- Department of Atmospheric Sciences, University of Washington, Seattle, WA, United States
The ability of light-absorbing impurities (LAI) to darken snow had been known for decades, even inspiring practical applications, but quantification of the radiative forcing awaited radiative-transfer modeling in 1980 and measurement of soot in Arctic snow in 1983-4. Climate-modeling interest in this forcing began in 2004, spurring a modern explosion of research on several topics: methods to measure black carbon (BC) and other LAI, Arctic air pollution, measurement of BC mixing ratio in snow over large areas, and radiative transfer modeling of this forcing and its climatic and hydrological effects. The BC-content of snow in large remote regions of the northern hemisphere is on the order of 20 parts per billion, causing albedo reductions of ∼1–2%. This reduction is climatically significant but difficult to detect by remote sensing, so quantification requires fieldwork to collect and analyze snow samples. This review is a personal account of early research at the National Center for Atmospheric Research and the University of Washington, followed by a brief summary of recent work by the author and his colleagues.
Beginnings
That the albedo of snow is reduced by light-absorbing impurities (LAI) is obvious to the eye when the LAI arrive in a massive deposition event, for example when volcanic ash falls out onto Icelandic glaciers (e.g., Wittmann et al., 2017). Such observations have no doubt been made for centuries, and the consequences for snowmelt rate were probably also sometimes noticed long before any scientific study was undertaken. But the discovery that most snow in the northern hemisphere contains enough LAI to reduce its albedo by climatically significant, yet invisible, amounts was a surprise; it resulted from field measurements and modeling in the 1970s and 1980s, described below.
How I developed a research focus on LAI in snow takes some explaining. I was trained as a physical chemist, then got into biophysics research with my Ph.D. project to solve the three-dimensional atomic structure of an enzyme using X-ray crystallography (Warren et al., 1973). I continued in that field as a post doc in Germany, on the fascinating structural-biology problem of a virus that infects tobacco plants (Stubbs et al., 1977). Although the work was interesting, my mind kept wandering to thoughts of geography and climate.
The opportunity to change careers came when I was accepted as a post doc at the National Center for Atmospheric Research (NCAR) in Boulder, Colorado, for retraining in atmospheric physics. My sponsor was Steve Schneider. On my arrival at NCAR in January 1978 I told Steve that I was interested in the ice ages, so he introduced me to Warren Wiscombe, his colleague in the Climate Section. Steve knew that Wiscombe shared an interest in the ice ages, although there was no published evidence of that interest; Wiscombe’s focus was on developing methods for computing atmospheric radiative transfer, and he had become the world leader in that field. When I met him, I was ignorant of the fame of his great accomplishments; I was even unfamiliar with the term “radiative transfer.” He told me “We won’t solve the ice-age problem, but we’ll chip away at it. We’ll solve one small but important part of the ice-age problem – we’ll explain the albedo of snow.”
My 1st year at NCAR I worked with Steve Schneider on energy-balance climate modeling (Warren and Schneider, 1979); meanwhile I enrolled in a graduate course on radiative transfer taught by Gary Thomas at the University of Colorado (also in Boulder). Professor Thomas distributed his handwritten notes to the students, which were later published as an authoritative textbook (Thomas and Stamnes, 1999). After that training I was ready to start working with Wiscombe. [I’m using surnames here because their given names can cause confusion: my two mentors at NCAR were named “Steve” and “Warren”].
Radiative Transfer Model for Snow
We used one of the radiative-transfer models that Wiscombe had invented, the delta-Eddington method, to compute the variation of snow albedo with wavelength (Wiscombe and Warren, 1980). The single-scattering computation, using Wiscombe’s (1980) implementation of Mie theory, required as input the complex refractive index of ice, which I had to compile from numerous published laboratory measurements, and which I eventually published (Warren, 1984b) and more recently have updated (Warren and Brandt, 2008). At any given wavelength, the albedo depends on the snow grain size, the solar zenith angle, the partitioning of direct and diffuse irradiance, and the snow depth.
We modeled the snowpack as a pack of ice spheres, and argued that what really mattered was the area-to-mass ratio of the snow grains (now called “specific surface area”; I wish we had thought of that name!). The radius of the spheres in our model snowpack would then be three times the volume-to-area ratio of the real non-spherical snow grains. The errors in this spherical representation were later quantified for various snow-crystal shapes (Grenfell and Warren, 1999; Neshyba et al., 2003; Grenfell et al., 2005). The extinction efficiency and single-scattering albedo are well-represented by the equivalent spheres. The scattering asymmetry factor for the spheres is too large, but its effect can be compensated by reducing the model’s grain size (Dang et al., 2016).
The modeling showed that the most important variable determining snow albedo is the size of the snow grains. Physically, a photon traveling in a snowpack has a chance to change direction at each air/ice interface, and has a chance to be absorbed while traveling through ice. In coarse-grained snow, a photon travels a longer distance through ice between opportunities for scattering than in fine-grained snow, so it is more likely to be absorbed, and therefore a snowpack of coarse grains has lower albedo. The area/mass ratio normally decreases with snow age by “destructive metamorphism” (LaChapelle, 1969), leading to the “equilibrium form” (Colbeck, 1982), so the radiatively effective grain size increases, and the albedo drops.
We tested the model against measurements of near-infrared (near-IR) reflectance by O’Brien and Munis (1975), who had collected natural snow in the back yard of the United States Army’s Cold Regions Research and Engineering Laboratory (CRREL) in New Hampshire. They had brought the snow into a cold-room laboratory for radiation measurements (they did not measure the grain size). The introduction of their report tells their motivation for this research: “The probability of detecting [military equipment and personnel] depends upon the contrast in reflection of solar radiation from objects compared with that from snow-covered backgrounds.”
To match the measurements of O’Brien and Munis for new snow and 2-day-old snow, we needed grain radii r = 50 μm and r = 200 μm, respectively. Our model, using grain radius as a free parameter, agreed well enough with the measurements, considering that the measurements were actually not of albedo but rather of bidirectional reflectance at a particular pair of angles (source 0°, detector 30°).
What’s Wrong With the Model?
At shorter wavelengths we ran into trouble. The most accurate measurements of visible albedo were those of Grenfell and Maykut (1977; hereafter GM) for snow at a research camp on Ice Island T-3 in the Arctic Ocean. [Tom Grenfell made the measurements in 1974, and Gary Maykut helped with the analysis; both worked at the University of Washington (UW)]. At visible wavelengths, the absorption by ice is extremely weak. For example, the absorption length (e-folding depth, reciprocal of the absorption coefficient) for blue light is ∼200 m, and even for red light it is 2 m (Warren and Brandt, 2008). Photons entering a snowpack of 100-μm spheres should experience numerous refraction events and eventually escape upward, having traveled much less than 2 m through ice. Correspondingly, the albedo across the visible spectrum should be 95–100% for all grain radii 50–1500 μm. But that was not what GM had found. At the blue wavelength 400 nm, their albedo for dry new snow was 92%, and for old melting snow it was much lower, ∼82%. We considered what our model had neglected: the non-sphericity of snow grains, and the shadowing caused by close-packing in high-density snow. But we showed that those defects of the model would actually have caused more error at near-IR wavelengths than at visible wavelengths. So we concluded that the snow measured by GM had contained something in addition to ice, something that absorbs visible radiation. What could it be?
Atmospheric Aerosols Can Get Into Snow
In my first few months at NCAR I had become aware that there were some scientists who had actually made a career of studying dust in the atmosphere (e.g., Patterson and Gillette, 1977). Dale Gillette was a friendly fellow, and I enjoyed his company. But I was astonished at his choice of research topics; I could hardly imagine anything more boring than atmospheric dust. But of course dust can fall out into snow, and now it looked to be important for snow albedo, so to my surprise I myself became fascinated with dust.
I consulted the literature, back as far as the 1920s. Dust from Oklahoma had reached Vermont in the “brown snowfall” of 1936; pinkish-colored snow in New Zealand in 1928 was attributed to red dust from Australia. Haeberli (1977) had written a nice review of Saharan dust in the Alps. Dirmhirn (1960) had measured the effect of a dust-fall event on the albedo of alpine snow: a reduction of 10% in broadband albedo and 16% in visible albedo. The champion for brevity of title headed a Russian paper by Khromov (1931), “Zhyoltyy Sneg,” which simply means “yellow snow.”
I got optical constants of red Saharan dust from Dale Gillette (Patterson et al., 1977). When we incorporated them into our radiative transfer model, the snow, not surprisingly, turned red. But the snow measured by Tom Grenfell in the Arctic Ocean was not visibly colored; if it had been he probably would not have measured it. And his spectral plots were flat across the visible spectrum, so what we needed was a gray absorber. One day when Wiscombe and I were walking down a corridor at NCAR, we bumped into Bob Charlson from UW. Wiscombe asked him, “What’s that light-absorber you’re finding in the atmospheric aerosol?” Bob revealed his not-yet-published secret, whispering in our ears: “It’s carbon!”
Some of the papers describing dirty snow did have enough soot to produce visible gray bands (Elgmork et al., 1973), and others had actually experimented with the use of black carbon to promote snowmelt in Japan, China, and Colorado (e.g., Meiman, 1973). A long paper in the Journal of Applied Meteorology by Professor Bill Gray of Colorado State University (Gray et al., 1976) advocated the use of carbon dust for weather modification, including speeding up snowmelt. His Figure 5 is a drawing of an airplane spewing carbon dust from its engines: after a B-52 airplane reaches cruising altitude it no longer needs all eight engines for power, so four of them can be switched to soot-production. This was apparently what some scientists in the 1970s thought to be the role of black carbon in the climate system, but now Gray’s paper is rarely cited; it was missing from the authoritative 173-page treatise by Tami Bond et al. (2013).
That literature-search was fun, but to make progress on research what we really needed was to find optical constants and size distributions for atmospheric soot, and to incorporate them into our radiative transfer model of snow. When we did that, to match Grenfell’s measurements we had to say that his snow at T-3 contained 150–200 nanograms of carbon per gram of snow (ng/g) (Warren and Wiscombe, 1980; Warren, 1982). These amounts of soot, reducing the visible albedo by nearly 20% without coloring it, would not make the snow visibly darker because the eye is a logarithmic detector, adapting to changes in illumination over a factor of 500,000 (judging from our ability to read a printed page illuminated by either the overhead Sun or the overhead full moon).
In our model, the addition of soot reduced the albedo only at wavelengths where the albedo of pure snow is very high (UV and visible). It did not reduce the near-IR albedo, where ice itself is moderately absorptive, so that trace amounts of impurities cannot do much.
We were left with two important unanswered questions. (1) Can we trust the model? We had not tested our model against measurements for snow known to be clean. (2) How much soot does the Arctic snow actually contain? The source of soot over wide areas would be fallout from the atmospheric “Arctic haze” (Rahn and McCaffrey, 1980; Cess, 1983), but Grenfell’s measurements had been made close to the T-3 research station where the snow was probably affected by local pollution and did not represent the wider area.
What is the Soot Content of Arctic Snow?
Answering both questions benefited from my move to UW. In 1981 Ed LaChapelle retired, and I was lucky to be hired as his successor. As part of my job interview I gave a seminar, “Reflection of sunlight by snow.” In the audience was Tony Clarke, a graduate student in Charlson’s lab. Tony was inspired by my talk to try to quantify the soot in Arctic snow. Tony had invented the “integrating sandwich” filter method to greatly increase the sensitivity for quantifying the small amounts of soot in clean remote air (Clarke, 1982). His fellow student John Ogren had developed methods to collect soot from rainwater, and Tony adapted John’s method for snow. In April 1983 Tony and I drove up to Mount Rainier on a Sunday to practice snow-collection. Tony’s wife Joan came along, but she became bored watching us discussing our snow-pit. She pulled out a cigarette and was just about to light it when it dawned on her that maybe she shouldn’t.
Tony got on the phone to researchers who were going to the Arctic, and many of them volunteered to collect snow for him. Tony instructed them on snow-sampling procedures (Please don’t smoke!). They shipped their still-frozen snow to Seattle, from Alaska, Canada, Greenland, Svalbard, and Scandinavia. A Masters student, Kevin Noone, worked with Tony to process the samples. Their measurements of filter transmission at four wavelengths allowed them to separate the absorption by soil dust from the absorption by BC. They found BC values in the range 5–50 ng/g (Clarke and Noone, 1985). Using our radiative-transfer results, they estimated that this soot content would reduce snow albedo by 0–4%, depending on the snow grain size, with radiative forcing similar to that of Arctic haze in the atmosphere (Cess, 1983; Warren and Clarke, 1986). An albedo reduction of 1–2% sounds small, and is often within the measurement uncertainty, but it is climatically important. For a typical daily average solar irradiance of 400 W m-2 in the Arctic during late spring and early summer, a 1% reduction of broadband snow albedo causes a positive forcing of 4 W m-2 locally, similar to the forcing caused by doubling CO2.
The Arctic haze exhibits a dramatic seasonal cycle, with high values in winter and low values in summer (e.g., Sharma et al., 2004). A chance conversation of Tony with Björn Holmgren at the IUGG meeting in 1983 led to an invitation for Kevin to investigate the transfer of soot from air into snow at the Abisko research station in northern Sweden. For his Master’s thesis, Kevin documented the springtime decline of Arctic soot, measuring BC in both air and falling snow at Abisko (Noone and Clarke, 1988).
Where Can We Find Clean Snow?
Being at UW in the same department with Tom Grenfell gave me the opportunity to get involved in fieldwork. In 1982 Tom and I wrote a proposal to NSF to go to the interior plateau of Antarctica, to measure the spectral albedo of snow we could be sure was clean, to see if the visible albedo was as high as the model predicted. Our proposal was rejected, by reviewers saying “Albedo has already been measured in Antarctica.” We needed to emphasize that it was spectral albedo, not broadband albedo, that was required, and that Tom was the world expert for measuring spectral albedo of polar surfaces. Our resubmitted proposal was approved by the NSF Program Manager, Ben Fogle, and in December 1985 we arrived at the South Pole Station, together with a graduate student, Peter Mullen. We spent 2 months there.
The Antarctic snow is extremely clean, but we were based at a station that burned diesel fuel and experienced 100 landings and takeoffs of C-130 airplanes every summer. So while Tom and Peter made numerous albedo measurements, under various sky conditions for both new and old snow, I conducted a soot survey. Tony and Kevin had given me a filtering apparatus and had instructed me on their procedures. I walked out along 3-km lines in all directions from the station, collecting snow at regular intervals, until I was able to make a contour map of soot-pollution (Warren and Clarke, 1990). The wind at the South Pole is strongly directional, and just 500 m upwind of the station, in the Clean Air Sector, at a site we had already chosen for albedo measurements, the soot content was only 0.3 ng/g, far too small to measurably affect snow albedo at the most sensitive wavelength. The albedo exceeded 0.98 across the UV and visible, in agreement with our model for pure snow. We showed this result in a brief report (Warren et al., 1986); we eventually published the full paper after we had also made similar measurements at Vostok Station (Grenfell et al., 1994).
Tangential Topics From the Early Years
Soot Can Lower Snow Albedo; Why Not Cloud Albedo?
Black carbon can get into snow by being attached to ice nuclei in clouds or scavenged by falling snow crystals, as well as by dry deposition. BC in snow reduces the snow albedo; what does it do to clouds? Cindy Twohy, another of Charlson’s students, had developed the Counterflow Virtual Impactor (CVI) for measurements from an airplane flying through a cloud, to separately quantify LAI inside cloud droplets and LAI in the interstitial air between cloud droplets. She asked me to compute the effect of the LAI on the visible albedo of the clouds she had sampled over the Pacific Ocean between California and Hawaii. The effect was insignificant. Our calculation (Twohy et al., 1989) showed that to achieve a given reduction of visible albedo in a cloud or in snow, the required mixing ratio of soot in the cloud (ng/g) would have to be 300 times the mixing ratio in snow, because of two differences between clouds and snow that determine their interaction with sunlight: (1) cloud particles are smaller, with effective radii ∼10 μm as opposed to 100 μm or more for snow, so photons can escape upward from the cloud without passing through much water; and (2) typical cloud optical thicknesses at visible wavelengths are only 15–30, whereas snow is effectively semi-infinite (just 1 cm of snow has an optical thickness of ∼40), so much of the light incident on a cloud escapes out the bottom without encountering impurity particles.
But now I have to back off from our categorical conclusion about the ineffectiveness of soot in clouds. Over the ocean off the southwest coast of India, Andy Ackerman showed that the clouds can be so polluted by smoke that they do absorb significant sunlight, enough to cause the clouds to heat up and evaporate (Ackerman et al., 2000).
CO2-Snow in the Polar Caps of Mars, Where the Impurity Is Water
In February 1981 Wiscombe returned to NCAR from a conference about climate change on Mars, and asked me to apply our model to CO2-snow in the seasonal polar caps, using some newly measured optical constants of CO2-ice that had been reported at the meeting. We calculated the visible spectral albedo and how it is lowered by Martian dust, but our primary motivation was to explain some strange satellite observations of low brightness temperature in the thermal infrared. Because absorption by CO2-ice is extremely weak between the 4.3- and 15-μm bands, the thermal emissivity of CO2-snow is sensitive to trace amounts of water-ice, whose absorption coefficient in the interband region is 2–3 orders of magnitude higher than that of CO2-ice. So in the radiative transfer model, our specification of the “impurity” became, to our amusement, not dust or BC but rather water-ice (Warren et al., 1990).
The Modern ERA
After the pioneering work of Clarke and Noone was published in 1985, Tony and I expected that climate modelers would take notice. But for many years they ignored it, so Tony and I pursued work on other topics (Tony worked on atmospheric aerosol studies; I worked on cloud climatology, sea-ice albedo, longwave radiation spectra, and sea-glaciers on Snowball Earth).
Finally, nearly 20 years later, interest in LAI in snow was renewed by Jim Hansen, who thought that soot-in-snow might be the missing radiative forcing in his modeled global warming of the 20th century, so he incorporated that process into the GISS climate model (Hansen and Nazarenko, 2004; hereafter HN). He asked me and Tony to review his paper, hoping to persuade us to return to our former research topic.
The HN paper generated wide interest, including among funding agencies. Tom Grenfell, Tony Clarke, and I got a grant from the NSF Arctic Program, and we were joined in the work by Sarah Doherty and Rich Brandt. We carried out large-area surveys of LAI in snow in the Arctic (Doherty et al., 2010) and North America (Doherty et al., 2014). Professor Qiang Fu in my department at UW established a collaboration with Lanzhou University so that we could carry out a similar survey across northern China (Huang et al., 2011). We carried out these large-area surveys as field trips because it is difficult to detect small yet climatically important amounts of LAI in snow by remote sensing, especially in the Arctic where the snow is often thin and patchy (Warren, 2013). [Also, those trips gave me the opportunity to travel in some exotic remote regions!] On trips where we were driving, we could specify the sampling interval. We typically collected samples every 100 km, and this seemed adequate, as evidenced for example in the smooth plot of BC versus latitude in northeast China (Figure 6 of Huang et al., 2011).
Tom built our laboratory photometer for analyzing filters (Grenfell et al., 2011). Sarah developed a spectroscopic method to separate the absorption by BC from that of other LAI, showing that typically ∼40% of the absorption by impurities was by non-BC LAI. [In some regions, BC is actually a minor contributor to the LAI; Tom Painter leads a group studying snow in mid-latitude mountain regions near deserts, where soil dust dominates the absorption (e.g., Painter et al., 2007)].
Hansen and Nazarenko’s paper also generated interest in the remote-sensing community. For several years after 2004 I was sometimes asked to review proposals that promised to quantify BC in Arctic snow by remote sensing from satellites or unmanned aerial vehicles (UAVs). One of those proposals requested several million dollars from NASA to accomplish a goal I considered impossible. To forestall such proposals, I wrote a paper to explain why I thought that such projects, if undertaken, were destined to fail (Warren, 2013). A particular problem is that much of the Arctic snowpack in spring is thin and patchy, and the spectral signature of thin snow resembles that of BC in snow. [However, in some other parts of the world the snow is so heavily loaded with LAI that remote sensing can be useful. Painter et al. (2012) used MODIS to infer radiative forcing by dust contamination of snow in the Himalaya and in southwest Colorado, checked with ground-truth measurements in Colorado. The snow was grossly contaminated, with concentrations in the parts-per-thousand range rather than parts per billion. The MODIS retrieval found an astonishingly large mean dust-in-snow forcing of ∼250 W m-2 in these regions].
Figure 1 shows some pictures from our fieldwork. Table 1 gives a global summary of our BC mixing ratios. They range over four orders of magnitude, from 0.2 ng/g in Antarctica to 2000 ng/g in northeast China. The values in this table all come from the same method of measurement that was developed by Clarke and Noone (1985). An alternative method using controlled combustion and measurement of CO2 release (“thermal-optical method”) tends to give smaller values (e.g., Forsström et al., 2013), possibly because of undercatch by the quartz fiber filter (Torres et al., 2014).
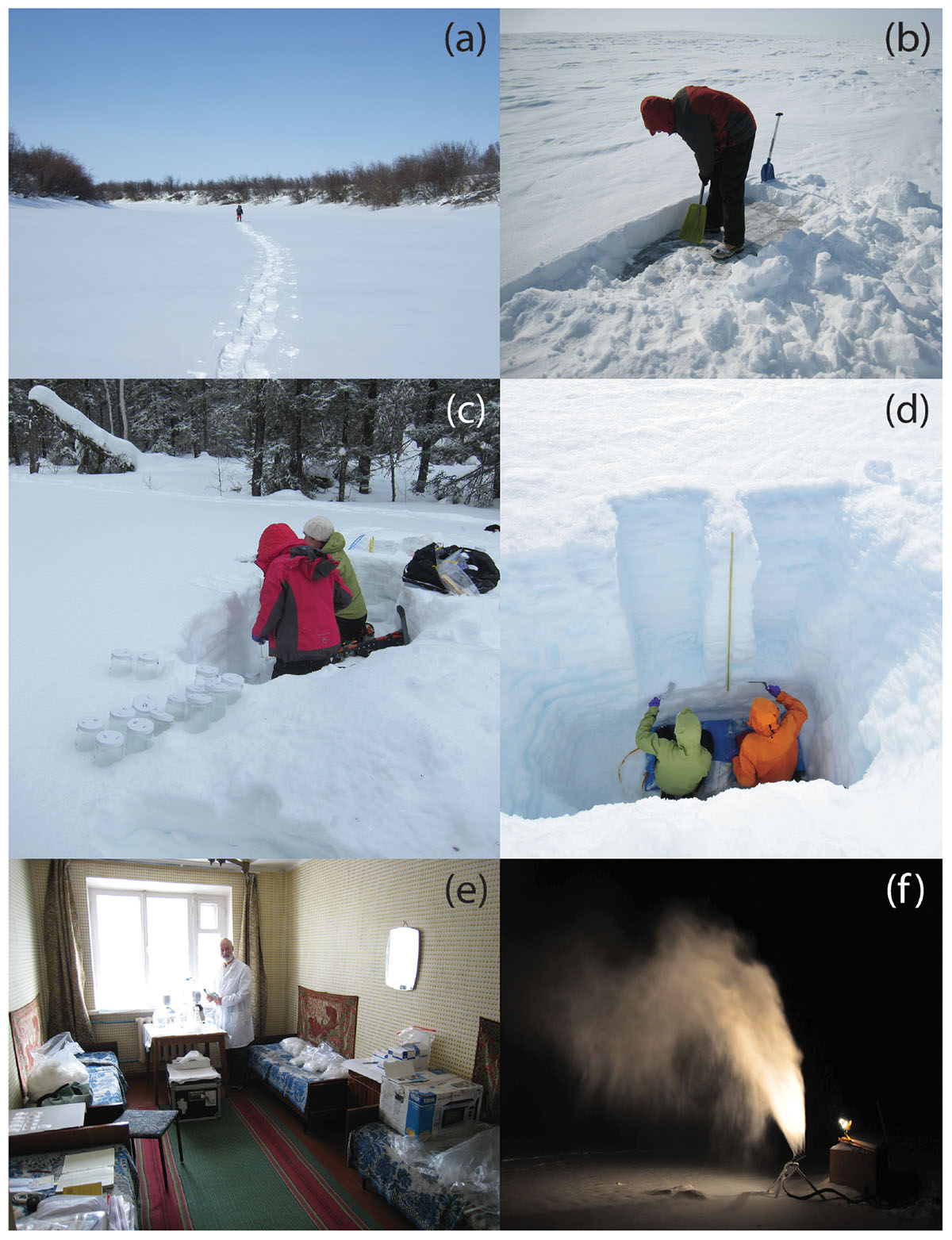
Figure 1. Photos from fieldwork. (a) In the Kolyma lowland, Yakutia. (b) Rich Brandt on a frozen lake in Arctic Canada. (c) Cheng Dang and Sarah Doherty in Manitoba. (d) With Sarah Doherty, finding buried summer melt-layers near Dye-2 in the percolation zone of South Greenland (photo by Rich Brandt). (e) Tom Grenfell filtering snowmelt in a hotel room in Tiksi near the Lena Delta. [Written informed consent has been obtained from him to publish this picture]. (f) Making artificial snowpacks in the Adirondack Mountains (Rich Brandt).
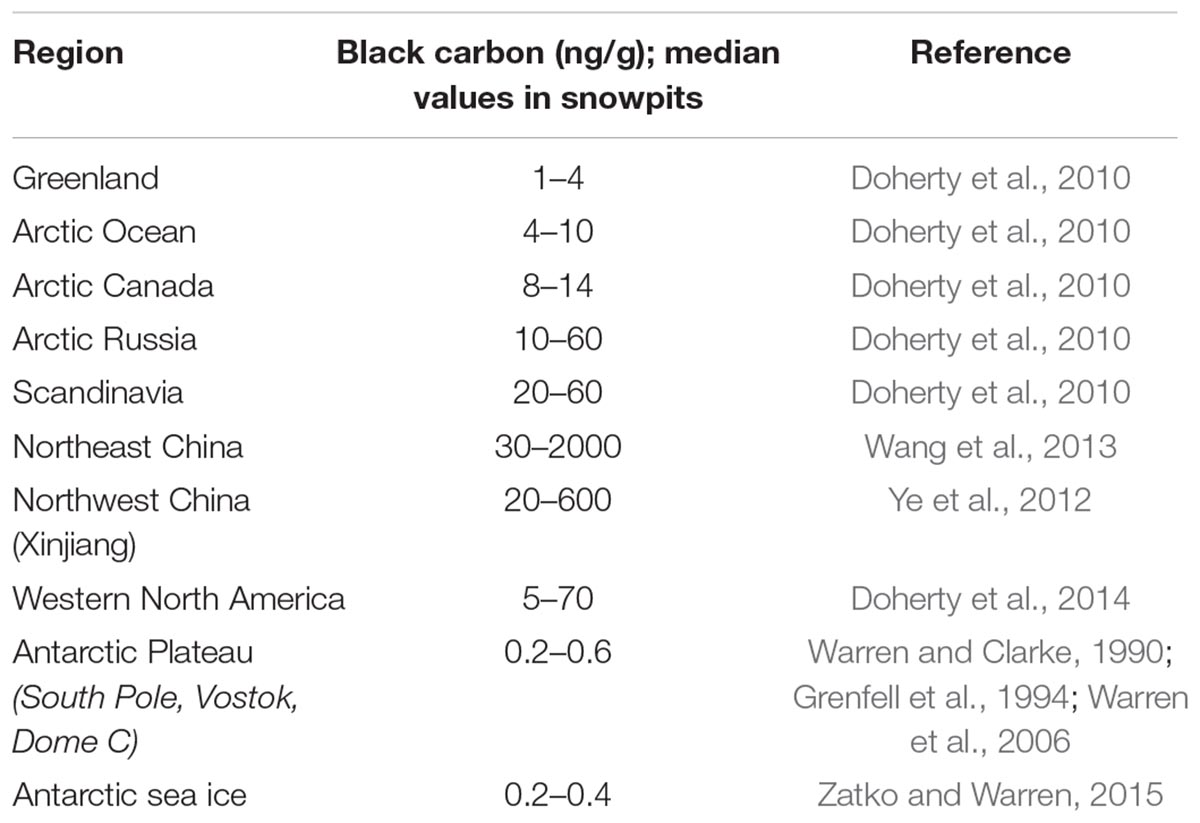
Table 1. Global summary of black-carbon mixing-ratios in snow before the onset of melt (winter in midlatitudes; spring in the Arctic and Antarctic).
Our Arctic survey found lower BC mixing-ratios than those of Clarke and Noone (1985), in regions that both studies had sampled. This result was anticipated by some measurements that Tom Grenfell had made in 1998 at the SHEBA site in the Beaufort Sea (Grenfell et al., 2002) and during an Arctic Ocean transect in 2005. Our lower BC mixing ratios were consistent with results from the continuous monitoring of BC in air since 1989 at the Alert station on Ellesmere Island (82°N) by Sangeeta Sharma and her coworkers (Gong et al., 2010). They showed that the Arctic atmosphere has become cleaner in the past 30 years, which they attributed to the demise of the Soviet Union. We concluded that BC in snow and ice of the Arctic Ocean is probably not contributing to the rapid decline of sea ice in recent decades, so other causes must be sought.
We studied the vertical redistribution of BC in melting snow in Greenland and Alaska, and also in Norway in a collaboration with Sanja Forsström (Doherty et al., 2013). This work was a follow-on to the study by Conway et al. (1996), who had spread artificial soots, both hydrophobic and hydrophilic, onto melting snow, and quantified the vertical redistribution. Because BC is largely hydrophobic, much of it gets left behind at the surface as the snow melts, enhancing the albedo reduction.
Rich Brandt tested our radiative-transfer modeling of BC-in-snow by making artificial snowpacks containing large amounts of soot (Brandt et al., 2011). Dean Hegg, a chemist just down the hall from me in the UW cloud-physics group, got interested in our project; he led a study on source-attribution of BC by looking for fingerprints in the pattern of 24 chemicals that he measured in our meltwater (Hegg et al., 2009, 2010). He surprised us by showing the dominance of biomass burning over industrial sources in most parts of the Arctic. A graduate student, Cheng Dang, worked with us to quantify the radiative effects of the LAI found in our large-area surveys (Dang et al., 2015, 2017).
Broader Impacts
Ice-core records show enhanced dust fallout during glacial maxima, as first shown by Lonnie Thompson (1977). The albedo-lowering effect of dust was suggested by Will Kellogg to cause a negative feedback on ice-sheet growth (Figure 8 of Kellogg, 1975), as discussed by Warren (1984a). Indeed, climate modeling of ice-age cycles now finds this albedo-lowering effect of dust necessary for deglaciation (Willeit and Ganopolski, 2018). And on a shorter time-scale, within the present interglacial, the “Little Ice Age” in the Alps is hypothesized to have been terminated by deposition of BC from the 19th-century industrialization of Europe (Painter et al., 2013).
The Future
I have been amazed to see the expansion of research by many groups worldwide, in Europe, North America, South America, Japan, China, and India, on all aspects of this problem, with sessions every year at EGU and AGU, and the publications in this special issue. Here are just a few suggested topics for future research that link to our recent measurements.
(1) Determine wet-deposition rates of particles, following on to the work of Noone and Clarke (1988). Riding the cable-car from Ny-Ålesund up to the Zeppelin Station on Svalbard stimulated our thinking on one way to do this, resulting in the paper by Hegg et al. (2011).
(2) Investigate lofting and deposition of dust on small scales that are currently missing from climate models, for example the transport of dust to shallow patchy snow from nearby soil (centimeter-scale), and to glaciers from mountain-walls (kilometer-scale). This problem became apparent to us in Inner Mongolia, where sheep grazing through thin snow would kick up soil that then fell on top of the neighboring snow.
(3) Further quantify the biases in our filter method (ISSW) and in the single-particle soot photometer (SP2) for snow samples containing BC mixed with organic carbon and/or mineral dust (Schwarz et al., 2012), and the capture-efficiency of the filter for the thermal-optical method (Torres et al., 2014).
Author Contributions
The author confirms being the sole contributor of this work and has approved it for publication.
Conflict of Interest Statement
The author declares that the research was conducted in the absence of any commercial or financial relationships that could be construed as a potential conflict of interest.
Acknowledgments
This work was made possible by collaborations with many colleagues: Warren Wiscombe, Tony Clarke, Tom Grenfell, Rich Brandt, Sarah Doherty, Dean Hegg, Qiang Fu, Cheng Dang, Rudong Zhang, Peter Mullen, Steve Hudson, and Sanja Forsström. Others who collected snow or participated in other ways include (from the United States) Matthew Sturm, Mike Steele, Angel Adames, Jamie Morison, Ron Sletten, Mike Town, Kat Huybers, Ryan Eastman, Maria Zatko, Ignatius Rigor, and Lora Koenig; (from China) Jianping Huang and Hao Ye; (from Russia) Vladimir Radionov, Sergei Zimov, Viktor Boyarsky, and Stas Kogan; and (from Europe) Carl Bøggild, Sebastian Gerland, Christina Pedersen, Johan Ström, Jane Carlsen, Koni Steffen, Kevin Noone, and Sebastian Simonsen. Thanks to OM, PD-W, Marie Dumont, and Biagio Di Mauro for inviting me to give this talk at their session of the EGU meeting (April 2018), and to write this paper. Helpful comments on the manuscript were provided by Sarah Doherty, Tony Clarke, Tom Grenfell, OM, Tom Painter, JD, and Tami Bond. Richard Brandt assisted in the preparation of Figure 1.
References
Ackerman, A. S., Toon, O. B., Stevens, D. E., Heymsfield, A. J., Ramanathan, V., and Welton, E. J. (2000). Reduction of tropical cloudiness by soot. Science 288, 1042–1047. doi: 10.1126/science.288.5468.1042
Bond, T. C., Doherty, S. J., Fahey, D. W., Forster, P. M., Berntsen, T., DeAngelo, B. J., et al. (2013). Bounding the role of black carbon in the climate system: a scientific assessment. J. Geophys. Res. 118, 1–173. doi: 10.1002/jgrd.50171
Brandt, R. E., Warren, S. G., and Clarke, A. D. (2011). A controlled snowmaking experiment testing the relation between black carbon content and reduction of snow albedo. J. Geophys. Res. 116:D08109. doi: 10.1029/2010JD015330
Cess, R. D. (1983). Arctic aerosols: model estimates of interactive influences upon the surface-atmosphere clear-sky radiation budget. Atmos. Environ. 17, 2555–2564. doi: 10.1016/0004-6981(83)90083-5
Clarke, A. D. (1982). Integrating sandwich: a new method of measurement of the light absorption coefficient for atmospheric particles. Appl. Opt. 21, 3011–3020. doi: 10.1364/AO.21.003011
Clarke, A. D., and Noone, K. J. (1985). Soot in the arctic snowpack: a cause for perturbations in radiative transfer. Atmos. Environ. 19, 2045–2053. doi: 10.1016/0004-6981(85)90113-1
Colbeck, S. C. (1982). An overview of seasonal snow metamorphism. Rev. Geophys. Space Phys. 20, 45–61. doi: 10.1029/RG020i001p00045
Conway, H., Gades, A., and Raymond, C. F. (1996). Albedo of dirty snow during conditions of melt. Water Resour. Res. 32, 1713–1718. doi: 10.1029/96WR00712
Dang, C., Brandt, R. E., and Warren, S. G. (2015). Parameterizations for narrowband and broadband albedo of pure snow, and snow containing mineral dust and black carbon. J. Geophys. Res. 120, 5446–5468. doi: 10.1002/2014JD022646
Dang, C., Fu, Q., and Warren, S. G. (2016). Effect of snow grain shape on snow albedo. J. Atmos. Sci. 73, 3573–3583. doi: 10.1175/JAS-D-15-0276.1
Dang, C., Warren, S. G., Fu, Q., Doherty, S. J., and Sturm, M. (2017). Measurements of light-absorbing particles in snow across the Arctic, North America, and China: effects on surface albedo. J. Geophys. Res. 122, 10149–10168. doi: 10.1002/2017JD027070
Dirmhirn, I. (1960). Starke absorptionsschichten auf den schneeoberflächen der alpengletscher. Wetter Leben 12, 152–153.
Doherty, S. J., Dang, C., Hegg, D. A., Zhang, R., and Warren, S. G. (2014). Black carbon and other light-absorbing particles in snow of central North America. J. Geophys. Res. 119, 12807–12831. doi: 10.1002/2014JD022350
Doherty, S. J., Grenfell, T. C., Forsström, S., Hegg, D. L., Brandt, R. E., and Warren, S. G. (2013). Observed vertical redistribution of black carbon and other insoluble light-absorbing particles in melting snow. J. Geophys. Res. 118, 5553–5569. doi: 10.1002/jgrd.50235
Doherty, S. J., Warren, S. G., Grenfell, T. C., Clarke, A. D., and Brandt, R. E. (2010). Light-absorbing impurities in Arctic snow. Atmos. Chem. Phys. 10, 11647–11680. doi: 10.5194/acp-10-11647-2010
Elgmork, K., Hagen, A., and Langeland, A. (1973). Polluted snow in southern Norway during the winters 1968-1971. Environ. Pollut. 4, 41–52. doi: 10.1016/0013-9327(73)90030-X
Forsström, S., Isaksson, E., Skeie, R. B., Ström, J., Pedersen, C. A., Hudson, S. R., et al. (2013). Elemental carbon measurements in European Arctic snow packs. J. Geophys. Res. Atmos. 118, 13614–13627. doi: 10.1002/2013JD019886
Gong, S. L., Zhao, T. L., Sharma, S., Toom-Sauntry, D., Lavoue, D., Zhang, X. B., et al. (2010). Identification of trends and inter-annual variability of sulphate and black carbon in the Canadian High Arctic: 1981 to 2007. J. Geophys. Res. 115:D07305. doi: 10.1029/2009JD012943
Gray, W. M., Frank, W. M., Corrin, M. L., and Stokes, C. A. (1976). Weather modification by carbon dust absorption of solar energy. J. Appl. Meteor. 15, 355–386. doi: 10.1175/1520-0450(1976)015<0355:WMBCDA>2.0.CO;2
Grenfell, T. C., Doherty, S. J., Clarke, A. D., and Warren, S. G. (2011). Light absorption from particulate impurities in snow and ice determined by spectrophotometric analysis of filters. Appl. Opt. 50, 2037–2048. doi: 10.1364/AO.50.002037
Grenfell, T. C., Light, B., and Sturm M. (2002). Spatial distribution and radiative effects of soot in the snow and sea ice during the SHEBA experiment. J. Geophys. Res. SHE 7-1–SHE 7-7. doi: 10.1029/2000JC000414
Grenfell, T. C., and Maykut, G. A. (1977). The optical properties of ice and snow in the Arctic basin. J. Glaciol. 18, 445–463. doi: 10.1017/S0022143000021122
Grenfell, T. C., Neshyba, S. P., and Warren, S. G. (2005). Representation of a nonspherical ice particle by a collection of independent spheres for scattering and absorption of radiation: 3, hollow columns and plates. J. Geophys. Res. (Atmospheres) 110:D17203. doi: 10.1029/2005JD005811
Grenfell, T. C., and Warren, S. G. (1999). Representation of a nonspherical ice particle by a collection of independent spheres for scattering and absorption of radiation. J. Geophys. Res. 104, 31697–31709. doi: 10.1029/1999JD900496
Grenfell, T. C., Warren, S. G., and Mullen, P. C. (1994). Reflection of solar radiation by the Antarctic snow surface at ultraviolet, visible, and near-infrared wavelengths. J. Geophys. Res. 99, 18669–18684. doi: 10.1029/94JD01484
Haeberli, W. (1977). Sahara dust in the Alps – A short review. Z. Gletscher. Glazialgeol. 13, 206–208.
Hansen, J., and Nazarenko, L. (2004). Soot climate forcing via snow and ice albedos. Proc. Natl. Acad. Sci. U.S.A. 101, 423–428. doi: 10.1073/pnas.2237157100
Hegg, D. A., Clarke, A. D., Doherty, S. J., and Ström, J. (2011). Measurements of black carbon aerosol washout ratio on Svalbard. Tellus B 63, 891–900. doi: 10.1111/j.1600-0889.2011.00577.x
Hegg, D. A., Warren, S. G., Grenfell, T. C., Doherty, S. J., and Clarke, A. D. (2010). Sources of light-absorbing aerosol in Arctic snow and their seasonal variation. Atmos. Chem. Phys. 10, 10923–10938. doi: 10.5194/acp-10-10923-2010
Hegg, D. A., Warren, S. G., Grenfell, T. C., Doherty, S. J., Larson, T. V., and Clarke, A. D. (2009). Source attribution of black carbon in arctic snow. Environ. Sci. Technol. 43, 4016–4021. doi: 10.1021/es803623f
Huang, J., Fu, Q., Zhang, W., Wang, X., Zhang, R., Ye, H., et al. (2011). Dust and black carbon in seasonal snow across northern China. Bull. Am. Meteor. Soc. 92, 175–181. doi: 10.1175/2010BAMS3064.1
Kellogg, W. W. (1975). “Climatic feedback mechanisms involving the polar regions,” in Climate of the Arctic, eds G. Weller and S. A. Bowling (Fairbanks, AK: University of Alaska, Geophysical Institute), 111–116.
LaChapelle, E. R. (1969). Field Guide to Snow Crystals. Seattle, WA: University of Washington Press, 101.
Meiman, J. (1973). “Snow surface modification,” in The Role of Snow and Ice in Hydrology, ed. I. C. Brown (London: International Association of Hydrological Sciences Publ. No. 107), 1376–1381.
Neshyba, S. P., Grenfell, T. C., and Warren, S. G. (2003). Representation of a nonspherical ice particle by a collection of independent spheres for scattering and absorption of radiation: II. Hexagonal columns and plates. J. Geophys. Res. 108:4448. doi: 10.1029/2002JD003302
Noone, K. J., and Clarke, A. D. (1988). Soot scavenging measurements in Arctic snowfall. Atmos. Environ. 22, 2773–2778. doi: 10.1016/0004-6981(88)90444-1
O’Brien, H. W., and Munis, R. H. (1975). Red and near-infrared spectral reflectance of snow. CRREL Res. Rep. 332:18.
Painter, T. H., Barrett, A. P., Landry, C. C., Neff, J. C., Cassidy, M. P., Lawrence, C. R., et al. (2007). Impact of disturbed desert soils on duration of mountain snow cover. Geophys. Res. Lett. 34:L12502. doi: 10.1029/2007GL030284
Painter, T. H., Bryant, A. C., and Skiles, S. M. (2012). Radiative forcing by light absorbing impurities in snow from MODIS surface reflectance data. Geophys. Res. Lett. 39:L17502. doi: 10.1029/2012GL052457
Painter, T. H., Flanner, M., Marzeion, B., Kaser, G., VanCuren, R., and Abdalati, W. (2013). End of the little ice age in the alps forced by black carbon. Proc. Nat. Acad. Sci. U.S.A. 110, 15216–15221. doi: 10.1073/pnas.1302570110
Patterson, E. M., and Gillette, D. A. (1977). Commonalities in measured size distributions for aerosols having a soil-derived component. J. Geophys. Res. 82, 2074–2082. doi: 10.1029/JC082i015p02074
Patterson, E. M., Gillette, D. A., and Stockton, B. H. (1977). Complex index of refraction between 300 and 700 nm for Saharan aerosols. J. Geophys. Res. 82, 3153–3160. doi: 10.1029/JC082i021p03153
Rahn, K. A., and McCaffrey, R. J. (1980). On the origin and transport of the winter Arctic aerosol. Ann. N. Y. Acad. Sci. 338, 486–503. doi: 10.1111/j.1749-6632.1980.tb17142.x
Schwarz, J. P., Doherty, S. J., Li, F., Ruggiero, S. T., Tanner, C. E., Perring, A. E., et al. (2012). Assessing single particle soot photometer and integrating sphere/integrating sandwich spectrophotometer measurement techniques for quantifying black carbon concentration in snow. Atmos. Meas. Tech. 5, 2581–2592. doi: 10.5194/amt-5-2581-2012
Sharma, S., Lavoué, D., Cachier, H., Barrie, L. A., and Gong, S. L. (2004). Long-term trends of the black carbon concentrations in the Canadian Arctic. J. Geophys. Res. 109:D15203. doi: 10.1029/2003JD004331
Stubbs, G., Warren, S., and Holmes, K. (1977). Structure of RNA and RNA binding site in tobacco mosaic virus from a 4-A map calculated from X-ray fibre diagrams. Nature 267, 216–221. doi: 10.1038/267216a0
Thomas, G. E., and Stamnes K. (1999). Radiative Transfer in the Atmosphere and Oceans. Cambridge: Cambridge Univ. Press, 517. doi: 10.1017/CBO9780511613470
Thompson, L. G. (1977). “Variations in microparticle concentration, size distribution and elemental composition found in camp century, greenland, and byrd station, antarctica, deep ice cores,” in Isotopes and Impurities in Snow and Ice, Vol. 118, ed. H. Oeschger (London: International Association of Hydrological Sciences), 351–364.
Torres, A., Bond, T. C., Lehmann, C. M. B., Subramanian, R., and Hadley, O. L. (2014). Measuring organic carbon and black carbon in rainwater: evaluation of methods. Aerosol. Sci. Tech. 48, 239–250. doi: 10.1080/02786826.2013.868596
Twohy, C. H., Clarke, A. D., Warren, S. G., Radke, L. F., and Charlson, R. J. (1989). Light-absorbing material extracted from cloud droplets and its effect on cloud albedo. J. Geophys. Res. 94, 8623–8631. doi: 10.1029/JD094iD06p08623
Wang, X., Doherty, S. J., and Huang, J. (2013). Black carbon and other light-absorbing impurities in snow across Northern China. J. Geophys. Res. Atmos. 118, 1471–1492. doi: 10.1029/2012JD018291
Warren, S. G. (1982). Optical properties of snow. Rev. Geophys. Space Phys. 20, 67–89. doi: 10.1029/RG020i001p00067
Warren, S. G. (1984a). Impurities in snow: effects on albedo and snowmelt. Ann. Glaciol. 5, 177–179. doi: 10.1017/S0260305500003700
Warren, S. G. (1984b). Optical constants of ice from the ultraviolet to the microwave. Appl. Opt. 23, 1206–1225. doi: 10.1364/AO.23.001206
Warren, S. G. (2013). Can black carbon in snow be detected by remote sensing? J. Geophys. Res. 118, 779–786. doi: 10.1029/2012JD018476
Warren, S. G., and Brandt, R. E. (2008). Optical constants of ice from the ultraviolet to the microwave: a revised compilation. J. Geophys. Res. 113:D14220. doi: 10.1029/2007JD009744
Warren, S. G., Brandt, R. E., and Grenfell, T. C. (2006). Visible and near-ultraviolet absorption spectrum of ice from transmission of solar radiation into snow. Appl. Opt. 45, 5320–5334. doi: 10.1364/AO.45.005320
Warren, S. G., and Clarke, A. D. (1986). “Soot from Arctic haze: radiative effects on the Arctic snowpack,” in Proceedings of the Snow Watch Workshop 1985: Glaciological Data, Vol. 18 (Boulder, CO: NSIDC, University of Colorado Boulder), 73–77.
Warren, S. G., and Clarke, A. D. (1990). Soot in the atmosphere and snow surface of Antarctica. J. Geophys. Res. 95, 1811–1816. doi: 10.1029/JD095iD02p01811
Warren, S. G., Edwards, B. F. P., Evans, D. R., Wiley, D. C., and Lipscomb, W. N. (1973). Aspartate transcarbamylase from Escherichia coli: electron density at 5.5 A resolution. Proc. Nat. Acad. Sci. U.S.A. 70, 1117–1121. doi: 10.1073/pnas.70.4.1117
Warren, S. G., Grenfell, T. C., and Mullen, P. C. (1986). Optical properties of antarctic snow. Antarct. J. U.S. 21, 247–248.
Warren, S. G., and Schneider, S. H. (1979). Seasonal simulation as a test for uncertainties in the parameterizations of a Budyko-Sellers zonal climate model. J. Atmos. Sci. 36, 1377–1391. doi: 10.1175/1520-0469(1979)036<1377:SSAATF>2.0.CO;2
Warren, S. G., and Wiscombe, W. J. (1980). A model for the spectral albedo of snow. II: Snow containing atmospheric aerosols. J. Atmos. Sci. 37, 2734–2745. doi: 10.1175/1520-0469(1980)037<2734:AMFTSA>2.0.CO;2
Warren, S. G., Wiscombe, W. J., and Firestone, J. F. (1990). Spectral albedo and emissivity of CO2-snow in martian polar caps: model results. J. Geophys. Res. 95, 14717–14741. doi: 10.1029/JB095iB09p14717
Willeit, M., and Ganopolski, A. (2018). The importance of snow albedo for ice sheet evolution over the last glacial cycle. Clim. Past 14, 697–707. doi: 10.5194/cp-14-697-2018
Wiscombe, W. J. (1980). Improved mie scattering algorithms. Appl. Opt. 19, 1505–1509. doi: 10.1364/AO.19.001505
Wiscombe, W. J., and Warren, S. G. (1980). A model for the spectral albedo of snow, I: pure snow. J. Atmos. Sci. 37, 2712–2733. doi: 10.1175/1520-0469(1980)037<2712:AMFTSA>2.0.CO;2
Wittmann, M., Zwaaftink, C. D. G., Schmidt, L. S., Guðmundsson, S., Pálsson, F., Arnalds, O. et al. (2017). Impact of dust deposition on the albedo of Vatnajökull ice cap, Iceland. Cryosphere 11, 741–754. doi: 10.5194/tc-11-741-2017
Ye, H., Zhang, R., Shi, J., Huang, J., Warren, S. G., and Fu, Q. (2012). Black carbon in seasonal snow across northern Xinjiang in northwestern China. Environ. Res. Lett. 7, 1–9. doi: 10.1088/1748-9326/7/4/044002
Keywords: snow, light-absorbing impurities in snow, snow albedo, Arctic, radiative transfer
Citation: Warren SG (2019) Light-Absorbing Impurities in Snow: A Personal and Historical Account. Front. Earth Sci. 6:250. doi: 10.3389/feart.2018.00250
Received: 24 July 2018; Accepted: 20 December 2018;
Published: 11 January 2019.
Edited by:
Pavla Dagsson-Waldhauserova, Agricultural University of Iceland, IcelandReviewed by:
Outi Meinander, Finnish Meteorological Institute, FinlandJeff Dozier, University of California, Santa Barbara, United States
Tami Bond, University of Illinois at Urbana–Champaign, United States
Copyright © 2019 Warren. This is an open-access article distributed under the terms of the Creative Commons Attribution License (CC BY). The use, distribution or reproduction in other forums is permitted, provided the original author(s) and the copyright owner(s) are credited and that the original publication in this journal is cited, in accordance with accepted academic practice. No use, distribution or reproduction is permitted which does not comply with these terms.
*Correspondence: Stephen G. Warren, c2d3QHV3LmVkdQ==