- 1Department of Geography, College of Science, Swansea University, Swansea, United Kingdom
- 2Department of Biosciences, College of Science, Swansea University, Swansea, United Kingdom
- 3Department of Soil Science and Agricultural Chemistry, University of Santiago de Compostela, Lugo, Spain
- 4Wildfire Lab, Hatherly Laboratories, University of Exeter, Exeter, United Kingdom
- 5Wildfire Operations Research, FPInnovations, Hinton, AB, Canada
Pyrogenic carbon (PyC, charcoal) is produced during vegetation fires at a rate of ~116–385 Tg C yr−1 globally. It represents one of the most degradation-resistant organic carbon pools, but its long-term fate and the processes leading to its degradation remain subject of debate. A frequently highlighted potential loss mechanism of PyC is its consumption in subsequent fires. However, only three studies to date have tested this hypothesis with reported losses of <8–37%, with the effects of PyC chemical characteristics and fire conditions on PyC loss in wildfires remaining unexplored. To address this, we placed materials with different degrees of thermal and chemical recalcitrance (A: wildfire charcoal, B: slash-pile charcoal, C: pine wood and D: cedar wood) on the ground surface just prior to a high-intensity and a low-intensity boreal forest wildfire. Mass losses were highly variable and dependent on fire- and sample characteristics. Mass losses across both fires (as % of dry weight) were for A: 66.5 ± 25.2, B: 41.7 ± 27.2, C: 78.2 ± 14.9, and D: 83.8 ± 18.9. Mass loss correlated significantly with maximum temperature (Tmax) recorded on sample surfaces (r = 0.65, p = 0.01), but only weakly (r = 0.33) with time >300°C. Mass losses also showed a significant negative correlation (r = −0.38, p = 0.05) with thermal recalcitrance (T50) determined using Differential Scanning Calorimetry (DSC) and Tmax with charcoal reflectance (Ro) determined after the fires (r = 0.46, p = 0.05). Losses in the high-intensity fire were significantly higher (p = 0.05) than in the low-intensity fire, but the latter had a higher rate of conversion of fuel to PyC. Our results demonstrate that exposure to fire can indeed be a significant removal mechanism for PyC that remains exposed on the ground after a previous fire. The losses found, however, are likely to represent an extreme upper range as most PyC produced in a fire would not remain exposed on the ground surface by the time the next fire occurs. Our data also demonstrate, for real wildfire conditions, the (i) contrasting resistance of different PyC types to combustion and (ii) contrasting net PyC losses between different fire intensities. The DSC and reflectance (Ro) results support the usefulness of these analyses in reflecting thermal degradation resistance and temperature exposure under actual wildfire conditions.
Introduction
Pyrogenic carbon (PyC, also known as charcoal, pyrogenic organic matter or black carbon) is a ubiquitous organic residue resulting from the incomplete combustion of organic fuels during vegetation fires (both natural and anthropogenic fires). It comprises a continuum of pyrogenic organic compounds ranging from partly charred material to soot (Santín et al., 2016a). Its chemical properties result in some of the PyC materials being highly resistant to biological breakdown, making PyC one of Earth's most degradation-resistant organic carbon pools. Estimates of its mean residence time range from decades or centuries (e.g., Steinbeiss et al., 2009; Bird et al., 2015) to millennia (e.g., Thevenon et al., 2010; de Lafontaine et al., 2011), and, importantly, consistently one or two orders of magnitude longer than those of their unburnt precursors (Bruun and EL-Zehery, 2012; Santos et al., 2012; Naisse et al., 2014). The most recent estimates of PyC global production range from between 63 and 140 Tg C yr−1 (Bird et al., 2015) and 116–385 Tg C yr−1 (Santín et al., 2016a), which is equivalent to ~0.1–0.6% of the annual terrestrial net primary productivity (Huston and Wolverton, 2009).
Production of PyC and its subsequent fate, therefore, have important implications for the global carbon cycle (Landry and Matthews, 2017). However, its long-term fate in the environment and the specific biotic and abiotic processes leading to its degradation remain the subject of much debate (Bird et al., 2015; Santín et al., 2016a). A potentially major mechanism highlighted in the literature for the abiotic terrestrial breakdown of PyC has been its in situ combustion in subsequent fires (e.g., Ohlson and Tryterud, 2000; Czimczik et al., 2005; Preston and Schmidt, 2006; Czimczik and Masiello, 2007; Kane et al., 2010; Foereid et al., 2011). PyC produced in a fire and not transported off site by wind or water, or protected through its transfer to sufficient depths below litter or soil surface layers, can act as fuel and be subject to combustion in subsequent fires. This abiotic loss mechanism for PyC could be particularly important in environments with short fire-return intervals, low bioturbation and topography, which limit, respectively, its vertical movement and off-site transport.
The efficacy of this proposed loss mechanism, however, has remained largely untested and was based, until recently, on little more than speculation. Indeed, to our knowledge, this “fire loss” hypothesis has, to date, been tested empirically in only the following three studies. In a high-intensity experimental boreal forest fire, Santín et al. (2013) found median losses of charcoal pieces (2–4 cm size) placed in the forest floor layer (~2 cm depth) to be <15%. Saiz et al. (2014) reported mean losses of <8% for charcoal pieces (0.5–1.5 cm size) placed on the soil surface in a prescribed fire in an open savannah woodland. Using coniferous fuels typical of the western US, Tinkham et al. (2016) found that repeated burning of litter beds in the laboratory reduced the PyC mass (>6 mm size) formed in the first fire, by 37% in the subsequent fire.
Given that vegetation fires as well as PyC materials vary widely in their characteristics, the current study aimed to explore the role of fire and PyC characteristics as potentially important variables in determining PyC removal rates during fires. We hypothesized that PyC with different degrees of carbonization exposed to fires of contrasting intensities would result in different PyC losses during burning. Thus, two different types of PyC (wildfire and slash-pile charcoal from jack pine wood) and, for comparison, uncharred wood (jack pine and western red cedar), were exposed to two contrasting types of wildfires: a high-intensity crown fire and a low-intensity surface fire. Temperature exposure of the samples during the fires was determined using thermocouples attached to sample surfaces, and the chemical and physical characteristics of the exposed and, where relevant, reference (unburnt) samples determined using thermogravimetric-differential scanning calorimetry, elemental- and charcoal surface reflectance analyses.
Materials and Methods
Study Area and Experimental Setup
Field experiments were carried out in the Great Slave Lake region of the Canadian Northwest Territories in summer 2015. This boreal region has a dry, sub-humid continental climate with an annual precipitation of ~300 mm, a wildfire season usually between May and September, and a fire recurrence interval in the region of 150 to 200 years. The forest is dominated by black spruce (Picea mariana) and jack pine (Pinus banksiana) with often thick organic soil layers. The topography is largely flat with an elevation between 100 and 200 m.a.s.l. (Alexander et al., 2004; Stocks et al., 2004).
Two types of PyC and two types of uncharred wood materials were used in the fire exposure experiments (Figures 1A,B):
(A) Wildfire charcoal generated during an experimental wildfire (June, 2012) in a mature jack pine stand in this region and collected in June 2013 (61°34'55” N; 117°11'55” W, see Santín et al. (2015), for site and fire characteristics).
(B) Charcoal from a jack pine slash-pile (a pile of slash generated in clearing/harvesting) burn in the winter of 2011/12 and collected in June 2012 at a site adjacent to where wildfire charcoal was sampled. Due to its production in a long-burning slash pile fire, this type of charcoal was speculated in previous work (Santín et al., 2013) to exhibit a higher degree of charring and therefore greater resistance to combustion when exposed to a subsequent fire than wildfire charcoal.
(C) Unburned jack pine down wood (small dead branches) collected in 2012 from an unburned area in the same study region.
(D) Unburned blocks cut from western red cedar (Thuja plicata) wood were included for comparison as a low-density wood used in a complementary study focusing specifically on charcoal reflectance (Belcher et al., 2018).
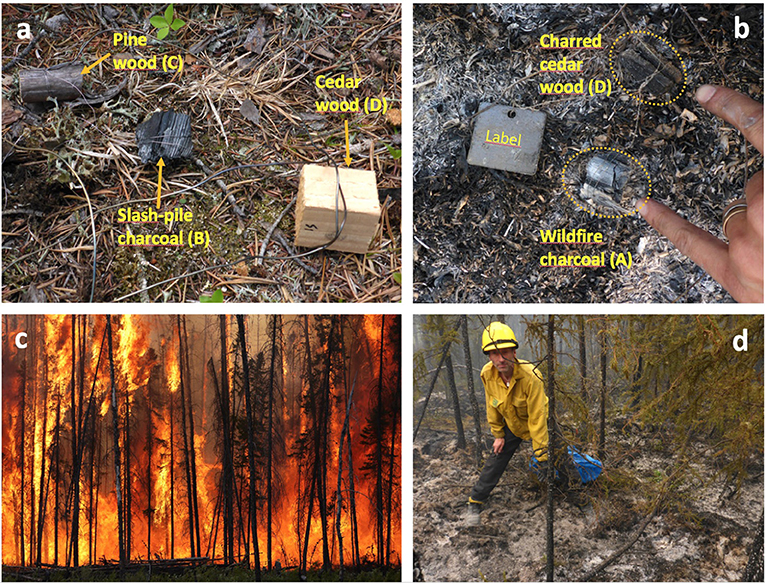
Figure 1. (A) A sample set placed on the forest floor prior to the high-intensity fire. The samples are connected by a thin wire to facilitate relocation. The thicker wires are thermocouples. (N.B. The pine wood sample appears dark, but was free of bark. The wildfire charcoal is not visible and was located just below the image frame.) (B) Examples of charred samples after the high-intensity fire. (C) The high intensity fire. (D) Sampling after the low-intensity fire where much of the standing vegetation remained unaffected. The figure is published with the consent of the depicted individual (the lead author) in (D).
A–C pieces were 2–3 cm in diameter and 2–4 cm in length. D blocks were 3 × 3 × 3 cm. All were free of bark. Sets were made with one piece of each type (A+B+C+D), and the four pieces were linked together by thin wire and with a metal label added to facilitate relocation after fire (Figure 1B). A total of 30 sets were placed onto the forest floor <24 h prior to exposure to two types of boreal wildfire conditions: a high-intensity crown fire and a low-intensity surface fire (i.e., 15 sets for each fire). Individual pieces were situated at least 5 cm apart (Figure 1A).
K-type thermocouples (≤ 1 mm diameter) were attached directly to the surface of the samples and connected to data loggers (Lascar, Easylog) buried in the adjacent soil to record the temperature at the sample surface-flame interface during the fire (at 10 s intervals). Given the limited number of loggers available, we chose to attach thermocouples to only one type of charcoal (A: wildfire charcoal) on the basis that any differences in temperature/duration data between the two types of charcoal might be expected to be less than between the charcoal and the contrasting types of wood. All B, C, and D samples had thermocouples attached.
Immediately after the fires samples were identified (Figure 1B), thermocouples and loggers removed and samples placed in sealed containers for subsequent laboratory analysis.
Wildfire Burn Experiments
(1) The high-intensity crown fire was an experimental fire conducted on 15/6/2015 as part of the Canadian Boreal Community FireSmart Project at Ft. Providence, (61°34'55” N; 117°10'13” W) and aimed at simulating wildfire conditions. The experimental plot (~2,000 m2) was a mature stand of black spruce and jack pine, originating from a stand-replacing wildfire in 1931, with a tree density (live and dead, >1.5 m high) of approximately 5,500 stems ha−1 and an average canopy tree height of 11 m (a typical mature stand height in this boreal region). The understorey was very sparse, the forest floor composed of litter, mosses and lichens, with some down wood present. Fifteen sets of A-D samples (Figures 1A,B) were placed randomly at least 1 m apart from each other within an area of 15 × 15 m. The fire was fast moving with a total burn time of <5 mins and flame lengths >5 m above canopy height (Figure 1C). The overall fireline intensity was estimated as ~8,000 kW m−1, which is within the typical range of high-intensity boreal crown fires in Canada (de Groot et al., 2009). The fire resulted in the homogenous and complete consumption of the canopy, thin branches and the understory, with no unburnt or low severity patches remaining within the plot.
(2) The low-intensity fire was situated ~170 km east of the high-intensity fire site (60°49′38″ N; 114°24′28″ W). It was a slow-moving, surface wildfire (Fire 28, Hay River) affecting a mixed black spruce and jack pine stand of comparable stand characteristics to the high-intensity fire, but with a peaty soil layer of up 50 cm depth. This lightning-caused wildfire burnt across the site on 29/6/2017 during calm atmospheric conditions, advancing at < 5 m h−1. This allowed sufficient time to place samples and thermocouples with loggers ahead of one of the flanks of the fire. Fifteen sets of A-D samples were placed 2 m apart along a transect parallel to the fire front. This surface fire led to the patchy smoldering combustion of the forest floor to a depth of up to 30 cm (Figure 1D), charring of trees to ~1 m height and the “torching” of a few individual trees, with some areas remaining unburned. The fireline intensity was estimated to not exceed 500 kW m−1 based on flame-length/intensity relationships for jack pine forest (Cruz and Alexander, 2010). Due to the patchy nature of this burn, not all samples were exposed to fire and, but in some cases, deep burning of the organic soil layer also led to the destruction of loggers and loss of data.
Further details about the fires are given in Table 1.
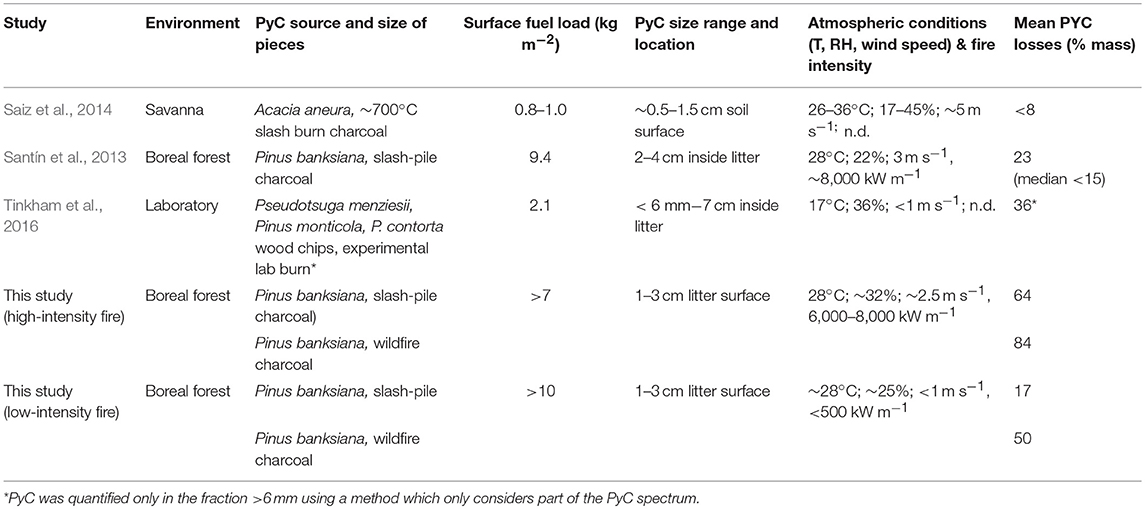
Table 1. Experimental conditions and resulting PyC losses in previous- and the current studies (n.d., no data; T, ambient air temperature, and RH, relative humidity immediately prior to ignition).
Laboratory Analyses
The air-dry weight of all samples (N = 120) was determined before and after exposure to fire, and mass loss calculated as:
Of the total 120 samples, 90 had thermocouples attached, and of those, 19 loggers were lost or did not record properly during the fires. A subset of 40 samples (5 of each type A-D for each fire) spanning a representative range of mass losses and temperature/duration records, and pre-fire reference samples (n = 1–5 for each type A–D) were selected for further analyses to allow the potential relationships between mass loss, sample characteristics, and temperature exposure during the fires to be examined.
Differential Scanning Calorimetry (DSC)
These analyses were carried out using a Mettler Toledo instrument to determine the thermolability (Merino et al., 2014) of the different samples before and after the exposure to the fires. Approximately 4 mg of ground sample was placed in aluminum pans under a flow of dry air (under O2 flux; flow rate, 50 mL s−1) at a scanning rate of 10°C min−1. The temperature ranged between 50 and 600°C. Samples of indium (mp: 156.6°C) were used to calibrate the calorimeter. Samples were analyzed in duplicate. The heat of combustion (Q) was determined by integrating the DSC thermographs with respect to time over the exothermic region (150 < T < 600°C). The region T < 150°C was not considered as it is dominated by endothermic reactions associated with water loss. The areas under the thermographs were divided into three temperature regions representing different levels of resistance of the organic material to thermal oxidation (Merino et al., 2014, 2015): between 150 and 375°C (Q1), between 375 and 475°C (Q2) and between 475 and 600°C (Q3). The temperature at which 50% of the total energy is released under the given conditions (T50) was also determined (Rovira et al., 2008).
Elemental Composition
Total carbon (C), nitrogen (N) and hydrogen (H) contents (%) were determined using a LECO elemental analyzer. Values reported were corrected by mineral ash content (as determined by DSC): e.g., C ash-corrected = (C*100)/Total OM loss.
The percentage of the C remaining in samples after fire (%Crem) compared to their initial C content was also calculated as follows using the ash-corrected C% values:
For the cedar and pine wood pieces (C & D sample types) that were fully charred following fire, this figure is equivalent to the conversion rate of fire-affected C to PyC (%PyC). This rate quantifies the fraction of fire-affected C that is retained as PyC in charred material rather than emitted to the atmosphere (Santín et al., 2015).
Charcoal Reflectance
Charcoals have been observed to have varying abilities to reflect light when studied under oil using a reflectance microscope, which has been shown to evolve throughout pyrolysis (e.g., Belcher and Hudspith, 2016). Therefore, we anticipated that charcoal reflectance might vary between the types of fires that created or modified the PyC examined in this study. In order to assess this, the PyC samples were sliced into sections using a band saw and embedded in polyester resin, then ground and polished using a Buehler MetaServ 250 grinder–polisher (Buehler, Neckar, Germany). The samples were placed in the resin such that the top surface exposed to the fire was the polished face following the method described in Belcher and Hudspith (2016). The polished samples were analyzed under oil (RI 1.514) at 238°C, using a Zeiss Axio-Scope A1 optical microscope, with a TIDAS-MSP 200 microspectrometer (SMCS Ltd, Baldock, UK). Thirty manual photometric reflectance measurements were taken at cell-wall junctions across the polished surface of all 35 sufficiently sized post-fire samples and the mean charcoal reflectance (Ro%) calculated for each sample.
Statistical Analysis
Statistical analyses were performed with the software IBM SPSS Statistics 20. The level of significance used for all tests was 5% (i.e., α = 0.05). Pearson product-moment correlation coefficients (r) were calculated to test linear correlations between pairs of variables. Variables examined were: mass loss (%), TG-DSC parameters (T50, Q1, Q3), C, N, H contents (%), initial C remaining (%Ci), and Ro (%), as well as maximum temperature recorded on samples (Tmax), time >300°C (s), integral of duration >300°C (°Cs). The threshold of 300°C was chosen because is widely considered the temperature above which charring begins in woody fuels (Drysdale, 2011), the main chemical transformations of biomass-derived materials during heating start around 200–300°C (Keiluweit et al., 2010), and it having been used in previous studies focusing on PyC (e.g., Santín et al., 2016b, 2017).
To analyze the differences in mass loss (%) between sample types, one-factor ANOVA was performed using sample type as the independent factor. Before the ANOVAs, the equality of variances for the studied groups was tested by the Levene's homoscedasticity test and the post-hoc Duncan's multiple range test (groups had equal variances) were performed to identify classes with significantly different means.
Results
Sample Properties Before the Fires
The two unburned wood samples used in this study (types C and D) showed the lowest % C, the highest contents of thermolabile biomass and the lowest thermal recalcitrance (Q1 >33%; T50 <420°C), with the cedar wood (D) being more thermolabile than the pine wood (Table 2, Figure 2). In comparison, both charcoal types (A and B) showed much higher C content and thermal recalcitrance, and lower thermolability (Q1 <21%; T50 >447°C). As expected, the slash-pine charcoal (B) presented, by far, the highest C% and thermal recalcitrance of all the samples before fire (Table 2).
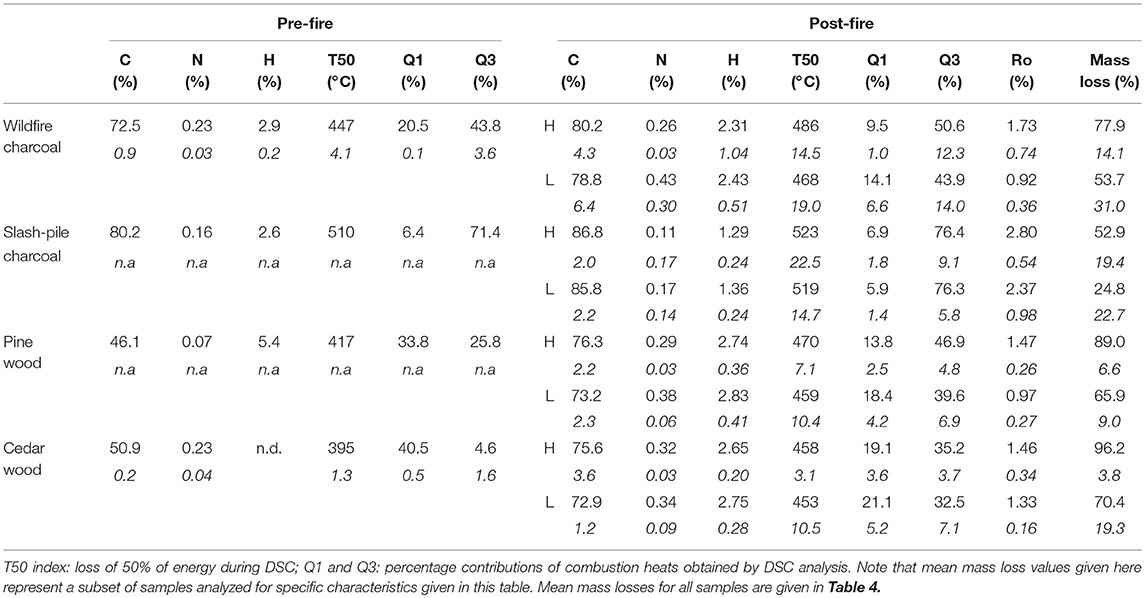
Table 2. Arithmetic mean values and standard deviation (italicized) of elemental composition and outcomes of thermal analysis (Differential Scanning Calorimetry; DSC) before and after fire, and of mass loss and reflectance (Ro) after fire, of the four different sample types of samples for the high-intensity (H) and low-intensity (L) fires.
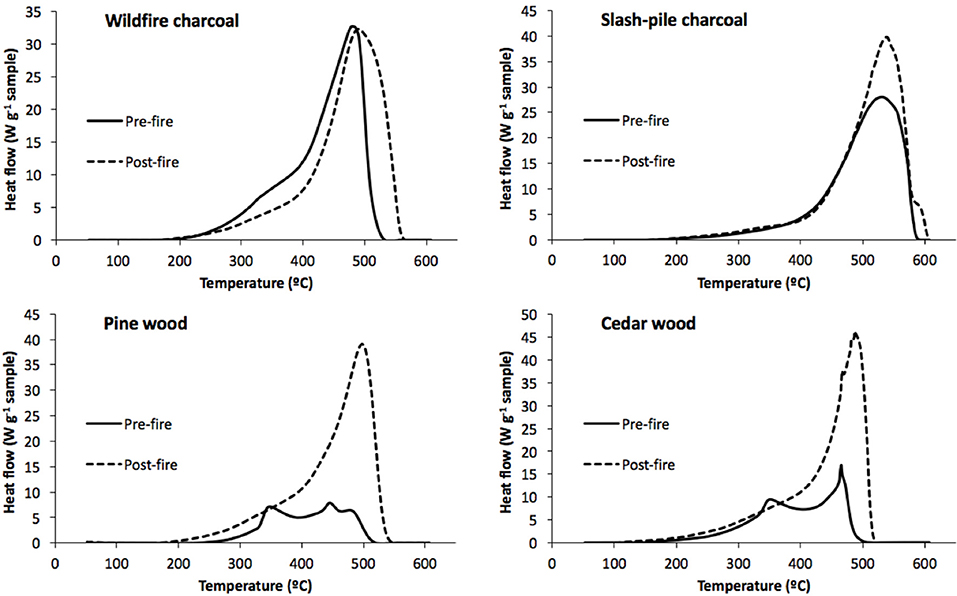
Figure 2. Comparison of the differential scanning calorimetry curves (arithmetic mean values of 5 samples each) of the four samples types before and after the fire.
Mass Losses and Their Correlations With Temperature/Duration Records
Considering data from both fires together (N = 101), wildfire charcoal (type A), exhibited a significantly higher mean mass loss (66.5 ± 25.2%) compared to slash-pile charcoal (Type B, 41.7 ± 27.2%). Pine wood (type C) showed higher mean mass losses (78.2 ± 14.9%) compared to both charcoal types A&B, and cedar wood (type D) the greatest losses (83.8 ± 18.9%). However, given the high variability in the data, the differences between mean mass losses between the groups of wildfire charcoal, pine wood and cedar wood were not significant.
For the combination of all the samples with temperature data associated (N = 71) and of the three fire parameters analyzed, Tmax showed the strongest positive correlation (r = 0.64) with mass loss (Table 3, Figure 3). Correlation between mass loss and the integral >300°C was also significant, but weaker (r = 0.48). No significant correlation was found between mass loss and time >300°C (r = 0.33) (Supplementary Table 1).
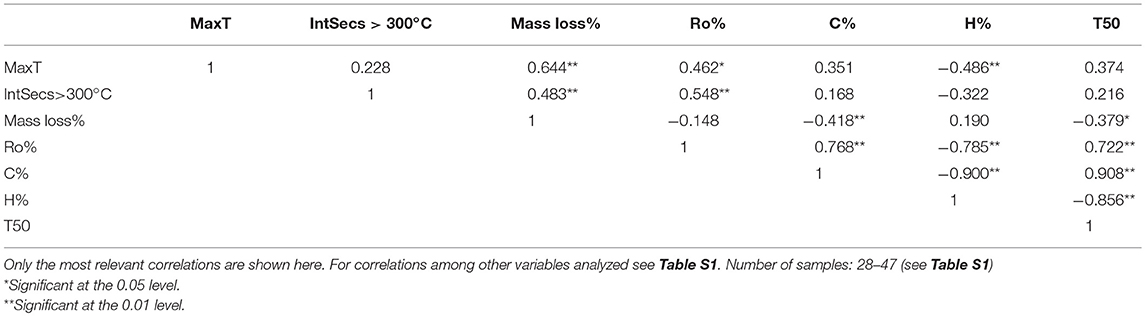
Table 3. Pearson product-moment correlation coefficients (r) between selected chemical and reflectance characteristics of the studied samples, and key fire parameters.
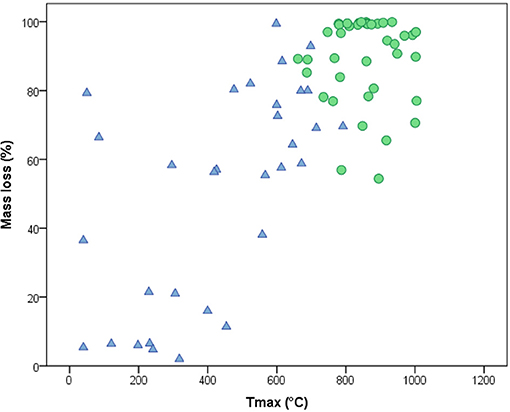
Figure 3. Mass loss versus maximum temperature recorded for all cedar wood, pine wood and wildfire charcoal samples (N = 71; slash-pile charcoal samples did not have temperature data associated). Green circles, high-intensity fire; blue circles, low-intensity fire.
When comparing the two fires (Figure 4), the mean maximum temperature (Tmax) recorded on all samples in the high-intensity fire was 855°C (range 661–1005°C; N = 39), whereas in the low-intensity fire it was only 434°C (range 40–792°C; N = 32). Indeed nearly all Tmax values recorded on samples in the high-intensity fire exceed those of low-intensity fire (Figure 4). The high-intensity fire also exhibited longer mean residence times (>300°C) than the low-intensity fire, although these differences between fires were less pronounced (Figure 4). Mean sample exposure durations >300°C were 245 s (range 70–570 s) and 142 s (0–620 s) for the high- and low-intensity fire respectively, and the mean integrals >300°C were 53,656°C s (range 20,689–122,798°C s) and 18,589°C s (range 0–99,327°C s) for these respective fires. All samples exposed to the high-intensity fire, and most samples exposed to the low-intensity fire experienced mass losses, with these mean losses being significantly higher in the wood pieces (80.7 ± 16.8%; n = 49) than in charcoal pieces (54.7 ± 28.5%; n = 52) and in the high-intensity than in the low-intensity fire (80.4 ± 18.6%; n = 47 vs. 53.7 ± 27.4%; n = 54). These significant differences in losses between fire types agree with their contrasting temperature records (Table 4, Figure 4). Regarding the differences in mass losses between the two fires for the different sample types, mass losses were consistently smaller in the low-intensity than in the high-intensity fire and in both cases following the order D>C>A>B (Figure 5). The variability of these losses was higher in the low-intensity fire in all cases except type D, the slash-pile charcoal (Figure 5).
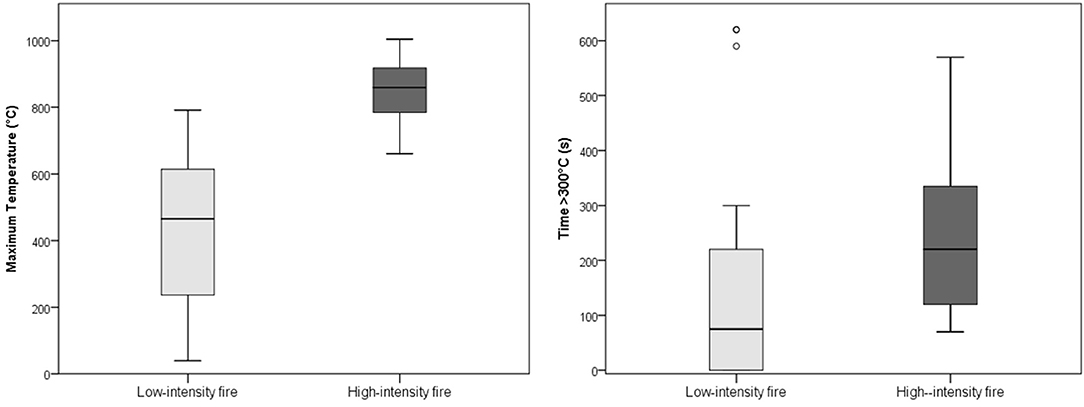
Figure 4. Maximum temperatures (°C) (left) and residence times of T > 300°C (right) recorded at the sample-flame interface during the low- and high-intensity fires for all sample types combined (N = 71). Circles indicate outliers.
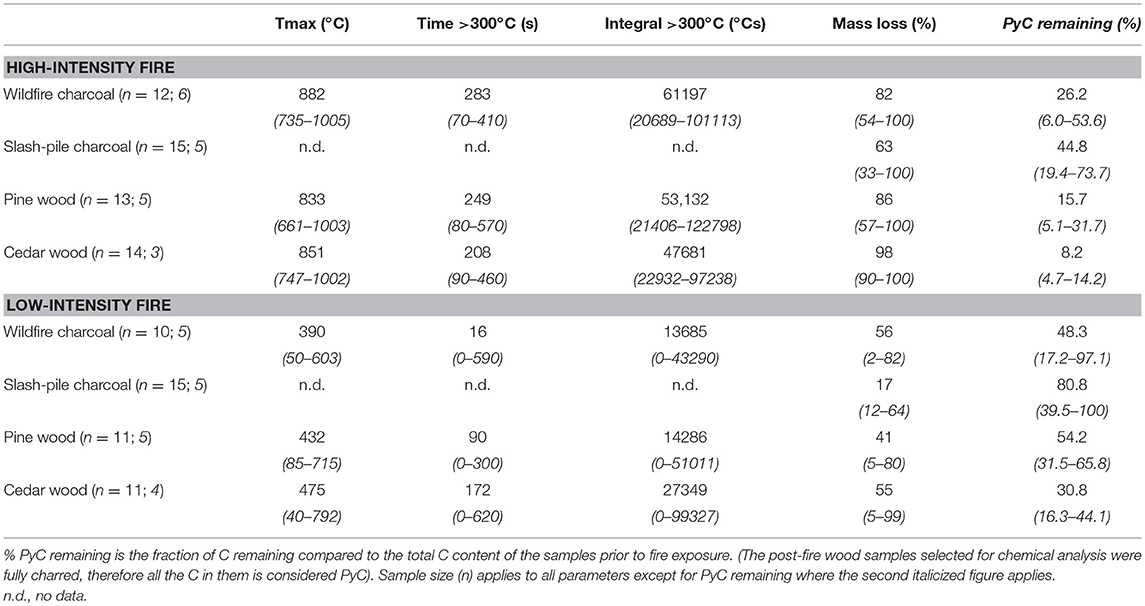
Table 4. Mean values (and range) of temperature characteristics, mass losses and % PyC remaining, separated by sample and fire types.
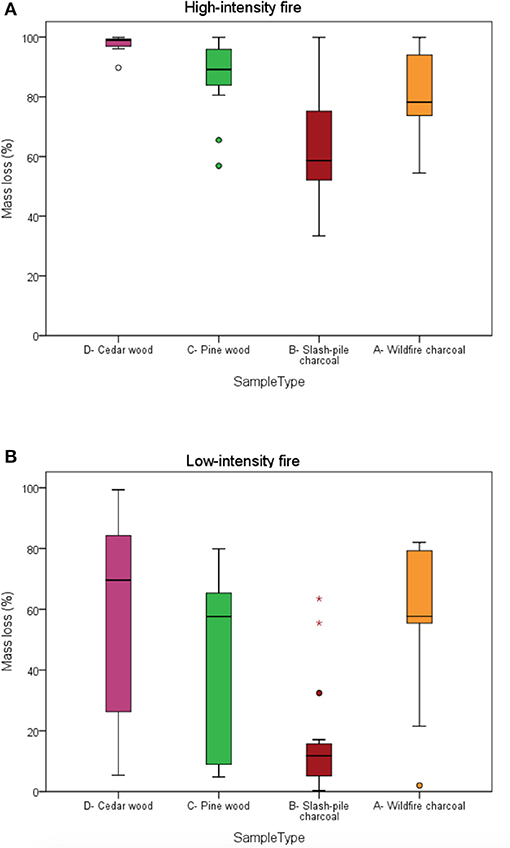
Figure 5. Mass losses for the different type of samples for the (A) high - and (B) low-intensity fire. Circles and stars indicate outliers. Total N = 101.
Thermal-, Elemental-, and Reflectance Characteristics
Results of the elemental-, DSC- and reflectance analyses for each fire are given in Table 2, and DSC curves for each sample type in Figure 2. As highlighted before, the DSC analyses show that, prior to the fires, the slash-pile charcoal was the most thermally recalcitrant (i.e., least thermolabile) material used in this experiment (mean T50 = 510°C) followed by wildfire charcoal (447°C), pine wood (417°C), and cedar wood (395°C). This order is reflected also in the increasing values for Q1 and decreasing values for Q3 (Table 2).
After sample exposure to fire, the same order is retained, but with substantial increases in thermal recalcitrance for the wood samples following charring, and only modest increases for the charcoal samples (Table 2, Figure 2). These increases in recalcitrance were greater in the high- than the low-intensity fire, which matches the greater thermal exposure of the samples in the former (Table 4).
The C concentration also increased in all sample types following fire exposure, but this change was much more pronounced for the wood samples than charcoal samples (Table 2). Given that exposure to fire does not only lead to mass- and therefore C losses through combustion, but also to relative enrichment in C in the remaining PyC through pyrolysis, we also calculated the % of PyC remaining in relation to the total C content of the samples prior to exposure (Table 4). Mean values of PyC% remaining were, respectively for high- and low-intensity fires: 26.2 vs. 48.3 (wildfire charcoal), 44.8 vs. 80.8 (slash charcoal), 15.7 vs. 54.2 (pine wood) and 8.2 vs. 30.8 (cedar wood). As highlighted in the methodology, for the fully charred wood samples C&D, this parameter represents the fuel C to PyC conversion rate used in Santín et al. (2015). It is interesting to note that the low-intensity fire resulted in a higher mean PyC production ratio (54.2 and 30.8%) than the high-intensity fire (15.7 and 8.2%) for the pine and cedar wood samples respectively.
Regarding N and H concentrations, N increased and H decreased after fire for most sample types (Table 2). Charcoal reflectance (Ro) following the fires was highest for slash-pile charcoal with values for the other sample types being around half this value (Table 2). Values following the high-intensity fire overall exceed those following the low-intensity fire with respectively mean Ro values of 2.80 vs. 2.37 (slash charcoal), 1.73 vs. 0.92 (wildfire charcoal), 1.47 vs. 0.97 (pine wood), and 1.46 vs. 1.33 (cedar wood).
Correlations Between Sample Characteristics, Charcoal Reflectance and Fire Properties
When considering all samples exposed to the fires together, significant correlations were found between some sample chemical and reflectance characteristics, and with fire properties (Table 3). For example, T50 showed positive correlations with C% (r = 0.91), Ro (r = 0.72) and a negative correlation with mass loss (−0.38) and with H% (r = −0.86). C% correlated with Ro (r = 0.72) and mass loss (−0.42). Maximum temperature at the fuel-flame interface (Tmax) showed not only a positive correlation with mass loss (r = 0.64) as presented in more detail above, but also with Ro (r = 0.46) and H% (r = −0.49). The duration integral of exposure >300°C was similarly well correlated to Ro (r = 0.55), and weaker (but still significantly) correlated to mass loss than Tmax (r = 0.48). All other relationships between fire characteristics, including exposure duration >300°C, showed weaker and non-significant relationships with sample chemical and reflectance characteristics (Table S1).
Discussion
Mass Losses Compared to Previous Studies
The only two previous studies examining PyC recombustion under field conditions reported mean mass losses of <8% for charcoal pieces of ~0.5–1.5 cm (Saiz et al., 2014) and of 23% (median value <15%) for charcoal pieces of 2–4 cm (Santín et al., 2013). In the current study, mass losses were substantially higher except for the slash-pile charcoal exposed to the low intensity fire, which had a mean loss of 17.0% (median 11.8%) (Table 4). In the high-intensity fire, slash-pile charcoal showed mean losses of 63.6% (median 58.6). Wildfire charcoal exhibited losses of 84.0% (median 81.9%) and 49.7% (median 58.6%) in the high- and low-intensity fires, respectively (Table 4). Thus, all losses recorded here across the two types of PyC and fires (i) exceed those previously reported from in-situ field studies and, except for the slash-pile charcoal in the low intensity fire, (ii) exceed also the mean losses of 36% for PyC fragments >6 mm reported in the laboratory study by Tinkham et al. (2016). The data presented in Table 1 allows comparison of the current study with fire and PyC characteristics in these previous studies.
The comparatively low losses in the study by Saiz et al. (2014) (<8%; Table 1) could be a reflection of a relatively high thermal resistance of their PyC combined with a low capacity of the fire to lead to PyC combustion. The source material, Acacia aneura, has roughly twice the density (1 g cm−3) of pine wood and was produced in a slash burn, which typically have much longer flame residence times than wildfire and therefore lead to a greater thermal degradation resistance of the charcoal produced than in a wildfire (Massman et al., 2008). The fire, although considered intense in the context of savanna fires, had the lowest fuel load and highest wind speed of all experimental fires listed in Table 1 and hence is likely to have led to a burn of comparatively low intensity and flame residence time.
The experimental crown fire examined by Santín et al. (2013) was carried out in the same fuel complex and study region as the current study and used the same source of slash-pile charcoal. Fuel load, atmospheric conditions and fireline intensity (~8,000 kW m−2) were also broadly comparable to the high intensity fire of current study (Table 1). The main difference between these two studies is that, in the current study, PyC pieces were placed on the forest floor surface, whereas in Santín et al. (2013) they were placed at ~2 cm depth in the forest floor layer. In the 2013 study, this could have resulted in the initial protection from thermal exposure of the charcoal pieces until the litter layer itself was consumed to this depth. This may have contributed to the substantially lower combustion of the slash-charcoal of 23% reported by Santín et al. (2013) compared to the 64% in the high-intensity fire of the current study (Table 1).
The mass losses of both slash-pile charcoal in the high-intensity fire and of wildfire charcoal in both fires of the current study are substantially greater than the 36% found in the laboratory burns of Tinkham et al. (2016) (Table 1). Their experiment, however, is not directly comparable to the current study not only due to it being carried out under laboratory conditions using masticated fuels. They also considered only PyC pieces >6 mm and within those, only the fraction that is resistant to chemical degradation based on the CTO375 method (Hatten and Zabowski, 2009). In addition, the sample placement on the litter surface, the substantially higher fuel loads and warmer and drier atmospheric conditions in the current study are all more conducive to a greater combustion completeness than those in the laboratory burn by Tinkham et al. (2016).
Mass Losses and Their Correlations With Temperature/Duration Records
Our research design allowed us to link PyC losses directly to temperature recorded on the fuel-flame interface of the respective PyC pieces. That mass losses show a reasonably strong correlation with Tmax (r = 0.65; Table 3) is perhaps not surprising. The thermocouple tip (≤ 1 mm diameter) reflects the temperature at the fuel-flame interface, which is influenced by the energy received from the surrounding combustion combined with that released from the burning PyC piece itself. Tmax could therefore be expected to be at least somewhat related to the mass loss of a PyC piece, with higher temperatures reflecting greater losses. What may be surprising is that mass losses are only weakly correlated with exposure duration >300°C (r = 0.33, not significant; Table S1). Three Hundred degree is widely considered the threshold above which charring begins in woody fuels (Drysdale, 2011) and hence the duration above this threshold could be expected to correlate more closely with mass loss than Tmax. Given that the correlation between mass loss and the integral >300°C was stronger (r = 0.48) than for time >300°C (and significant), suggests that the faster rate of combustion associated with the process that generates higher temperature readings in thermocouples is more important in the overall mass loss process observed here than the total time during which mass loss occurs. This notion is supported by a study examining organic matter in a soil block affected by experimental laboratory burns, where the time >300°C also showed no correlation with C loss (Merino et al., 2018).
The fact that the samples exposed to the high-intensity fire recorded substantially higher mean values for Tmax, the time integral (s) >300°C and exposure duration >300°C than the lower intensity fire (Table 4) provide not only an additional set of measures confirming the contrasting intensities of these fires. It also suggests that once combustion of individual PyC pieces begins, the greater rate of thermal energy release during the more intense fire facilitates a greater rate of loss of individual PyC pieces. This, together with the fact that exposure duration >300°C showed only a weak (and not significant) correlation with mass loss (Table S1), suggest that the energy released from the combustion of the surrounding fuel may be a more important factor in PyC mass loss than that released from the combustion of a PyC piece itself.
Mass Losses, PyC Characteristics and Thermal Recalcitrance
Our data also allows PyC losses in a fire to be evaluated in relation to the specific PyC characteristics. The importance of considering PyC type when evaluating potential losses in fires is clearly highlighted by our finding that slash-pile charcoal, which exhibits higher thermal degradation resistance according to the DSC analysis (Table 2), indeed exhibited a significantly lower mass loss under actual wildfire conditions (41.7 ± 27.2%; mean of both fires combined) compared to wildfire charcoal (66.5 ± 25.2%). This supports the speculation made by Santín et al. (2013), that the slash-pile charcoal placed in their fire could be expected to be more thermally resistant and may therefore have given lower rates of losses than if wildfire charcoal had been used. Prior to the new fire exposure, the slash-pile charcoal was more thermally recalcitrant (mean T50 = 510°C) than wildfire charcoal (447°C; Table 2) due to its origin in a pile burn, which generates prolonged burning conditions with restricted oxygen supply compared to typical wildfires (Santín and Doerr, 2016). This difference in thermal resistance is also reflected in the higher C content and lower values of Q1, and higher values of Q3 for the slash-pile compared to the wildfire charcoal (Table 2). Following fire exposure, both charcoal types exhibit an additional, albeit small, increase in mean values of T50, C%, and Q3 (Table 2, Figure 2). This suggests that the exposure of this charcoal to a new fire has led to its further increased thermal recalcitrance, particularly in the high-intensity fire, which can be expected to lower the susceptibility to combustion of this remaining PyC in a subsequent fire. Unsurprisingly, the wood pieces that were exposed to the high-intensity fire showed greater mass loss than in the low-intensity fire.
It is noteworthy that the low-intensity fire led to a higher PyC production rate than the high-intensity fire (as reflected by percentage PyC remaining for the wood samples; Table 4). This could suggest that more intense fires produce less PyC in relation to fuel consumed and hence emit a greater proportion of C to the atmosphere than is converted to PyC and remains in situ for a given amount of fuel affected by burning (Santín et al., 2015).
The significant correlations between chemical characteristics of samples, and their relationship with fire properties (Table 3), are also noteworthy. For example, they provide valuable evidence from real wildfires that some chemical parameters, which are often utilized to indicate thermal and chemical recalcitrance (C%, T50, Q3) are well correlated (Table 3) and indeed provide useful indicators of the resistance of PyC materials to combustion in field-scale fire conditions.
Furthermore, our data support the notion that charcoal reflectance (Ro) can be related to post-fire chemical recalcitrance parameters such as resistance to dichromate etch and the size of polyaromatic units remaining used in other studies (Ascough et al., 2010, 2011). The significant correlations relationships of Ro% with T50 (r = 0.72) and C% (0.77; Table 3), as well as with other DSC parameters (Table S1), suggest that Ro may be a useful indicator of charcoal resistance to thermal degradation. This is important because it implies that PyC exhibiting higher Ro% will not only be more chemically inert in the post-fire environment (e.g., Ascough et al., 2010; Belcher et al., 2018), but also that such PyC will be more resistant to thermal degradation in subsequent fires. The potential of this additional metric for exploring charcoal recalcitrance, its resistance to thermal degradation in future wildfires, and more generally fire effects on C storage in wood and charcoal, is explored in more detail in Belcher et al. (2018). The results also highlight that the DSC analysis methods used here provide a relatively rapid and economic approach for the assessment of the resistance of PyC to removal from subsequent fires.
Significance and Implications for Wildfire as a PyC Production and Removal Mechanism
The data presented here add to the still small, but growing body of evidence for the relevance of fire as a removal mechanism of any PyC exposed at the ground surface. Whilst experimental conditions and PyC analysis methods are not fully comparable across the four studies examining this process experimentally to date (Table 1), the current study stands out with the highest overall PyC losses from recombustion reported to date. The mean losses of 82 and 56% for wildfire charcoal in the high- and low-intensity boreal wildfires found here respectively, suggest that fire can indeed be a significant removal mechanism for any PyC that was produced in previous fires and remains exposed at the ground surface. These values, however, should be considered as a being in the extreme upper range of what might be expected under natural conditions for the following reasons. First, the PyC pieces were placed on the surface and not inside the litter layer. Under most natural situations, PyC would be subject to bioturbation and burial in the soil or within the accumulating litter, resulting in reduced exposure to thermal energy and hence combustion. This may be particularly relevant in the North American boreal region where the typical intervals between fires is relatively long, ranging from 150 to 200 years (Stocks et al., 2003; Balshi et al., 2009). Second, the pieces used here were within a size range of 1–3 cm, which represents only a fraction of the full size-range of PyC in the environment (Santín et al., 2015). For example, PyC present on the surface of larger pieces of down wood or standing timber could be expected to show lower losses due to their relatively smaller fuel surface area/volume ratio. To further improve our understanding of the role of wildfire as a removal mechanism of PyC, we need not only more experimental data on PyC recombustion from different types of fires and environments, but critically also a better understanding of the fate and movement of PyC between fires [see review by (Abney and Behre, 2018)] in order to assess how much of the PyC produced in a given fire is likely to be exposed to combustion in a subsequent fire.
What this study has demonstrated for the first time under field conditions is (i) the contrasting resistance of different types of PyC to combustion even when produced from the same material (in this case pine wood), and (ii) the contrasting net PyC losses different types of fire can cause. The significantly greater losses found for wildfire PyC compared to slash-pile PyC found here also match what would be expected based on the chemical and reflectance characteristics of the PyC types used here (Table 2) and supports the usefulness of these characteristics in reflecting thermal degradation resistance under field conditions.
The results of this study also demonstrate that wildfire charcoals do present different chemical characteristics and resistance to combustion than other types of man-made PyC such as the slash-pile charcoal used here. The latter may be more comparable to the more degradation resistant charcoal produced in kilns or, to some extent, also to biochar than to wildfire charcoal, confirming the need for considering their direct comparability with caution as highlighted by Santín et al. (2017) in their study comparing wildfire charcoal and biochar. This is especially relevant in the context of the C sequestration abilities of different PyC materials (Santín et al., 2017).
Based on the results presented here, it could be argued that more intense flaming fires that lead to higher temperatures at the fuel-flame interface will produce new PyC of higher recalcitrance compared to lower-intensity (and “cooler”) flaming fires. However, as also seen here, more intense flaming fires are likely to also produce less PyC in relation to fuel consumed, but (ii) potentially also consume overall more of any old PyC that may be available for combustion. If these notions prove to be widely applicable, they have implications for the accounting of PyC production and fate in local- to global-scale carbon budget modeling of fires.
In this context, it is important to consider that deep-burning smoldering fires, that would also be considered as low-intensity, would not fit this pattern as they lead to relatively complete combustion of the affected fuel (Rein, 2013), which would include any PyC present. To what degree fire intensity (or indeed fire behavior in general) and other factors such as fuel characteristics, PyC redistribution and its breakdown in the landscape between fires, influence (i) the total amount of PyC present in the landscape and (ii) its resistance to biotic and abiotic degradation, remains poorly understood today. Recent work by Hudspith et al. (2017), for example, has highlighted important differences between specific fuel types in how much charcoal they produce under comparable laboratory burn conditions. Further work representative of a range of environmental and fire behavior characteristics is clearly needed to provide a more complete understanding of the production and fate of PyC, and its role in the global carbon cycle. Developing this understanding is particularly relevant given that human-induced climate- and land cover changes are leading to large-scale changes in fuel characteristic, fire occurrence, and fire severity (Jolly et al., 2015; Abatzoglou and Williams, 2016). These shifts will affect the overall role of fire in the global carbon balance, which in turn will be affected by the balance of PyC production and its fate (Bird et al., 2015; Santín et al., 2016a).
Author Contributions
SD, CS, and CB conceived the work. SD, CS, and GB carried out the field experimentation, AM conducted the thermal and chemical-, and CB the reflectance analyses. All authors contributed substantially to the interpretation of the data and to drafting the manuscript. All authors gave final approval of the version submitted and agree to be accountable for all aspects of the work in ensuring that questions related to the accuracy or integrity of any part of the work are appropriately investigated and resolved.
Funding
Fieldwork and sample analysis was supported a Leverhulme Trust grant to SD (RPG-2014-095) and a European Research Council Starter Grant ERC-2013-StG-335891-ECOFLAM to CB. During manuscript preparation, SD was supported by a Leverhulme Trust Fellowship (RF-2016-456\2) and CS by a Sêr Cymru Fellowship co-funded by European Union's Horizon 2020 research and innovation programme (Marie Skłodowska-Curie grant agreement No 663830).
Conflict of Interest Statement
The authors declare that the research was conducted in the absence of any commercial or financial relationships that could be construed as a potential conflict of interest.
The reviewer, RL, declared a shared affiliation, with no collaboration, with one of the authors, CB, to the handling editor at time of review.
Acknowledgments
Special thanks go to Westly Steed and the Department of Environment and Natural Resources, Government of the Northwest Territories, Canada for supporting the field work. Thanks also the staff of FPInnovations, Alberta Environment and Sustainable Resource Development and the residents of Fort Providence for their support during fieldwork.
Supplementary Material
The Supplementary Material for this article can be found online at: https://www.frontiersin.org/articles/10.3389/feart.2018.00127/full#supplementary-material
References
Abatzoglou, J. T., and Williams, A. P. (2016). Impact of anthropogenic climate change on wildfire across western US forests. Proc. Natl. Acad. Sci. U.S.A. 113, 11770–11775. doi: 10.1073/pnas.1607171113
Abney, R. B., and Behre, A. A. (2018). Pyrogenic carbon erosion: implications for stock and persistence of pyrogenic carbon in soil. Front. Earth Sci. Biogeosci. 6:26. doi: 10.3389/feart.2018.00026
Alexander, M. E., Stefner, C. N., Mason, J. A., Stocks, B. J., and Hartley, G. R. (2004). Characterizing the Jack Pine—Black Spruce Fuel Complex of the International Crown Fire Modelling Experiment (ICFME). Sault Ste. Marie: Canadian Forest Service.
Ascough, P. L., Bird, M. I., Francis, S. M., Thornton, B., Midwood, J., Scott, A. C., et al. (2011). Variability in oxidative degradation of charcoal: influence of production conditions and environmental exposure. Geochim. Cosmochim. Acta 75, 2361–2378. doi: 10.1016/j.gca.2011.02.002
Ascough, P. L., Bird, M. I., Scott, A. C., Collinson, M. E., Cohen-Ofri, I., Snape, C. E., et al. (2010). Charcoal reflectance measurements: implications for structural characterization and assessment of diagenetic alteration. J. Archaeol. Sci. 37, 1590–1599. doi: 10.1016/j.jas.2010.01.020
Balshi, M. S., McGuire, A. D., Duffy, P., Flannigan, M., Walsh, J., and Melillo, J. (2009). Assessing the response of area burned to changing climate in western boreal North America using a multivariate adaptive regression splines (MARS) approach. Glob. Change Biol. 15, 578–600. doi: 10.1111/j.1365-2486.2008.01679.x
Belcher, C. M., and Hudspith, V. A. (2016). The formation of charcoal reflectance and its potential use in post-fire assessments. Int. J. Wildl. Fire 25, 775–779. doi: 10.1071/WF15185
Belcher, C. M., New, S. L., Santín, C., Doerr, S. H., Grosvenor, M. J., Dewhirst, R., et al. (2018). What can charcoal reflectance tell us about energy release in wildfires and charcoal properties? Front. Earth Sci. Biogeosci. 6:169. doi: 10.3389/feart.2018.00169
Bird, M. I., Wynn, J. G., Saiz, G., Wurster, C. M., and McBeath, A. (2015). The pyrogenic carbon cycle. Annu. Rev. Earth Planet. Sci. 43, 273–298. doi: 10.1146/annurev-earth-060614-105038
Bruun, S., and EL-Zehery, N. (2012). Biochar effect on the mineralization of soil organic matter. Pesq. Agropec. Bras. 47, 665–671. doi: 10.1590/S0100-204X2012000500005
Cruz, M. G., and Alexander, M. E. (2010). Assessing crown fire potential in coniferous forests of western North America: a critique of current approaches and recent simulation studies. Int. J. Wildl. Fire 19, 377–398. doi: 10.1071/WF08132
Czimczik, C. I., and Masiello, C. A. (2007). Controls on black carbon storage in soils. Glob. Biogeochem. Cycles 21, 1–8. doi: 10.1029/2006GB002798
Czimczik, C. I., Schmidt, M. W. I., and Schulze, E. D. (2005). Effects of increasing fire frequency on black carbon and organic matter in Podzols of Siberian Scots pine forests. Eur. J. Soil Sci. 56, 417–428. doi: 10.1111/j.1365-2389.2004.00665.x
de Groot, W. J., Pritchard, J. M., and Lynham, T. J. (2009). Forest floor fuel consumption and carbon emissions in Canadian boreal forest fires. Can. J. For. Res. 39, 367–382. doi: 10.1139/X08-192
de Lafontaine, G., Couillard, P. L., and Payette, S. (2011). Permineralization process promotes preservation of Holocene macrofossil charcoal in soils. J. Quat. Sci. 26, 571–575. doi: 10.1002/jqs.1529
Foereid, B., Lehmann, J., and Major, J. (2011). Modeling black carbon degradation and movement in soil. Plant Soil 345, 223–236. doi: 10.1007/s11104-011-0773-3
Hatten, J. A., and Zabowski, D. (2009). Changes in soil organic matter pools and carbon mineralization as influenced by fire severity. Soil Sci. Soc. Am. J. 73, 262–273. doi: 10.2136/sssaj2007.0304
Hudspith, V. A., Belcher, C. M., Barnes, J., Dash, C. B., Kelly, R., and Hu, F. S. (2017). Charcoal reflectance suggests heating duration and fuel moisture affected burn severity in four Alaskan tundra wildfires. Int. J. Wildl. Fire 26, 306–316. doi: 10.1071/WF16177
Huston, M. A., and Wolverton, S. (2009). The global distribution of net primary production: resolving the paradox. Ecol. Monogr. 79, 343–377. doi: 10.1890/08-0588.1
Jolly, W. M., Cochrane, M. A., Freeborn, P. H., Holden, Z. A., Brown, T. J., Williamson, G. J., et al. (2015). Climate-induced variations in global wildfire danger from 1979 to 2013. Nat. Commun. 6:7537. doi: 10.1038/ncomms8537
Kane, E. S., Hockaday, W. C., Turetsky, M. R., Masiello, C. A., Valentine, D. W., Finney, B. P., et al. (2010). Topographic controls on black carbon accumulation in Alaskan black spruce forest soils: implications for organic matter dynamics. Biogeochemistry 100, 39–56. doi: 10.1007/s10533-009-9403-z
Keiluweit, M., Nico, P. S., Johnson, M. G., and Kleber, M. (2010). Dynamic molecular structure of plant biomass-derived black carbon (biochar). Environ. Sci. Technol. 44, 1247–1253. doi: 10.1021/es9031419
Landry, J.-S., and Matthews, H. D. (2017). The global pyrogenic carbon cycle and its impact on the level of atmospheric CO 2 over past and future centuries. Glob. Chang. Biol. 23, 3205–3218. doi: 10.1111/gcb.13603
Massman, W. J., Frank, J. M., and Reisch, N. B. (2008). Long-term impacts of prescribed burns on soil thermal conductivity and soil heating at a Colorado Rocky Mountain site: a data/model fusion study. Int. J. Wildl. Fire 17, 131–146. doi: 10.1071/WF06118
Merino, A., Chávez-vergara, B., Salgado, J., Fonturbel, M. T., García-oliva, F., and Vega, J. A. (2015). Variability in the composition of charred litter generated by wildfire in different ecosystems. Catena 133, 52–63. doi: 10.1016/j.catena.2015.04.016
Merino, A., Ferreiro, A., Salgado, J., Fontúrbel, M. T., Barros, N., Fernández, C., et al. (2014). Use of thermal analysis and solid-state 13C CP-MAS NMR spectroscopy to diagnose organic matter quality in relation to burn severity in Atlantic soils. Geoderma 226–227, 376–386. doi: 10.1016/j.geoderma.2014.03.009
Merino, A., Fonturbel, M. T., Fernández, C., Chávez-vergara, B., Salgado, J., García-oliva, F., and Vega, J. (2018). Inferring changes in soil organic matter in soil burn severities after wildfire in temperate climates. Sci. Total Environ. 627, 622–632. doi: 10.1016/j.scitotenv.2018.01.189
Naisse, C., Girardin, C., Davasse, B., Chabbi, A., and Rumpel, C. (2014). Effect of biochar addition on C mineralisation and soil organic matter priming in two subsoil horizons. J. Soil Sediments 15, 825–832. doi: 10.1007/s11368-014-1002-5
Ohlson, M., and Tryterud, E. (2000). Interpretation of the charcoal record in forest soils: forest fires and their production and deposition of macroscopic charcoal. Holocene 10, 519–525. doi: 10.1191/095968300667442551
Preston, C. M., and Schmidt, M. W. I. (2006). Black (pyrogenic) carbon in boreal forests: a synthesis of current knowledge and uncertainties. Biogeoscience 3, 211–271. doi: 10.5194/bgd-3-211-2006
Rein, G. (2013). “Smouldering fires and natural fuels,” in Fire Phenomena in the Earth System–An Interdisciplinary Approach to Fire Science, ed C. Belcher (London: Wiley and Sons), 15–34.
Rovira, P., Kurz-Besson, C., Coûteaux, M. M., and Ramón Vallejo, V. (2008). Changes in litter properties during decomposition: a study by differential thermogravimetry and scanning calorimetry. Soil Biol. Biochem. 40, 172–185. doi: 10.1016/j.soilbio.2007.07.021
Saiz, G., Goodrick, I., Wurster, C. M., Zimmermann, M., Nelson, P. N., and Bird, M. I. (2014). Charcoal re-combustion efficiency in tropical savannas. Geoderma 219–220, 40–45. doi: 10.1016/j.geoderma.2013.12.019
Santín, C., and Doerr, S. H. (2016). Fire effects on soils: the human dimension. Proc. R. Soc. B Biol. Sci. 371:20150171. doi: 10.1098/rstb.2015.0171
Santín, C., Doerr, S. H., Kane, E. S., Masiello, C. A., Ohlson, M., de la Rosa, J. M., et al. (2016a). Towards a global assessment of pyrogenic carbon from vegetation fires. Glob. Chang. Biol. 22, 76–91. doi: 10.1111/gcb.12985
Santín, C., Doerr, S. H., Merino, A., Bryant, R., and Loader, N. J. (2016b). Forest floor chemical transformations in a boreal forest fire and their correlations with temperature and heating duration. Geoderma 264, 71–80. doi: 10.1016/j.geoderma.2015.09.021
Santín, C., Doerr, S. H., Merino, A., Bucheli, T. D., Bryant, R., Ascough, P., et al. (2017). Carbon sequestration potential and physicochemical properties differ between wildfire charcoals and slow-pyrolysis biochars. Sci. Rep. 7:11233. doi: 10.1038/s41598-017-10455-2
Santín, C., Doerr, S. H., Preston, C., and Bryant, R. (2013). Consumption of residual pyrogenic carbon by wildfire. Int. J. Wildl. Fire 22, 1072–1077. doi: 10.1071/WF12190
Santín, C., Doerr, S. H., Preston, C. M., and González-Rodríguez, G. (2015). Pyrogenic organic matter production from wildfires: a missing sink in the global carbon cycle. Glob. Change Biol. 21, 1621–1633. doi: 10.1111/gcb.12800
Santos, F., Torn, M. S., and Bird, J. (2012). Biological degradation of pyrogenic organic matter in temperate forest soils. Soil Biol. Biochem. 51, 115–124. doi: 10.1016/j.soilbio.2012.04.005
Steinbeiss, S., Gleixner, G., and Antonietti, M. (2009). Effect of biochar amendment on soil carbon balance and soil microbial activity. Soil Biol. Biochem. 41, 1301–1310. doi: 10.1016/j.soilbio.2009.03.016
Stocks, B. J., Alexander, M. E., Wotton, B. M., Stefner, C. N., Flannigan, M. D., Taylor, S. W., et al. (2004). Crown fire behaviour in a northern jack pine - black spruce forest. Can. J. For. Res. 34, 1548–1560. doi: 10.1139/x04-054
Stocks, B. J., Mason, J., Todd, J. B., Bosch, E. M., Wotton, B. M., Amiro, B. D., et al. (2003). Large forest fires in Canada, 1959–1997. J. Geophys. Res. 108:8149. doi: 10.1029/2001JD000484
Thevenon, F., Williamson, D., Bard, E., Anselmetti, F. S., Beaufort, L., and Cachier, H. (2010). Combining charcoal and elemental black carbon analysis in sedimentary archives: implications for past fire regimes, the pyrogenic carbon cycle, and the human-climate interactions. Glob. Planet. Change 72, 381–389. doi: 10.1016/j.gloplacha.2010.01.014
Keywords: black carbon, boreal forest, carbon balance, charcoal, charcoal reflectance, wildfire, management burn, thermal analysis
Citation: Doerr SH, Santín C, Merino A, Belcher CM and Baxter G (2018) Fire as a Removal Mechanism of Pyrogenic Carbon From the Environment: Effects of Fire and Pyrogenic Carbon Characteristics. Front. Earth Sci. 6:127. doi: 10.3389/feart.2018.00127
Received: 15 December 2017; Accepted: 10 August 2018;
Published: 10 October 2018.
Edited by:
Satish Myneni, Princeton University, United StatesReviewed by:
Ronny Lauerwald, Free University of Brussels, BelgiumDimitris Poursanidis, Foundation for Research and Technology Hellas, Greece
Copyright © 2018 Doerr, Santín, Merino, Belcher and Baxter. This is an open-access article distributed under the terms of the Creative Commons Attribution License (CC BY). The use, distribution or reproduction in other forums is permitted, provided the original author(s) and the copyright owner(s) are credited and that the original publication in this journal is cited, in accordance with accepted academic practice. No use, distribution or reproduction is permitted which does not comply with these terms.
*Correspondence: Stefan H. Doerr, cy5kb2VyckBzd2FuLmFjLnVr