, A., García-Casco, A., Fernández, C., Corretgé, L. G., Moreno-Ventas, I., Gerya, T., et al. (2009). Ordovician ferrosilicic magmas: experimental evidence for ultrahigh temperatures affecting a metagreywacke source. Gondwana Res. 16, 622–632. doi: 10.1016/j.gr.2008.12.011
Chappell, B. W., Bryant, C. J., Wyborn, D., White, A. J. R., and Williams, I. S. (1998). High- and low-temperature I-type granites. Res. Geol. 48, 225–235. doi: 10.1111/j.1751-3928.1998.tb00020.x
Chappell, B. W., and White, A. J. R. (1992). I- and S-type granites in the Lachlan Fold Belt. Trans. R. Soc. Edinb. Earth Sci. 83, 1–26. doi: 10.1130/SPE272-p1
Chappell, B. W., White, A. J. R., Williams, I. S., and Wyborn, D. (2004). Low-and high-temperature granites. Spec. Pap. Geol. Soc. Am. 389, 125–140. doi: 10.1130/0-8137-2389-2.125
Chappell, B. W., White, A. J. R., and Wyborn, D. (1987). The importance of residual source material (restite) in granite petrogenesis. J. Petrol. 28, 1111–1138. doi: 10.1093/petrology/28.6.1111
Chen, Y. D., and Williams, I. S. (1990). Zircon inheritance in mafic inclusions from Bega Batholith granites, southeastern Australia: an ion microprobe study. J. Geophys. Res. 95, 787–717. doi: 10.1029/JB095iB11p17787
Cirrincione, R., Fazio, E., Fiannacca, P., Ortolano, G., Pezzino, A., and Punturo, R. (2015). The Calabria–Peloritani Orogen, a composite terrane in Central Mediterranean; its overall architecture and geodynamic significance for a pre-Alpine scenario around the Tethyan basin. Per. Mineral. 84, 701–749. doi: 10.2451/2015PM0446
Cirrincione, R., Fiannacca, P., Lustrino, M., Romano, V., and Tranchina, A. (2014). Late Triassic tholeiitic magmatism in Western Sicily: a possible extension of the Central Atlantic Magmatic Province (CAMP) in the Central Mediterranean area? Lithos 188, 60–71. doi: 10.1016/j.lithos.2013.10.009
Cirrincione, R., Fiannacca, P., Lustrino, M., Romano, V., Tranchina, A., and Villa, I. M. (2016). Enriched asthenosphere melting beneath the nascent North African margin: trace element and Nd isotope evidence in middle–late Triassic alkali basalts from central Sicily (Italy). Int. J. Earth Sci. 105, 595–609. doi: 10.1007/s00531-015-1190-2
D’Amico, C., Messina, A., Puglisi, G., Rottura, A., and Russo, S. (1973). Confronti petrografici nel cristallino delle due sponde delle Stretto di Messina. Implicazioni geodinamiche. Boll. Soc. Geol. Ital. 92, 939–953.
Debon, F., and Le Fort, P. (1983). A chemical–mineralogical classification of common plutonic rocks and associations. Trans. R. Soc. Edinb. Earth Sci. 73, 135–149. doi: 10.1017/S0263593300010117
Del Moro, A., Fornelli, A., and Piccarreta, G. (2000a). Disequilibrium melting in granulite-facies metasedimentary rocks of the Northern Serre (Calabria-Southern Italy). Mineral. Petrol. 70, 89–104. doi: 10.1007/s0071000700
Del Moro, A., Fornelli, A., and Piccarreta, G. (2000b). Tectonothermal history of the Hercynian continental crust of the Serre (southern Calabria, Italy) monitored by Rb–Sr biotite resetting. Terra Nova 12, 239–244. doi: 10.1046/j.1365-3121.2000.00304.x
Díaz-Alvarado, J., Fernández, C., Chichorro, M., Castro, A., and Pereira, M. F. (2016). Tracing the Cambro-Ordovician ferrosilicic to calc-alkaline magmatic association in Iberia by in situ U–Pb SHRIMP zircon geochronology (Gredos massif, Spanish Central System Batholith). Tectonophysics 681, 95–110. doi: 10.1016/j.tecto.2016.02.031
Engi, M., Lanari, P., and Kohn, M. J. (2017). Significant ages - an introduction to petrochronology. Rev. Mineral. Geochem. 83, 1–12. doi: 10.2138/rmg.2017.83.1
Farina, F., Dini, A., Rocchi, S., and Stevens, G. (2014). Extreme mineral-scale Sr isotopic heterogeneity in granites by disequilibrium melting of the crust. Earth Planet. Sci. Lett. 399, 103–115. doi: 10.1016/j.epsl.2014.05.018
Farina, F., and Stevens, G. (2011). Source controlled 87Sr/86Sr isotope variability in granitic magmas: the inevitable consequence of mineral-scale isotopic disequilibrium in the protolith. Lithos 122, 189–200. doi: 10.1016/j.lithos.2011.01.001
Fernández, C., Becchio, R., Castro, A., Viramonte, J. M., Moreno-Ventas, I., and Corretgé, L. G. (2008). Massive generation of atypical ferrosilicic magmas along the Gondwana active margin: implications for cold plumes and back-arc magma generation. Gondwana Res. 14, 451–473. doi: 10.1016/j.gr.2008.04.001
Festa, V., Langone, A., Caggianelli, A., and Rottura, A. (2010). Dike magmatism in the Sila Grande (Calabria, southern Italy): evidence of Pennsylvanian–Early Permian exhumation. Geosphere 6, 549–566. doi: 10.1130/GES00578.1
Fiannacca, P. (2000). Petrologia dei Corpi Leucocratici a Plagioclasio dei Peloritani Nord-Orientali (Sicilia Nord-Orientale). Ph.D. thesis, University of Catania, Catania.
Fiannacca, P., Basei, M. A. S., Cirrincione, R., Pezzino, A., and Russo, D. (2019). “Water-assisted production of late-orogenic trondhjemites at magmatic and subsolidus conditions”, in Post-Archean Granitic Rocks: Petrogenetic Processes and Tectonic Environments, eds V. Janoušek, B. Bonin, W. J. Collins, F. Farina, and P. Bowden (London: Special Publications, Geological Society), 491. doi: 10.1144/SP491-2018-113 (accessed Februray 6, 2019).
Fiannacca, P., Brotzu, P., Cirrincione, R., Mazzoleni, P., and Pezzino, A. (2005). Alkali metasomatism as a process for trondhjemite genesis: evidence from Aspromonte Unit, northeastern Peloritani, Sicily. Mineral. Petrol. 84, 19–45. doi: 10.1007/s00710-005-0073-9
Fiannacca, P., Cirrincione, R., Bonanno, F., and Carciotto, M. M. (2015). Source-inherited compositional diversity in granite batholiths: the geochemical message of Late Paleozoic intrusive magmatism in central Calabria (southern Italy). Lithos 23, 123–140. doi: 10.1016/j.lithos.2015.09.003
Fiannacca, P., Lo Pò, D., Ortolano, G., Cirrincione, R., and Pezzino, A. (2012). Thermodynamic modeling assisted by multivariate statistical image analysis as a tool for unraveling metamorphic P–T–d evolution: an example from ilmenite–garnet-bearing metapelite of the Peloritani Mountains, southern Italy. Mineral. Petrol. 106, 151–171. doi: 10.1007/s00710-012-0228-4
Fiannacca, P., Williams, I. S., and Cirrincione, R. (2017). Timescales and mechanisms of batholith construction: constraints from zircon oxygen isotopes and geochronology of the late Variscan Serre Batholith (Calabria, southern Italy). Lithos 277, 302–314. doi: 10.1016/j.lithos.2016.06.011
Fiannacca, P., Williams, I. S., Cirrincione, R., and Hegner, E. (2016). Suffering Zircon from a Variscan Lower Crustal Environment: U-Pb Ages and Oxygen Isotopic Compositions in Mafic and Felsic Granulites from the Serre Massif (Calabria, Italy). Granada: VIII International SHRIMP Workshop, 26–28.
Fiannacca, P., Williams, I. S., Cirrincione, R., and Pezzino, A. (2008). Crustal contributions to late Hercynian peraluminous magmatism in the southern Calabria–Peloritani Orogen, southern Italy: petrogenetic inferences and the Gondwana connection. J. Petrol. 49, 1497–1514. doi: 10.1093/petrology/egn035
Fiannacca, P., Williams, I. S., Cirrincione, R., and Pezzino, A. (2013). The augen gneisses of the Peloritani Mountains (NE Sicily): granitoid magma production during rapid evolution of the northern Gondwana margin at the end of the Precambrian. Gondwana Res. 23, 782–796. doi: 10.1016/j.gr.2012.05.019
Fornelli, A., Micheletti, F., and Piccarreta, G. (2016). Late-Proterozoic to Paleozoic history of the peri-Gondwana Calabria–Peloritani Terrane inferred from a review of zircon chronology. Springerplus 5:212. doi: 10.1186/s40064-016-1839-8
Froude, D. O., Ireland, T. R., Kinny, P. D., Williams, I. S., Compston, W., Williams, I. R., et al. (1983). Ion microprobe identification of 4,100-4,200 Myr-old terrestrial zircons. Nature 304, 616–618. doi: 10.1038/304616a0
Graessner, T., Schenk, V., Brocker, M., and Mezger, K. (2000). Geochronological constraints on timing of granitoid magmatism, metamorphism and post-metamorphic cooling in the Hercynian crustal cross-section of Calabria. J. Metam. Geol. 18, 409–421. doi: 10.1046/j.1525-1314.2000.00267.x
Ioppolo, S., and Puglisi, G. (1989). Studio petrologico di alcune metamorfiti erciniche dei Monti Peloritani nord orientali (Sicilia). Rend. Soc. Ital. Mineral. Petrol. 43, 643–656.
Janoušek, V., Farrow, C. M., and Erban, V. (2006). Interpretation of whole rock geochemical data in igneous geochemistry: introducing geochemical data toolkit (GCDkit). J. Petrol. 47, 1255–1259. doi: 10.1093/petrology/egl013
Jeon, H., and Williams, I. S. (2018). Trace inheritance—Clarifying the zircon O-Hf isotopic fingerprint of I-type granite sources: implications for the restite model. Chem. Geol. 476, 456–468. doi: 10.1016/j.chemgeo.2017.11.041
Jeon, H., Williams, I. S., and Chappell, B. W. (2012). Magma to mud to magma: rapid crustal recycling by Permian granite magmatism near the eastern Gondwana margin. Earth Planet. Sci. Lett. 319–320, 104–117. doi: 10.1016/j.epsl.2011.12.010
Knesel, K. M., and Davidson, J. P. (2002). Insight into collisional magmatism from isotopic fingerprints of melting reactions. Science 296, 2206–2208. doi: 10.1126/science.1070622
Langone, A. (2008). Hercynian Low-Pressure Metamorphism: Tectono-Thermal Evolution of the Mandatoriccio Complex (Sila Massif, Calabria). Ph.D. thesis, Università di Bologna, Bologna.
Langone, A., Caggianelli, A., Festa, V., and Prosser, G. (2014). Time constraints on the building of the Serre batholith: consequences for the thermal evolution of the Hercynian continental crust exposed in Calabria (southern Italy). J. Geol. 122, 183–199. doi: 10.1086/675227
Linnemann, U., Gerdes, A., Drost, K., and Buschmann, B. (2007). “The continuum between Cadomian Orogenesis and opening of the Rheic Ocean: constraints from LA-ICP-MS U–Pb zircon dating and analysis of plate-tectonic setting (Saxo-Thuringian Zone, NE Bohemian Massif, Germany),” in The Evolution of the Rheic Ocean: from Avalonian–Cadomian Active Margin to Alleghenian–Variscan Collision, Vol. 423, eds U. Linnemann, D. Nance, P. Kraft, and G. Zulauf (Boulder, CO: Geological Society of America Special Paper), 61–96. doi: 10.1130/2007.2423(03)
Linnemann, U., Pereira, F., Jeffries, T. E., Drost, K., and Gerdes, A. (2008). The Cadomian orogeny and the opening of the Rheic Ocean: the diacrony of geotectonic processes constrained by LA–ICP-MS U–Pb zircon dating (Ossa–Morena and Saxo–Thuringian Zones, Iberian and Bohemian Massifs). Tectonophysics 461, 21–43. doi: 10.1016/j.tecto.2008.05.002
McDonough, W. F., and Sun, S. (1995). The composition of the Earth. Chem. Geol. 120, 223–253. doi: 10.1016/0009-2541(94)00140-4
Messina, A., Rottura, A., and Russo, S. (1974). Le leucogranodioriti muscovitiche dell’entroterra di Villa S. Giovanni (Reggio Calabria). Per. Mineral. 43, 51–92. doi: 10.1039/c6md00575f
Micheletti, F., Barbey, P., Fornelli, A., Piccarreta, G., and Deloule, E. (2007). Latest Precambrian to Early Cambrian U–Pb zircon ages of augen gneisses from Calabria (Italy), with inference to the Alboran microplate in the evolution of the peri-Gondwana terranes. Int. J. Earth Sci. 96, 843–860. doi: 10.1007/s00531-006-0136-0
Micheletti, F., Fornelli, A., Piccarreta, G., Barbey, P., and Tiepolo, M. (2008). The basement of Calabria (southern Italy) within the context of the Southern European Variscides: LA–ICPMS and SIMS U–Pb zircon study. Lithos 104, 1–11. doi: 10.1016/j.lithos.2007.11.003
Miller, C. F., McDowell, S. M., and Mapes, R. W. (2003). Hot and cold granites? Implications of zircon saturation temperatures and preservation of inheritance. Geology 31, 529–532.
Moyen, J.-F., Laurent, O., Chelle-Michou, C., Couzinié, S., Vanderhaeghe, O., Zeh, A., et al. (2017). Collision vs. subduction-related magmatism: two contrasting ways of granite formation and implications for crustal growth. Lithos 277, 154–177. doi: 10.1016/j.lithos.2016.09.018
Nance, R., Gutiérrez-Alonso, G., Keppie, J. D., Linnemann, U., Murphy, J. B., Quesada, C., et al. (2010). Evolution of the Rheic Ocean. Gondwana Res. 17, 194–222. doi: 10.1016/j.gr.2009.08.001
Nance, R. D., Murphy, J. B., and Santosh, M. (2014). The supercontinent cycle: a retrospective essay. Gondwana Res. 25, 4–29. doi: 10.1016/j.gr.2012.12.026
Ogniben, L. (1960). Nota illustrativa dello schema geologico della Sicilia nord-orientale. Riv. Min. Sic. 64–65, 183–212.
Ogniben, L. (1973). Schema geologico della Calabria in base ai dati odierni. Geol. Rom. 12, 243–585.
Ortolano, G., Cirrincione, R., and Pezzino, A. (2005). PT evolution of Alpine metamorphism in the southern Aspromonte Massif (Calabria - Italy). Schweiz. Mineral. Petrogr. Mitt. 85, 31–56.
Ortolano, G., Visalli, R., Cirrincione, R., and Rebay, G. (2014). PT-path reconstruction via unraveling of peculiar zoning pattern in atoll shaped garnets via image assisted analysis: an example from the Santa Lucia del Mela garnet micaschists (North eastern Sicily-Italy). Per. Mineral. 83, 257–297. doi: 10.2451/2013PM0015
Otamendi, J. E., and Patiño Douce, A. E. (2001). Partial melting of aluminous metagreywackes in the northern sierra de comechingones, Central Argentina. J. Petrol. 42, 1751–1772. doi: 10.1093/petrology/42.9.1751
Paterson, B. A., Stephens, W. E., Rogers, G., Williams, I. S., Hinton, R. W., and Herd, D. A. (1992). The nature of zircon inheritance in two granite plutons. Spec. Pap. Geol. Soc. Am. 272, 459–471. doi: 10.1130/SPE272-p459
Payne, J. L., McInerney, D. J., Barovich, K. M., Kirkland, C. L., Pearson, N. J., and Hand, M. (2016). Strengths and limitations of zircon Lu–Hf and O isotopes in modelling crustal growth. Lithos 248–251, 175–192. doi: 10.1016/j.lithos.2015.12.015
Puglisi, G., and Rottura, A. (1973). Le leucogranodioriti muscovitiche della zona di Capo Rasocolmo. (Messina). Per. Mineral. 42, 207–256.
Romano, V., Cirrincione, R., Fiannacca, P., Lustrino, M., and Tranchina, A. (2011). Late-Hercynian post-collisional dyke magmatism in central Calabria (Serre Massif, southern Italy). Per. Mineral. 80, 489–515. doi: 10.2451/2011PM0032
Romano, V., Cirrincione, R., Fiannacca, P., Tranchina, A., and Villa, I. M. (2012). Petrologic constraints on post-Variscan andesite dyke magmatism in the Sila Massif (northern Calabria). Rend. Online Soc. Geol. Ital. 21, 148–150.
Rotolo, S., and De Fazio, P. (2001). Clinopyroxene bearing garnet amphibolites from the Ferrà Valley (northern Peloritani Mts., Sicily). Boll. Soc. Geol. Ital. 120, 31–35.
Rottura, A., Bargossi, G. M., Caironi, V., Del Moro, A., Maccarrone, E., Macera, P., et al. (1989). Petrology and geochemistry and Sr, Nd isotopes of contrasting Hercynian granitoids from the southern Calabrian Arc (southern Italy). Mineral. Petrol. Acta 32, 1–36.
Rottura, A., Bargossi, G. M., Caironi, V., Del Moro, A., Maccarrone, E., Macera, P., et al. (1990). Petrogenesis of contrasting Hercynian granitoids from the Calabrian Arc, Southern Italy. Lithos 24, 97–119. doi: 10.1016/0024-4937(90)90019-W
Rottura, A., Caggianelli, A., Campana, R., and Del Moro, A. (1993). Petrogenesis of Hercynian peraluminous granites from the Calabrian Arc, Italy. Eur. J. Mineral. 5, 737–754. doi: 10.1127/ejm/5/4/0737
Rottura, A., Del Moro, A., Pinarelli, L., Petrini, R., Peccerillo, A., Caggianelli, A., et al. (1991). Relationships between intermediate and acidic rocks in orogenic granitoid suites: petrological, geochemical and isotopic (Sr, Nd, Pb) data from Capo Vaticano (southern Calabria, Italy). Chem. Geol. 92, 153–176. doi: 10.1016/0009-2541(91)90054-U
Samperton, K. M., Schoene, B., Cottle, J. M., Brenhin Keller, C., Crowley, J. L., and Schmitz, M. D. (2015). Magma emplacement, differentiation and cooling in the middle crust: integrated zircon geochronological-geochemical constraints from the Bergell Intrusion, Central Alps. Chem. Geol. 417, 322–340. doi: 10.1016/j.chemgeo.2015.10.024
Schaltegger, U., and Davies, J. H. F. L. (2018). Petrochronology of zircon and baddeleyite in igneous rocks: reconstructing magmatic processes at high temporal resolution. Rev. Mineral. Geochem. 83, 297–328. doi: 10.2138/rmg.2017.83.10
Schulmann, K., Schaltegger, U., Ježek, J., Thompson, A. B., and Edel, J. B. (2002). Rapid burial and exhumation during orogeny: thickening and synconvergent exhumation of thermally weakened and thinned crust (Variscan orogen in Western Europe). Am. J. Sci. 302, 856–879. doi: 10.2475/ajs.302.10.856
Shand, S. J. (1943). Eruptive Rocks. Their Genesis, Composition, Classification, and Their Relation to Ore-Deposits with a Chapter on Meteorite. New York: John Wiley and Sons.
Stevens, G., Villaros, A., and Moyen, J.-F. (2007). Selective peritectic garnet entertainment as the origin of geochemical diversity in S-type granites. Geology 35, 9–12. doi: 10.1130/G22959A.1
Streckeisen, A. L., and Le Maitre, R. W. (1979). A chemical approximation to the modal QAPF classification of the igneous rock. Neues Jahrb. Mineral. Abh. 136, 169–206.
Sylvester, P. J. (1998). Post-collisional strongly peraluminous granites. Lithos 45, 29–44. doi: 10.1016/s0024-4937(98)00024-3
Vance, D., Müller, W., and Villa, I. M. (2003). Geochronology: linking the isotopic record with petrology and textures - An introduction. Geol. Soc. Lond. Spec. Publ. 220, 1–24. doi: 10.1144/GSL.SP.2003.220.01.01
Villaseca, C., Barbero, L., and Herreros, V. (1998). A re-examination of the typology of peraluminous granite types in intracontinental orogenic belts. Trans. R. Soc. Edinb. Earth Sci. 89, 113–119. doi: 10.1017/S0263593300007045
von Raumer, J. F., Bussy, F., Schaltegger, U., Schulz, B., and Stampfli, G. M. (2013). Pre-Mesozoic Alpine basements-their place in the European Paleozoic framework. Geol. Soc. Am. Bull. 125, 89–108. doi: 10.1130/B30654.1
Wilde, S. A., Valley, J. W., Peck, W. H., and Graham, C. M. (2001). Evidence from detrital zircons for the existence of continental crust and oceans on the Earth 4.4 Gyr ago. Nature 409, 175–178. doi: 10.1038/35051550
Williams, I. S. (1992). Some observations on the use of zircon U-Pb geochronology in the study of granitic rocks. Spec. Pap. Geol. Soc. Am. 272, 447–458. doi: 10.1130/SPE272-p447
Williams, I. S. (2001). Response of detrital and zircon and monazite, and their U-Pb isotopic systems, to regional metamorphism and host-rock partial melting, Cooma Complex, Southeastern Australia. Aust. J. Earth Sci. 48, 557–580. doi: 10.1046/j.1440-0952.2001.00883.x
Williams, I. S., Fiannacca, P., Cirrincione, R., and Pezzino, A. (2012). Peri-Gondwanan origin and early geodynamic history of NE Sicily: a zircon tale from the basement of the Peloritani Mountains. Gondwana Res. 22, 855–865. doi: 10.1016/j.gr.2011.12.007
Zeck, H. P., and Williams, I. S. (2002). Inherited and magmatic zircon from Neogene Hoyazo cordierite dacite, SE Spain - Anatectic source rock provenance and magmatic evolution. J. Petrol. 43, 1089–1104. doi: 10.1093/petrology/43.6.1089
Keywords: S-type granites, meta-greywackes, zircon inheritance, whole rock chemistry, crustal melting, Cadomian, Variscan, Aspromonte-Peloritani
Citation: Fiannacca P, Williams IS, Cirrincione R and Pezzino A (2019) Poly-Orogenic Melting of Metasedimentary Crust From a Granite Geochemistry and Inherited Zircon Perspective (Southern Calabria-Peloritani Orogen, Italy). Front. Earth Sci. 7:119. doi: 10.3389/feart.2019.00119
Received: 11 March 2019; Accepted: 06 May 2019;
Published: 24 May 2019.
Edited by:
Julien Leuthold, ETH Zürich, SwitzerlandReviewed by:
Herve Rezeau, Massachusetts Institute of Technology, United StatesJuan Díaz-Alvarado, University of Huelva, Spain
Carlos Villaseca, Complutense University of Madrid, Spain
Copyright © 2019 Fiannacca, Williams, Cirrincione and Pezzino. This is an open-access article distributed under the terms of the Creative Commons Attribution License (CC BY). The use, distribution or reproduction in other forums is permitted, provided the original author(s) and the copyright owner(s) are credited and that the original publication in this journal is cited, in accordance with accepted academic practice. No use, distribution or reproduction is permitted which does not comply with these terms.
*Correspondence: Patrizia Fiannacca, cGZpYW5uYUB1bmljdC5pdA==