- 1Department of Geology, Wittenberg University, Springfield, OH, United States
- 2Mendenhall Laboratory, School of Earth Sciences and Byrd Polar Research Center, Ohio State University, Columbus, OH, United States
We present a synthesis of the trace element chemistry in melt on the surface Canada Glacier, Taylor Valley, McMurdo Dry Valleys (MDV), Antarctica (~78°S). The MDV is largely ice-free. Low accumulation rates, strong winds, and proximity to the valley floor make these glaciers dusty in comparison to their inland counterparts. This study examines both supraglacial melt streams and cryoconite holes. Supraglacial streams on the lower Canada Glacier have median dissolved (<0.4 μm) concentrations of Fe, Mn, As, Cu, and V of 71.5, 75.5, 3.7, 4.6, and 4.3 nM. All dissolved Cd concentrations and the vast majority of Pb values are below our analytical detection (i.e., 0.4 and 0.06 nM). Chemical behavior did not follow similar trends for eastern and western draining waters. Heterogeneity likely reflects distinctions eolian deposition, rock:water ratios, and hydrologic connectivity. Future increases in wind-delivered sediment will likely drive dynamic responses in melt chemistry. For elements above detection limits, dissolved concentrations in glacier surface melt are within an order of magnitude of concentrations observed in proglacial streams (i.e., flowing on the valley floor). The Fe enrichment of cryoconite water relative to N, P, or Si exceeds enrichment observed in marine phytoplankton. This suggests that the glacier surface is an important source of Fe to downstream ecosystems.
Introduction
Cryoconite holes are small, water-filled holes formed on the surface of glacier ice by the deposition and accumulation of eolian material (Fountain and Tranter, 2008). Cryoconite holes on polar glaciers have frozen lids that minimize contact with the atmosphere (Bagshaw et al., 2013). Through time, cryoconite holes increase their interconnectivity and form supraglacial streams (Fountain and Tranter, 2008). Streams typically include a series of ice-covered pool and riffle sequences of varying size and dimensions that can fill and drain rapidly during the ablation season (Fountain et al., 2004; Bagshaw et al., 2007; Tranter et al., 2010). The large pool (10–50 m in diameter) have been termed cryolakes by Tranter et al. (2010). Glacier surface melt contribute most of the downstream because MDV glacier have frozen beds (Fountain et al., 2004; Tranter et al., 2010).
The eolian materials that initiate the development of cryoconite holes and supraglacial stream supply geologic material and inoculate the glacier surface with organisms. Organism can grow and develop thereby mobilizing nutrients and adding autochthonous production. Thus, unique ecosystems that include bacteria, diatoms, algal mats, and invertebrates develop in environments where nutrients such as N and P are recycled, organic carbon produced (Cameron et al., 2012; Porazinska et al., 2012; Stanish et al., 2013; Telling et al., 2014). Studies over the past decade have demonstrated that both solutes and organic matter accumulate in cryoconite holes through time (e.g., Bagshaw et al., 2007, 2011, 2013). Both nutrients and labile dissolved organic carbon (DOC) can not only be produced in these systems, but may also be transferred to downstream ecosystems as hydrologic connectivity is established during the ablation season (Bagshaw et al., 2013; Dubnick et al., 2017; SanClements et al., 2017). The accumulation of solutes is brought about through high rock:water ratios and relatively high residence times of eolian material encrusted in the cryoconite holes (Tranter et al., 2005; Bagshaw et al., 2013; Dubnick et al., 2017).
Studies of cryoconite and supraglacial stream biogeochemistry have focused on carbon and macronutrient (i.e., N and P) dynamics (e.g., Bagshaw et al., 2013). There have been only a few studies that have investigated major solute behavior (Fortner et al., 2005; Bagshaw et al., 2013). Still unknown is the composition of minor and trace elements in these environments. In fact only a few studies have measured trace element concentrations in cryoconite holes and these have focused on solids or leachates (Wientjes et al., 2011; Singh et al., 2012) or in liquid that had not been filtered (Fortner et al., 2011). The availability of bioaccessible trace elements may impact species composition, abundance, or functioning. Our work considers trace element concentrations from the filterable (< 0.4 μm) fraction of cryoconite holes and supraglacial streams. We examine the fate of this dissolved fraction as it is transported from supraglacial environments to the proglacial stream environment, and finally into its terminal path, the closed-basin lakes.
Materials and Methods
Site Description
The Canada Glacier is located on the northern side of Taylor Valley, Antarctica, 14 km west of the Ross Sea (Figure 1). Like all MVD glaciers, Canada Glacier features low accumulation rates, high sublimation rates, and cold temperatures (Fountain et al., 2004). Fountain et al. (2008) observed that 50% of the cryoconite holes are hydrologically connected and the other half are isolated. Seasonally, these holes are frozen most of the year and begin to thaw in November and by January they begin to freeze again (Bagshaw et al., 2007). The initial melt pulse from cryoconite holes releases high concentrations of solutes (Telling et al., 2014). Cryolakes are full, draining, and frequently drain during the peak of the ablation season. Discharge from these lakes on the Canada Glacier frequently reaches 2 l s−1 with maximums measured at 75 l s−1 (Bagshaw et al., 2007). This variability makes it difficult to develop predictive physical models based on to estimate their contributions to hydrology. The isolation time or residence time of water in cryoconite holes also varies. The mean residence time of cryoconite holes is 3.4 years on the Canada Glacier (Telling et al., 2014). The flux of chemistry from supraglacial environments into downstream proglacial streams and lakes depends on the age distribution of the cryoconite holes and their hydrologic connectivity (Bagshaw et al., 2007; Telling et al., 2014). This, of course, complicates flux calculations. Redox sensitive chemistry also varies substantively depending on the conditions during sampling. Supersaturations of O2 and high pHs have been observed in cryoconite holes due to photosynthesis and isolation from the atmosphere that limits gas exchange (i.e., both O2 and CO2 in this case) (Tranter et al., 2004; Bagshaw et al., 2011, 2013).
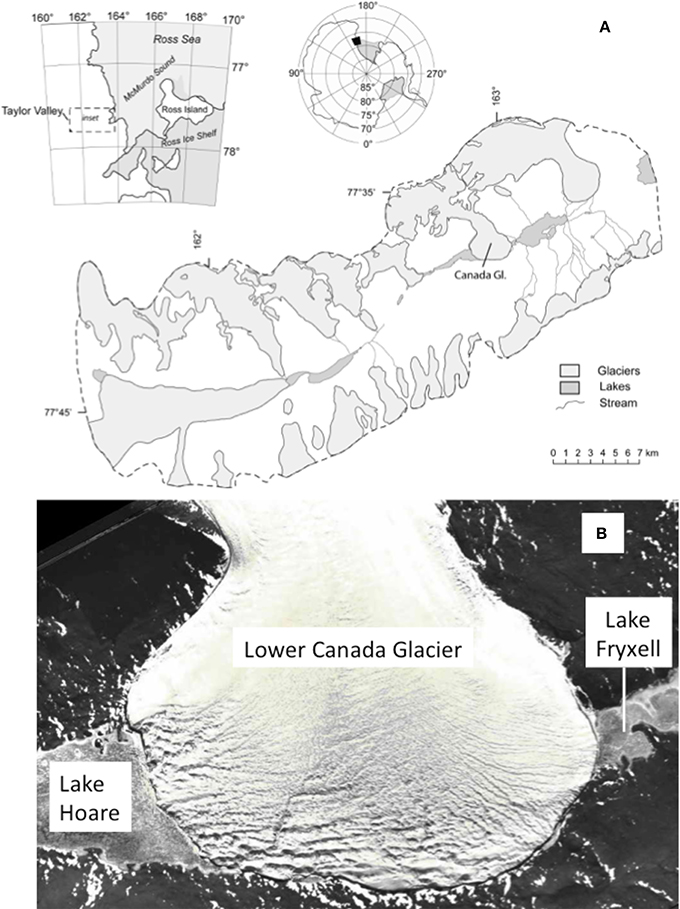
Figure 1. (A) Map of Taylor Valley Antarctica with Canada Glacier shown (reprinted from Lyons et al., 1998 with permission of the copyright holder AGU). (B) The lower Canada Glacier where cryoconite and supraglacial stream sampling occurred. Note the lower glacier feeds Andersen Creek and Canada Stream which drains into Lake Hoare and Lake Fryxell, respectively.
Supraglacial melt on the western side of the Canada Glacier either flows into Lake Hoare via a proglacial stream Andersen Creek or via direct inflow into the lake. On the eastern side of the glacier, supraglacial waters flow into Canada Stream and continue along the valley floor before reaching Lake Fryxell. The two proglacial streams have distinct biological, geomorphological and geochemical characteristics, in part, because of their position with respect to dominant W-E foehn winds that deposit eolian material onto the glacier surface (Lyons et al., 2003; Fortner et al., 2011, 2012).
Field Methods
In December 2006 and 2007 a total of 33 supraglacial streams and 4 cryoconite hole samples were collected for trace element analyses from the Canada Glacier ablation zone (Figure 1). The sampled holes were located primarily in the center of the lower ablation area of the glacier (Figure 2). The pH conditions was not measured for all cryoconite holes and supraglacial streams, but other studies report that cryoconites that have established hydrologic connectivity with supraglacial streams and supraglacial streams have somewhat acidic to circumneutral pH values (Fortner et al., 2005; Bagshaw et al., 2007; Foreman et al., 2007). Bagshaw et al. (2007) found that Canada Glacier wet cryoconite holes had a median pH of 7.2. Supraglacial stream sampling was conducted at locations that had obvious drainage into either the western and eastern side of glacier into the respective lake basins. Single samples were also collected from the moat waters of Lake Hoare and Lake Fryxell.
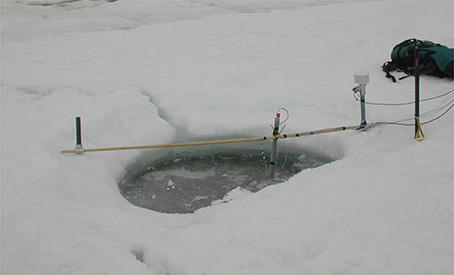
Figure 2. Cryoconite hole in Canada Glacier draining into a supraglacial stream. The diameter of the cryoconite hole is 1.5 m.
Low density polyethylene (LDPE) bottles were prepared for trace element sampling by soaking them in a10% v/v trace metal grade HCl/18 MΩ deionized water (DI). Bottles were then triple rinsed and filled with 1% ultraclean Optima™ HNO3/18 MΩ DI and stored in plastic bags. Sample collection was conducted by an individual wearing a Tyvek non-particulating suit, clean vinyl gloves that were changed prior to collecting each sample, and with careful attention to position downwind of the collection. Bottle blanks, LDPE bottles filled with 18 MΩ DI that were opened and shut in the field and filled with 18 MΩ DI in the lab, were all below sample detection limits. Samples were stored at 4°C and transported at this temperature back to Ohio State University where they were filtered using 0.45 μm Nucleopore™ membranes and then acidified to 2% v/v using Optima™ HNO3. This processing is similar to the techniques used for proglacial stream sampling (Fortner et al., 2012). Previous work (Fortner et al., 2011) compared unfiltered and acidified, termed “environmentally available” trace element concentrations in snow, supraglacial and proglacial streams to discern the partitioning of elements affiliated with particulate sources. The methods described herein relate to determining more soluble, filterable (< 0.4 μm) fraction that is reactive and bio-available.
Laboratory Methods
A Thermo Finnegan Element 2 Inductively Coupled Plasma Sector-Field Mass Spectrometer (ICP-SF-MS) was used to determine trace element concentrations as described in Fortner et al. (2011). Six calibration standards were used to capture the range of trace element concentrations. An internal check standard relative standard deviation (RSD) values never exceeded 10%. Accuracy was better than 15% for all elements as determined by the analysis of the certified reference standard, NWRI TM-RAIN 95, a trace metal rainwater, approximately every five samples. Detection limits were calculated as three times the standard deviation of the blanks, or equivalent to the worst filtration blank. These and other details on the technique and laboratory procedures are further described in Fortner et al. (2012). Cl− was measured, along with other major anions (not reported here), using an Ion Chromatograph using the methods of Welch et al. (2010). Precision of the Cl− data were better than 5% determined from replicates of the lowest standard.
Results
Medians, ranges, and detection limits of dissolved As, Cu, V, Fe, Mn concentrations measured in Canada Glacier cryoonite holes, western supraglacial streams and eastern supraglacial streams are reported in Table 1. Pb and Cd are not included because they were below detection limits of 0.4 and 0.06 nM. In the cryoconite holes median Fe and Mn concentrations were similar (71.5 and 75.5 nM). Likewise, As, Cu, and V concentrations were all approximately 4 nM. Low cryconite hole sample numbers limit our interpretation of dynamics, but they are still offer a first examination of trace elements in these systems. Western supraglacial streams had a greater median dissolved Fe concentration (102 nM) than eastern supraglacial streams (43 nM). Mn, As, Cu, and V all occurred in higher median concentrations in the eastern supraglacial streams.

Table 1. Median and range () of dissolved element concentrations (nM) in supraglacial waters of Canada Glacier, Taylor Valley, Antarctica.
We plotted differences in median chemistry between supraglacial and proglacial waters (Figure 3). Flow from the Canada Glacier surface enters two distinct basins. Therefore, we present chemistry for the western and eastern flowpaths. Only the cryoconite data collected near the flow divide is the same. Dissolved Fe increased from cryoconite holes to supraglacial streams to proglacial stream on the western side of the glacier. The western lake surface had a similar concentration to the cryconite holes. The eastern lake, Fryxell, had greater median dissolved Fe (130 nM) than the median concentration (72 nM) western lake (Lake Hoare). This is interesting because the eastern supraglacial waters occurred in lower median concentrations than on the western surface. Dissolved Mn decreased from cryoconite holes to supraglacial streams to proglacial streams on the western side of Canada Glacier, but remained relatively constant from cryoconite holes to eastern supraglacial streams. Dissolved Mn had similar concentrations to the moat waters of both Lake Hoare and Lake Fryxell even though their inflows from supra and proglacial waters were distinct. Dissolved As, Cu, and V similarly did not behave the same on both sides of the glacier. For example on the west, median dissolved As concentrations were greatest in the lake (4.6 nM) followed by cryoconite holes (3.7 nM), the proglacial stream (0.6 nM), and supraglacial stream (< 0.2 nM). In the western drainage, dissolved Cu occurred in its highest median concentrations in the cryoconite holes (4.6 nM) followed by proglacial streams (1.8 nM), supraglacial streams (0.8 nM), and Lake Hoare (0.3 nM). In the east, Lake Fryxell had the greatest Cu concentrations (5.4 nM) and both supraglacial streams and the proglacial stream were an order of magnitude lower. V concentrations were lower in the supraglacial streams than the cryoconite holes on both sides of the glacier, but interestingly the western proglacial stream had a greater median V concentration (9.6 nM) than the eastern proglacial stream (4.8 nM). Lakes were fairly similar to cryoconite hole concentrations.
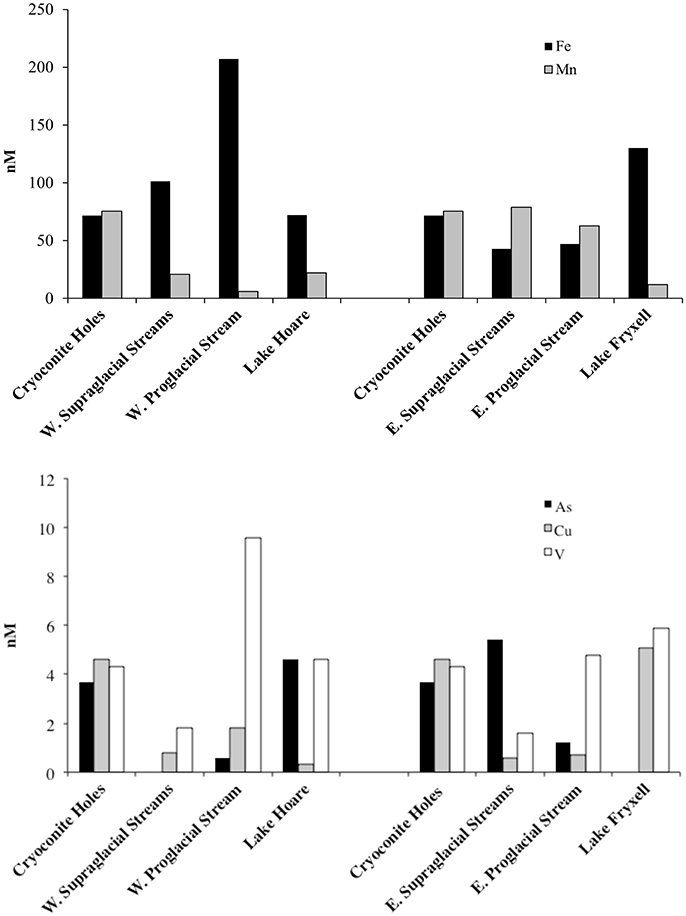
Figure 3. (Top) Median dissolved Fe and Mn concentrations (nM) in cryoconite holes, supraglacial streams, proglacial streams, and lakes. Note that western waters are presented on the left and eastern waters are on the right. (Bottom) Median dissolved As, Cu, and V concentrations (nM) in cryoconite holes, supraglacial streams, proglacial streams, and lakes. Note As was not measured in Lake Fryxell.
Discussion
Recent work has clearly demonstrated that the majority of eolian material is local (Deuerling et al., 2014). Therefore we interpret in-situ dissolution as the major source of solutes measured in the supraglacial environment. Although scientific activities contribute to material sources, they are minor contributor of trace elements (Lyons et al., 2000; Fortner et al., 2011). The behavior of trace and minor elements from glacier headwater (i.e., supraglacial streams and cryconite holes) into proglacial environments (i.e., proglacial streams and lakes) differs on between both sides of the drainage divide. This likely reflects highly-localized controls on chemistry such as valley floor composition, wind direction and strength, channel morphology, and the abundance and compositions of organisms in the materials blown onto the glacier surface (Porazinska et al., 2012). This work agrees with previous work showing that element loading to the glacier surface is more than an order of magnitude greater than inland Antarctic sites (Witherow et al., 2006; Williamson et al., 2007; Witherow and Lyons, 2008; Fortner et al., 2011). Differences between supraglacial and proglacial streams probably also reflect differences in substrate, and hence hyporheic exchange and chemical weathering (Nezat et al., 2001; Gooseff et al., 2002; Maurice et al., 2002; Dowling et al., 2013). Additionally, ice melt may release soluble iron into the cryoconites and streams similar to the release of dissolved Fe from Antarctic icebergs into seawater (Raiswell et al., 2008).
To think about biogeochemical reactivity in aquatic systems we have normalized dissolved As, Cu, V, Fe, and Mn to Cl− in Figure 4. Because Cl− behaves conservatively and does not take part in any biogeochemical process at these low total dissolved solids (TDS), this normalization allows us to better understand the behavior of these elements as they are transported from the glacier surface to the surface water of the lakes. For example, the As:Cl− ratio along the western and the eastern flowpaths show removal of As from the glacier surface into the proglacial streams. This suggests little chemical weathering input from the proglacial streams. However, As:Cl− increases from the proglacial stream values into the surface water of Lake Hoare. This may indicate that direct supraglacial input dominates the As concentrations in the lake. Cryoconite holes and Lake Hoare have approximately the same dissolved As concentration. Cu:Cl− decreases in the west, but not the east. The eastern fluctuating behavior is similar to variations in Cu:Cl− from melting snow to the proglacial stream environment on the Eliot Glacier Mount Hood (Fortner et al., 2009) which was attributed to desorption from particulate matter in the proglacial environment. Unlike Lake Hoare, Lake Fryxell surface waters have higher Cu:Cl− ratios than the supra and proglacial streams that supply them. The higher relative values in Lake Fryxell may reflect higher Cu2+ contributions from the other 10 streams contributing water to the lake. This is not likely a result of lake surface pH conditions (i.e., measured pH ≥ 8 by Green et al., 1988). In both flow directions the V:Cl− had the highest values in the proglacial streams indicating possible V solubilization in stream environments. Previous research has linked dissolved V input to increased aluminosilicate weathering (Shiller and Mao, 2000). Silicate weathering is a documented source of ions within MDV proglacial stream channels (Nezat et al., 2001).
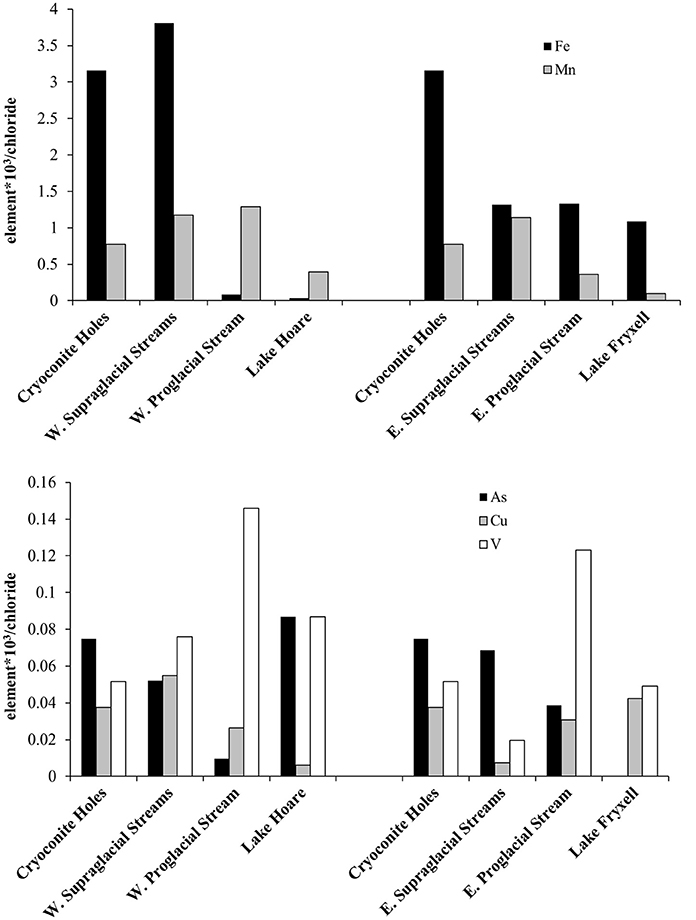
Figure 4. (Top) Median dissolved Fe and Mn concentrations normalized to chloride in cryoconite holes, supraglacial streams, proglacial streams and lakes. Note that western waters are presented on the left and eastern waters are on the right. (Bottom) Median dissolved As, Cu, and V concentrations normalized to chloride in cryoconite holes, supraglacial streams, proglacial streams and lakes.
Fe:Cl− ratio decreases dramatically in the western flowpath, while a large decrease only from the cryoconite holes to the supraglacial streams is observed in the eastern flowpath. In the eastern system, the Fe:Cl− stays relatively constant from supraglacial to proglacial stream to lakes suggesting either little Fe2+ loss from the system or a balance between input and output. Higher Fe:Cl− ratios on the east may also reflect the very much higher organic matter concentrations in Canada Stream (Cathey et al., 1981) with organic matter complexation impact the uptake and release of Fe. Organic matter may also impact Cu complexation in Antarctic environments (Bundy et al., 2013). Our limited examination showed that Cu:Cl ratios were lower in the eastern proglacial stream than the western one, unlike Fe. The Mn:Cl− ratio show a pattern of Mn loss in the lake waters, with relatively constant ratios prior to the lake. Previous work documents dynamic temporal changes in cryoconite hole redox conditions (Tranter et al., 2004) another important variable in metal dynamics. Similarly, UV sensitivity impacts the behavior of trace elements including Fe in the Southern Ocean (Rijkenberg et al., 2005), so future efforts should monitor these conditions. Overall, these patterns indicate non-conservative behavior of these elements as water moves through the supraglacial to lake hydrologic system. To better understand specific drivers of element behavior in glacier melt systems would take increased spatial and temporal monitoring to capture the evolution of glacier surface water and downstream dynamics. For example it would be important to capture longer isolation periods that generate greater solute concentrations as well as dilute connected systems (Tranter et al., 2004). A next step would be to monitor variations in microbial activity through time and observe the response of trace and minor elements. This has recently been accomplished in nearby Antarctic pond environments (Safi et al., 2012). Finally, other work suggests a need to explore particulate sources of trace elements in Antarctic streams (Hodson et al., 2017). This might be particularly important in these dusty MDV glaciers.
Castendyk et al. (2016) documented that increased foehn winds generated more sediment on glacier surfaces drove the rising levels of MDV Lake Vanda and other lakes not analyzed in detail. Wind deliveries of eolian sediment (Castendyk et al., 2016) coupled with increased warming around the edges of Antarctica may make MDV glaciers important sources of Fe to the Southern Ocean (Lyons et al., 2015). This is important because Fe is a limiting nutrient in the Southern Ocean (Raiswell et al., 2008). For this reason we explore N:P:Si:Fe nutrient stoichiometry of cyoconite holes. Using the dissolved inorganic nitrogen and dissolved phosphorus and silicon data from Bagshaw et al. (2013), and the filterable Fe data presented here, we establish the cryoconite hole N:P:Si:Fe ratio as 25:1:25:0.36 (Table 2). Note that the relative amount of iron in cryoconite holes is orders of magnitude higher than marine phytoplankton. Therefore, glacier surfaces, and not just proglacial streams (Lyons et al., 2015) may be an important future source of Fe to the Southern Ocean. Work in the Pine Island region also suggests that glacier melt, contributes substantively to dissolved iron loaded into the ocean (Hodson et al., 2017).
Conclusions
While this study does not constrain elemental dynamics, it is an important first look at the relative abundance and heterogeneity of trace element chemistry in glacier surface melt. The ultimate fate of dissolved solutes from eolian-derived material is important to understanding MDV and Southern Ocean ecosystems. Temporal studies are needed to understand how trace element geochemistry evolves alongside melt evolution (i.e., from isolated cryoconite hole to hydrogically connected cryoconite hole-supraglacial stream). Future work might also explore solute and solid chemistry dynamics. If foehn winds continue to drive increased melt into the future, glacier surfaces will potentially increase their chemical deliveries to downstream ecosystems. Many questions remain, but our work suggests that as the ice margin warms, glacier surface melt will be an important source of trace element chemistry.
Author Contributions
SF and WL contributed to sample design, results, and discussion language. SF contributed figure analyses methods.
Conflict of Interest Statement
The authors declare that the research was conducted in the absence of any commercial or financial relationships that could be construed as a potential conflict of interest.
Acknowledgments
We would like to thank Anthony Lutton, John Olesik, Rae Spain, and Kathleen Welch for their help with laboratory or field operations. This work was supported by NSF Grant ANT-0423595.
References
Bagshaw, E. A., Tranter, M., Fountain, A. G., Welch, K. A., Basagic, H., and Lyons, W. B. (2007). Biogeochemical evolution of cryoconite holes on Canada Glacier, Taylor Valley, Antarctica. J. Geophys. Res. Biogeosci. 112:G04S35. doi: 10.1029/2007JG000442
Bagshaw, E. A., Tranter, M., Fountain, A. G., Welch, K., Basagic, H. J., and Lyons, W. B. (2013). Do cryoconite holes have the potential to be significant sources of C, N, and P to downstream depauperate ecosystems of Taylor valley, Antarctica? Arctic Antarctic Alpine Res. 45, 440–454. doi: 10.1657/1938-4246-45.4.440
Bagshaw, E. A., Tranter, M., Wadham, J. L., Fountain, A. G., and Mowlem, M. (2011). High-resolution monitoring reveals dissolved oxygen dynamics in an Antarctic cryoconite hole. Hydrol. Process. 25, 2868–2877. doi: 10.1002/hyp.8049
Brzezinski, M. A., Dickson, M. L., Nelson, D. M., and Sambrotto, R. (2003). Ratios of Si, C, and N uptake by microplankton in the Southern Ocean. Deep Sea Res. II Topical Stud. Oceanogr. 50, 619–633. doi: 10.1016/S0967-0645(02)00587-8
Bundy, R. M., Barbeau, K. A., and Buck, K. N. (2013). Sources of strong copper-binding ligands in Antarctic Peninsula surface waters. Deep Sea Res II Topical Stud. Ocean 90, 134–146. doi: 10.1016/j.dsr2.2012.07.023
Cameron, K. A., Hodson, A. J., and Osborn, A. M. (2012). Carbon and nitrogen biogeochemical cycling potentials of supraglacial cryoconite communities. Pol. Biol. 35, 1375–1393. doi: 10.1007/s00300-012-1178-3
Castendyk, D. N., Obryk, M. K., Leidman, S. Z., Gooseff, M., and Hawes, I. (2016). Lake Vanda: A sentinel for climate change in the McMurdo Sound Region of Antarctica. Glob. Planet. Change 144, 213–227. doi: 10.1016/j.gloplacha.2016.06.007
Cathey, D. D., Parker, B. C. Jr., Simmons G. M. Jr., Yongue, W. H., and Brunt, M. R. V. (1981). The microfauna of algal mats and artificial substrates in Southern Victoria Land lakes of Antarctica. Hydrobiology 85, 3–15. doi: 10.1007/BF00011340
Deuerling, K. M., Lyons, W. B., Welch, S. A., and Welch, K. A. (2014). The characterization and role of aeolian deposition on water quality, McMurdo Dry Valleys, Antarctica. Aeolian Res. 13, 7–17. doi: 10.1016/j.aeolia.2014.01.002
Dowling, C. B., Lyons, W. B., and Welch, K. A. (2013). Strontium isotopic signatures of streams from Taylor Valley, Antarctica, revisited: the role of carbonate mineral dissolution. Aquat. Geochem. 19, 231–240. doi: 10.1007/s10498-013-9189-4
Dubnick, A., Wadham, J., Tranter, M., Sharp, M., Orwin, J., Barker, J., et al. (2017). Trickle or treat: the dynamics of nutrient export from polar glaciers. Hydrol. Process 31, 1776–1789. doi: 10.1002/hyp.11149
Foreman, C. M., Sattler, B., Mikucki, J. A., Porazinska, D. L., and Priscu, J. C. (2007). Metabolic activity and diversity of cryoconites in the Taylor Valley, Antarctica. J. Geophys. Res. Biogeosci. 112, 1–11. doi: 10.1029/2006JG000358
Fortner, S. K., Berry Lyons, W., and Olesik, J. W. (2011). Eolian deposition of trace elements onto Taylor Valley Antarctic glaciers. Appl. Geochem. 26, 1897–1904. doi: 10.1016/j.apgeochem.2011.06.013
Fortner, S. K., Lyons, W. B., Fountain, A. G., Welch, K. A., and Kehrwald, N. M. (2009). Trace element and major ion concentrations and dynamics in glacier snow and melt: Eliot Glacier, Oregon Cascades. Hydrol. Process. 23, 2987–2996. doi: 10.1002/hyp.7418
Fortner, S. K., Lyons, W. B., and Munk, L. (2012). Diel stream geochemistry, Taylor Valley, Antarctica. Hydrol. Process. 27, 394–404. doi: 10.1002/hyp.9255
Fortner, S. K., Tranter, M., Fountain, A., Lyons, W. B., and Welch, K. A. (2005). The geochemistry of supraglacial streams of Canada glacier, Taylor Valley (Antarctica), and their evolution into proglacial waters. Aquat. Geochem. 11, 391–412. doi: 10.1007/s10498-004-7373-2
Fountain, A. G., Nylen, T. H., Tranter, M., and Bagshaw, E. (2008). Temporal variations in physical and chemical features of cryoconite holes on Canada Glacier, McMurdo Dry Valleys, Antarctica. J. Geophys. Res. 113:G01S92. doi: 10.1029/2007JG000430
Fountain, A. G., Tranter, M., Nylen, T. H., Lewis, K. J., and Mueller, D. R. (2004). Evolution of cryoconite holes and their contribution to meltwater runoff from glaciers in the McMurdo Dry Valleys, Antarctica. J. Glaciol. 50, 35–45. doi: 10.3189/172756504781830312
Gooseff, M. N., McKnight, D. M., Lyons, W. B., and Blum, A. E. (2002). Weathering reactions and hyporheic exchange controls on stream water chemistry in a glacial meltwater stream in the McMurdo Dry Valleys. Water Res. Res. 38, 15-1–15-17. doi: 10.1029/2001WR000834
Green, W. J., Angle, M. P., and Chave, K. E. (1988). The geochemistry of antarctic streams and their role in the evolution of four lakes of the McMurdo dry valleys. Geochim. Cosmochim. Acta 52, 1265–1274. doi: 10.1016/0016-7037(88)90280-3
Hodson, A., Nowak, A., Sabacka, M., Jungblut, A., Navarro, F., Pearce, D., et al. (2017). Climatically sensitive transfer of iron to maritime Antarctic ecosystems by surface runoff. Nature Comm. 8:14499. doi: 10.1038/ncomms14499
Ho, T. Y., Quigg, A., Finkel, Z. V., Milligan, A. J., Wyman, K., Falkowski, P. G., et al. (2003). The elemental composition of some marine phytoplankton. J. Phycol. 39, 1145–1159. doi: 10.1111/j.0022-3646.2003.03-090.x
Lyons, W. B., Dailey, K. R., Welch, K. A., Deuerling, K. M., Welch, S. A., and McKnight, D. M. (2015). Antarctic streams as a potential source of iron for the Southern Ocean. Geology 43:G36989.1. doi: 10.1130/G36989.1
Lyons, W. B., Nezat, C. A., Welch, K. A., Kottmeier, S. T., and Doran, P. T. (2000). Fossil fuel burning in Taylor Valley, southern Victoria Land, Antarctica: estimating the role of scientific activities on carbon and nitrogen reservoirs and fluxes. Environ. Sci. Technol. 34, 1659–1662. doi: 10.1021/es990794l
Lyons, W. B., Welch, K. A., Fountain, A. G., Dana, G. L., Vaughn, B. H., and McKnight, D. M. (2003). Surface glaciochemistry of Taylor Valley, southern Victoria Land, Antarctica and its relationship to stream chemistry. Hydrol. Process.17, 115–130. doi: 10.1002/hyp.1205
Lyons, W. B., Welch, K. A., Neumann, K., Toxey, J. K., McArthur, R., Williams, C., et al. (1998). “Geochemical linkages among glaciers, streams and lakes within the Taylor Valley, geochemical linkages among glaciers, streams and lakes within the Taylor Valley, Antartica,” in Ecosystem Dynamics in a Polar Desert: the Mcmurdo Dry Valleys, Antarctica, ed J. C. Priscu (Washington, DC: American Geophysical Union), 77–92.
Maurice, P. A., McKnight, D. M., Leff, L., Fulghum, J. E., and Gooseff, M. (2002). Direct observations of aluminosilicate weathering in the hyporheic zone of an Antarctic dry valley stream. Geochim. Cosmochim. Acta 66, 1335–1347. doi: 10.1016/S0016-7037(01)00890-0
Nezat, C. A., Lyons, W. B., and Welch, K. A. (2001). Chemical weathering in streams of a polar desert (Taylor Valley, Antarctica). Geol. Sci. Am. Bull. 113, 1401–1408. doi: 10.1130/0016-7606(2001)113<1401:CWISOA>2.0.CO;2
Porazinska, D. L., Fountain, A. G., Nylen, T. H., Tranter, M., Virginia, R. A., and Wall, D. H. (2012). The biodiversity and biogeochemistry of cryoconite holes from McMurdo Dry Valley glaciers, Antarctica. Arctic Antarctic Alpine Res. 36, 84–91. doi: 10.1657/1523-0430(2004)036[0084:TBABOC]2.0.CO;2
Raiswell, R., Benning, L. G., Tranter, M., and Tulaczyk, S. (2008). Bioavailable iron in the Southern Ocean: the significance of the iceberg conveyor belt. Geochem. Trans. 9:7. doi: 10.1186/1467-4866-9-7
Rijkenberg, M. J. A., Fischer, A. C., Kroon, J. J., Gerringa, L. J. A., Timmermans, K. R., Wolterbeek, H. T., et al. (2005). The influence of UV irradiation on the photoreduction of iron in the Southern Ocean. Mar. Chem. 93, 119–129. doi: 10.1016/j.marchem.2004.03.021
Safi, K., Hawes, I., and Sorrell, B. (2012). Microbial population responses in three stratified Antarctic meltwater ponds during the autumn freeze. Antarctic Sci. FirstView. 24, 571–588. doi: 10.1017/S0954102012000636
SanClements, M. D., Smith, H. J., Foreman, C. M., Tedesco, M., Chin, Y. P., Jaros, C., et al. (2017). Biogeophysical properties of an expansive Antarctic supraglacial stream. Antarctic Sci. 29, 33–44. doi: 10.1017/S0954102016000456
Shiller, A. M., and Mao, L. (2000). Dissolved vanadium in rivers: effects of silicate weathering. Chem. Geol. 165, 13–22. doi: 10.1016/S0009-2541(99)00160-6
Singh, S. M., Sharma, J., Gawas-Sakhalkar, P., Upadhyay, A. K., Naik, S., Pedneker, S. M., et al. (2012). Atmospheric deposition studies of heavy metals in Arctic by comparative analysis of lichens and cryoconite. Environ. Monitor. Assess. 185, 1367–1376. doi: 10.1007/s10661-012-2638-5
Stanish, L. F., Bagshaw, E. A., McKnight, D. M., Fountain, A. G., and Tranter, M. (2013). Environmental factors influencing diatom communities in Antarctic cryoconite holes. Environ. Res. Lett. 8:045006. doi: 10.1088/1748-9326/8/4/045006
Telling, J., Anesio, A. M., Tranter, M., Fountain, A. G., Nylen, T., Hawkings, J., et al. (2014). Spring thaw ionic pulses boost nutrient availability and microbial growth in entombed Antarctic dry valley cryoconite holes. Front. Microbiol. 5:694. doi: 10.3389/fmicb.2014.00694
Tranter, M., Bagshaw, E., Fountain, A., and Foreman, C. (2010). “The biogeochemistry and hydrology of McMurdo Dry valley glaciers: is there life on Martian ice now,” in Life in Antarctic Deserts and Other Cold, Dry Environments: Astrobiol Analogues, eds. P. T. Doran, W. B. Lyons, and D. M. McKnight (Cambridge, UK: Cambridge University Press), 195–220.
Tranter, M., Fountain, A. G., Fritsen, C. H., Berry Lyons, W., Priscu, J. C., Statham, P. J., et al. (2004). Extreme hydrochemical conditions in natural microcosms entombed within Antarctic ice. Hydrol. Process. 18, 379–387. doi: 10.1002/hyp.5217
Tranter, M., Fountain, A. G., Lyons, W. B., Nylen, T. H., and Welch, K. A. (2005). The chemical composition of runoff from Canada Glacier, Antarctica: implications for glacier hydrology during a cool summer. Ann. Glaciol. 40, 15–19. doi: 10.3189/172756405781813753
Welch, K. A., Lyons, W. B., Whisner, C., Gardner, C. B., Gooseff, M. N., McKnight, D. M., et al. (2010). Spatial variations in the geochemistry of glacial meltwater streams in the Taylor Valley, Antarctica. Antarctic Sci. 22, 662–672. doi: 10.1017/S0954102010000702
Wientjes, I. G. M., Van de Wal, R. S. W., Reichart, G. J., Sluijs, A., and Oerlemans, J. (2011). Dust from the dark region in the western ablation zone of the Greenland ice sheet. Cryosphere 5, 589–601. doi: 10.5194/tc-5-589-2011
Williamson, B. R., Kreutz, K. J., Mayewski, P. A., Bertler, N. A. N., Sneed, S., Handley, M., et al. (2007). A coastal transect of McMurdo Dry Valleys (Antarctica) snow and firn: marine and terrestrial influences on glaciochemistry. J. Glaciol. 53, 681–693. doi: 10.3189/002214307784409225
Witherow, R. A., and Lyons, W. B. (2008). Mercury deposition in a polar desert ecosystem. Environ. Sci. Technol. 42, 4710–4716. doi: 10.1021/es800022g
Keywords: trace elements, cryoconite holes, supraglacial streams, Antarctica, glacier melt chemistry
Citation: Fortner SK and Lyons WB (2018) Dissolved Trace and Minor Elements in Cryoconite Holes and Supraglacial Streams, Canada Glacier, Antarctica. Front. Earth Sci. 6:31. doi: 10.3389/feart.2018.00031
Received: 20 December 2017; Accepted: 23 March 2018;
Published: 11 April 2018.
Edited by:
Martyn Tranter, University of Bristol, United KingdomReviewed by:
Andrew C. Mitchell, Aberystwyth University, United KingdomFrancois L. L. Muller, Qatar University, Qatar
Copyright © 2018 Fortner and Lyons. This is an open-access article distributed under the terms of the Creative Commons Attribution License (CC BY). The use, distribution or reproduction in other forums is permitted, provided the original author(s) and the copyright owner are credited and that the original publication in this journal is cited, in accordance with accepted academic practice. No use, distribution or reproduction is permitted which does not comply with these terms.
*Correspondence: Sarah K. Fortner, c2ZvcnRuZXJAd2l0dGVuYmVyZy5lZHU=