- 1Department of Geology, University of Illinois, Urbana, IL, United States
- 2Department of Earth and Environmental Sciences, University of Iowa, Iowa City, IA, United States
- 3Illinois State Geological Survey, Prairie Research Institute, University of Illinois, Urbana, IL, United States
- 4Department of Civil and Environmental Engineering, University of Illinois, Urbana, IL, United States
The concept of a critical zone (CZ) supporting terrestrial life has fostered groundbreaking interdisciplinary science addressing complex interactions among water, soil, rock, air, and life near Earth's surface. Pioneering work has focused on the CZ in areas with residual soils and steady-state or erosional topography. CZ evolution in these areas is conceptualized as progressive weathering of local bedrock (e.g., in the flow-through reactor model). However, this model is not applicable to areas in which weathering profiles form in transported materials including the formerly glaciated portion of the Central Lowland of North America. We present a new conceptual model of CZ evolution in landscapes impacted by continental glaciation based on investigations at three study sites in the Intensively Managed Landscapes Critical Zone Observatory (IML-CZO) The IML-CZO is devoted to the study of CZ processes in a region characterized by thick surficial deposits resulting from multiple continental glaciations, with bedrock at depths of up to 150 m. Here the physical (glacial ice, loess, developing soil profiles) and biological (microbes, tundra, forest, prairie) components of the CZ vary significantly in time. Moreover, the spatial relationships between mineral components of the CZ record a history of glacial-interglacial cycles and landscape evolution. We present cross-sections from IML-CZO sites to provide specific examples of how environmental change is recorded by the structure of the mineral components of the CZ. We build on these examples to create an idealized model of CZ evolution through a glacial cycle that represents the IML-CZO sites and other areas of low relief that have experienced continental glaciation. In addition, we identify two main characteristics of CZ structure which should be included in a conceptual model of CZ development in the IML-CZO and similar settings: (1) mineral components have diverse origins and transport trajectories including alteration in past CZs, and, (2) variability in climate, ecosystems, and hydrology during glacial-interglacial cycles profoundly influence the CZ composition, creating a legacy retained in its structure. This legacy is important because the current physical CZ structure influences the occurrence and rates of CZ processes, as well as future CZ responses to land use and climate change.
Introduction
The formerly glaciated portions of the Central Lowland of the USA (Fenneman and Johnson, 1946) are among the most intensively managed landscapes and agriculturally productive croplands in the world. The combination of deep fertile soils, temperate climate, and concentrated human interactions to improve growing conditions enables the highest rates of photosynthesis on the Earth (Guanter et al., 2014). Interactions between the atmosphere, hydrosphere, biosphere and lithosphere provide ecosystem services not only in pristine landscapes, but also in this human modified environment. Pioneering research into these interrelated systems at the Earth's surface recognizes a “critical zone” (CZ) that regulates habitats and determines the availability of life-sustaining resources. The CZ was originally defined as stretching from the top of the vegetation canopy to the lower limits of groundwater (e.g., NRC, 2001; Brantley et al., 2007). Yet emerging research suggests the lower CZ limit is better defined as the depth at which meteoric water is in chemical equilibrium with minerals (Riebe et al., 2017). The CZ thus includes the soil, rock, sediment, water, air, organic matter and organisms through the full depth of actively forming soils and extends above to the top of vegetation and below into materials hosting flowing, reactive groundwater. Study of CZ processes requires knowledge of the composition and physical arrangement (structure) of the surficial geologic materials. Furthermore, the structure of the CZ depends on a history stretching back well before the modern anthropogenic land-use changes. Therefore, both ongoing CZ processes and the response of the CZ to future external forcing remain sensitive to the longer history that has shaped the CZ. The Midwest US contains a CZ where current processes are strongly impacted by profound anthropogenic changes, but are ultimately controlled by the events of past global glacial-interglacial climate cycles.
The Intensively Managed Landscapes Critical Zone Observatory (IML-CZO) with field laboratories at Clear Creek Watershed (CCW) in eastern Iowa, Upper Sangamon River Basin (USRB) in central Illinois, and the Minnesota River Valley (MRV) in southern Minnesota is dedicated to studying the CZ processes of land under row crop agriculture. We focus on the interactions in the CZ that control the rates and trajectories of processes responsible for transforming and transporting water, nutrients, sediment, and energy over timescales ranging from minutes to a few million years. Continuous deposits of glacial and glaciofluvial sediment comprise ~20% of the land surface of the continental United States, including the glaciated portions of the Central Lowland of the Interior Plains (Fenneman and Johnson, 1946; Soller et al., 2009; Figure 1). The IML-CZO is characterized by successive periods of rapid sediment aggradation and erosion associated with continental glaciations punctuated by long (tens to hundreds of thousands of years) interglacial or post-glacial times of surficial weathering and soil development, a fundamentally different landsystem than that of the steady state or erosional topographic conditions of the other eight observatories in the NSF-supported national CZO network (Figure 1). A fundamental challenge for researchers working in the IML-CZO field laboratories has been to understand how the CZ evolved through multiple glacial/interglacial cycles in this region of overall net deposition, and how these landscapes are controlled by past geologic processes. This inheritance of past geologic events in glaciated landscapes of the mid-continent is known as the “glacial legacy,” which still today influences the CZ processes and trajectory of landscape and CZ evolution under intensively managed land-use practices (Phillips and Desloges, 2015).
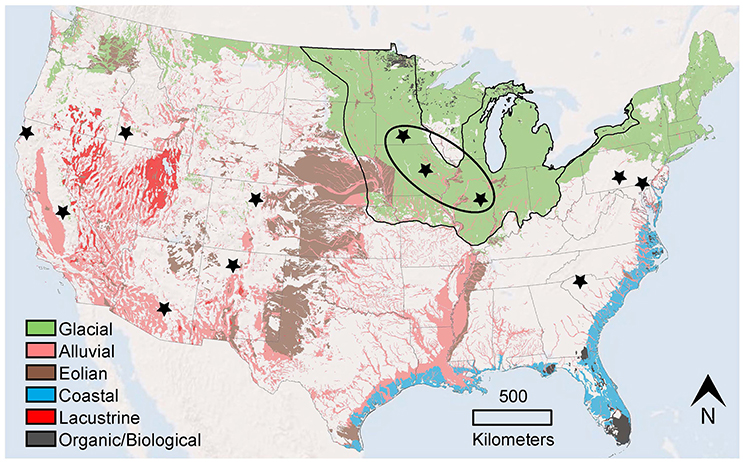
Figure 1. Generalized map showing surficial materials (Source of data: U.S Geological Survey, Soller et al., 2009) and the locations of CZO field observatories within the conterminous United States. The glaciated portion of the Central Lowland of the Interior Plains (Source of data: U.S Geological Survey, Fenneman and Johnson, 1946) is indicated by the narrow black line. The US CZO network is indicated by the black stars with the exception of Loquillo, Puerto Rico, which is not within the map area. The bold black oval surrounds the three IML-CZO field sites. Colored areas denote continuous cover of unconsolidated sediment. Green “Glacial” areas are underlain by glacial till, glaciofluvial, glaciolacustrine, and ice contact deposits. Brown “Eolian” are areas where loess or dune sand is at least 20 feet (6 m) thick at the surface. Blue “Coastal” areas contain both fine-grained sediments characteristic of lagoons and estuaries and coarser beach or shoreline deposits. The red “Lacustrine” areas are underlain by lake and playa deposits. Salmon-colored “Alluvial” areas are underlain by alluvial and colluvial deposits along major river valleys and inland basins. The dark gray areas “Organic/Biological” include recent organic sediments (peat) and biological sediments (marl and shelly materials). The white areas include bedrock or residuum developed from weathering of the underlying bedrock.
In an effort to understand the CZ of this region, we confront the fact that the primary CZ conceptual model—progressive weathering of local bedrock moving through a weathering profile in areas of net surface erosion or steady-state topography—, the feed-through reactor of Anderson et al. (2007), has serious limitations in the IML-CZO where thick, continuous glacial sediments blanket the landscape, and in other areas where alluvial, lacustrine, coastal, or eolian sediments predominate. In the feed-through reactor model, as the weathering front moves downward through the subsurface, rock fragments move vertically upward undergoing a progression of chemical and physical weathering reactions. As a result, the CZ weathering record is preserved locally within the soil and weathering profiles. Applying this model across landscapes and regions allows the geological principle of substituting space for time to model the evolution of the CZ in different lithologies under varying environmental conditions. However, in regions with thick glacial deposits, the physical and geochemical properties of geologic materials in the CZ often have little to no affinity with the local bedrock. Here the materials did not form in situ from the local bedrock, but instead are a mix of far-traveled and local sediment, episodically deposited, that underwent varying degrees of weathering prior to and along their transport pathways. Additionally, the significant differences between glacial and interglacial climates, and their associated geomorphological, hydrological, and ecological systems, provide a temporal variability in CZ processes that is of unmistakable importance. Our detailed examination motivates us to develop a new conceptual model of CZ evolution in the IML-CZO and the glaciated portions of the Central Lowlands of the Interior Plains that encapsulates two important elements. First, the geologic materials may have diverse origins and distinctive properties acquired during transport and subsequent deposition that do not reflect the composition of the underlying bedrock. Second, the history of change, whether it be in climate, geomorphic processes, or by human-induced modifications is significant and crucial for modeling critical zone structure and processes.
We begin by presenting a series of generalized cross-sections for the three field laboratories of the IML-CZO showing the heterogeneity and distribution of geologic materials in the glaciated central USA. Note that these cross-sections show specific examples of geomorphic settings typical of this CZO and of the Central Lowlands of the Interior Plain more broadly. These cross-sections motivate an idealization of the evolving structure of the CZ over the course of a single glacial cycle. This evolution highlights the key elements that need representation in a conceptual model of the CZ for landscapes characterized by net deposition of glacial sediments (e.g., Kołodynska-Gawrysiak et al., 2017), specifically, the diverse histories of the geologic materials and the dynamic evolution of the CZ through time. Finally we discuss our conceptual model for the CZ of the Central Lowlands of the Interior Plains and assess the applicability of the key elements of this model to CZs more broadly.
Glacial History and CZ Structure of IML-CZO Field Areas
In order to consider the glacial legacy in the IML-CZO, it is necessary to consider the important differences in the history of Quaternary glacial-interglacial cycles at each field laboratory. For example, glaciers last covered CCW almost 500,000 years ago whereas the most recent advance of the Laurentide Ice Sheet in the MRV ended ~15,000 years ago. The three IML-CZO field observatories encompass the range of glacial histories in the Central Lowlands (Figure 2). During the past 2.6 million years, cycles of physical and chemical weathering, soil formation, landscape development, and ecosystem change in the CZ were by driven by successive glacial advances that reshaped the landscape, altered the surface and subsurface hydrology, and buried or excavated all or part of ancient CZs while depositing “fresh” glacial deposits. The record of landscape development in the stratigraphic record (Willman and Frye, 1970; Hansel and Johnson, 1996; Curry et al., 2011), established the initial geological framework for understanding the subsequent CZ evolution.
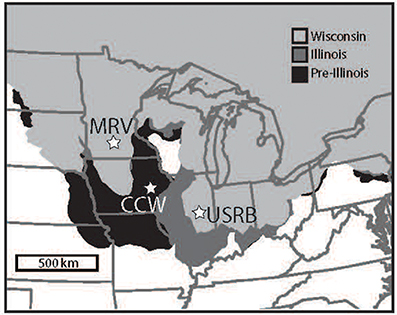
Figure 2. Extent of Midwest USA glaciations based on Fullerton et al. (2003). Source of data U.S. Geological Survey. Wisconsin Episode includes Marine Isotope Stages (MIS) 2-4. Illinois Episode correlates with MIS 6. Pre-Illinois Episode includes multiple episodes stretching to the earliest Pleistocene. IML-CZO sites shown as stars include the Minnesota River Valley (MRV), Clear Creek (CC), and the Upper Sangamon River Basin (USRB).
We illustrate the composition and distribution of Quaternary successions in the IML-CZO using a series of cross sections at a range of scales from the three field laboratories. The major critical zone events in the three areas are summarized in Table 1. The CCW was last glaciated during the early Quaternary (pre-Illinois Episode) and the USRB and MRV were last glaciated during the late Quaternary (Wisconsin Episode). The glacial successions are dominated by stacked, fine-grained till deposited during multiple glaciations. These very poorly sorted sediments (matrix-supported fine-grained diamictons) are intercalated or interspersed with deposits of well-sorted, coarse- to fine-grained glaciofluvial and glaciolacustrine sediments that often infill valleys, channels and low areas on the bedrock surface or on surfaces incised in older glacial deposits. Variably thick loess mantles the morainal uplands and outwash plains across landscapes both within and beyond late Wisconsin Episode ice margins. The physical, chemical, and hydrologic properties of the glacial sediments vary due to differences in lithology at the source area, subsequent reworking during transport, and post-depositional alterations that have controlled types and rates of CZ change.
Clear Creek Watershed, Iowa
Glacial landforms from the pre-Illinois Episode glaciation are not preserved, although there are scattered, loess-mantled tabular divides underlain by thick, clayey paleosols, which are likely remains of the latest pre-Illinois Episode “drift” plain (Ruhe, 1969). The bedrock surface in the CCW is crosscut by a palimpsest set of channel segments, up to 60 m deep, which formed during the Tertiary and Early Quaternary. Thick, continuous deposits of sand and gravel do not infill the bedrock channels. The glacial succession generally thins from about 100 to 125m in the headwaters area to 15–30m in the lower part of the watershed where Paleozoic carbonates are often exposed at the land surface. Locally, the thickest glacial successions are associated with the bedrock channels.
The sequence of pre-Illinois Episode (~2.6–0.5 Ma) glacial deposits includes seven different till sheets, each with an average thickness of 15–20 m, represented by two formations that are differentiated by clay mineral content (Hallberg, 1986; Bettis et al., 2010) (Figure 3). The two lowermost tills, both in the Alburnett Formation, have reversed magnetic polarity (older than 0.78 Ma) and are enriched in kaolinite in the clay fraction. Alburnett Formation tills contain significant amounts of eroded material derived from Tertiary weathering profiles (including kaolinite) eroded from sources north and northwest of CCW during the earliest advances of the Laurentide Ice Sheet (Roy et al., 2004). In addition, the oldest till forms a discontinuous layer of “stony” material on the bedrock surface and contains and abundance of local bedrock clasts (Figure 3). Tills of the overlying Wolf Creek Formation have normal polarity and are enriched in expandable clay minerals. Other than the lowest till, all the tills are similar in their physical properties and have a shared northern to northwestern provenance (Roy et al., 2007). In some places, tills in both formations are buried by deposits of sand and gravel (outwash), or have been completely eroded by meltwater. Following each glacial advance, CZ evolution in the tills produced color changes (primarily reflecting changes in the oxidation state of iron), leaching of primary carbonate minerals, mineral weathering, fracturing, and pedogenically-developed concretions and cementation. During the next glacial advance, erosion by ice or water usually removed all or part of the upland CZ while burying and preserving the CZ formed in valley positions.
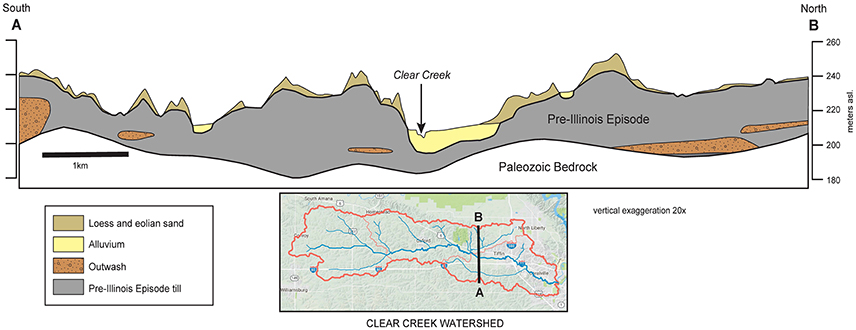
Figure 3. Cross-section of the eastern portion of the Clear Creek Watershed, Iowa. (A,B) Inset map shows the location of the cross-section within the Clear Creek Watershed, outlined in red. Pre-Illinois Episode tills of the Alburnett and Wolf Creek formations occupy broad bedrock valleys cut into Devonian bedrock. Sand and gravel bodies, interpreted as glacial outwash, are present within and between these till units. At the surface, thick deposits of loess dating to the late Wisconsin—earliest Holocene are present along with alluvium deposited in modern river valleys.
The modern landscape the CCW formed as the drainage network expanded and slopes were reshaped during the last two glacial-interglacial cycles occurring over the past half-million years. Although the CCW remained unglaciated during this time period, periglacial processes and distal glaciofluvial processes significantly affected development of the (1) regional drainage system, (2) local topography, and (3) CZ processes. As surface drainage networks, hillslopes, and the shallow groundwater system developed, oxygenated water and organic matter were delivered to the CZ and translocated downward into the unaltered glacial till and deeper part of the glacial succession.
Drainage divides, interfluves, drainage lines, and most hillslope elements on the modern landscape, as well as well-expressed soils and associated deep weathering profiles were in place at the close of the last interglacial (Sangamon Episode). The most recent (late Wisconsin Episode) glaciation was unique in the US Midwest because of the unprecedented magnitude and areal dispersal of loess (Bettis et al., 2003; Mason et al., 2007). Beyond the late-glacial ice margin, loess composed of carbonate-rich silt-loam accumulated in the developing boreal forest and parkland between 22 and 12 ka, which buried the glaciated landscape and associated CZ. In the CCW 3–7 m of loess mantles the uplands and old glacial terraces, completely burying the ancient CZ and its associated clayey paleosols and weathering profiles (Figure 3). This burial altered the chemistry of the pre-existing weathering profile and soils through organic matter degradation, additions of calcite and other easily weathered minerals and redox-related changes in iron species. The modern CZ in CCW extends through former CZs that strongly influence the structure and processes operating in the current CZ. For example, the clayey paleosol of the pre-loess CZ acts as an aquitard that “perches” the water table on uplands and focuses subsurface flow laterally as the overlying loess thins downslope. The subsurface distribution of the paleosol and its outcrop pattern on the landscape strongly control shallow groundwater flow and the distribution of seasonally saturated intervals in the shallow subsurface that impact weathering and nutrient loss.
Upper Sangamon River Basin, Illinois
The Upper Sangamon River Basin (USRB) was glaciated multiple times during the Pleistocene, including during the most recent (Wisconsin Episode) glaciation (Figure 2). The thickness of unconsolidated deposits, above Paleozoic bedrock, ranges from about 50 to 150 m (Soller et al., 1999; Stumpf and Dey, 2012). Much of the USRB overlies the buried preglacial Mahomet Bedrock Valley (MBV), a prominent feature on the bedrock surface (Horberg, 1945; Kempton et al., 1991). The MBV is part of the Teays-Mahomet Bedrock Valley system that stretches from West Virginia to Illinois (Melhorn and Klempton, 1991) and contained an active river system during the Pliocene and early Pleistocene. Underlying much of the central and southern USRB, the buried MBV ranges from 8 to 32 km wide and is filled with up to 50 m of sediment (Figures 4, 5A), mainly sand to gravelly sand. The coarse-grained fill in this bedrock valley, deposited by glacial meltwaters during the pre-Illinois and Illinois Episodes (Figure 5), contains the regionally important Mahomet Aquifer (Herzog et al., 1995; Roadcap et al., 2011). During the pre-Illinois Episode in the USRB (~1.2–0.4 Ma), glaciofluvial sediment (or outwash) was repeatedly deposited and excavated from the MBV. Such outwash mainly filled the preglacial bedrock valley and is overlain by till sequences with some glaciolacustrine sediment (Figure 5C; Kempton et al., 1991; Stumpf and Atkinson, 2015). Three or more pre-Illinois Episode till sequences, together up to 30 m thick, occur in areas outside the MBV or overlie the pre-Illinois Episode glaciofluvial deposits (Kempton et al., 1991; Stumpf and Atkinson, 2015).
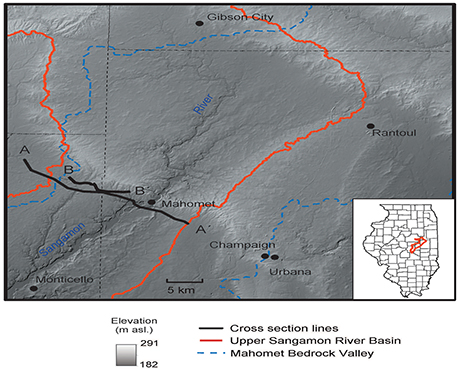
Figure 4. Digital elevation map of a portion of east-central Illinois, showing the locations of cross sections (A–A′) and (B–B′) in the USRB with respect to the buried Mahomet Valley. Surface expression of constructional glacial topography (moraines) is dominant. The moraines were formed between about 24 and 21 ka, as a fluctuating glacial ice margin receded to the northeast (Caron and Curry, 2016; Grimley, 2016a). Late-glacial and postglacial river valleys (e.g., Sangamon Valley) are dissected into the glacial topography and have fills of outwash and alluvium.
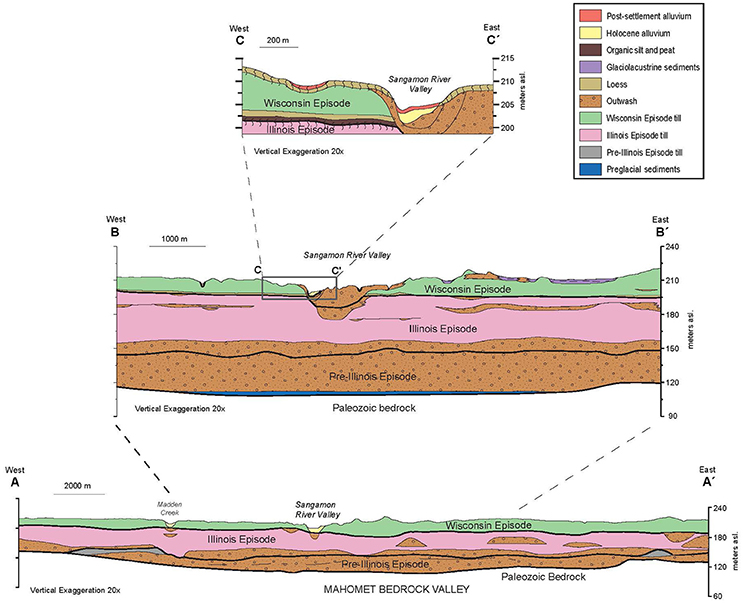
Figure 5. Structure of the CZ in in the USRB. (A,A′) Geologic cross section across the MBV showing stacked, laterally extensive till units from multiple glacial events with less extensive, isolated coarse-grained unites in the subsurface and in modern valleys. Modified from Stumpf and Atkinson (2015). © 2015 University of Illinois Board of Trustees. Used by permission of the Illinois State Geological Survey. (B,B′) Geologic cross section over the western half of the MBV showing divisions with tills from multiple events, two generations of coarse-grained deposits stacked in the Sangamon River Valley and glacio-lacustrine deposits in the near surface at the eastern end of the cross-section. Modified from Grimley et al. (2016b). © 2016 University of Illinois Board of Trustees. Used by permission of the Illinois State Geological Survey. (C,C′) Geologic cross section crossing the Sangamon River Valley showing loess draping the current land surface and a portion of an ancient CZ from a previous interglacial preserved beneath Wisconsin Episode till, loess, and peat. Post-settlement alluvium is present within depressions on the upland and above Holocene alluvium. The dominant depositional processes throughout the Pleistocene have been associated with the glaciations. Yet late-glacial and postglacial processes causing river dissection and sedimentation in river valleys have also impacted the structure of the CZ.
Following the pre-Illinois Episode glaciations, a long period of weathering, soil formation and landscape denudation occurred during the Yarmouth interglacial episode (~0.4–0.2 Ma) (Grimley et al., 2003; Curry et al., 2011). A paleosol is found in some areas of the USRB, but was typically eroded by the initial glacial advance of the Illinois Episode. During the late Illinois Episode (~0.16–0.13 Ma), glacial ice advanced out of the Lake Michigan Basin across the USRB to southernmost Illinois and westward to eastern Iowa (Figure 2). In Illinois, multiple sequences of glacial deposition, related to ice margin fluctuations, are found statewide (Willman and Frye, 1970; Hansel and McKay, 2010). In the USRB region, multiple depositional sequences contain dense loamy till interstratified with glacial outwash and finer-grained glaciolacustrine sediments (Figure 5B). Such sediment packages represent one or more glacial advance-retreat sequences (Kempton et al., 1991; Stumpf and Atkinson, 2015; Grimley et al., 2016b). Proglacial outwash below Illinois Episode till, in the MBV, is hydrologically connected with older pre-Illinois Episode outwash (Figures 5A,B, 6) and together comprise the Mahomet Aquifer. During the final glacial retreat of the Illinois Episode, some glacial sequences were incised by meltwaters and created drainageways that were in places reoccupied during the Wisconsin Episode or postglacial period. For example, outwash underlying the present-day Sangamon River Valley (Figure 5B) may represent a proto-Sangamon River that formed during a deglacial phase of Illinois Episode glaciation (Grimley et al., 2016b). After the Illinois Episode and before the Wisconsin Episode glaciation, a interglacial paleosol formed during the Sangamon Episode (~0.13–0.05 Ma). The weathering profile of this ancient CZ was partially or completely eroded during the last (Wisconsin Episode) glaciation across most of the USRB, but is preserved in some area in the subsurface (Figure 5C).
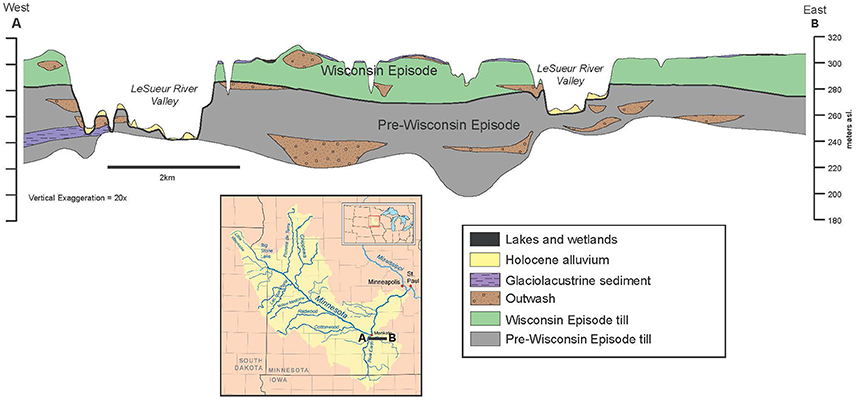
Figure 6. Simplified geologic cross-section through a typical portion of the Minnesota River basin, modified from the central/eastern portion of cross-section B–B′ on plate 4 of the Digital Geologic Atlas of Blue Earth County (Runkel et al., 2011). Used by permission of the Minnesota Geological Survey. Minnesota Pre-Wisconsin tills are likely pre-Illinois in age. The surficial geology features abundant glacial lake deposits with modern lake and wetland deposits also present. Knickpoint retreat up the LeSueur River has created multiple terrace surfaces, shown as Holocene Alluvium. Isolated bodies of sorted sand and gravel are present in the subsurface within both Wisconsin Episode and pre-Wisconsin Episode tills.
Following the last interglacial, the USRB was covered by glacial ice of the Lake Michigan Lobe of the Laurentide Ice Sheet during the last glacial maximum (Wisconsin Episode), ~25–20 ka (Grimley, 2016a). This region includes several morainic systems (Figure 4) that mark multiple ice margin positions during the Wisconsin Episode glaciation (Hansel and Johnson, 1996; Curry et al., 2011). At least two till units were deposited as a result of ice margin fluctuations (Wickham, 1979; Grimley et al., 2016b), with peat and proglacial loess preserved in places below the oldest till (Figure 6C). Glaciofluvial sediment (gravelly sand to fine sand) were deposited in coalescing fans along moraine fronts and in meltwater streams that drained the ice margin. Some of these streams appear to have reoccupied older drainageways or lowlands that existed during the penultimate glaciation. Large proglacial lakes in the USRB during Wisconsin Episode deglaciation were not common, as advancing glaciers flowed down the regional topographic gradient and the ice margin was fairly well-drained by large streams leading to the southwest. However, in the present-day landscape, many small depressions remain poorly drained and stream networks are poorly integrated (although modified by agricultural ditching and drainage tiling) because of drainage disruptions and landscape flattening that resulted from multiple successive glaciations. Immediately following ice retreat, thin deposits of fluvial and lake sediment accumulated in lowlands within the low-relief landscape of the USRB. Thin discontinuous layers of sand and gravel, too minor to show in typical cross-sections (e.g., Figure 5C), are commonly found immediately above Wisconsin Episode till deposits. Loess deposits, 1–2 m thick, accumulated above the till from ~22 to 16 ka (Nash et al., 2017) and effectively blanketed other glacial sediments. Periglacial climatic conditions for some centuries, immediately after ice margin retreat in the USRB, is evidenced by relict patterned ground and ice-wedge casts (Johnson, 1990). Such periglacial conditions may have led to some re-mobilization of loess and resedimentation into depressional and low-lying areas. The relict periglacial features, along with other aspects of the glacial legacy, today influence the diversity of soil types, soil moisture conditions, and shallow groundwater flow.
The history of Quaternary glacial-interglacial cycles significantly impacts the landscapes and soils found today in the USRB. The CZ here is developed in loess and glacial sediments that accumulated on an older interglacial landscape containing the Sangamon Geosol. Vestiges of this older landscape, including the paleosol and weathered lacustrine or alluvial sediments, are exposed in places along the Sangamon River Valley. Elsewhere, this older landscape is completely buried by deposits of the Wisconsin Episode glaciation and post-glacial streams. In places, late-glacial erosion has incised through modern alluvium into shallowly buried outwash. Additionally, anthropogenic excavations of outwash and alluvium in the Sangamon River Valley for aggregate has penetrated more deeply, perhaps into Illinois Episode outwash. The superposition or connectivity of permeable sedimentary units can provide a hydrologic connection between the surface and groundwater systems and a pathway for cycling nutrients and dissolved ions.
Soil development into loess and underlying glacial sediments has occurred on uplands during the last ca. 16 ka. Over this time period, soil development has occurred under a succession of vegetation including tundra, boreal and deciduous woodland, prairie, and cultivated landscapes. Today, the dominant soil type in the USRB are Mollisols (Mount, 1982), reflecting the dominance of prairie vegetation over much of the landscape during the Holocene. Pre-settlement woodlands were more typical within about 1 km of the Sangamon River valley (INHS, 2003), where Alfisols occur today. Approximately 2–5 m of fine-grained alluvial sediment are found in the valley, of which 0.5–0.9 m was deposited following European settlement in the nineteenth-century (Figure 5C; Grimley et al., 2017). Widespread devegetation and ditching of landscapes to increase agricultural land has resulted in an order of magnitude increase in alluvial sedimentation rates in the Sangamon Valley, averaging ~5 mm/yr during the last 150 years compared with ~0.7 mm/yr during pre-settlement times (Grimley et al., 2017).
Topographic controls on deposition and erosion are evident at several temporal and spatial scales. Over the course of the Pleistocene, the MBV was preferentially filled with sediment so that today's surface topography, dominated by last glacial constructional landforms, no longer reflects this feature (Figures 4, 5A, 6). Stretches of the current Sangamon River occupy a former glacial meltwater channel filled with last glacial outwash. In some areas, the near-surface Wisconsin Episode outwash may be immediately underlain by Illinois Episode (Figure 5B), indicating that glacial meltwater drainage was focused into the same area during two separate glacial events. The modern Sangamon River Valley contains a Holocene alluvial record that indicates increased rates of floodplain sedimentation following the establishment of intensive agriculture in the late 1800s (Grimley et al., 2017) (Figure 5C). At scales of tens to hundreds of meters, erosion of local topographic highs and sedimentation within swales is evident in soil profiles. Rates of erosion and deposition at this scale were also accelerated by land-use change (e.g., Norton, 1986; Kreznor et al., 1990). Even in low-relief landscapes such as in the USRB, subtle topographic gradients can significantly impact shallow groundwater flow and soil moisture conditions. Glacial moraines, along with finer-scale periglacial features and other undulations on the glacial till plain (Figure 4), impose trends in soil drainage, resulting in soil associations (Mount, 1982) tied, in part, to glacial landforms and sediments at the macro- and meso-scales.
Minnesota River Valley, Minnesota
In south-central Minnesota, Quaternary deposits are typically ~100 m thick (Figure 6). Flat-lying sedimentary Paleozoic bedrock beneath the Quaternary section is exposed in places along the Minnesota River valley and in the lower reaches of some major tributaries of the Minnesota River (Jennings et al., 2011). Valleys cut into the Paleozoic bedrock section are present, but smaller in scale than the Mahomet Bedrock Valley in the USRB.
The majority of Quaternary sediments are glacial in origin, derived from repeated advances of multiple lobes of the Laurentide Ice Sheet. These distinct lobes were fed by ice from different regions with different bedrock geology and four distinct provenances for glacial tills have been identified based on clast lithology: Riding Mountain (NW), Winnipeg (N), Rainy (NE), and Superior (ENE) (Johnson et al., 2016). In east-central Blue Earth County, the pre-Wisconsin Episode units include unnamed tills of Winnipeg, Superior, and Rainy Provenance (Meyer et al., 2011) (Figure 6). These tills are stratigraphically below the Browerville Formation, a widespread but discontinuous buried diamicton, that is also pre-Wisconsin Episode and likely pre-Illinois Episode in age (Johnson et al., 2016). Deposits of sorted sand and gravel, interpreted as meltwater deposits of these glaciations are present as discontinuous lenses, especially at the boundaries between till units (Meyer et al., 2011).
During the Wisconsin Episode glaciation, tills of Riding Mountain provenance and mixed Riding Mountain and Winnipeg provenance were deposited in the east-central Blue Earth County (Meyer et al., 2011). These tills comprise the New Ulm Formation, which is bounded below by an erosional unconformity where the advancing ice eroded earlier glacial deposits (Jennings et al., 2011). Till sheets with distinct textures and distributions of clast lithology are recognizable within the New Ulm Formation and were likely deposited by adjacent ice streams draining distinct areas within what is commonly referred to as the Des Moines Lobe (Lusardi et al., 2011). The uppermost portions of the New Ulm Formation include supraglacial tills formed by melting of stagnant ice during the retreat of the Des Moines Lobe (Jennings et al., 2011).
Glacial Lake Minnesota was a large proglacial lake that formed during the retreat of the Des Moines Lobe as water ponded behind the stagnant ice. Portions of Glacial Lake Minnesota were supraglacial and features such as ice walled lake plains are present where lake sedimentation was constrained by ice during deposition, leaving vaguely circular flat-topped hills of fine-grained sediment standing above subglacial tills (Jennings et al., 2011). Radiocarbon dates place Glacial Lake Minnesota at a maximum age of between 14.6 and 13.8 ka (Jennings et al., 2011). Glacial Lake Minnesota drained through a series of outlets with the youngest being to the north along the trend of a bedrock valley (Patterson and Hobbs, 1995). This same spillway was further incised by Glacial River Warren during the catastrophic drainage of Glacial Lake Agassiz.
Glacial Lake Agassiz, the largest proglacial lake in North America during the most recent deglaciation, formed as the Red River Lobe of the Laurentide Ice Sheet retreated north of the drainage divide separating the Mississippi basin from Hudson Bay. During its 5,000 year history, Glacial Lake Agassiz drained through four different outlets including the Minnesota and Mississippi drainages (Teller and Leverington, 2004). Drainage through the southern outlet is referred to as Glacial River Warren and this drainage carved a valley 45–70 m deep, now occupied by the Minnesota River, through pre-Wisconsin tills and Paleozoic saprolite, exposing relatively unweathered bedrock in places (Matsch, 1983). This southern outlet was occupied primarily early in the history of Lake Agassiz from ~13.4 to 10.9 ka (Matsch, 1983) with a short-lived reoccupation of the southern outlet ending by 9.4 ka (Fisher, 2003). The abrupt downcutting of Glacial River Warren left tributaries including the Blue Earth River and LeSueur River stranded above the trunk stream (Gran et al., 2009). Knickpoints formed in these tributaries and continue to cut back today. In the LeSueur River more than 400 terrace surfaces are present in the basin below the knickpoint which has migrated ~30 km upstream of the confluence with the Minnesota (Gran et al., 2009) (Figure 7). Within the lower LeSueur basin, bluff erosion and gully formation contribute to high turbidity levels and rapid sedimentation within Lake Peppin, a natural lake on the Mississippi River downstream of the junction with the Minnesota River (Gran et al., 2009, 2013).
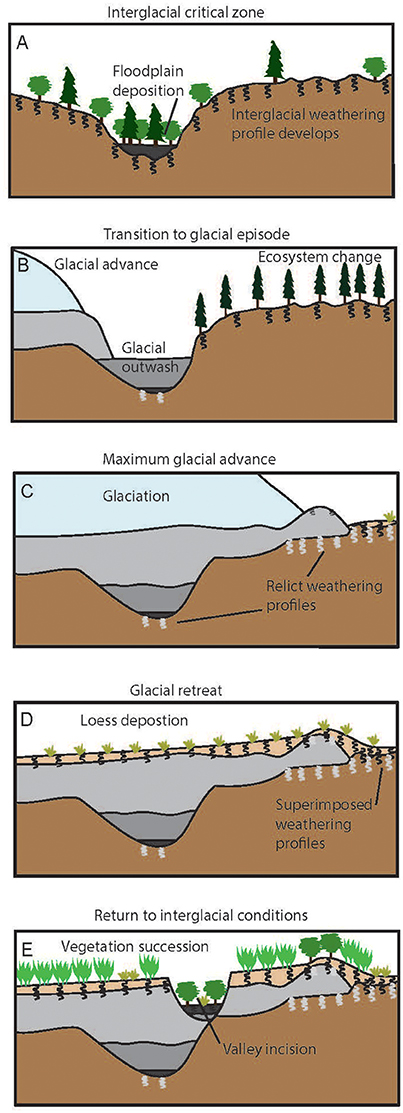
Figure 7. Cartoon of idealized CZ evolution through a glacial cycle. (A) The landscape developed prior to glaciation. A Weathering profile (black squiggles) is developed in the surficial sediments, including alluvium (black) in the major river valleys. (B) As glaciers (light blue) crossed the region, they actively eroded their beds depositing glacial till (light gray). Sorted fluvial or lacustrine sediments (dark gray) were deposited in front of the ice margin partially filling the bedrock valleys. Weathering profiles developed on former land surfaces (light gray squiggles) are preserved where glacial erosion did not entirely remove the older sediments. Vegetation beyond the glacial margin was typical of tundra and boreal forest found in northern latitudes today. (C) Glacial conditions (dry and cold) continue beyond the ice margin. Windblown sand and silt (loess), shown in tan, accumulated, covering soils developed in the bedrock and preglacial sediments. Minimal chemical and physical weathering occurred beneath the glacier. The weathering profile continued to develop in the landscape beyond the glacial margin. In places, soil formation is superimposed on previous weathering profiles. Sparse tundra vegetation likely colonized areas near the ice margin. (D) Following glacier retreat, loess further accumulated on the land surface, completely burying the glacial deposits. The vegetation and soil succession began as tundra was replaced by boreal forest, and later by mixed hardwood/coniferous forest. (E) As the climatic conditions moderated during the interglacial, tallgrass prairie, and oak-hickory savannas replaced the hardwood forests. Continuing fluvial activity established new drainage networks, and valleys were incised into loess and glacial deposits.
Because of its late deglaciation compared with the USRB and CCW, the MRV has very thin or no loess deposited at the surface. Instead, the most recent deposits other than river terraces include modern lake and wetland deposits (Jennings et al., 2011) (Figure 6). The shallow lakes and wetlands were largely drained to allow for agricultural use and drainage ditches now connect the low-relief uplands to the naturally steep and incised tributaries of the Minnesota River. In the Minnesota River Basin the legacy of glacial history is, therefore, felt in both the subsurface composition of the CZ and in the topography shaped by glacial processes that controls shallow subsurface water flow and the distribution of soils.
Idealized Evolution of the CZ Through Glacial Cycles
The CZ structure of each field laboratory in the IML-CZO clearly reflects variability in the climate, geomorphological, biological and geochemical systems that were dominant in the glacial-interglacial cycles. We generalize the histories revealed in the cross sections to produce an idealized model of interactions between the Laurentide Ice Sheet, landscapes of the Central Lowlands of the Interior Plain, and sedimentary processes during the most recent glacial-interglacial cycle. We assume, generally, that the sequence of events was generally similar during each of the Quaternary glacial episodes. One significant difference ais that the flow directions of earlier ice advances were likely controlled by topography on the bedrock surface until deeply incised valleys were filled in. In addition, the preglacial landscapes were covered with thick residuum or saprolite that was incorporated in glacially-transported sediment (Patterson and Boerboom, 1999). In many places, the oldest glacial till contains an abundance of local weathered material derived from preglacial soils and weathering profiles, and fragments of the local bedrock (Willman et al., 1966).
Prior to the last glaciation, the interglacial landscape had an integrated drainage network, variable relief, a hydrologically connected soil cover and associated interglacial weathering profile that was formed in fluvial, lacustrine, colluvial, and eolian deposits overlying a sequence of older sediments from previous glacial-interglacial cycles (Figure 7A). The vegetation cover at that time was likelysimilar to the pre-settlement vegetation in the region including oak-hickory forest, savannah, and prairie (Grüger, 1972; Curry et al., 2010). Soils were dominantly fertile Alfisols, and Mollisols, similar in composition to those occurring in the CZO today (Follmer, 1983).
As the Laurentide Ice Sheet flowed into the region, the CZ underwent dramatic changes over a relatively short period of geologic time (Figures 7B,C). The regional climate cooled during the onset of glaciation, which led to profound changes in the rates and trajectories of all CZ processes. At the time that the ice sheet margins advanced southward into the region, a parkland or boreal forest ecosystem had colonized the landscape (Garry et al., 1990; Curry and Baker, 2000).
As the ice sheet flowed across the region toward its terminal position, the glaciers actively eroded their beds in many places and compacted or deformed underlying materials to varying extent (Figure 7C). Subglacial tills and associated meltwater sediments were deposited. Partial or complete weathering profiles (paleosols) developed on former land surfaces were sometimes preserved beneath the ice, primarily in lower parts of former landscape where subglacial erosion was less effective. In front of the ice margin, sorted glaciofluvial and glaciolacustrine sediments accumulated, partially or completely filled pre-existing valleys. Locally, the glaciers hosted diverse microbial life both on the ice surface and beneath (Boetius et al., 2015). During glacial maxima a zone of permafrost extended beyond the ice margin in some areas (Garry et al., 1990) and tundra plants surrounded ice-walled lakes in former supraglacial environments (Curry and Petras, 2011). Cold climate soils (Gelisols) and tundra vegetation occupied landscapes dominated by intensive solifluction, irregular, and fluctuating stream flows and localized eolian deposition (Johnson, 1990; Schwert et al., 1997; Mason, 2015). Beyond the permafrost zone, or in more southern parts of the Central Lowlands, boreal forest and associated cool climate soils (Cryalfs and Spodosols) occupied the landscape.
Critical zone processes were radically different under full glacial conditions than they had been during the interglacial. Beneath the glacier erosion and deposition significantly modified the CZ structure and added new sediments. The biogeochemical system was altered and shifted from a relatively warm, oxygen-rich environment to an oxygen deficient, cool, and biologically limiting condition (Anderson et al., 1997; Skidmore et al., 2000; Boetius et al., 2015). In the tundra zone beyond the ice margin, similarly cool and oxygen deficient conditions dominated all but the uppermost part of the CZ. In the annually thawed part of the CZ (the active zone), components of past CZs were reworked and transformed by mass wasting, eolian and fluvial activity, and enriched in organic matter as a result of significant reduction in the rate of carbon decomposition, although organic carbon inputs were low relative to warmer periods. The CZ was also significantly impacted in the boreal forest zone where dry and cool conditions prevailed, fostering intense seasonal eolian deposition. Deflation of sand and silt from outwash deposits up-wind resulted in the accumulation of wind-blown sand and silt (loess). In the CZ, this material was aggraded at varying rates through time, depending upon the distance from the source and multi-centennial fluctuations in climate and/or glacial sediment production (Bettis et al., 2003; Muhs and Bettis, 2003; Nash et al., 2017). CZ processes in this zone were very different than in the periglacial zone and beneath the ice sheet. Here a warmer (but still cool), oxygenated, biologically enriched environment having internal (vertical) connectivity inherited from the interglacial period drove active chemical weathering and maintained the connection between the active weathering zone and deeper parts of the CZ.
Termination of the glacial period, including a shift to warmer climatic conditions, occurred over a much shorter time period than the glacial advance phase (Lisiecki and Raymo, 2005). Glacial retreat was not a continuous process, and often the stagnant ice margins readvanced, overriding previously deglaciated terrain (Curry et al., 2011). A series of recessional moraines were formed during these readvances. In the decades and centuries immediately following deglaciation, the dominant geomorphic processes shifted from an active or stagnant ice system to paraglacial, lacustrine, eolian, and fluvial processes. Over millennia, loess blanketed the uplands downwind of the major valleys (Figure 7D). As the climate moderated and glacial sedimentation ceased, vegetation began to recolonize recently glaciated areasand ecosystemsevolved from tundra (Figure 7D) to boreal forest, and finally to hardwood forest (e.g., King, 1981; Davis and Shaw, 2001; Curry et al., 2010). These changes in vegetation type were accompanied by an evolution of soils from Gelisols and Cryalfs to Alfisols and Spodosols characteristic of the boreal forest, and later to Alfisols under mixed hardwood/coniferous forest vegetation (Figures 7D,E).
As the climate warmed further during the interglacial, tallgrass prairie, and oak-hickory savannas replaced the hardwood forests (Figure 7E). As vegetation changed through the period in response to variations in climate and fire regime, soils evolved from the Alfisols of the early postglacial period to Mollisols and transitional soils (Mollic Hapludalfs) in the prairie and savanna (Bartlein et al., 1984; Baker et al., 1992; Nelson et al., 2006). The postglacial fluvial system continued to develop from initial headward extension, through to meander belt development and episodes of alluvial deposition punctuated by channel incision (Knox, 1972; Bettis et al., 2008).
Transformation of ecosystems and climatic conditions during the glacial-interglacial cycle caused long-term shifts in water flux, chemical weathering, and CZ evolution. This idealized progression of CZ evolution illustrates the dynamic nature of the subsurface, and highlights its direct relationship with geomorphic processes, and the complexity of the geologic materials.
Evolution of the Modern CZ
We have illustrated three examples of the structure of the CZ within the formerly glaciated Midwest US (e.g., Figures 3, 5, 6) and shown an idealized evolution of the CZ through a glacial cycle (Figure 7). We argue that the structure of the CZ plays an important role in the current state and future evolution of the CZ within the glaciated portion of the Central Lowlands, now under intensive human management. Management practices vary geographically to accommodate soil and drainage differences associated with glacial, periglacial, and fluvial landforms. Thus, the history of environmental change influences CZ structure in terms of the distribution of materials in the subsurface and the surface topography. Both topography and subsurface sedimentary structure influence ongoing CZ processes and the response of the CZ to anthropogenic forcing. Perhaps most important is the recognition that different areas of the Central Lowlands have different preglacial, glacial, and interglacial histories, creating different CZ structure and influencing future evolution.
Modern soil profiles in the glaciated parts of the Central Lowlands represent the transition between the above and below ground parts of the CZ. Their properties are the cumulative effects of additions, removals, transfers and transformations of energy, mineral, and biological materials in an evolving landscape over the past 15 ka (late glacial and Holocene). These soil profiles (and their horizonation) evolved over changing climate and vegetation systems, but resulted in mainly Alfisol or Mollisol profiles in upland areas of the study area (Mount, 1982; Ruhe, 1984; Baker et al., 1992). In Illinois and Iowa, the base of the A horizon (organic-rich topsoil) occurs at ~0.1–0.5 m depth and the base of the B horizon (pedogenically altered subsoil) at ~1–1.5 m depth. The depth to the base of the C horizon (oxidized, slightly altered) depends on the sub-regional geologic history, and typically occurs at ~3–5 m depth in parts of the region glaciated during the Wisconsin Episode it (Mount, 1982; Ruhe, 1984; Tandarich et al., 1994). In such areas, matrix carbonates (calcite, dolomite) have been leached to 2–3.5 m below the surface. Below the C horizon is a D horizon that consists of essentially unaltered, unoxidized (containing minor pyrite) and calcareous glacial till or other glacial sediment that has generally remained below the long-term water table (Tandarich et al., 1994). Beyond the Wisconsin Episode glacial margin, where the modern soil is formed in thicker loess, the oxidized C horizon is thicker and carbonate leaching ranges from 1.5 to >8m along a west-east precipitation gradient (Ruhe, 1984). In addition to climatic effects, soil profile thicknesses, leaching depths, and oxidation depths also vary on a more local scale with soil drainage, vegetation type, parent material, and soil age (Birkeland and, 1999).
Landscape relief is one way in which history and CZ structure influence ongoing processes and the trajectories for future evolution. Pre-agricultural landscape relief and drainage were strong controls on the pace of agricultural development (e.g., Bogue, 1963) and influenced both land management and system responses to intensive agriculture. This pre-agricultural landscape relief is itself a strong function of the local glacial history. Mature fluvial networks were present in CCW prior to agricultural development because deposition during recent glaciations was limited to loess that blanketed the pre-existing landscape and did not rearrange drainage patterns. These mature fluvial networks create landscape relief that provides energy for sediment transport down hillslopes and, ultimately in to stream networks (Abban et al., 2016). In contrast, fluvial networks in the USRB prior to intensive agriculture, were limited in extent and did not drain large areas of the upland surface (Rhoads et al., 2016). The limited landscape relief in the USRB likely contributes to lower rates of post-settlement alluvial deposition than in CCW (Grimley et al., 2017) and a fluvial suspended load dominated by near-channel sources rather than agricultural fields (Neal and Anders, 2015). In the MRV, the legacy of drainage of Lake Agassiz strongly controls landscape relief and ongoing erosion and sediment transport that is focused near the entrenched Minnesota River and its tributaries (Gran et al., 2013).
The impact of glacial history and CZ structure on modern CZ processes stretches beyond the topographic influences of landscape relief. For example, differential permeability in the materials within the CZ creates pathways for preferential flow of groundwater in the near surface and in deep aquifers (Atkinson et al., 2014), controlling spatial variability in the flux of reactive water through the CZ. The distribution of sorted sand and gravel and clayey paleosols in the subsurface is thus crucial for predicting subsurface reactions. Sorted sand and gravel deposits occur in a variety of contexts in the IML-CZO. Stacked units of sorted sediment of different ages are present in the USRB at depth within Mahomet Bedrock Valley (pre-glacial alluvium and pre-Illinois Episode outwash Figure 5B) and on the surface (modern alluvium, Wisconsin Episode and Illinois Episode outwash Figure 5C). In CCW, there is also juxtaposition of sorted sediment of different ages (pre-Illinois Episode outwash and Holocene alluvium Figure 3). Isolated and/or thin bodies of sand and gravel also occur within the glacial units at all IML-CZO sites. The geometry of these deposits and their connectedness is vital for predicting the interactions between surface and subsurface water in the CZ (Stumpf and Dey, 2012; Atkinson et al., 2014). The importance of coarse sediments in the subsurface extends past their role in influencing subsurface weathering and groundwater flow: the water stored within these sediment is a resource. Groundwater within the Mahomet Aquifer is a valuable resource for humans, providing high-quality drinking water for much of east-central Illinois, including the Champaign-Urbana area, and was designated a Sole Source Aquifer (United States Environmental Protection Agency Notice, 2015). In much of the USRB, the aquifer is protected by multiple overlying fine-grained till units from various glaciations; however, a groundwater age of <11,000 years from isotopic data (Hackley et al., 2010) suggests a hydrologic connection with surface recharge over millennial time scales. Another central Illinois city in the USRB (Decatur, IL) relies on surface water from a reservoir for its drinking water and is thus vulnerable to water quality issues resulting from high nutrient levels in the Sangamon River (Keefer et al., 2010).
As we continue exploring the CZ at the IML-CZO we anticipate finding more examples illustrating how the geologic structure and history of CZ evolution constrain the present function and future evolution of the CZ. The importance of CZ structure to understanding CZ process in the IML-CZO further motivates us to present a new conceptual framework for understanding CZ development in the glaciated Midwest.
The Patchwork CZ: New Metaphor for the IML-CZO and Glaciated Central Lowlands CZ
The CZ of the IML-CZO and the CZs of other depositional landscapes (Figure 1) are not well-described by the feed-through reactor model of the CZ (Anderson et al., 2007). We propose a new metaphor for the CZ of the glaciated portion of the Central Lowlands of the Interior Plain based on the human social systems of this region. Beginning at least 13,000 years ago (Lothrop et al., 2016) a diverse group of people with a variety of histories and origins began to arrive in waves. Acknowledging that the history of people in this region features conflict and colonization, we suggest that the present inhabitants were shaped by their individual and shared experiences of changing local, regional, national, and global human culture. The story of this culture must include both the diversity of its constituents and their ongoing evolution together. The patchwork quilt is a metaphor that has been used to describe American culture (e.g., Chametzky, 1989) which we propose as an image that captures the essential features of the IML-CZO study sites and, more broadly, the CZ of areas that experienced net deposition through cycles of continental glaciation. While the regular division of agricultural fields in the region along evenly spaced section lines easily brings to mind geometrical patchwork quilt designs, the vertical structure of the CZ is more akin to a crazy quilt which features irregularly shaped pieces, exotic fabrics, and embroidery added on top of the fabric (Figure 8).
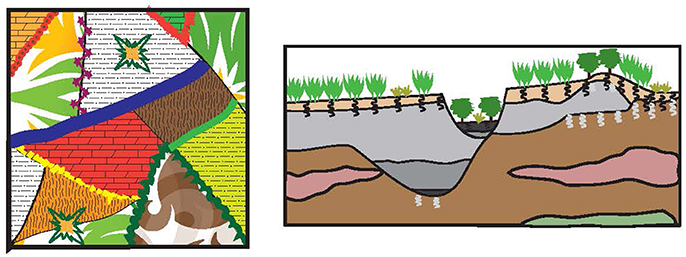
Figure 8. An illustration of the patchwork CZ conceptual model. The left panel is an example of a crazy quilt design. Irregularly shaped pieces of a range of different fabrics are stitched together and embellished with embroidery. This is similar to the CZ shown on the right in which a range of diverse materials are juxtaposed and weathering reactions alter some of their boundaries.
This image of the patchwork CZ emphasizes two key characteristics of the CZ of the IML-CZO sites: (1) the mineral components of the CZ are spatially variable and have been influenced by their individual histories of transport and weathering, and, (2) CZ processes vary enormously at timescales ranging from glacial cycles (105 years) to decades. As is evident from the cross sections presented (Figures 3, 5, 6), the CZs of the IML-CZO are characterized by spatial variability in the mineral and geologic components. The physical and chemical properties of the sediments in the CZ vary due to differences in their source areas, prior weathering histories, processes of erosion and deposition, and postglacial alterations. This multi-sequence evolution of CZ materials before, during and subsequent to transport, is a primary control on CZ structure in the IML-CZO and in other areas with accretionary sedimentary sequences, including areas where the CZ is formed in sedimentary bedrock. The CZ, like the soil profile in its upper part, is a historical body, one whose history must be appreciated to understand its present condition and function. The dramatic and quantifiable impacts of climate change, geomorphic processes, soil development, and vegetation on the CZ structure over the last few million years highlights the need to consider CZ process timescales in reference to variations of climate, vegetation, microbial, and geomorphic processes over those timescales. This point is important for critical zone science even beyond the glaciated Central Lowlands. Climate variability at timescales of centuries to several hundred thousand years is characteristic of the last few million years of Earth history, globally. This variability affected the chemical, physical and biological processes in the CZ across the planet. Similarly, while recent anthropogenic impacts on ecosystem services (Richardson and Kumar, 2017), hydrology, and climate are pervasive in heavily modified landscapes like the IML-CZO, more subtle changes in land use, climate and water are occurring in the CZ elsewhere. The imprint of anthropogenic changes on CZ structure and processes is occurring in tandem with the pre-settlement glacial legacy of the landscape (Kołodynska-Gawrysiak et al., 2017).
We hypothesize that the diversity of materials and variability in climate and ecosystems characteristic of the glaciated Central Lowland influence not only the structure of the CZ but also its current function and its trajectory of future evolution. Future work at the IML-CZO sites will explore the importance of the subsurface permeability contrasts in glacial materials and their geometry in driving current CZ process and the response of the CZ to changing agricultural practices and climate variability. Another target for future research is the importance of small-scale glacial and periglacial topography including hummocky moraines and meter-scale closed depressions in setting the rates of physical, chemical and biological CZ processes. Additionally, soils and weathering profiles provide an integrated record of CZ processes through time and we seek to develop CZ process models capable of hindcasting the observed spatial variability. Moreover, the influences of previous history and temporal variability also impact CZs beyond the areas glaciated by the Laurentide Ice Sheet. Thus, our new conceptual model of the CZ in the glaciated Central Lowlands as a patchwork quilt motivates future research in other regions with thick sedimentary deposits (e.g., Coastal Plain, Great Plains, large basins) for consideration of the mechanisms that shape CZ structure, timescales over which the structure is shaped, and potential for CZ structure to influence near-surface processes and respond to future environmental changes.
Author Contributions
All authors contributed to framing of the problem, discussion to create and revise the conceptual model, and the writing and revision of the manuscript and figures. EB led on Clear Creek Watershed and Figure 3 and made significant contributions to Figure 7 and sections Glacial History and CZ Structure of IML-CZO Field Areas and Evolution of the Modern CZ. DG and AS led on Upper Sangamon River Basin and contributed significantly to sections Glacial History and CZ Structure of IML-CZO Field Areas and Evolution of the Modern CZ. DG produced Figures 4, 5. AS provided holistic revision to the manuscript. PK contributed to the development of the conceptual model and conceived of Figure 7. AA led on sections Introduction, Minnesota River Valley, and The Patchwork CZ: New Metaphor for the IML-CZO and Glaciated Central Lowlands CZ and produced Figures 1, 2, 6–8.
Funding
This work was funded by NSF-EAR award #1331906 Critical Zone Observatory for Intensively Managed Landscapes (IML-CZO) to PK (PI), AA (co-PI), and EB (co-PI).
Conflict of Interest Statement
The authors declare that the research was conducted in the absence of any commercial or financial relationships that could be construed as a potential conflict of interest.
Acknowledgments
We thank Harvey Thorleifson and Richard Lively of the Minnesota Geological Survey for providing electronic files of published cross-sections of Blue Earth County, Minnesota as a starting point for our illustration, and Phil Kerr, Iowa Geological Survey for assistance with the CCW cross-section.
References
Abban, B., Papanicolaou, A. N., Cowles, M. K., Wilson, C. G., Abaci, O., Wacha, K., et al. (2016). An enhanced Bayesian fingerprinting framework for studying sediment source dynamics in intensively managed landscapes. Water Resour. Res. 52, 4646–4673. doi: 10.1002/2015WR018030
Anderson, S. P., Drever, J. I., and Humphrey, N. F. (1997). Chemical weathering in glacial environments. Geology 25, 399–402. doi: 10.1130/0091-7613(1997)025<0399:CWIGE>2.3.CO;2
Anderson, S. P., von Blanckenburg, F., and White, A. F. (2007). Physical and chemical controls on the critical zone. Elements 3, 315–319. doi: 10.2113/gselements.3.5.315
Atkinson, L. A., Ross, M., and Stumpf, A. J. (2014). Three-dimensional hydrofacies assemblages in ice-contact/proximal sediments forming a heterogeneous ‘hybrid’ hydrostratigraphic unit in central Illinois, USA. Hydrogeol. J. 22, 1605–1624. doi: 10.1007/s10040-014-1156-7
Baker, R. G., Maher, L. J., Chumbley, C. A., and Van Zant, K. L. (1992). Patterns of Holocene environmental change in the midwestern United States. Quat. Res. 37, 379–389. doi: 10.1016/0033-5894(92)90074-S
Bartlein, P. J., Webb, T. III., and Fleri, E. (1984). Holocene climatic change in the northern Midwest: pollen-derived estimates. Quat. Res. 22, 361–374. doi: 10.1016/0033-5894(84)90029-2
Bettis, E. A. III., Benn, D. W., and Hajic, E. R. (2008). Landscape evolution, alluvial architecture, environmental history, and the archaeological record of the Upper Mississippi River Valley. Geomorphology 101, 362–377. doi: 10.1016/j.geomorph.2008.05.030
Bettis, E. A. III., Muhs, D. R., Roberts, H. M., and Wintle, A. G. (2003). Last glacial loess in the conterminous USA. Quat. Sci. Rev. 22, 1907–1946. doi: 10.1016/S0277-3791(03)00169-0
Bettis, E. A. III., Tassier-Surine, S., and Quade, D. J. (2010). “Quaternary geology of the Iowa City Area,” in The Geology of Klein and Conklin Quarries, Johnson County, Iowa. Geological Society of Iowa Guidebook 87, eds T. Marshall and C. Fields (Iowa: Geological Society of Iowa), 143–159.
Boetius, A., Anesio, A. M., Deming, J. W., Mikucki, J. A., and Rapp, J. Z. (2015). Microbial ecology of the cryosphere: sea ice and glacial habitats. Nat. Rev. Microbiol. 13, 677–690. doi: 10.1038/nrmicro3522
Bogue, A. G. (1963). From Prairie to Corn Belt: Farming on the Illinois and Iowa Prairies in the Nineteenth Century. Chicago, IL: University of Chicago Press.
Brantley, S. L., Goldhaber, M. B., and Ragnarsdottir, K. V. (2007). Crossing disciplines and scales to understand the critical zone. Elements 3, 307–314. doi: 10.2113/gselements.3.5.307
Caron, O. J., and Curry, B. B. (2016). “The Quaternary Geology of the Southern Metropolitan Area in The Chicago Outlet, Morainic Systems, Glacial Chronology, and Kankakee Torrent,” in Illinois State Geological Survey Guidebook (Champaign, IL: Illinois State Geological Survey), 43, 3–27.
Chametzky, J. (1989). Beyond melting pots, cultural pluralism, ethnicity: or, déjà vu all over again. Melus 16, 3–17. doi: 10.2307/467098
Curry, B. B., and Baker, R. G. (2000). Palaeohydrology, vegetation, and climate since the late Illinois Episode (~130 ka) in south-central Illinois. Palaeogeogr. Palaeoclimatol. Palaeoecol. 155, 59–81. doi: 10.1016/S0031-0182(99)00094-2
Curry, B. B., Wang, H., Panno, S. V., and Keith Hackley, C. (2010).“Chapter 13: Quaternary paleoclimate,” in Geology of Illinois, eds D. R. Kolata and C. K. Nimz (Champaign, IL: Illinois State Geological Survey), 248–260.
Curry, B., and Petras, J. (2011). Chronological framework for the deglaciation of the Lake Michigan lobe of the Laurentide Ice Sheet from ice-walled lake deposits. J. Quat. Sci. 26, 402–410. doi: 10.1002/jqs.1466
Curry, B. B., Grimley, D. A., and McKay, E. D. III. (2011). “Quaternary glaciations in Illinois,” in Quaternary Glaciations - Extent and Chronology A Closer Look, eds J. Ehlers, P. L. Gibbard, and P. Hughes (Amsterdam: Elsevier), 467–487.
Davis, M. B., and Shaw, R. G. (2001). Range shifts and adaptive responses to Quaternary climate change. Science 292, 673–679. doi: 10.1126/science.292.5517.673
Fenneman, N. M., and Johnson, D. W. (1946). Physiographic Divisions of the Conterminous U.S. Reston, VA: USGS Special Map, USGS.
Fisher, T. G. (2003). Chronology of glacial Lake Agassiz meltwater routed to the Gulf of Mexico. Quat. Res. 59, 271–276. doi: 10.1016/S0033-5894(03)00011-5
Follmer, L. R. (1983). “Sangamon and Wisconsinan Pedogenesis in the Midwestern United States,” in Late Quaternary Environments of the United States, Vol 1, eds S. C. Porter, K. L. Pierce, and T. D. Hamilton (Minneapolis, MN: University of Minnesota Press), 138–144.
Fullerton, D. S., Bush, C. A., and Pennell, J. N. (2003). Map of Surficial Deposits and Materials in the Eastern and Central United States (East of 102 Degrees West Longitude). Technical Report, U.S. Geological Survey Miscellaneous Investigations Series Map I-2789.
Garry, C. E., Schwert, D. P., Baker, R. G., Kemmis, T. J., Horton, D. G., and Sullivan, A. E. (1990). Plant and insect remains from the Wisconsinan interstadial/stadial transition at Wedron, northcentral Illinois. Quat. Res. 33, 387–399. doi: 10.1016/0033-5894(90)90064-R
Gran, K. B., Belmont, P., Day, S. S., Jennings, C., Johnson, A., Perg, L., et al. (2009). “Geomorphic evolution of the Le Sueur River, Minnesota, USA, and implications for current sediment loading,” in Management and Restoration of Fluvial Systems with Broad Historical Changes and Human Impacts: Geological Society of America Special Paper 451, eds A. L. James, S. L. Rathburn, and G. R. Whittecar (Boulder, CO: Geological Society of America), 119–130.
Gran, K. B., Finnegan, N., Johnson, A. L., Belmont, P., Wittkop, C., and Rittenour, T. (2013). Landscape evolution, valley excavation, and terrace development following abrupt postglacial base-level fall. Geol. Soc. Am. Bull. 125, 1851–1864. doi: 10.1130/B30772.1
Grimley, D. A., Anders, A. M., and Stumpf, A. J. (2016a). “Quaternary Geology of the Upper Sangamon River Basin: Glacial, Postglacial, and Postsettlement History,” in 1967–2016—Celebrating 50 Years of Geoscience in the Mid-Continent: Guidebook for the 50th Annual Meeting of the Geological Society of America–North-Central Section, eds Z. Lasemi and S. D. Elrick (Champaign, IL: Illinois State Geological Survey Guidebook), 55–96.
Grimley, D. A., Anders, A. M., Bettis, A. E. III, Bates, B. L., Wang, J. J., Butler, S. K., et al. (2017). Using magnetic fly ash to identify post-settlement alluvium and its record of atmospheric pollution, central USA. Anthropocene 17, 84–98. doi: 10.1016/j.ancene.2017.02.001
Grimley, D. A., Follmer, L. R., Hughes, R. E., and Solheid, P. A. (2003). Modern, Sangamon and Yarmouth soil development in loess of unglaciated southwestern Illinois. Quat. Sci. Rev. 22, 225–244. doi: 10.1016/S0277-3791(02)00039-2
Grimley, D. A., Wang, J. J., and Oien, R. P. (2016b). Surficial Geology of Mahomet Quadrangle, Champaign and Piatt Counties, Illinois. Illinois State Geological Survey, USGS-STATEMAP contract report, 1:24,000.
Grüger, E. (1972). Late Quaternary vegetation development in south-central Illinois. Quat. Res. 2, 217IN3-231IN4.
Guanter, L., Zhang, Y., Jung, M., Joiner, J., Voigt, M., Berry, J. A., Frankenberg, C., et al. (2014). Global and time-resolved monitoring of crop photosynthesis with chlorophyll fluorescence. Proc. Natl. Acad. Sci. U.S.A. 111, E1327–E1333. doi: 10.1073/pnas.1320008111
Hackley, K. C., Panno, S. V., and Anderson, T. F. (2010). Chemical and isotopic indicators of groundwater evolution in the basal sands of a buried bedrock valley in the midwestern United States: implications for recharge, rock-water interactions, and mixing. Geol. Soc. Am. Bull. 122, 1047–1066. doi: 10.1130/B26574.1
Hallberg, G. R. (1986). Pre-Wisconsin Glacial Stratigraphy of the Central Plains Region in Iowa, Nebraska, Kansas, and Missouri. Quat. Sci. Rev. 5, 11–15.
Hansel, A. K., and McKay, E. D. III. (2010). “Quaternary Period,” in Geology of Illinois, eds D. R. Kolata and C. K. Nimz, (Champaign, IL: Illinois State Geological Survey), 216–247.
Hansel, A. K., and Johnson, H. W. (1996). Wedron and Mason Groups: Lithostratigraphic Reclassification of Deposits of the Wisconsin Episode, Lake Michigan Lobe Area, 104. Champaign, IL: Illinois State Geological Survey Bulletin.
Herzog, B. L., Wilson, S. D., Larson, D. R., Smith, E. C., Larson, T. H., and Greenslate, M. L. (1995). Hydrogeology and Groundwater Availability in Southwest McLean and Southeast Tazewell Counties: Part 1, Aquifer Characterization, 17. Illinois State Geological Survey, Cooperative Groundwater Report.
Horberg, L. (1945). A major buried valley in east-central Illinois and its regional relationships. J. Geol. 53, 349–359. doi: 10.1086/625294
INHS, (2003). (Illinois Natural History Survey) Land Cover of Illinois in the Early 1800s: Vector Digital Data, Version 6.0. Available Online at: http://clearinghouse.isgs.illinois.edu/data/landcover/illinois-landcover-early-1800s
Jennings, C. E., Lusardi, B. A., and Gowan, A. S. (2011). “Plate 3-Surficial Geology” in C-26 Geologic Atlas of Blue Earth County, Minnesota [Part A]. Minnesota Geological Survey, eds A. C. Runkel, G. N. Meyer, and B. A. Lusardi. Available Online at: http://hdl.handle.net/11299/116097
Johnson, M. D., Adams, R. S., Gowan, A. S., Harris, K. L., Hobbs, H. C., Jennings, C. E., et al. (2016). RI-68 Quaternary Lithostratigraphic Units of Minnesota. Minnesota Geological Survey. Available online at: http://hdl.handle.net/11299/177675
Johnson, W. H. (1990). Ice-wedge casts and relict patterned ground in central Illinois and their environmental significance. Quat. Res. 33, 51–72. doi: 10.1016/0033-5894(90)90084-X
Keefer, L., Bauer, E., and Markus, M. M. (2010). Hydrologic and Nutrient Monitoring of the Lake Decatur Watershed: Final Report 1993–2008. Champaign, IL: Illinois State Water Survey.
Kempton, J. P., Hilton Johnson, W., Heigold, P. C., and Cartwright, K. (1991). “Mahomet Bedrock Valley in east-central Illinois; topography, glacial drift stratigraphy, and hydrogeology,” in Geology and hydrogeology of the Teays-Mahomet Bedrock Valley System, GSA Special Paper 258, eds W. N. Melhorn, and J. P. Kempton (Boulder, CO: Geological Society of America), 91–129.
King, J. E. (1981). Late Quaternary vegetational history of Illinois. Ecol. Monogr. 51, 43–62. doi: 10.2307/2937306
Knox, J. C. (1972). Valley alluviation in southwestern Wisconsin. Ann. Assoc. Am. Geogr. 62, 401–410. doi: 10.1111/j.1467-8306.1972.tb00872.x
Kołodynska-Gawrysiak, R., Chodorowski, J., Mroczek, P., Plak, A., Zgłobicki, W., Kiebała, A., et al. (2017). The impact of natural and anthropogenic processes on the evolution of closed depressions in loess areas. A multi-proxy case study from Nałeczów Plateau, Eastern Poland. CATENA 149, 1–18. doi: 10.1016/j.catena.2016.07.029
Kreznor, W. R., Olson, K. R., Jones, R. L., and Johnson, D. L. (1990). Quantification of postsettlement deposition in a northwestern Illinois sediment basin. Soil Sci. Soc. Am. J. 54, 1393–1401. doi: 10.2136/sssaj1990.03615995005400050031x
Lisiecki, L. E., and Raymo, M. E. (2005). A Pliocene-Pleistocene stack of 57 globally distributed benthic δ18O records. Paleoceanography 20:1. doi: 10.1029/2004PA001071
Lothrop, J. C., Lowery, D. L., Spiess, A. E., and Ellis, C. J. (2016). Early human settlement of Northeastern North America. PaleoAmerica 2, 192–251, doi: 10.1080/20555563.2016.1212178
Lusardi, B. A., Jennings, C. E., and Harris, K. L. (2011). Provenance of Des Moines lobe till records ice-stream catchment evolution during Laurentide deglaciation. Boreas 40, 585–597. doi: 10.1111/j.1502-3885.2011.00208.x
Mason, J. A. (2015). Up in the refrigerator: geomorphic response to periglacial environments in the Upper Mississippi River Basin, USA. Geomorphology 248, 363–381. doi: 10.1016/j.geomorph.2015.08.004
Mason, J. A., Joeckel, R. M., and Bettis, E. A. III. (2007). Middle to late Pleistocene Loess Record in Eastern Nebraska, U.S.A., and Implications for the Unique Nature of Oxygen Isotope Stage 2. Quat. Sci. Rev. 26, 773–792. doi: 10.1016/j.quascirev.2006.10.007
Matsch, C. I. (1983). “River Warren, the southern outlet of Lake Agassiz,” in Glacial Lake Agassiz: Geological Association of Canada Special Paper 26, eds J. T. Teller and L. L. Clayton (St. John's: Geological Association of Canada), 232–244.
Melhorn, W. N., and Klempton, J. P. (1991). Geology and hydrogeology of the Teays-Mahomet Bedrock Valley System. Boulder, CO: Geological Society of America, Special Paper 258:128.
Meyer, G. N., Knaeble, A. R., Lusardi, B. A., Jennings, C. E., and Gowan, A. S. (2011). “Quaternary stratigraphy,” in C-26 Geologic Atlas of Blue Earth County, Minnesota [Part A]. Minnesota Geological Survey, eds A. C. Runkel, G. N. Meyer, and B. A. Lusardi. Available online at: http://hdl.handle.net/11299/116097
Mount, H. R. (1982). Soil Survey of Champaign County, Illinois. Champaign, IL: U.S. Department of Agriculture and Illinois Agriculture Experiment Station: 178.
Muhs, D. R., and Bettis, E. A. III. (2003). “Quaternary loess-paleosol sequences as examples of climate-driven sedimentary extremes,” in Extreme Depositions Environments: Mega End Members in Geologic Time, 370, eds M. A. Chan and A. W. Archer (Boulder, CO: Geological Society of America), 53–74.
Nash, T. A., Conroy, J. L., Grimley, D. A., Guenthner, W. R., and Curry, B. B. (2017). Episodic deposition of Illinois Valley Peoria Silt in association with Lake Michigan Lobe fluctuations during the Last Glacial Maximum. Quat. Res. doi: 10.1017/qua.2017.66. [Epub ahead of print].
Neal, C. W. M., and Anders, A. M. (2015). Suspended sediment supply dominated by bank erosion in a low-gradient agricultural watershed, Wildcat Slough, Fisher, Illinois, United States. J. Soil Water Conserv. 70, 145–155. doi: 10.2489/jswc.70.3.145
Nelson, D. M., Sheng Hu, F., Grimm, E. C., Brandon Curry, B., and Slate, J. E. (2006). The influence of aridity and fire on Holocene prairie communities in the eastern Prairie Peninsula. Ecology 87, 2523–2536. doi: 10.1890/0012-9658(2006)87[2523:TIOAAF]2.0.CO;2
Norton, L. D. (1986). Erosion-sedimentation in a closed drainage basin in northwest Indiana. Soil Sci. Soc. Am. J. 50, 209–213. doi: 10.2136/sssaj1986.03615995005000010040x
NRC, I. (2001). (US National Research Council) Basic Research Opportunities in Earth Science. Washington, DC: National Academy Press.
Patterson, C. J., and Boerboom, T. J. (1999). The significance of pre-existing, deeply weathered crystalline rock in interpreting the effects of glaciation in the Minnesota River valley, U.S.A. Ann. Glaciol. 28, 53–58. doi: 10.3189/172756499781821995
Patterson, C. J., and Hobbs, H. C. (1995). “Surficial geology,” in Geologic Atlas of Rice County, Minnesota: Minnesota Geological Survey County Atlas C-9, ed H. C. Hobbs (St. Paul, MN: Plate 3, Minnesota Geological Survey).
Phillips, R. T. J., and Desloges, J. R. (2015). Glacial legacy effects on river landforms of the southern Laurentian Great Lakes. J. Great Lakes Res. 41, 951–964. doi: 10.1016/j.jglr.2015.09.005
Rhoads, B. L., Lewis, Q. W., and Andresen, W. (2016). Historical changes in channel network extent and channel planform in an intensively managed landscape: Natural versus human-induced effects. Geomorphology 252, 17–31. doi: 10.1016/j.geomorph.2015.04.021
Richardson, M., and Kumar, P. (2017). Critical Zone services as environmental assessment criteria in intensively managed landscapes. Earth's Future 5, 617–632. doi: 10.1002/2016EF000517
Riebe, C. S., Jesse Hahm, W., and Brantley, S. L. (2017). Controls on deep critical zone architecture: a historical review and four testable hypotheses. Earth Surface Proc. Landforms 42, 128–156. doi: 10.1002/esp.4052
Roadcap, G. S., Vernon Knapp, H., Allen Wehrmann, H., and Larson, D. R. (2011). Meeting East-Central Illinois Water Needs to 2050: Potential Impacts on the Mahomet Aquifer and Surface Reservoirs. Champaign, IL: Illinois State Water Survey, Circular 2011-08: 179.
Roy, M., Clark, P. U., Duncan, R. A., and Hemming, S. R. (2007). Insights into the late Cenozoic configuration of the Laurentide Ice Sheet from 40Ar/39Ar dating of glacially transported minerals in midcontinent tills. Geochem. Geophys. Geosyst. 8, 1–12. doi: 10.1029/2006GC001572
Roy, M., Clark, P. U., Raisbeck, G. M., and Yiou, F. (2004). Geochemical constraints on the regolith hypothesis for the middle Pleistocene transition. Earth Planet. Sci. Lett. 227, 281–296. doi: 10.1016/j.epsl.2004.09.001
Ruhe, R. V. (1984). Soil-climate system across the prairies in Midwestern U.S.A. Geoderma 34, 201–219. doi: 10.1016/0016-7061(84)90039-9
Runkel, A. C., Meyer, G. N., and Lusardi, B. A. (2011). C-26 Geological Atlas of Blue Earth County, Minnesota [Part A]. St. Paul, MN: Minnesota Geological Survey.
Schwert, D. P., Torpen-Kreft, H. J., and Hajic, E. R. (1997). “Characterization of the Late-Wisconsinan forest–tundra transition in midcontinental North America using assemblages of beetle fossils,” in Studies in Quaternary Entomology – an Inordinate Fondness for Insects, eds A. C. Ashworth, P. C. Buckland, and J. P. Sadler (Chichester, UK: John Wiley and Sons), 237–243.
Skidmore, M. L., Foght, J. M., and Sharp, M. J. (2000). Microbial life beneath a high Arctic glacier. Appl. Environ. Microbiol. 66, 3214–3220. doi: 10.1128/AEM.66.8.3214-3220.2000
Soller, D. R., Price, S. D., Kempton, J. P., and Berg, R. C. (1999). Three-Dimensional Geologic Maps of Quaternary Sediments in East-Central. Illinois: U.S. Geological Survey, Geologic Investigations Series Map I-2669: 1:500,000.
Soller, D. R., Reheis, M. C., Garrity, C. P., and Van Sistine, D. R. (2009). Map Database for Surficial Materials in the Conterminous United States. Reston, VA: U.S. Geological Survey Data Series 425. Available online at: http://pubs.usgs.gov/ds/425/
Stumpf, A. J., and Dey, W. S. (2012). Understanding the Mahomet Aquifer: Geological, Geophysical, and Hydrogeological Studies in Champaign County and Adjacent Areas. Champaign, IL: Illinois State Geological Survey, draft report to Illinois American Water, contract no. 2007-02899. Available online at: http://hdl.handle.net/2142/95787
Stumpf, A. J., and Atkinson, L. A. (2015). Geologic Cross Sections across the Mahomet Bedrock Valley, Champaign, Ford, McLean, Piatt, and Vermilion Counties, Illinois. Champaign, IL: Illinois State Geological Survey, Illinois Map IMAP 19, 1:48,000.
Tandarich, J. P., Darmody, R. G., and Follmer, L. R. (1994). “The Pedo-Weathering Profile: A Paradigm for Whole-Regolith Pedology from the Glaciated Midcontinental United States of America,” in Whole Regolith Pedology, eds D. L. Cremeens, R. B. Brown, J. H. Huddleston (Madison, WI: SSSA Spec. Publ. 34. Soil Science Society of America), 97–117.
Teller, J. T., and Leverington, D. W. (2004). Glacial Lake Agassiz: A 5000 yr history of change and its relationship to the δ18O record of Greenland. Geol. Soc. Am. Bull. 116, 729–742. doi: 10.1130/B25316.1
United States Environmental Protection Agency Notice 80 FR 14370 (2015):14370-14371. Available online at: https://www.federalregister.gov/documents/2015/03/19/2015-06365/sole-source-aquifer-designation-of-the-mahomet-aquifer-system-in-east-central-illinois
Wickham, J. T. (1979). Glacial Geology of North-Central and Western Champaign County. Champaign, IL: Illinois State Geological Survey, Circular 506, 30.
Willman, H. B., and Frye, J. C. (1970). Pleistocene Stratigraphy of Illinois. Champaign, IL: Illinois State Geological Survey Bulletin no. 094. Available online at: http://hdl.handle.net/2142/43629
Keywords: critical zone, depositional environment, soils formed in glacial sediments, IML-CZO, US Interior Lowlands
Citation: Anders AM, Bettis EA III, Grimley DA, Stumpf AJ and Kumar P (2018) Impacts of Quaternary History on Critical Zone Structure and Processes: Examples and a Conceptual Model From the Intensively Managed Landscapes Critical Zone Observatory. Front. Earth Sci. 6:24. doi: 10.3389/feart.2018.00024
Received: 13 November 2017; Accepted: 05 March 2018;
Published: 20 March 2018.
Edited by:
Gary E. Stinchcomb, Murray State University, United StatesReviewed by:
Lee Nordt, Baylor University, United StatesJulia Perdrial, University of Vermont, United States
Copyright © 2018 Anders, Bettis, Grimley, Stumpf and Kumar. This is an open-access article distributed under the terms of the Creative Commons Attribution License (CC BY). The use, distribution or reproduction in other forums is permitted, provided the original author(s) and the copyright owner are credited and that the original publication in this journal is cited, in accordance with accepted academic practice. No use, distribution or reproduction is permitted which does not comply with these terms.
*Correspondence: Alison M. Anders, YW1hbmRlcnNAaWxsaW5vaXMuZWR1