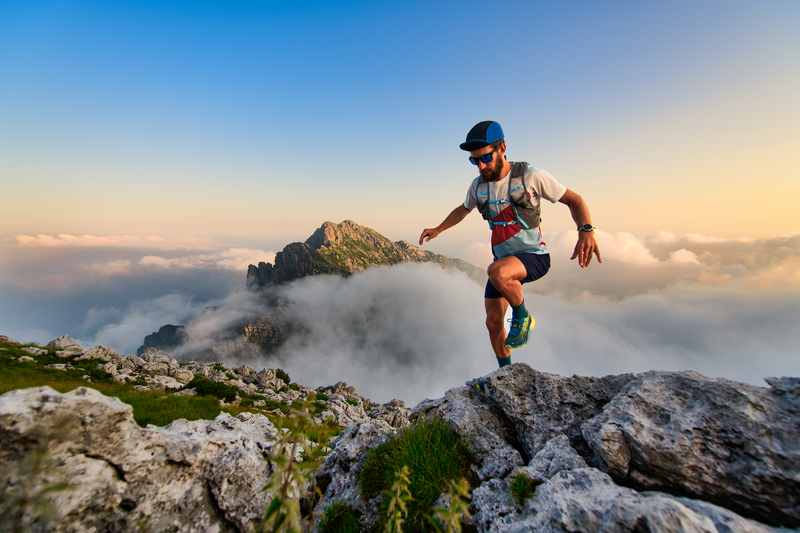
95% of researchers rate our articles as excellent or good
Learn more about the work of our research integrity team to safeguard the quality of each article we publish.
Find out more
ORIGINAL RESEARCH article
Front. Earth Sci. , 22 February 2019
Sec. Quaternary Science, Geomorphology and Paleoenvironment
Volume 7 - 2019 | https://doi.org/10.3389/feart.2019.00028
This article is part of the Research Topic North Pacific Environment and Paleoclimate from the Late Pleistocene to Present View all 13 articles
We use three-dimensional modeling of the basin of Hidden Lake, Montana, to assess the influence of effective fetch on diatom-inferred changes in mixing depths throughout the Holocene. The basin of Hidden Lake is characterized by a complex morphometry; for example, three-dimensional modeling of the lake basin indicates that a decrease in lake level of 2 m would result in complete isolation of the deepest part of the lake basin from the rest of the lake. Our model suggests that small changes in the lake surface elevation at Hidden Lake would produce threshold-like responses in effective fetch, which in turn would have a profound influence on average lake mixing depth. The present-day planktic diatom community of Hidden Lake is comprised of three species. Neo-ecological experiments revealed the effect of mixing depth and nutrient levels on growth rates of these species. A sediment core collected from the deepest part of the lake basin and spanning the last 8,640 years was analyzed for diatoms. Here we show how changes in fetch through the Holocene explain changes in the dominant planktic diatom species by modification of the thermal structure of Hidden Lake. Additionally, the timing of diatom-inferred changes in effective moisture and thermal structure from Hidden Lake were compared to late Holocene patterns reconstructed from other regional lake records. Between 8.64 – 7.61 ka the diatom record from Hidden Lake suggests that the lake was deep and fresh, although somewhat lower than the modern lake. After 7.61 ka, water levels rose, expanding the available benthic diatom habitat. Between 6.18 and 4.13 ka, lake level declined and seasonal stratification was enhanced. After 1.4 ka, the lake became deeper and less stratified in response to the effects of enhanced fetch. We argue that changes in effective fetch may play an important, and underexplored, role in planktic diatom community structure over longer time scales and should be more broadly considered in paleolimnological studies.
Lakes that are sensitive to changes in effective moisture are highly desirable for paleolimnological studies because variations in lake surface elevation can readily alter the water chemistry, nutrient cycling, and availability of limnobiotic habitats (Stone and Fritz, 2004; Fritz, 2008; Wolin and Stone, 2010). Ideally, limnologic responses faithfully record changing climates and environmental variables through time by archiving variations in sediment characteristics, geochemistry, and remains of environmentally sensitive organisms (Fritz, 1996).
Morphometric parameters of most lake basins, such as depth, surface area, volume, and basin shape, typically are persistent factors over shorter timeframes. These factors combine with the positional framework components of a basin, such as fetch, aspect, and exposure to wind, to ensure that most lake environments are relatively stable. However, these features of the basin also make the sensitivity to hydroclimate variations over time unique for each lake, even within the same region (Ragotzkie, 1978; Fritz et al., 2000; Håkanson, 2005; Wigdahl et al., 2014).
Physical properties are critical factors that influence the heat budget of a lake (Ragotzkie, 1978). Among other things, they significantly impact the timing of ice formation and breakup, the thermal structure of the lake throughout the season (Davies-Colley, 1988; Gorham and Boyce, 1989; Robertson and Ragotzkie, 1990; Pal’shin et al., 2008), the maximum heat content during the ice free period, and snow coverage (Scott, 1964; Ragotzkie, 1978). Consequently, the influence of physical properties reverberates throughout the limnologic environment. It affects physical limnological settings, such as nutrient cycling (Osgood, 1988), light penetration and turbidity (Bernhardt et al., 2008; von Einem and Graneli, 2010), sediment redistribution (Evans, 1994; Blais and Kalff, 1995; Hamilton and Mitchell, 1996; Bachmann et al., 2000), and sedimentation rates (Lehman, 1975; Anderson, 1990). Hence, it also influences nearly every biological aspect of the ecosystem from phytoplankton production (Fee, 1979; Davies-Colley, 1988; Carrick et al., 1993; Diehl et al., 2002) to availability of fish habitats (Håkanson, 2005).
Despite the relevance of these factors to understanding lake and lake-ecosystem response to climate change, paleolimnological studies rarely examine the effects of changing lake surface elevation on morphometric basin parameters, other than water depth (Mason et al., 1994; Verschuren, 1999). This is somewhat surprising, considering the relative ease and inexpensiveness of generating lake morphometric data (Håkanson, 1981) compared to the potential for utilizing this type of information to improve paleolimnological inferences. From a bathymetric map and software capable of three-dimensional modeling, it is possible to generate all of the morphometric parameters listed above for a modern lake, as well as potential lake morphometries under various lake surface elevations, including conditions substantially deeper and shallower than the modern lake (Stone and Fritz, 2004; Wigdahl et al., 2014).
Fetch, which is the distance that wind blows across a lake surface, is a simple morphometric parameter to measure from a bathymetric map (Håkanson, 1981). Since wind rarely blows in a constant direction, fetch is commonly calculated as effective fetch, which takes into consideration a range of possible wind directions and includes the influence of lake width across those directions on wave energy (Smith and Sinclair, 1972; Håkanson and Jansson, 1983). The influence of fetch on most lake systems is profound; fetch is a principle factor affecting wave energy, and as a result, the influence of fetch on seasonal mixing and thermocline development is substantial (Ragotzkie, 1978; Gorham and Boyce, 1989; Håkanson, 2005; von Einem and Graneli, 2010). In shallower areas of a lake, wave energy and the depth of convective mixing controls resuspension of lake sediment (Håkanson and Jansson, 1983; Whitmore et al., 1996; Bachmann et al., 2000). As a result, fetch is also a primary control on macrophyte distribution (Rea et al., 1998; Thomaz et al., 2003). In smaller, temperate lakes, fetch exerts a critical influence over the temperature threshold at which a lake will freeze over and can influence snow coverage, and thus ice thickness and the timing of ice breakup (Scott, 1964; Ragotzkie, 1978). Together, fetch combines with wind strength and duration, air temperature, winter precipitation, average depth, and irradiance/light penetration (Fee et al., 1996; Snucins and Gunn, 2000; Houser, 2006; Persson and Jones, 2008) to control the seasonal progression of the thermal structure of a lake.
In all lakes that are sensitive to changes in effective moisture, as lake levels fluctuate, there is a potential for a change in fetch. Since the lake surface area grows or shrinks with changes in lake volume, the straight-line distance from shore to the shore may be substantially altered. This is particularly true of lakes with a complex bathymetry (multiple-basin lakes), where a relatively small change in lake level may result in a substantial change in lake surface area, for example when lake basins become isolated from each other under shallowing conditions or coalesce when lake levels rise (Stone and Fritz, 2004). These threshold responses in fetch create an intriguing potential for paleolimnological studies, where a series of cascading changes in fundamental lake dynamics may be affected by a fairly minor change in lake surface elevation.
Because of their sensitivity to a variety of changes in the lake environment, particularly changes in lake level, water chemistry, mixing depth, and nutrient conditions, fossil diatom assemblages are used frequently in paleolimnologic studies (Fritz, 1996; Diehl et al., 2002; Wolin and Stone, 2010; Saros et al., 2012; Stone et al., 2016). Saros et al. (2012) explicitly explored the autecology of three planktic diatom species (Lindavia intermedia, Lindavia comensis, and Discostella stelligera) that occur in the modern diatom community of Hidden Lake. Using a set of neo-ecological experiments designed to gauge the influence of the interaction of mixing depth and nutrients on growth rates, these three diatom species were determined to express a growth preference reflecting a gradient of mixing depths. These experiments were coupled with the collection of a sediment core from Hidden Lake, which was used to reconstruct the recent (past few centuries) record of changes in mixing depth over time, using the same three diatom species which frequently occur in fossil diatom assemblages of the core.
The modern bathymetry of Hidden Lake is complex (Figure 1), and has a linear geometry with multiple sub-basins. Because of the linear geometry, relatively large surface area, and the fact that it is inset into a relatively narrow valley, fetch invariably plays a large role in the energy of the modern lake (Smith and Sinclair, 1972; Håkanson and Jansson, 1983). Additionally, the depth of the sub-basins is highly asymmetric; the basin has one deep sub-basin connected by a relatively shallow arch to the rest of the lake. Over very long timeframes, such as the Holocene, the influence of the basin morphology, and its interaction with changes in lake level, are substantially more likely to have played a role in the thermal structure of the lake. To test this hypothesis, we created a three-dimensional model of the lake basin using a bathymetric map we generated by measuring depths throughout the lake. We used this information to calculate lake surface areas across a range of potential lake levels. We then used this information to estimate effective fetch and compared this modeled information against the diatom-inferred mixing depth reconstructed from the sediment record for the last 8,500 years.
Figure 1. Bathymetric map of Hidden Lake. Core location marked by filled star. Contour intervals shown are in 5 m intervals. Location of Hidden Lake, Foy Lake (FL), and Jones Lake (JL) are marked on inset map.
Hidden Lake (N48° 40′53″, W113° 44′34.5″) is located in Glacier National Park, immediately west of the Continental Divide (Figure 1). Local bedrock is composed of Proterozoic sedimentary rocks, mainly mudstones, of the Belt Supergroup. The lake is inset into a steep-walled basin oriented primarily NW-SE. Talus deposits border the eastern margin of the lake (particularly on the southern end), composed of unsorted and mainly unvegetated, angular rubble. The surrounding basin is primarily exposed bedrock with rocky alpine meadows on flat areas and linear copses of evergreens along moderate slopes.
Hidden Lake is a low-nutrient system [total phosphorus (TP) 1.2 μg/L, Nitrate-N (NO3-N) 25 μg/L, dissolved organic carbon (DOC) 0.4 mg/L] with high light penetration [July 1% photosynthetically active radiation (PAR) 34 m]. Consequently, the sedimentation rate is very low (0.08 mm/year). Measurements taken in July 2007 indicated a mixed layer depth of 5 m, with an epilimnion temperature of ∼15°C. This site was selected for analysis because the primary planktic diatom species that occur in the modern lake system are composed of Lindavia comensis, with smaller percentages of Discostella stelligera, and Lindavia intermedia. The ecology of these three species, within the context of alpine and subalpine lakes of the Northern and Central US Rocky Mountains, were examined in a broader component of this research project in a series of assay experiments performed in situ, which found the primary controls on growth to be correlated to (either independently or in combination) NO3-N and mixing depth (thickness of the mixed layer) under stratified lake conditions (Saros et al., 2012; Saros and Anderson, 2015).
Twenty-seven measurements of the depth to the sediment-water interface were taken with a hand-held depth-finder from a series of transects on Hidden Lake. Depth measurements were overlain onto a topographic map and the basin was digitized using Surfer 10.0 (Golden Software, 2010). A scaled, three-dimensional model of the lake bathymetry (Figure 1) and topography of the surrounding basin was generated by gridding the data using a minimum curvature method at a 3 m equant horizontal resolution. Values for lake volume, water surface area, and lake basin (sediment-water) surface area, were calculated within the program Surfer. 3D modeling of the lake basin using Surfer allowed for iterative calculations of every potential lake surface elevation from the modern lake surface elevation down to the minimum potential lake surface in 0.5-m resolution depth steps. Modern Hidden Lake currently overflows via a small stream (Hidden Creek), which would limit significant rise of lake level within the existing basin. Because of this, potential lake surface elevations above the modern lake elevation were ignored when modeling. Regions outside the modern lake margin and shallower sub-basins within the lake that became isolated from the deepest basin (where the core was collected) when potential lake elevation surfaces were lower were ‘blanked’ from the model to remove them from contributing to lake volume and surface area calculations. Mean lake depth was calculated by dividing the total lake volume at a given modeled lake elevation by the total planar surface area for that elevation.
Lake volumes calculated using Surfer were created by averaging the values from three estimation techniques (Trapezoidal Rule, Simpson’s Rule, and Simpson’s 3/8th Rule), with an average relative error of approximately 0.02% between the three techniques. Values for planar surface area and basin surface area for each contour interval also were calculated within Surfer, using the equation for calculating the area of a polygon with n vertices (where x and y are coordinates in Cartesian space):
Average depth values for the lake were calculated for each potential lake elevation by dividing the calculated volume (m3) by the calculated planar surface area (m2).
Fetch was calculated at each modeled lake surface elevation from a fixed point in the middle of the deepest basin (near the core site; Figure 1). Maximum effective fetch was also calculated for the lake at every potential modeled lake elevation. Effective fetch was calculated using the following formula, after Håkanson and Jansson (1983):
Where xi is the distance to the shore at angles in increments of ±6° (γ) from 0 to 42° from the direction of maximum fetch.
Sediment was collected from an inflatable boat from the deepest basin (∼30 mbls) of Hidden Lake (Figure 1) with a simple pole-driven (Griffith) piston corer during the summer of 2007. The sediment-water interface was preserved intact, in a polycarbonate barrel that captured a sedimentary sequence 65 cm in length. The complete core was sub-sampled in the field, continuously, in 0.5-cm increments. A set of materials were collected for dating (Table 1) using a combination of 210Pb-dating (Appleby and Oldfield, 1978) for near-surface sediments and a series of AMS radiocarbon-dated materials, including wood/plant fragments (Stuiver et al., 1998) that were recovered throughout the core (Table 1). 210Pb activities were counted by gamma ray spectroscopy methods; the 210Pb chronology was based on the constant rate of supply (CRS) model. An age model for the core was constructed (Figure 2) using the Bayesian age-depth modeling software BACON (version 2.2) (Blaauw and Christen, 2011). All ages reported herein as “ka” are calibrated ages in 1000s of years before 1950.
Figure 2. Age-depth model of sediment core from Hidden Lake (red line) and associated error envelope (gray). Calibrated radiocarbon dates from Table 1 are plotted as purple circles.
One hundred and thirty-one samples were collected from the core for diatom analysis. Each sample was treated with 10% HCl and 35% H2O2 at room temperature to digest carbonate and organic material. Known quantities of polystyrene microspheres were added to estimate diatom concentrations (Battarbee, 2003); diatoms were mounted in Zrax, a high-refractive index medium. Diatom frustules were analyzed using a Zeiss AxioSkop2 transmitted light microscope at 1000x magnification under differential interference contrast. Diatom species were identified to the lowest taxonomic level possible using the following taxonomic guides: Foged (1981); Cumming et al. (1995), Lange-Bertalot et al. (1996); Camburn and Charles (2000), Fallu et al. (2000), and Bahls (2004, 2005). Modern taxonomic concepts were applied to diatom identifications (see Supplementary Data) using the websites Diatoms of the United States (Spaulding et al., 2010) and AlgaeBase (Guiry and Guiry, 2017). When possible, at least 300 diatom valves were identified from each sample interval. The ratio of planktic to benthic diatoms was calculated for each sample based on autecological information provided in the flora and taxonomic websites listed above. Constrained cluster analysis of the fossil diatom assemblages (CONISS method on square-root transformed data) was performed using the R package rioja (Juggins, 2015). Statistical significance of the constrained clusters was determined using the Broken Stick method.
Diatom-inferred mixing depths (DI-MDs) were reconstructed for each sample using a simple weighted averaging technique applied to the relative abundances of the three dominant planktic diatom species, here extended throughout the length of the core. Optimum mixing depths for each species were shown to be significantly different and, when taken together, form a gradient of species preference for mixing depths: L. intermedia (13.8 m), L. comensis (9 m), D. stelligera (4 m) (Saros et al., 2012). In the sediment record, only one other planktic diatom species was common (L. radiosa), occurring in the lower half of the core. However, because it is not a significant component of the modern diatom community of Hidden Lake, was not observed in our original lake calibration dataset, and was not included in neo-ecological experiments presented in Saros et al. (2012), we had no known autecological information regarding mixing depth preferences for this species within this lake system. As a result, this taxon was excluded from the DI-MD reconstructions.
Three-dimensional modeling of the lake basin produced a series of values for lake surface area, basin surface area, and volume of the lake throughout all potential lake elevations (Figure 3). Average lake depth for the modern lake elevation was calculated to be ∼8 m; average lake depth varied non-linearly with maximum depth, reaching a maximum average depth of ∼15 m when the lake level was modeled to be 5 m lower than the modern surface (Figure 3).
Figure 3. Calculated morphometric parameters at each potential lake surface elevation of Hidden Lake, based upon 3-D modeling of lake bathymetry.
Three-dimensional modeling of the lake basin under different potential lake surface elevations indicates that the deepest sub-basin in the lake, where the sediment core was collected, would become completely isolated from the remaining lake sub-basins with a drop in lake surface elevation of less than 2 m (Figure 4). Given the complex bathymetry of the lake, it is not surprising that basin surface area, lake surface area, and volume show a threshold response to decreasing lake surface elevation as the deep basin becomes isolated. Modeling the lake elevation to be 2 m shallower decreases the planar lake surface area (within the isolated deepest sub-basin) by nearly 70% (Figure 3). At this modeled lake elevation, the potential maximum effective fetch decreases by ∼25% relative to the modern lake system. Potential fetch calculated from the core site produced a slightly larger drop over the same change in lake elevation, decreasing by nearly 35% (Figure 5).
Figure 4. Basin isolation maps showing how small changes in lake surface elevation result in a separation of modern sub-basins for Hidden Lake, based upon 3-D modeling of lake bathymetry. Bathymetry of Hidden Lake at three lake surface elevations: (A) 1947.5 m, (B) 1946.5 m, and (C) 1945.5 m.
Figure 5. Calculations of effective fetch at the core site and maximum effective fetch for Hidden Lake, based upon 3-D modeling of the lake bathymetry.
Diatom assemblages for the past 8500 years at Hidden Lake are characterized mostly by the abundance of small colonial tychoplanktic species, primarily Pseudostaurosira brevistriata (20–40%) and Staurosirella pinnata (5–20%). These species co-occur throughout the core with the most common planktic diatom taxa, Lindavia comensis (10 to 60%), found in various combinations with L. intermedia, L. radiosa, and Discostella stelligera, all of which are typically present in relative abundances between 0 and 10% (Figure 6). With the exception of one section of the core representing the early Holocene (∼8–6 ka), with unusually high abundances of benthic monoraphid species (Karayevia suchlandtii, K. nitidiformis, and Cocconeis sp.), strictly benthic species were present in low abundances. More commonly, benthic taxa comprised less than 20% of all valves counted.
Figure 6. Diatom stratigraphy of Hidden Lake displaying relative abundance of major taxa in the sediment assemblages (left). Constrained cluster dendrogram and associated diatom zones (right). Diatom taxa plots are color coded by habitat type: planktic (blue), tychoplanktic (teal), and benthic (orange). Red dotted line corresponds to clusters defined by Broken Stick Method.
In general, we interpret the consistent abundance of small colonial tychoplankton (P. brevistriata, P. pseudoconstruens, S. pinnata), which are pervasive throughout the sediment record, to be representative of cold, moderately deep, low nutrient environments within the lake (Lotter and Bigler, 2000; Kingsbury et al., 2012). As discussed above, the most common modern planktic diatom species (L. intermedia, L. comensis, and D. stelligera) were used as part of the selection criteria for this lake. Based upon in situ neo-ecological experiments and published autecological information (Saros et al., 2012; Saros and Anderson, 2015) their co-occurrence in the modern lake most likely reflects seasonal changes in lake mixing depths in Hidden Lake. Because sediment samples average time, changes in their abundances throughout the Holocene are interpreted to represent long-term changes in the average state of mixing depths over time (Figure 7). The remaining major diatom species observed preferred strictly benthic habitats (attached to plant, rock, or sediment surfaces); because diatoms are photosynthetic, most benthic diatoms represent the shallow sunlit habitats that mantle the margin of lakes. In clear, oligotrophic, alpine lake systems, photosynthetically active radiation necessary for photosynthesis may penetrate fairly deep into the lake, but most benthic diatom species rapidly decline in relative abundance with depth. In lakes with complex bathymetries, such as Hidden Lake, available surfaces that can be colonized by benthic diatoms may increase or decrease with changing lake levels or light penetration (Stone and Fritz, 2004; Wolin and Stone, 2010; Wigdahl et al., 2014). However, dynamic changes in the ratio of planktic to benthic diatoms through time likely reflect fluctuations in lake level, with the direction of lake level change potentially being uncertain in lakes with complex bathymetries, such as Hidden Lake (Stone and Fritz, 2004).
Figure 7. Summary diatom figure for sediment core collected from Hidden Lake. Major planktic diatom taxa used for diatom-inferred mixing depth (DI-MD) plotted (left). DI-MD and planktic to benthic index plotted along with diatom zones (right). DI-MD figure plotted on a reference line indicating average mixing depth in meters; dotted gray line reflect intervals where mixing depth inferences may be less reliable resulting from low diversity in the planktic species used in modeling mixed depths.
Based upon the constrained cluster analysis and associated broken stick modeling, statistically significant changes in the fossil diatom assemblages occur at three points in the lake’s history: 7.61, 6.18, and 4.13ka (Figure 6). These significant changes are defined by the rise of benthic monoraphid diatoms (Diatom Zone 2), the reversal of this transition to dominance by plankton and tychoplankton (Diatom Zone 3), and the eventual disappearance of Lindavia radiosa in any substantial abundance from the lake plankton (Diatom Zone 4). Just below the threshold for significance, there are several other important transitions in the fossil diatom assemblages, which we define here as subzones used for descriptive purposes.
Diatom sub-zone 1a characterizes the early part of the lake’s history as one dominated by plankton (L. comensis 20–30%, L. radiosa∼5%) and small colonial tychoplankton (P. brevistriata 20–30%, S. pinnata 10–20%) and low abundances (∼20% total) of benthic diatom species. Diatom sub-zone 1b includes the same dominant diatom species as 1a, but is distinguished by the progressive increase (from 8% to ∼30%) in monoraphid benthic diatom species (especially K. suchlandtii∼15%), which replaces the dominant planktic species, L. comensis, from the base of the sub-zone to the top.
This zone is defined by a dominance of monoraphid species (K. suchlandtii, K. nitidiformis, Cocconeis sp.), which typically represent about half of the total assemblage for every sample. Colonial fragilarioid tychoplankton commonly comprise about 25–35% of the assemblage throughout the zone, increasing slightly toward the top of sub-zone 2b. A slight decrease in the relative abundance of the planktic species L. radiosa and the presence of uncommon biraphid benthic species, such as Cavinula aff. jaernefeltii and Sellaphora pupula, characterize DZ2. Sub-zone 2b is defined primarily by the gradual disappearance of K. nitidiformis and the appearance of the planktic species Lindavia intermedia, which represents about 5% of all assemblages throughout sub-zone 2b.
This diatom zone is defined by the rapid decline of monoraphid benthic diatoms (which represent < 5% of the total assemblage after DZ2) and a gradual increase in two planktic diatom species (L. comensis, L. radiosa). Colonial tychoplankton remain common (∼40–50%) throughout this zone. Biraphid benthic diatom species, including Diploneis elliptica, D. marginestriata, and C. aff. jaernefeldtii, increase in relative abundance, typically comprising ∼15–20% of the total diatom assemblage in DZ3. Sub-zone 3b is distinguished by the appearance of the planktic species Discostella stelligera, which typically occurs as about 5% of the total diatom assemblage throughout the core afterward.
After about 4ka, the diatom assemblages from Hidden Lake stabilize and for most of the remaining record, the diatom assemblages essentially resemble the modern surface samples. Strictly benthic diatoms usually comprise 15–20% of the total assemblage, tychoplankton make up about 40–50% of the total assemblage, and planktic species typically range from about 30% at the base of DZ4 to about 60% at the top of the zone. The sub-zones in this part of the core are defined entirely by transitions between the planktic species L. comensis and L. intermedia. Subzone 4a is typified by elevated relative abundances of L. comensis (∼35% of the assemblage). Sub-zone 4b is marked by an increase in L. intermedia (from ∼5% to nearly 20%). Subzone 4c is characterized by a rapid rise in L. comensis, which reaches its greatest relative abundance in the last 200 years of the record. The rise in relative abundance of L. comensis is subzone 4c occurs at the expense of L. intermedia and small colonial tychoplankton, which both rapidly decrease throughout this period.
DI-MDs for Hidden Lake ranged from approximately 8–12 m (Figure 7) throughout the core. Prior to sub-zone 2b, planktic diatom species occurred with limited relative abundance and are mostly represented by L. comensis, and as may not provide consistently reliable information. After this point, however, benthic diatoms become substantially less common in the sediment record and L. intermedia becomes much more abundant. Throughout subzone 2b, inferred mixing depths suggest that the lake was mixing fairly deeply, with the thickness of the epilimnion typically ranging between 12 and 10 m. Throughout DZ3, the inferred mixing depths show a progressive decline; the average thickness of the epilimnion decreases by nearly 3 m from the top of sub-zone 2b to the top of sub-zone 3b. Sub-zone 4a represents a period of relatively stable thermal stratification, with the epilimnion averaging around 8.5–9 m in thickness throughout. Through sub-zone 4b, the DI-MD suggests an abrupt deepening of the thermocline, which averages ∼10–10.5 m. This condition abruptly reverses in the final sub-zone, with the epilimnion thickness dropping to approximate 8 m in modern samples.
An idealized sequence for dimictic temperate lakes follows a progression with seasonal variation in radiation (Ragotzkie, 1978). Starting from complete ice cover in the winter, dimictic lakes are inversely stratified. As solar irradiance increases in the spring and winter air temperatures warm, this leads to ice break up which culminates with complete mixing of the water column under isothermic water conditions. Through spring and into early summer, thermoclines gradually develop, usually punctuated by short-lived destratification events until the thermocline strengthens. With increasing solar irradiance, thermal stratification is enhanced throughout the summer months. Solar irradiance and air temperatures decline into the fall, where destratification events lead to a return of isothermal lake conditions, eventually leading to sustained cooling of the surface waters and ice cover (Catalan et al., 2002; Ragotzkie, 1978).
In temperate lakes, the thickness of the mixed layer is the result of a complex interaction between multiple factors: solar irradiance, light penetration, wind duration and intensity, and air temperature (Ragotzkie, 1978; Schindler, 1997; Livingstone and Dokulil, 2001; Catalan et al., 2002). The impact of each of these factors upon the thermal structure of any lake is strongly influenced by basin morphometry. For example, the influence of wind intensity and duration is strongly controlled by the surface area and the surface area to depth ratio (Fee et al., 1996; Robertson and Ragotzkie, 1990). Empirical studies have shown that a primary control over summer mixing depth is the maximum fetch (Ragotzkie, 1978; Gorham and Boyce, 1989; Håkanson, 2005), which determines the ability of wind energy to induce mixing in stratified lake systems. Water clarity and solar irradiance are also major factors that determine the depth of the mixed layer; however, these elements typically have a larger influence on lakes with smaller surface areas, where fetch is less influential (Ragotzkie, 1978; Sterner, 1990; Fee et al., 1996; Persson and Jones, 2008; von Einem and Graneli, 2010).
While air temperature has limited direct control over lake thermal structure, it can strongly influences the seasonal duration of ice and snow cover. Hence air temperature most commonly influences lake ice phenology, which indirectly influences the timing of light penetration and transference of energy through wind. However, lake ice phenology, particularly freeze-up, is still strongly influenced by basin morphology (Ragotzkie, 1978; Robertson and Ragotzkie, 1990). In alpine lake systems, air temperature can also substantially alter landscape-lake interactions by changing the basin vegetation, soil development rates and frequency of fires. Thus air temperature may also indirectly modify factors that influence water clarity, such as soil erosion, inputs of dissolved organic matter, and nutrient fluxes to lake systems (Wright and Bailey, 1982; Bayley et al., 1992; Fee et al., 1996; Fritz et al., 2004; Rosén and Hammarlund, 2007; Saros et al., 2012).
Below we interpret the observed changes in the diatom assemblages throughout the last 8.5 ka using a combination of inferences based upon the changes in diatom assemblages within each zone, the calculated planktic to benthic ratio, DI-MD, and our modeled three-dimension morphology of the lake basin. It is important to note that while the lake basin has probably infilled somewhat through the Holocene, sedimentation rates are exceptionally low in Hidden Lake, thus the three-dimensional model we have created based upon modern sediment-water interfaces probably is not substantially different (less than 1 m different) from those that would have been present in the lake throughout the Holocene.
We interpret the earliest part of the diatom assemblage record (DZ1) to represent a deep, alkaline lake. We infer the high proportion of tychoplanktic diatoms in this zone and throughout the core (Figure 6) to generally reflect that the lake regularly undergoes seasonal moating during the spring and early summer in response to snow and ice cover (Lotter and Bigler, 2000). Planktic to benthic ratios increase at the base of DZ1, which we interpret as gradually rising lake levels. Hidden Lake occurs in a glaciated basin. This change in lake level probably represents gradual melting of the local glacier systems in response to early Holocene warming. DI-MD through this zone ranges from ∼8 to 9 m and is highly stable throughout most of the zone. Because of the shallow mixing depths inferred, we interpret that DZ1 represented a period when lake elevations were shallower than 1945.5 m asl, the lake surface area was substantially smaller in the deep sub-basin than the modern lake, and the effective fetch was lower, resulting in a shallow mixed layer. At lake surface elevations below this depth, our modeled lake suggests that the modern deep sub-basin would have been completely isolated from the rest of Hidden Lake (see Figure 4C).
In this zone, we infer a substantial change in lake depth occurred, leading to an abrupt increase in benthic taxa comprised mostly of monoraphid diatoms. The dominant benthic diatom taxa in this zone, represented by K. suchlandtii, K. nitidiformis, and Cocconeis sp., probably reflect an abundance of submerged aquatic macrophytes or other benthic substrates in the lake environment. The strong threshold-like response of the diatom record is probably the result of an interaction between lake elevation and the complex basin morphology, which led to an expansion of benthic diatom habitats. An inferred increase in macrophyte habitat area suggests that water transparency conditions also may have increased relative to those in DZ1. Because of the complex basin morphology, an increase in available benthic habitat might indicate either an increase or decrease in lake level (relative to DZ1). For example, modeled surface areas in the lake suggest that the greatest increase in benthic habitat area for the deepest sub-basin actually occurs when the lake surface elevation is around 1946 m asl (∼1.5 m below modern lake levels). We propose two potential models for the transitions observed in DZ2.
One explanation for the observed trends in the diatom assemblage data in DZ2 is that the deep sub-basin remained isolated throughout the entire period. In this ‘shallow lake’ model, lake levels were probably low enough (or the water column was clear enough) that the entire lake floor of the deep sub-basin was sunlight, effectively maximizing the benthic habitat of the lake. A compelling argument supporting this idea is that the DZ2 diatom assemblage, dominated by benthic diatoms, is unique to the lower part of the record, suggesting that the lake has not since returned to this state in the entire Holocene. In other words, this was an unusual occurrence and not something that could be reproduced by relatively small changes in regional hydroclimate variability; and thus a fairly large change in lake level (and transparency) must have occurred to produce this change in the assemblage because of deep nature of the lake basin.
An alternative explanation for the abrupt increase in benthic diatom species in DZ2 is that the diatom assemblages in DZ2a represent the joining of all sub-basins to form one continuous lake, but where the available benthic habitat area was substantially higher than it is in the modern lake, perhaps 1–1.5 m below the modern lake level (see Figure 4B). A compelling argument for this interpretation is that reconstructed DI-MD during DZ1 indicates that the lake was more stratified (i.e., shallower mixed layer) than DZ2. A shallower DI-MD would be produced by a lake that had a smaller maximum effective fetch (reducing its surface area). Interestingly, the upper part of DZ2 (2b) is defined by the first major occurrence of L. intermedia and our DI-MD suggests that the mixing depth was abruptly quite a bit deeper (>11 m) during this interval. Benthic diatom species still dominate the fossil assemblage in DZ2b, but these gradually decline as L. intermedia increases and inferred mixing peaks. Given the dependence upon mixing depth for the planktic species in the diatom assemblage, DZ2b could represent a gradual deepening of the lake, resulting in an increase in maximum effective fetch at the core site. Given the relatively small change in lake level required to isolate the deep sub-basin of Hidden Lake, the lake ‘deepening DZ2 lake’ model described above relies upon the idea that only very small changes in lake level were required to produce a very large change in the mixing depth, because the fetch increased substantially and imparted a greater energy to the upper mixed layer. However, this model also suggested that similar benthic habitat expansion did not recur throughout the remainder of the Holocene, and thus very limited changes in lake level have would have occurred since DZ2b. However, the higher energy state of the lake, resulting from increasing fetch, potentially could have destroyed the potential for macrophyte growth throughout the Holocene, meaning that a return to a similar lake state might not have been possible even if lake levels were at similar elevations at some later point in the Holocene.
The abrupt nature of this transition in both planktic to benthic ratio and DI-MD creates an interesting dilemma. Either lake levels shallowed drastically, exposing the deep sub-basin floor to sunlight, or lake levels simply rose gradually and connected the sub-basins, increasing the benthic habitat in the process and increasing fetch to increase lake mixing depths. We favor the deepening lake DZ2 model, since it relies on less abrupt changes in lake level and might represent a continuation of rising lake levels resulting from glacial melting in response to warming through the Holocene Climatic Optimum.
In this period, the lake begins to establish conditions that are more similar to the modern lake. Benthic species become substantially reduced at the transition to DZ3a, suggesting that lake levels initially continued to rise very gradually. Midway through DZ3 (3b), Discostella stelligera makes it first substantial appearance in the record. However, the fact that Lindavia intermedia does not decline substantially at any point in DZ3 suggests that rather than a major change in lake level, this transition may reflect an enhanced influence of seasonality in the thermal structure of the lake. One way this might be produced is by warmer average temperatures during the winter season, resulting in a longer ice-free period. Evidence of increased warming throughout the mid-Holocene period is common from many other lake records from this region of the Rocky Mountains (Bracht et al., 2008; Shapley et al., 2009; Shuman et al., 2009; Whitlock et al., 2012; Slemmons et al., 2015; Stone et al., 2016). Alternatively, it might be the result of the decline in average lake depth with increasing total lake depth, leading to warmer water settings in the epilimnion and accelerated onset of seasonal lake stratification. A similar process might also result from enhanced development of soils in the basin and a concomitant decline in water clarity. Several recent studies (Saros et al., 2014; Malik and Saros, 2016; Malik et al., 2017) have shown a relationship between L. radiosa and higher light exposure. Although L. radiosa is present in low relative abundance in the sediment record, these observations support the idea that the replacement of L. radiosa with D. stelligera at the top of this zone may be partially related to a change in water clarity.
The planktic to benthic ratio throughout the upper half of the core expresses a greater level of stability than earlier periods in the sediment record. On average, planktic species are more abundant, tychoplankton is slightly lower. After ∼3 ka, the planktic diatom abundance (particularly L. comensis) generally increases. The last 200 years of the record show a sharp rise in species indicative of increased stratification, probably associated with increasing temperatures, which has been previously addressed in Saros et al. (2012). Tychoplanktic species, which in this setting probably represent intermediate lake depths and ice moating conditions (Lotter and Bigler, 2000), rapidly decline in response to 20th century warming, which is also evidenced throughout Glacier National Park by the loss of glaciers and snowpack (Pederson et al., 2004, 2013).
Most regional records suggest that the conditions for the Rocky Mountains in the late Holocene were generally cooler and/or wetter between 3,500 and 1,400 years ago (Osborn and Karlstrom, 1988; Luckman et al., 1993; Palmer et al., 2002; Stevens et al., 2006; Stone and Fritz, 2006; Chase et al., 2008; Walker and Pellatt, 2008; Shuman et al., 2009). Records from nearby paleolimnological sites suggest it was probably mostly warmer but substantially wetter in the past 1,400 years (Stevens et al., 2006; Bracht et al., 2008; Shapley et al., 2009; Shuman et al., 2009). Interestingly, Stone et al. (2016) provided clear evidence of coherent trends of increased lake mixing (diametric to inferred changes observed here) across three lakes in the US Rocky Mountains throughout this period, which were interpreted to result from increased winter storms linked to phase changes in the Aleutian Low. Outside of the last 200 years, sedimentation rates within the lake have not undergone major changes, suggesting changes in nutrient loading, which may have substantially affected light penetration, have probably not occurred. Therefore, we interpret the discrepancy between shallower mixing depths and the expected response to cooler climate conditions through this period to suggest that Hidden Lake’s thermal structure may have been influenced by the lake basin morphology.
Hidden Lake has a complex basin morphometry, and morphometric parameters that significantly influence mixing depth (particularly surface area and fetch) and are highly dependent upon lake level (Figure 3). Three-dimensional modeling indicates that at a lake surface elevation threshold 2–3 m below that of the modern lake, the deep basin of the lake would become isolated from the rest of the lake (Figure 4), with a considerably smaller surface area and effective fetch. Since fetch plays a significant role in average lake mixing depth, a substantial decrease in effective fetch as a result of lower lake surface elevation explains the observed shift in mixing depths toward shallower values, even under cooler conditions. A change in effective fetch with lake-level also provides an explanation for the relationship between changes in lake mixing and changing benthic habitats: as the lake level continues to rise to its modern elevation, available benthic habitats expand as the lake begins to flood the surrounding terrain. Ultimately, once the lake surface elevation increases enough that it joins with the adjacent sub-basins, wind intensity picks up considerably with increasing effective fetch, substantially increasing lake mixing, which leads to the sudden increase of L. intermedia at the base of sub-zone 4b. This reverses briefly during the period of the Little Ice Age, when plankton losses are generally associated with increases in S. pinnata, likely as a result of enhanced snow/ice cover and decreased planktic productivity.
The broader context of these results and their implications for the Late Holocene can best be observed as state changes in the average mixing depth of Hidden Lake in association with changes in hydroclimate (Figure 8). The general patterns of lake mixing inferred in the Hidden Lake record tend to be coherent with previously reported regional stratification indicators (Stone et al., 2016) prior to about 3.5 ka. However, after this point, changes in lake level have a profound influence on the response on the thermal structure of Hidden Lake. When other regional records of effective moisture suggest lower lake states associated with the period of glacial advances, between 3.5 and 1.4 ka (Stevens et al., 2006; Shapley et al., 2009; Shuman et al., 2009), the diatom-inferred mixing depth is typically asynchronous with other regional records of lake mixing. We attribute these differences to hydroclimate variability obfuscating the response of lake mixing. Around 1.4 ka, when the records from Foy and Jones Lakes show substantial deepening and freshening, the diatom-inferred mixing depth from Hidden Lake suggests much deeper mixing (Figure 8, blue bar). We associate this change with a pronounced change in lake fetch, associated with the lake at its deepest state. The complexity of the Hidden Lake basin morphology appears to have a distinct impact on the lake response to thermal stratification, but the timing of major changes in variability closely mirrors substantial changes in effective moisture in the region.
Figure 8. Comparison of Hidden Lake record with other regional diatom records over the past 4.5 ka. Diatom-inferred stratification indices from three nearby lakes (left, modified from Stone et al., 2016) and hydroclimate variability inferred from the percent planktic diatoms from Foy Lake (right, modified from Stone and Fritz, 2006). Diatom-inferred mixing depth (DI-MD) plotted on a reference line indicating average mixing depth in meters. Yellow bar represents the period before major state changes in stratification observed in Stone et al. (2016) associated with regional glacial advances. Blue bar represents enhanced moisture periods observed in Foy and Jones Lakes (Stone and Fritz, 2006; Shapley et al., 2009).
Between 8.64 and 7.61 ka the diatom record from Hidden Lake suggests that the lake was deep and fresh, with limited benthic habitat, but lower than the modern lake system. After 7.61 ka, the lake level likely rose, inundating the surrounding lake marginal area and expanding the available benthic diatom habitat substantially. Between 6.18 and 4.13 ka, lake level probably declined somewhat, leading to enhanced seasonal stratification. This pattern of enhanced aridity in the mid-Holocene has been observed in many lake records throughout the western US (Shuman et al., 2009; Williams et al., 2009). After 1.4 ka, the record from Hidden Lake suggests that the lake became deeper and less stratified in response to the effects of enhanced fetch.
The results of this research highlight the importance of considering the basin morphology and the potential impacts of fetch when reconstructing changes in lake records that may undergo substantial lake level change. Additionally, this study exemplifies the strengths of using neo-ecological techniques and three-dimensional lake morphometry modeling together when interpreting paleolimnological records using fossil diatom assemblages. The early part of the record is marked by significant changes in lake level that are manifested as changes in the planktic to benthic ratio. Planktic species from the fossil record exhibit long-term changes in mixing depth through the Holocene record from Hidden Lake; the dynamic changes in plankton are particularly helpful for interpreting past lake conditions where the direction of lake level changes are difficult to determine. Because of the complex lake basin morphometry, the interaction of effective fetch and lake level change may be responsible for modifying the lake’s response to long-term climatological changes in hydroclimate. Importantly, exploring the interaction between hydroclimate variability and thermal structure showcases how sets of regionally coherent lake records can help to disentangle the signals from records with non-linear responses in complex lake basins.
The influence of changing lake levels on past fetch conditions has not often been the focus of paleolimnological research, but the combination of three-dimensional lake modeling, combined with changes in the planktic to benthic ratio and robust changes in the long-term diatom-inferred mixing depths may provide new opportunities to explore this approach, particularly in lake basins with complex morphologies.
The raw data supporting the conclusions of this manuscript will be made available by the authors, without undue reservation, to any qualified researcher.
JRS was the lead composer of the body of the text. In addition, JRS developed the lake bathymetry, developed the three-dimensional modeling and morphometric analyses, diatom analysis interpretation, statistical analyses, and was responsible for core collection, sub-sampling, and age modeling. JES was the secondary composer of the body of the text, provided a conceptual basis for the research, and was primarily responsible for the design of neo-ecological experiments and their analyses and assisted with diatom interpretation. TS was responsible for diatom analyses and assisted with the concept of the manuscript text.
This work was supported by a grant from the National Science Foundation (Ecology DEB#0639901).
The authors declare that the research was conducted in the absence of any commercial or financial relationships that could be construed as a potential conflict of interest.
Karlyn Westover and Audrey Bamberg assisted with the collection of sediment cores and bathymetric data. Steve Wooden processed and prepared the diatom slides. Lab preparations and microscope analyses were performed at the Fritz Lab (University of Nebraska–Lincoln). Discussions with David Jewson and comments from Sherilyn C. Fritz, Karlyn Westover, two reviewers, and Lesleigh Anderson improved clarity of the manuscript.
The Supplementary Material for this article can be found online at: https://www.frontiersin.org/articles/10.3389/feart.2019.00028/full#supplementary-material
Anderson, N. J. (1990). Spatial pattern of recent sediment and diatom accumulation in a small, monomictic, eutrophic lake. J. Paleolimnol. 3, 143–160. doi: 10.1007/BF00414068
Appleby, P. G., and Oldfield, F. (1978). The calculation of lead-210 dates assuming a constant rate of supply of unsupported 210Pb to the sediment. Catena 5, 1–8. doi: 10.1016/S0341-8162(78)80002-80002
Bachmann, R. W., Hoyer, M. V., and Canfield, D. E. J. (2000). The potential for wave disturbance in shallow Florida lakes. Lakes Reserv. Manag. 16, 281–291. doi: 10.1080/07438140009354236
Bahls, L. L. (2004). Northwest Diatoms: A Photographic Catalogue of Species in the Montana Diatom Collection with Ecological Optima, Associates, and Distribution Records for the Nine Northwestern United States. Helena: Montana Diatom Collection, 1.
Bahls, L. L. (2005). Northwest Diatoms: A Photographic Catalogue of Species in the Montana Diatom Collection with Ecological Optima, Associates, and Distribution Records for the Nine Northwestern United States. Helena: Montana Diatom Collection, 2.
Battarbee, R. W. (2003). “Diatom analysis,” in Handbook of Holocene Palaeoecology and Palaeohydrology, eds B. E. Berglund and M. Ralska-Jasiewiczowa (Caldwell, NJ: Blackburn Press), 527–570.
Bayley, S. E., Schindler, D. W., Beaty, K. G., Parker, B. R., and Stainton, M. P. (1992). Effects of multiple fires on nutrient yields from streams draining boreal forest and fen watersheds: nitrogen and phosphorus. Can. J. Fish. Aquat. Sci. 49, 584–596. doi: 10.1139/f92-068
Bernhardt, J., Elliott, J. A., and Jones, I. D. (2008). Modelling the effects on phytoplankton communities of changing mixed depth and background extinction coefficient on three contrasting lakes in the English Lake District. Freshw. Biol. 53, 2573–2586. doi: 10.1111/j.1365-2427.2008.02083.x
Blaauw, M., and Christen, J. A. (2011). Flexible paleoclimate age-depth models using an autoregressive gamma process. Bayesian Anal. 6, 457–474. doi: 10.1214/11-BA618
Blais, J. M., and Kalff, J. (1995). The influence of lake morphometry on sediment focusing. Limnol. Oceanogr. 40, 582–588. doi: 10.4319/lo.1995.40.3.0582
Bracht, B. B., Stone, J. R., and Fritz, S. C. (2008). A diatom record of late Holocene climate variation in the Northern Range of Yellowstone National Park. USA. Quat. Int. 188, 149–155. doi: 10.1016/j.quaint.2007.08.043
Camburn, K. E., and Charles, D. F. (2000). Diatoms of Low-Alkalinity Lakes in the Northeastern United States. Philadelphia: The Academy of Natural Sciences of Philadelphia.
Carrick, H. J., Aldridge, F. J., and Schelske, C. L. (1993). Wind influences phytoplankton biomass and composition in a shallow, productive lake. Limnol. Oceanogr. 38, 1179–1192. doi: 10.4319/lo.1993.38.6.1179
Catalan, J., Ventura, M., Brancelj, A., Granados, I., Thies, H., Nickus, U., et al. (2002). Seasonal ecosystem variability in remote mountain lakes: implications for detecting climatic signals in sediment records. J. Paleolimnol. 28, 25–46. doi: 10.1023/A:1020315817235
Chase, M., Bleskie, C., Walker, I. R., Gavin, D. G., and Hu, F. S. (2008). Midge-inferred Holocene summer temperatures in Southeastern British Columbia, Canada. Palaeogeogr. Palaeoclimatol. Palaeoecol. 257, 244–259. doi: 10.1016/j.palaeo.2007.10.020
Cumming, B. F., Wilson, S. E., Hall, R. I., and Smol, J. P. (1995). “Bibliotheca diatomologica,” in Diatoms from British Columbia Lakes, Vol. 31, ed. H. Lange-Bertalot (Berlin: J. Cramer).
Davies-Colley, R. J. (1988). Mixing depths in New Zealand lakes. New Zeal. J. Mar. Freshw. Res. 22, 517–528. doi: 10.1080/00288330.1988.9516322
Diehl, S., Berger, S., Ptacnik, R., and Wild, A. (2002). Phytoplankton, light, and nutrients in a gradient of mixing depths: field experiments. Ecology 83, 399–411. doi: 10.1890/0012-9658(2002)083[0399:PLANIA]2.0.CO;2
Evans, R. D. (1994). Empirical evidence of the importance of sediment resuspension in lakes. Hydrobiologia 284, 5–12. doi: 10.1007/BF00005727
Fallu, M.-A., Allaire, N., and Pienitz, R. (2000). Biblotheca Diatomologica Band 45: Freshwater Diatoms from northern Québec and Labrador (Canada) - Species-Environment Relationships in Lakes of Boreal Forest, Forest-Tundra and Tundra Regions. Berlin: J. Cramer.
Fee, E. J. (1979). A relation between lake morphometry and primary productivity and its use in interpreting whole-lake eutrophication experiments. Limnol. Oceanogr. 24, 401–416. doi: 10.4319/lo.1979.24.3.0401
Fee, E. J., Hecky, R. E., Kasian, S. E. M., and Cruikshank, D. R. (1996). Effects of lake size, water clarity, and climatic variability on mixing depths in Canadian shield lakes. Limnol. Oceanogr. 41, 912–920. doi: 10.4319/lo.1996.41.5.0912
Foged, N. (1981). Diatoms in Alaska, Bibliotheca Phycologica, Vol. 53. Port Jervis, NY: Lubrecht & Cramer, Limited.
Fritz, S. C. (1996). Paleolimnological records of climatic change in North America. Limnol. Oceanogr. 41, 882–889. doi: 10.4319/lo.1996.41.5.0882
Fritz, S. C. (2008). Deciphering climatic history from lake sediments. J. Paleolimnol. 39, 5–16. doi: 10.1007/s10933-007-9134-x
Fritz, S. C., Engstrom, D. R., and Juggins, S. (2004). Patterns of early lake evolution in boreal landscapes: a comparison of stratigraphic inferences with a modern chronosequence in Glacier Bay. Alaska. Holocene 14, 828–840. doi: 10.1191/0959683604hl763rp
Fritz, S. C., Ito, E., Yu, Z., Laird, K. R., and Engstrom, D. R. (2000). Hydrologic variation in the Northern Great Plains during the last two millennia. Quat. Res. 53, 175–184. doi: 10.1006/qres.1999.2115
Golden Software (2010). Surfer (Version 10). Golden, CO: Golden Software, LLC. Available at: www.goldensoftware.com
Gorham, E., and Boyce, F. M. (1989). Influence of lake surface area and depth upon thermal stratification and the depth of the summer thermocline. J. Great Lakes Res. 15, 233–245. doi: 10.1016/S0380-1330(89)71479-9
Guiry, M., and Guiry, G. (2017). AlgaeBase. World-wide Electronic Publication. Galway: National University of Ireland.
Håkanson, L. (1981). A Manual of Lake Morphometry. Berlin: Springer-Verlag. doi: 10.1007/978-3-642-81563-8
Håkanson, L. (2005). The importance of lake morphometry for the structure and function of lakes. Int. Rev. Hydrobiol. 90, 433–461. doi: 10.1002/iroh.200410775
Håkanson, L., and Jansson, M. (1983). Principles of Lake Sedimentology. Berlin: Springer. doi: 10.1007/978-3-642-69274-1
Hamilton, D. P., and Mitchell, S. F. (1996). An empirical model for sediment resuspension in shallow lakes. Hydrobiologia 317, 209–220. doi: 10.1371/journal.pone.0131431
Houser, J. N. (2006). Water color affects the stratification, surface temperature, heat content, and mean epilimnetic irradiance of small lakes. Can. J. Fish. Aquat. Sci. 63, 2447–2455. doi: 10.1139/F06-131
Juggins, S. (2015). Rioja: Analysis of Quaternary Science Data, R package Version (0.9-9). Available at: https://cran.r-project.org/web/packages/rioja/index.html.
Kingsbury, M. V., Laird, K. R., and Cumming, B. F. (2012). Consistent patterns in diatom assemblages and diversity measures across water-depth gradients from eight Boreal lakes from north-western Ontario (Canada). Freshw. Biol. 57, 1151–1165. doi: 10.1111/j.1365-2427.2012.02781.x
Lange-Bertalot, H., Kulbs, K., Lauser, T., Norpel-Schempp, M., and Willmann, M. (1996). “Iconographia Diatomologica,” in Diatom Taxa Introduced by Georg Krasske Documentation and Revision, Vol. 3, ed. H. Lange-Bertalot Koenigstein (Königstein: Koeltz Scientific Books).
Lehman, J. T. (1975). Reconstructing the rate of accumulation of lake sediment: the effect of sediment focusing. Quat. Res. 5, 541–550. doi: 10.1016/0033-5894(75)90015-0
Livingstone, D. M., and Dokulil, M. T. (2001). Eighty years of spatially coherent Austrian lake surface temperatures and their relationship to regional air temperature and the North Atlantic Oscillation. Limnol. Oceanogr. 46, 1220–1227. doi: 10.4319/lo.2001.46.5.1220
Lotter, A. F., and Bigler, C. (2000). Do diatoms in the Swiss Alps reflect the length of ice-cover? Aquat. Sci. 62, 125–141. doi: 10.1007/s000270050002
Luckman, B. H., Holdsworth, G., and Osborn, G. D. (1993). Neoglacial glacier fluctuations in the Canadian Rockies. Quat. Res. 39, 144–153. doi: 10.1006/qres.1993.1018
Malik, H. I., Northington, R. M., and Saros, J. E. (2017). Nutrient limitation status of Arctic lakes affects the responses of Cyclotella sensu lato diatom species to light: implications for distribution patterns. Polar Biol. 40, 2445–2456. doi: 10.1007/s00300-017-2156-2156
Malik, H. I., and Saros, J. E. (2016). Effects of temperature, light and nutrients on five Cyclotella sensu lato taxa assessed with in situ experiments in arctic lakes. J. Plankton Res. 38, 431–442. doi: 10.1093/plankt/fbw002
Mason, I. M., Guzkowska, M. A. J., Rapley, C. G., and Street-Perrott, F. A. (1994). The response of lake levels and areas to climatic change. Clim. Change 27, 161–197.
Osborn, G. D., and Karlstrom, E. T. (1988). Holocene history of Bugaboo glacier. Br. Columbia. Geol. 16, 1015–1017. doi: 10.1130/0091-7613(1988)016<1015:HHOTBG>2.3.CO;2
Palmer, S., Walker, I. R., Heinrichs, M. L., Hebda, R. J., and Scudder, G. (2002). Postglacial midge community change and Holocene palaeotemperature reconstructions near treeline, southern British Columbia (Canada). J. Paleolimnol. 28, 469–490. doi: 10.1023/A:1021644122727
Pal’shin, N. I., Efremova, T. V., and Potakhin, M. S. (2008). The effect of morphometric characteristics and geographic zonality on thermal stratification of lakes. Water Resour. 35, 191–198. doi: 10.1134/S0097807808020073
Pederson, G. T., Betancourt, J. L., and McCabe, G. J. (2013). Regional patterns and proximal causes of the recent snowpack decline in the Rocky Mountains. U.S. Geophys. Res. Lett. 40, 1811–1816. doi: 10.1002/grl.50424
Pederson, G. T., Fagre, D. B., Gray, S. T., and Graumlich, L. J. (2004). Decadal-scale climate drivers for glacial dynamics in Glacier National Park, Montana, USA. Geophys. Res. Lett. 31:L12203. doi: 10.1029/2004GL019770
Persson, I., and Jones, I. D. (2008). The effect of water colour on lake hydrodynamics: a modelling study. Freshw. Biol. 53, 2345–2355. doi: 10.1111/j.1365-2427.2008.02049.x
Ragotzkie, R. A. (1978). “Heat budgets of lakes,” in Lakes: Chemistry, Geology, Physics, ed. A. Lerman (New York, NY: Springer-Verlag), 1–19.
Rea, T. E., Karapatakis, D. J., Guy, K. K., Pinder, J. E. I., and Mackey, H. E. J. (1998). The relative effects of water depth, fetch and other physical factors on the development of macrophytes in a small southeastern US pond. Aquat. Bot. 61, 289–299. doi: 10.1016/S0304-3770(98)00069-2
Robertson, D. M., and Ragotzkie, R. A. (1990). Thermal structure of a multibasin lake: influence of morphometry, interbasin exchange, and groundwater. Can. J. Fish. Aquat. Sci. 47, 1206–1212. doi: 10.1139/f90-140
Rosén, P., and Hammarlund, D. (2007). Effects of climate, fire and vegetation development on Holocene changes in total organic carbon concentration in three boreal forest lakes in northern Sweden. Biogeosciences 4, 975–984. doi: 10.5194/bgd-4-1329-2007
Saros, J. E., and Anderson, N. J. (2015). The ecology of the planktonic diatom Cyclotella and its implications for global environmental change studies. Biol. Rev. 90, 522–541. doi: 10.1111/brv.12120
Saros, J. E., Stone, J. R., Pederson, G. T., Slemmons, K. E. H., Spanbauer, T. L., Schliep, A., et al. (2012). Climate-induced changes in lake ecosystem structure inferred from coupled neo- and paleoecological approaches. Ecology 93, 2155–2164. doi: 10.1890/11-2218.1
Saros, J. E., Strock, K. E., Mccue, J., Hogan, E., and Anderson, N. J. (2014). Response of Cyclotella species to nutrients and incubation depth in Arctic lakes. J. Plankton Res. 36, 450–460. doi: 10.1093/plankt/fbt126
Schindler, D. W. (1997). Widespread effects of climatic warming on freshwater ecosystems in North America. Hydrol. Process. 11, 1043–1067. doi: 10.1002/(SICI)1099-1085(19970630)11:8<1043::AID-HYP517>3.0.CO;2-5
Scott, J. T. (1964). A Comparison of the Heat Balance of Lakes in Winter. Ph.D. thesis, Wisconsin University, Madison.
Shapley, M. D., Ito, E., and Donovan, J. J. (2009). Lateglacial and Holocene hydroclimate inferred from a groundwater flow-through lake, Northern Rocky Mountains, USA. Holocene 19, 523–535. doi: 10.1177/0959683609104029
Shuman, B., Henderson, A. K., Colman, S. M., Stone, J. R., Fritz, S. C., Stevens, L. R., et al. (2009). Holocene lake-level trends in the Rocky Mountains. U.S.A. Quat. Sci. Rev. 28, 1861–1879. doi: 10.1016/j.quascirev.2009.03.003
Slemmons, K. E. H., Saros, J. E., Stone, J. R., McGowan, S., Hess, C. T. T., and Cahl, D. (2015). Effects of glacier meltwater on the algal sedimentary record of an alpine lake in the central US Rocky Mountains throughout the late Holocene. J. Paleolimnol. 53, 385–399. doi: 10.1007/s10933-015-9829-9823
Smith, I. R., and Sinclair, I. J. (1972). Deep water waves in lakes. Freshw. Biol. 2, 387–399. doi: 10.1111/j.1365-2427.1972.tb00378.x
Snucins, E., and Gunn, J. (2000). Interannual variation in the thermal structure of clear and colored lakes. Limnol. Oceanogr. 45, 1639–1646. doi: 10.4319/lo.2000.45.7.1639
Spaulding, S. A., Lubinski, D. J., and Potapova, M. G. (2010). Diatoms of the United States. Available at: http://westerndiatoms.colorado.edu/ [accessed March 29, 2018].
Sterner, R. W. (1990). Lake morphometry and light in the surface layer. Can. J. Fish. Aquat. Sci. 47, 687–692.
Stevens, L. R., Stone, J. R., Campbell, J., and Fritz, S. C. (2006). A 2200-yr record of hydrologic variability from Foy Lake, Montana, USA, inferred from diatom and geochemical data. Quat. Res. 65, 264–274. doi: 10.1016/j.yqres.2005.08.024
Stone, J. R., and Fritz, S. C. (2004). Three-dimensional modeling of lacustrine diatom habitat areas: improving paleolimnological interpretation of planktic:benthic ratios. Limnol. Oceanogr. 49, 1540–1548. doi: 10.4319/lo.2004.49.5.1540
Stone, J. R., and Fritz, S. C. (2006). Multidecadal drought and Holocene climate instability in the Rocky Mountains. Geology 34, 409–412. doi: 10.1130/G22225.1
Stone, J. R., Saros, J. E., and Pederson, G. T. (2016). Coherent late-Holocene climate-driven shifts in the structure of three Rocky Mountain lakes. Holocene 26, 1103–1111. doi: 10.1177/0959683616632886
Stuiver, M., Reimer, P. J., Bard, E., Beck, J. W., Burr, G. S., Hughen, K. A., et al. (1998). INTCAL98 Radiocarbon age calibration 24,000 - 0 cal BP. Radiocarbon 40, 1041–1083. doi: 10.1017/S0033822200019123
Thomaz, S. M., Souza, D. C., and Bini, L. M. (2003). Species richness and beta diversity of aquatic macrophytes in a large subtropical reservoir (Itaipu Reservoir, Brazil): the influence of limnology and morphometry. Hydrobiologia 505, 119–128. doi: 10.1023/B:HYDR.0000007300.78143.e1
Verschuren, D. (1999). Influence of depth and mixing regime on sedimentation in a small, fluctuating tropical soda lake. Limnol. Oceanogr. 44, 1103–1113. doi: 10.4319/lo.1999.44.4.1103
von Einem, J., and Graneli, W. (2010). Effects of fetch and dissolved organic carbon on epilimnion depth and light climate in small forest lakes in southern Sweden. Limnol. Oceanogr. 55, 920–930. doi: 10.4319/lo.2010.55.2.0920
Walker, I. R., and Pellatt, M. (2008). Climate change and ecosystem response in the northern Columbia River basin - A paleoenvironmental perspective. Environ. Rev. 16, 113–140. doi: 10.1139/A08-004
Whitlock, C., Dean, W. E., Fritz, S. C., Stevens, L. R., Stone, J. R., Power, M. J., et al. (2012). Holocene seasonal variability inferred from multiple proxy records from Crevice Lake, Yellowstone National Park, USA. Palaeogeogr. Palaeoclimatol. Palaeoecol. 33, 90–103. doi: 10.1016/j.palaeo.2012.03.001
Whitmore, T. J., Brenner, M., and Schelske, C. L. (1996). Highly variable sediment distribution in shallow, wind-stressed lakes: a case for sediment-mapping surveys in paleolimnological studies. J. Paleolimnol. 15, 207–221. doi: 10.1007/BF00213041
Wigdahl, C. R., Saros, J. E., Fritz, S. C., Stone, J. R., and Engstrom, D. R. (2014). The influence of basin morphometry on the regional coherence of patterns of diatom-inferred salinity in lakes of the northern Great Plains (USA). Holocene 24, 603–613. doi: 10.1177/0959683614523154
Williams, J. W., Shuman, B., and Bartlein, P. J. (2009). Rapid responses of the prairie-forest ecotone to early Holocene aridity in mid-continental North America. Glob. Planet. Change 66, 195–207. doi: 10.1016/j.gloplacha.2008.10.012
Wolin, J. A., and Stone, J. R. (2010). “Diatoms as indicators of water-level change in freshwater lakes,” in The Diatoms: Applications for Environmental and Earth Sciences, eds E. F. Stoermer and J. P. Smol (Cambridge: Cambridge University Press), 174–185. doi: 10.1017/CBO9780511763175.010
Keywords: diatoms, fetch, paleolimnology, thermal structure, lakes, lake level, Holocene
Citation: Stone JR, Saros JE and Spanbauer TL (2019) The Influence of Fetch on the Holocene Thermal Structure of Hidden Lake, Glacier National Park. Front. Earth Sci. 7:28. doi: 10.3389/feart.2019.00028
Received: 02 October 2018; Accepted: 05 February 2019;
Published: 22 February 2019.
Edited by:
Lesleigh Anderson, United States Geological Survey, United StatesReviewed by:
Jesse C. Vermaire, Carleton University, CanadaCopyright © 2019 Stone, Saros and Spanbauer. This is an open-access article distributed under the terms of the Creative Commons Attribution License (CC BY). The use, distribution or reproduction in other forums is permitted, provided the original author(s) and the copyright owner(s) are credited and that the original publication in this journal is cited, in accordance with accepted academic practice. No use, distribution or reproduction is permitted which does not comply with these terms.
*Correspondence: Jeffery R. Stone, amVmZmVyeS5zdG9uZUBpbmRzdGF0ZS5lZHU=
Disclaimer: All claims expressed in this article are solely those of the authors and do not necessarily represent those of their affiliated organizations, or those of the publisher, the editors and the reviewers. Any product that may be evaluated in this article or claim that may be made by its manufacturer is not guaranteed or endorsed by the publisher.
Research integrity at Frontiers
Learn more about the work of our research integrity team to safeguard the quality of each article we publish.