- 1Department of Geological Sciences, University of Florida, Gainesville, FL, United States
- 2Department of Geological Sciences, East Carolina University, Greenville, NC, United States
Our understanding of the cycling of fire-derived, i.e., pyrogenic organic matter (pyOM), as well as the goals of the community of researchers who study it, may be inhibited by the many terms and methods currently used in its quantification and characterization. Terms currently used for pyOM have evolved by convention, but are often poorly defined. Further, each of the different methods now used to quantify solid and dissolved pyrogenic carbon (pyC) comes with its own biases and artifacts. That is, each detects only a fraction of the total pyrogenic products produced by fire, while, at the same time, include some fraction of non-pyrogenic OM. This may be evident in the commonly observed correlations between pyC and total organic C reported for both soils and dissolved OM in many different systems. We suggest that our research area can be placed on a stronger footing by: (1) agreement upon a common set of terms tied to the method used for detection (e.g., of the form pyCmethod), (2) implementation of another “ring trial” study with a wider set of natural soil and water samples that cross-compare more recently developed methods, and (3) further investigation of the processes which preserve/degrade/transport pyOM in the environment.
Introduction
The understanding that fire and pyrogenic organic matter (pyOM) have contributed to shaping Earth's biosphere is one that has evolved within a number of disparate fields including geology, ecology, atmosphere science, agriculture/soil science, and anthropology. In each field, this realization, along with associated terminology and methodology, has tended to evolve separately, with limited cross-disciplinary communication. Humans have been using pyOM in industry (charcoal used in the smelting of copper as early as 5,000 BCE) and medicine for thousands of years (Scott and Damblon, 2010). However, formal research into charcoal's properties began as early the beginning of the twentieth century (e.g., Hedin, 1907; Sweetser, 1908; Homfray, 1910). Charcoal was noted in soils even earlier (e.g., Heer, 1866; Fliche, 1907; Godwin and Tansley, 1941), but was not treated quantitatively and used as an indicator of past vegetation and human settlement by paleoecologists and archeologists, respectively, until much later (Western, 1963; Camps, 1971). Detection and quantification of charcoal in marine and lake sediments led to the first use of pyOM as a proxy for past fire-frequency and climate in the 1970's (Smith et al., 1973; Herring, 1976; Swain, 1978), though there was some debate at this time as to its pyrogenic origin (Schopf, 1975).
Consideration of the pyrogenic component of aerosols and the development of the first instrument to measure aerosol soot concentrations (Thomas, 1952) was sparked by the “London fog” of December 1952 which killed at least 4,000 (Wilkins, 1954). The dark color of the filters used to collect the “soot” derived from coal-burning led to use of the term “black carbon” (BC) within the field of atmospheric chemistry (e.g., Novakov, 1981, 1984; Gundel et al., 1984). Novakov (1981, 1984) defined BC as “combustion-produced black particulate carbon having a graphitic microstructure” and soon, the term “BC” was applied more broadly to pyOM in both its atmospheric and geological forms (Goldberg, 1985). However, the term “elemental carbon,” reflecting its C-rich character, was and is still also widely used by the atmospheric community, which has largely been concerned with its light absorption and associated direct radiative forcing of Earth's climate (Bond et al., 2004). Although there have been quite a few inter-laboratory comparisons of methods used to quantify aerosol BC (e.g., Countess, 1990; Birch, 1998; Hitzenberger et al., 1999, 2006; Schmid et al., 2001; ten Brink et al., 2004), there is still no universally accepted method for isolating aerosol pyOM (discussed further in next section).
Awareness of elevated atmosphere CO2 and other atmosphere greenhouse gas concentrations in the 1970's led to consideration of the role of fire in the global C cycle. The first estimate of global biomass burning and charcoal production, 500–700 Tg yr−1 (Seiler and Crutzen, 1980) was an attempt to balance the poor match between C inputs to the atmosphere with known removal mechanisms. This estimate was since revised downward and is now generally agreed to be in the range of 50–300 Tg yr−1 (Kuhlbusch and Crutzen, 1996; Forbes et al., 2006; Bird et al., 2015; Santin et al., 2016). Another turning point in geochemists' understanding of pyOM was the “BC combustion continuum” first proposed by Hedges et al. (2000) and Schmidt and Noack (2000) and later elaborated upon by others (Masiello, 2004; Elmquist et al., 2006; Preston and Schmidt, 2006). It maintained that pyOM is composed of a wide range of materials from slightly charred biomass to highly condensed graphite and soot. With this “continuum perspective” came the wider recognition that pyOM cannot be wholly refractory, but is degraded to different extents through a variety of abiotic and microbially-enhanced processes.
A call to compare methods used to quantify pyrogenic carbon (pyC) in soils and sediments, which requires the use of techniques different from those used in atmospheric sciences due to the presence of interfering matrices, came at much the same time. The first method comparison efforts examined a limited range of sample types (Currie et al., 2002) or resulted in widely ranging values for individual samples (Schmidt et al., 2001). Thus, a “Steering Committee for Black Carbon Reference Materials” was established and recommended a larger set of pyrogenic and non-pyrogenic test materials (Schmidt et al., 2003). The resulting comprehensive evaluation of pyC quantification methods (Hammes et al., 2007), the so called “BC ring trial,” made it clear that each analytical method is selective for a different part of the pyOM continuum.
At the same time that soil scientists and agronomists were realizing the potential of soil amendments of pyC to enhance soil fertility and mitigate climate change through C sequestration (Lehmann et al., 2002; Lehmann, 2007a,b), environmental scientists identified substantial amounts of pyC in an ever-widening range of settings including river water (Ding et al., 2013; Jaffe et al., 2013), marshes (Dittmar et al., 2012b) and the ocean (Dittmar, 2008; Stubbins et al., 2010; Ziolkowski and Druffel, 2010). However, all of these pursuits require the ability to accurately quantify pyC and to track the chemical evolution and transport of pyOM in the environment.
Pyrogenic Substances Quantification Methods
The methods used to quantify pyC vary widely in their cost, ease of application, and unfortunately, in the pyOM fraction that they target. These methods can be categorized as those that rely on pyOM detection of: (1) morphology, (2) light absorption, (3) thermal or chemical stability, and (4) chemical composition. Archeologists and some geoscientists commonly use light microscopy and particle morphology to count the number and size of charcoal particles (e.g., Smith et al., 1975; Figueiral and Mosbrugger, 2000; Scott and Damblon, 2010). However, these methods cannot quantify pyC and are biased toward detection of particles of larger sizes (Masiello, 2004; Crawford and Belcher, 2016). Atmospheric scientists quantify pyC using optical techniques (Rosen and Novakov, 1977), thermal heating combined with optical methods, or laser-induced incandescence (Watson et al., 2005; for a historical perspective, see Novakov and Rosen, 2013). While many of these methods are subject to interferences introduced during mixing of combustion-derived aerosols with non-pyrogenic OM (Bond et al., 2013), quantification of pyC in soil or sedimentary matrices is even more difficult due to the even greater presence of minerals and complex non-pyrogenic geopolymers. To cope with this, many approaches assume pyOM to be the most refractory OM fraction. Thus, different thermal and/or chemical oxidation techniques (e.g., CTO-375, dichromate oxidation, UV oxidation, catalytic hydrogen pyrolysis (hy-py) have been used to remove more labile OM and assume the residual to be pyOM (e.g., Lim and Cachier, 1996; Gustafsson et al., 1997, 2001; Thevenon et al., 2010).
A final group of methods identify pyOM using some aspect of its chemical structure. The abundance of both levoglucosan, an anhydrous sugar formed during cellulose combustion (Elias et al., 2001; Kuo et al., 2008), and benzenepolycarboxylic acids (BPCAs) which are formed via nitric acid oxidation (Glaser et al., 1998; Brodowski et al., 2005; Dittmar, 2008) have been used as chemical markers for lower temperature charred OM and condensed aromatic C, respectively. Another technique infers the pyC content of a sample using the sorptive characteristics of pyrene onto the sample (Flores-Cervantes et al., 2009a,b). Spectroscopic tools such as 13C-nuclear magnetic resonance (NMR) and mid-infrared (MIR) spectroscopy provide information on the structure and chemical bonds of OM present in a material. In the case of NMR, it is generally aromatic C that is quantified and associated with pyC (Simpson and Hatcher, 2004; Nelson and Baldock, 2005), though specialized techniques have been developed to estimate the condensed aromatic fraction (Knicker et al., 2005; McBeath et al., 2011). Though MIR also examines a sample's spectral characteristics, it requires calibration using one of the other pyC methods in order to be quantitative (Janik et al., 2007; Bornemann et al., 2008; Cotrufo et al., 2016). More detailed reviews of methods used to quantify pyC can be found elsewhere (Meredith et al., 2012; Bird et al., 2015).
Problems and Perplexities
While the methods used to study pyOM production and cycling have advanced greatly in the last decade, we believe that there are still terminology, methodology/conceptual impediments to further progress that urgently need to be addressed. This inhibits both research funding opportunities and implementation of the fire-science community findings and recommendations, be they in settings of agriculture, policy, or climate/geochemical model incorporation.
Terminology Issues
From the discussion above, it should be clear that different fire research sub-communities have adopted various terms for pyOM or pyOM fractions, often for historical reasons only. Confusion arises because these terms are often not associated with specific definitions, or because terms have been adopted across research community boundaries without regard for their original narrow definitions. For example, the atmospheric community refers to BC as combustion-generated aerosols that absorb visible light, are insoluble in water, and exist as aggregates of carbon spherules (Novakov, 1984; Bond et al., 2013). On the other hand, BC has come to be used by many as shorthand for pyrogenic C of all forms. The term “dissolved black carbon” conflicts with the atmospheric community's definition that BC should not be soluble in water. Organic compounds detected in the aqueous fraction of combustion-derived aerosols are typically referred to by the atmospheric community as water soluble organic carbon (Decesari et al., 2000; Mayol-Bracero et al., 2002). In the past, soot was defined as the total carbonaceous material produced by combustion (Novakov, 1984). However, for others, soot is the condensate of combustion gases and has graphitic structure (Hammes et al., 2007). The materials referred to as charcoal, biochar, and agrichar may all be the same, or somewhat different (Lehmann and Joseph, 2015). While it is understood that different analytical methods target different pyC fractions, there is as yet no consistent method or property-based terminology applied across different research communities. This lack of consistency may inhibit cross-disciplinary communication and fertilization of new concepts.
Methodology/Conceptual Issues
Several methodological issues and recent observations should cause us to question our ability to quantify the amount of pyOM (or pyC) in natural samples. The first issue is that many of these methods require a “conversion factor” of some type to transform measured parameters such as a post-treatment residue weight or compound abundance to an amount of pyOM or pyC present in a sample. For example, a conversion factor has been calculated based on theoretical BPCA yields of organic structures of marine DOM observed via ultrahigh-resolution mass spectrometry (about 3, Dittmar, 2008; Stubbins et al., 2012) or from the BPCA yields of various aromatic substances including activated C (2.27, Glaser et al., 1998) PAHs, soot and C nanotubes (about 4, Ziolkowski et al., 2011). However, application of any single BPCA conversion factor to a variety of pyOM types has been called into question (Brodowski et al., 2005). For the dichromate oxidation procedure, a correction factor derived from the residual yield of plant char oxidation was used to account for pyC losses during the chemical treatment (Knicker et al., 2008). A degree of uncertainty has been generated in the fire science community because of the variety and range of conversion and correction factors used, even within specific analytical methods.
The second issue is that pyC can be falsely identified as non-pyC and vice versa. Underestimates in pyC quantification certainly occur because not all pyC is recalcitrant or purely condensed aromatic, as assumed by most analytical methods (e.g., Bostick et al., this issue, Nguyen and Lehmann, 2009; Zimmerman, 2010; Singh et al., 2012). Some portion of pyC is likely destroyed in the harsh thermal and chemical oxidation steps used. Overestimates can occur in a number of ways. Not all non-pyC is removed by thermal/chemical oxidation steps (e.g., Knicker et al., 2007). Oxidative treatments can even generate apparently “pyrogenic” OM (e.g., Derenne and Largeau, 2001; Hammes et al., 2007; Novakov and Rosen, 2013). This has been shown to occur, for example, during the oxidation required to form BPCA compounds (Brodowski et al., 2005; Kappenberg et al., 2016) as well as in steps used to remove non-pyrogenic aromatic C prior to NMR analysis (Simpson and Hatcher, 2004). Other NMR quantification approaches use spectral editing or molecular mixing models to separate condensed aromatic from non-condensed aromatic biomolecules, both of which require assumptions or corrections that have not been fully validated (Cusack et al., 2012; Paetsch et al., 2017). Light absorption-based methods used mainly by atmosphere chemists suffer from uncertainties related to non-linearities in the light attenuation coefficient which vary with filter loading and particle type, as well as to interferences from non-pyrogenic OM (reviewed in Kirchstetter and Novakov, 2007).
Light absorption, BPCA and some NMR quantification techniques use the assumption that only pyOM, takes the form of condensed aromatics structures. But increasingly, this has been shown not to be the case. For instance, melanoidins, several plants, fungi and pigments yielded quantities of BPCAs (even highly-carboxylated BPCAs which are indicative of very condensed aromatic OM) like that of charred plant material (Brodowski et al., 2005; Glaser and Knorr, 2008). These may be derived from lignin or tannin, which include a wide variety of polycondensed aromatics (Hernes and Hedges, 2000; Waggoner et al., 2015). Other non-pyrogenic OM sources of condensed aromatic OM are abundant in the geosphere, including woody peat, coal, kerogen, and oil (Yoshioka and Ishiwatari, 2005; Hammes et al., 2007; Wang et al., 2012; Hartman et al., 2015; Li et al., 2017). Still other studies have found that non-pyrogenic OM can be readily transformed to condensed aromatic OM, which would appear to be pyrogenic, through photolytic, microbial, or chemical degradative processes (Glaser and Knorr, 2008; Chen et al., 2014; Waggoner et al., 2015; DiDonato et al., 2016).
Given that we are finding ever more ways in which pyOM can cross the analytical window into non-pyOM and vice versa, it is perhaps not surprising that many have observed correlations between pyC and total C concentrations in soils regionally (Glaser and Amelung, 2003; Jauss et al., 2015; Ahmed et al., 2017; Qi et al., 2017) and globally (Reisser et al., 2016), and dissolved in natural waters regionally (Dittmar et al., 2012a; Ding et al., 2013, 2014, 2015; Guerena et al., 2015) and globally (Jaffe et al., 2013; Wagner et al., 2015) and even in aqueous leachates of marine aerosols (Bao et al., 2017). These correlations indicate, as do other more detailed statistical examinations (in the same studies), a lack of dependence of pyC concentrations on fire history or climate. A plot of data compiled by a recent literature review (Reisser et al., 2016) shows a significant relationship between pyC and total C in global soils across all quantification methods and within each method (except CTO-375, Figure 1). The strongest correlations are found for data derived from BPCA and NMR, suggesting that these methods may have the greatest likelihood for artifacts that misidentify pyC. Alternatively, the finding of correlation between pyC and total C regardless of the analytical method used, might suggest the relationship is present in nature. That is, production, degradation/preservation or mobilization processes may act on pyC and non-pyC in ways that cause them to co-vary. For example, regions of higher productivity, thus higher soil C, also have more biomass to burn and are therefore likely to have greater pyC in soils and drainage waters (Alexis et al., 2007; van Leeuwen et al., 2014). Soils with greater amounts of clay or metal oxide mineral or even charcoals, are likely to sorb and therefore protect both pyOM and non-pyOM from microbial mineralization through sorptive protection (Kasozi et al., 2010; Zimmerman et al., 2011) or aggregate stabilization (Wang et al., 2017). And soil translocation, erosion, leaching, and other hydrologic/climatic-related processes of a region are likely to act to mobilize both pyOM and non-pyOM in similar ways (Hilscher and Knicker, 2011; Jien and Wang, 2013), though not necessarily to equal extents (Rumpel et al., 2009). Finally, it has been suggested that pyC mobilization may occur via association with other OM in dissolved (Jaffe et al., 2013) or perhaps colloidal (Zand and Grathwohl, 2016; Kumari et al., 2017) form, but the controlling mechanisms are still unknown (Wagner et al., 2017).
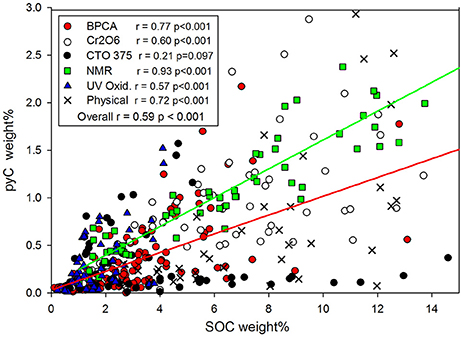
Figure 1. Relationships between total soil organic carbon (SOC) and pyrogenic carbon (pyC) as determined by different methods. Pearson linear correlation coefficients (r) and levels of significance (p) are given in the legend. Data are taken from Reisser et al. (2016), a compilation of results of global soil data from 55 studies.
Recommendations
Despite, or possibly even because of the many issues facing the pyC cycling research community, we may be on the brink of making great advances in this field, but only if these issues are acknowledged and dealt with. First, we suggest more stringent use of terminology. The terms “pyOM” or “pyC” should be used when referring to the totality of fire-derived carbonaceous substances. These terms represent the broadest short forms for the total substance of pyrogenic origin and the carbon in these substances, respectively. When reporting analytical results, we suggest that terms tied to the method or properties used for detection should be used. For greatest clarity, we these terms could take the form “pyCmethod” (Figure 2). For example, substances quantified via light microscopy might be referred to as pyCmic. Substances isolated based on their chemical or thermal resistance could be designated pyCCTR. The term “pyCLE” should be used for substances detected based upon their near complete light extinction properties, but failing to convince the mainly atmospheric community of this, we suggest that terrestrial and aquatic scientists leave the term “black carbon” to them. Use of these terms will serve as continuous reminder that a quantity of C refers only to a portion of the substances produced by fires and may even contain a non-pyrogenic portion.
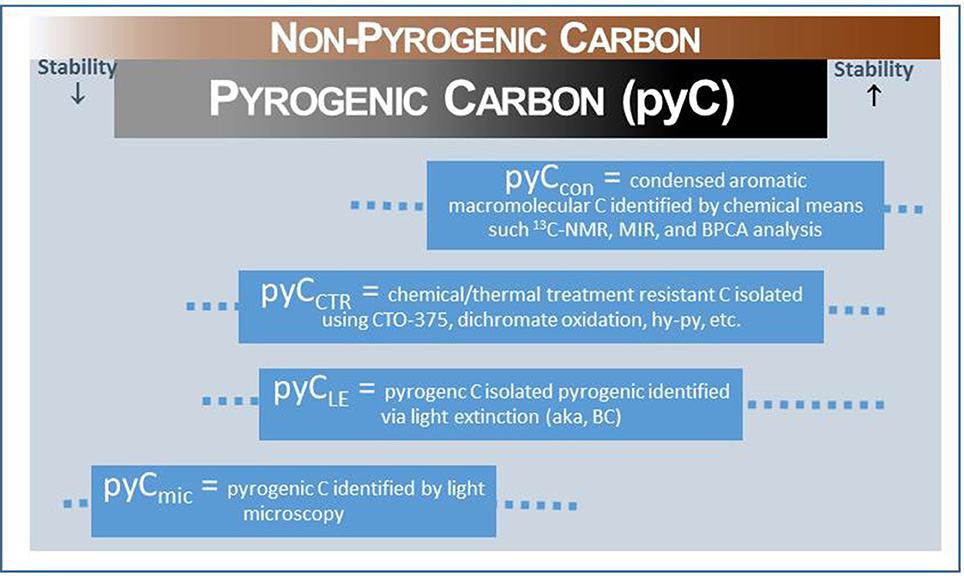
Figure 2. Proposed pyrogenic carbon terminology based upon methods used for identification/quantification and estimated placement of these materials on the “combustion/stability continuum.” Dotted lines represent estimated uncertainly range.
Regarding the potential for analytical artifacts that plague pyC cycling research, we recommend, first, that another “ring trial” study be conducted so that techniques that have been developed since the last ring trial (such as MIR spectroscopy and catalytic hydrogen pyrolysis and improvements in NMR and BPCA analytical methods) can be compared and their relative strengths and weaknesses re-evaluated. This ring study should include not just geochemists, but also those that study pyOM from the anthropology, atmosphere and agriculture communities. Moreover, the new ring trial should also make use of modern analytical techniques that can deconvolve composition of pyOM at unprecedented levels (e.g., aerosol mass spectrometer, Fourier transform ion cyclotron resonance mass spectrometer). In addition to the set of pyrogenic and “potentially interfering” materials included in the first ring trial (Hammes et al., 2007), this new ring trial should include a biochar thermal series, which would be expected to have a regularly increasing degree of aromatic condensation (Cao et al., 2012). Furthermore, we recommend a broader set of atmosphere-derived samples such as the diesel soot standard (NIST SRM 2975) and aqueous samples (preferably not freeze-dried) isolated from river and ocean water. Additional non-pyrogenic materials such as wood biomass, leachate from this biomass, and photodegraded biomass leachate should also be considered for analyses. To evaluate matrix effects associated with each method, a standard addition experiment should be added to the method intercomparison, whereby different amounts of a pyOM, such as wood char, are added to a soil-like mixture containing no pyOM. This ring trial should be followed up not only with a report of results, but with a best-practices paper that includes consensus recommendations for use of terminology.
Until now, the focus of many fire science studies has been to establish properties of pyrogenic substances and their inventories in different systems. Given the major question of the cause of the often-observed pyC/TOC correlation, a greater focus should be placed on studies that compare transformation and movement of pyrogenic relative to different types of non-pyrogenic substances. Mechanistic pyOM investigations are needed to understand both preservation processes such as adsorption, metal-complexation and aggregate formation, and transformation processes such as solubilization, volatilization, and microbial, chemical and photo-degradation. In addition, pyC mobilization studies should focus not just on particle movement in soil via translocation and erosion, but transport in aerosol, colloid, and dissolved forms via atmosphere and aqueous processes. We hope this comment stimulates greater dialog between research communities that study various aspects of pyrogenic substances. The desired result would be not only a more complete understanding of the production and cycling of pyC, but also a greater application of these insights in such areas as agriculture and climate modeling.
Author Contributions
All authors listed have made a substantial, direct and intellectual contribution to the work, and approved it for publication.
Funding
This work was funded by the U.S. National Science Foundation—Geobiology and Low-Temperature Geochemistry Program (EAR-1451367).
Conflict of Interest Statement
The authors declare that the research was conducted in the absence of any commercial or financial relationships that could be construed as a potential conflict of interest.
Acknowledgments
This work was substantially improved through helpful conversations with Drs. Gerard Cornelissen and Michael Bird.
References
Ahmed, Z. U., Woodbury, P. B., Sanderman, J., Hawke, B., Jauss, V., Solomon, D., et al. (2017). Assessing soil carbon vulnerability in the Western USA by geospatial modeling of pyrogenic and particulate carbon stocks. J. Geophys. Res. Biogeosci. 122, 354–369. doi: 10.1002/2016JG003488
Alexis, M. A., Rasse, D. P., Rumpel, C., Bardoux, G., Pechot, N., Schmalzer, P., et al. (2007). Fire impact on C and N losses and charcoal production in a scrub oak ecosystem. Biogeochemistry 82, 201–216. doi: 10.1007/s10533-006-9063-1
Bao, H. Y., Niggemann, J., Luo, L., Dittmar, T., and Kao, S. J. (2017). Aerosols as a source of dissolved black carbon to the ocean. Nat. Commun. 8, 1–7. doi: 10.1038/s41467-017-00437-3
Birch, M. E. (1998). Analysis of carbonaceous aerosols: interlaboratory comparison. Analyst 123, 851–857. doi: 10.1039/a800028j
Bird, M. I., Wynn, J. G., Saiz, G., Wurster, C. M., and McBeath, A. (2015). The Pyrogenic Carbon Cycle. Annu. Rev. Earth Planet. Sci. Vol. 43, 273–298. doi: 10.1146/annurev-earth-060614-105038
Bond, T. C., Doherty, S. J., Fahey, D. W., Forster, P. M., Berntsen, T., DeAngelo, B. J., et al. (2013). Bounding the role of black carbon in the climate system: a scientific assessment. J. Geophys. Res. Atmospheres 118, 5380–5552. doi: 10.1002/jgrd.50171
Bond, T. C., Streets, D. G., Yarber, K. F., Nelson, S. M., Woo, J. H., and Klimont, Z. (2004). A technology-based global inventory of black and organic carbon emissions from combustion. J. Geophys. Res. 109:D14203. doi: 10.1029/2003JD003697
Bornemann, L., Welp, G., Brodowski, S., Rodionov, A., and Amelung, W. (2008). Rapid assessment of black carbon in soil organic matter using mid-infrared spectroscopy. Org. Geochem. 39, 1537–1544. doi: 10.1016/j.orggeochem.2008.07.012
Brodowski, S., Rodionov, A., Haumaier, L., Glaser, B., and Amelung, W. (2005). Revised black carbon assessment using benzene polycarboxylic acids. Org. Geochem. 36, 1299–1310. doi: 10.1016/j.orggeochem.2005.03.011
Camps, G. (1971). Study of prehistoric charcoal – preparation of thin sections and structural analysis – French - Couvert, M. Anthropologie 75, 282–283.
Cao, X., Pignatello, J. J., Li, Y., Lattao, C., Chappell, M. A., Chen, N., et al. (2012). Characterization of wood chars produced at different temperatures using advanced solid-state 13C NMR spectroscopic techniques. Energy Fuels 26, 5983–5991. doi: 10.1021/ef300947s
Chen, H. M., Abdulla, H. A. N., Sanders, R. L., Myneni, S. C. B., Mopper, K., and Hatcher, P. G. (2014). Production of black carbon-like and aliphatic molecules from terrestrial dissolved organic matter in the presence of sunlight and iron. Environ. Sci. Technol. Lett. 1, 399–404. doi: 10.1021/ez5002598
Cotrufo, M. F., Boot, C., Abiven, S., Foster, E. J., Haddix, M., Reisser, M., et al. (2016). Quantification of pyrogenic carbon in the environment: an integration of analytical approaches. Org. Geochem. 100, 42–50. doi: 10.1016/j.orggeochem.2016.07.007
Countess, R. J. (1990). Interlaboratory analysis of carbonaceous aerosol samples. Aerosol Sci. Technol. 12, 114–121. doi: 10.1080/02786829008959331
Crawford, A. J., and Belcher, C. M. (2016). Area-volume relationships for fossil charcoal and their relevance for fire history reconstruction. Holocene 26, 822–826. doi: 10.1177/0959683615618264
Currie, L. A., Benner, B. A. Jr., Kessler, J. D., Klinedinst, D. B., Klouda, G. A., Marolf, J. V., et al. (2002). A critical evaluation of interlaboratory data on total, elemental, and isotopic carbon in the carbonaceous particle reference material, NIST SRM 1649a. J. Natl. Inst. Stand. Technol. 107, 279–298. doi: 10.6028/jres.107.022
Cusack, D. F., Chadwick, O. A., Hockaday, W. C., and Vitousek, P. M. (2012). Mineralogical controls on soil black carbon preservation. Glob. Biogeochem. Cycle 26:GB2019. doi: 10.1029/2011GB004109
Decesari, S., Facchini, M. C., Fuzzi, S., and Tagliavini, E. (2000). Characterization of water-soluble organic compounds in atmospheric aerosol: a new approach. J. Geophys. Res. Atmos. 105, 1481–1489. doi: 10.1029/1999JD900950
Derenne, S., and Largeau, C. (2001). A review of some important families of refractory macromolecules: composition, origin, and fate in soils and sediments. Soil Sci. 166, 833–847. doi: 10.1097/00010694-200111000-00008
DiDonato, N., Chen, H. M., Waggoner, D., and Hatcher, P. G. (2016). Potential origin and formation for molecular components of humic acids in soils. Geochim. Cosmochim. Acta 178, 210–222. doi: 10.1016/j.gca.2016.01.013
Ding, Y., Cawley, K. M., da Cunha, C. N., and Jaffe, R. (2014). Environmental dynamics of dissolved black carbon in wetlands. Biogeochemistry 119, 259–273. doi: 10.1007/s10533-014-9964-3
Ding, Y., Yamashita, Y., Dodds, W. K., and Jaffe, R. (2013). Dissolved black carbon in grassland streams: is there an effect of recent fire history? Chemosphere 90, 2557–2562. doi: 10.1016/j.chemosphere.2012.10.098
Ding, Y., Yamashita, Y., Jones, J., and Jaffe, R. (2015). Dissolved black carbon in boreal forest and glacial rivers of central Alaska: assessment of biomass burning versus anthropogenic sources. Biogeochemistry 123, 15–25. doi: 10.1007/s10533-014-0050-7
Dittmar, T. (2008). The molecular level determination of black carbon in marine dissolved organic matter. Org. Geochem. 39, 396–407. doi: 10.1016/j.orggeochem.2008.01.015
Dittmar, T., de Rezende, C. E., Manecki, M., Niggemann, J., Ovalle, A. R. C., Stubbins, A., et al. (2012a). Continuous flux of dissolved black carbon from a vanished tropical forest biome. Nat. Geosci. 5, 618–622. doi: 10.1038/ngeo1541
Dittmar, T., Paeng, J., Gihring, T. M., Suryaputra, I., and Huettel, M. (2012b). Discharge of dissolved black carbon from a fire-affected intertidal system. Limnol. Oceanogr. 57, 1171–1181. doi: 10.4319/lo.2012.57.4.1171
Elias, V. O., Simoneit, B. R. T., Cordeiro, R. C., and Turcq, B. (2001). Evaluating levoglucosan as an indicator of biomass burning in Carajas, Amazonia: a comparison to the charcoal record. Geochim. Cosmochim. Acta 65, 267–272. doi: 10.1016/S0016-7037(00)00522-6
Elmquist, M., Cornelissen, G., Kukulska, Z., and Gustafsson, Ö. (2006). Distinct oxidative stabilities of char versus soot black carbon: implications for quantification and environmental recalcitrance. Glob. Biogeochem. Cycle 20:GB2009. doi: 10.1029/2005GB002629
Figueiral, I., and Mosbrugger, V. (2000). A review of charcoal analysis as a tool for assessing quaternary and tertiary environments: achievements and limits. Palaeogeogr. Palaeoclimatol. Palaeoecol. 164, 397–407. doi: 10.1016/S0031-0182(00)00195-4
Fliche, P. (1907). Note sur un charbon quaternaire de Chataignier. Bull. Soc. Bot. Fr. 54, 132–136. doi: 10.1080/00378941.1907.10831239
Flores-Cervantes, D. X., Plata, D. L., MacFarlane, J. K., Reddy, C. M., and Gschwend, P. M. (2009a). Black carbon in marine particulate organic carbon: inputs and cycling of highly recalcitrant organic carbon in the Gulf of Maine. Mar. Chem. 113, 172–181. doi: 10.1016/j.marchem.2009.01.012
Flores-Cervantes, D. X., Reddy, C. M., and Gschwend, P. M. (2009b). Inferring black carbon concentrations in particulate organic matter by observing pyrene fluorescence losses. Environ. Sci. Technol. 43, 4864–4870. doi: 10.1021/es900043c
Forbes, M. S., Raison, R. J., and Skjemstad, J. O. (2006). Formation, transformation and transport of black carbon (charcoal) in terrestrial and aquatic ecosystems. Sci. Total Environ. 370, 190–206. doi: 10.1016/j.scitotenv.2006.06.007
Glaser, B., and Amelung, W. (2003). Pyrogenic carbon in native grassland soils along a climosequence in North America. Glob. Biogeochem. Cycle 17, 1064. doi: 10.1029/2002GB002019
Glaser, B., Haumaier, L., Guggenberger, G., and Zech, W. (1998). Black carbon in soils: the use of benzenecarboxylic acid as specific markers. Org. Geochem. 29, 811–819. doi: 10.1016/S0146-6380(98)00194-6
Glaser, B., and Knorr, K. H. (2008). Isotopic evidence for condensed aromatics from non-pyrogenic sources in soils - implications for current methods for quantifying soil black carbon. Rapid Commun. Mass Spectrom. 22, 935–942. doi: 10.1002/rcm.3448
Godwin, H., and Tansley, A. G. (1941). Prehistoric charcoals as evidence of former vegetation, soil and climate. J. Ecol. 29, 117–126. doi: 10.2307/2256222
Goldberg, E. (1985). Black Carbon in the Environment: Properties and Distribution. New York, NY: John Wiley and Sons.
Guerena, D. T., Lehmann, J., Walter, T., Enders, A., Neufeldt, H., Odiwour, H., et al. (2015). Terrestrial pyrogenic carbon export to fluvial ecosystems: lessons learned from the White Nile watershed of East Africa. Glob. Biogeochem. Cycle 29, 1911–1928. doi: 10.1002/2015GB005095
Gundel, L. A., Dod, R. L., Rosen, H., and Novakov, T. (1984). The relationship between optical attenuation and black carbon concentration for ambient and source particles. Sci. Total Environ. 36, 197–202. doi: 10.1016/0048-9697(84)90266-3
Gustafsson, O., Bucheli, T. D., Kululska, M., Andersson, C., Largeau, C., Rouzard, J. N., et al. (2001). Evaluation of a protocol for the quantification of black carbon in sediments, soils, and aquatic sediments. Glob. Biogeochem. Cycle 15, 881–890. doi: 10.1029/2000GB001380
Gustafsson, O., Haghseta, F., Chan, C., MacFarlane, J., and Gschwend, P. M. (1997). Quantification of the dilute sedimentary soot phase: implications for PAH speciation and bioavailability. Environ. Sci. Technol. 31, 203–209. doi: 10.1021/es960317s
Hammes, K., Schmidt, M. W. I., Smernik, R. J., Currie, L. A., Ball, W. P., Nguyen, T. H., et al. (2007). Comparison of quantification methods to measure fire-derived (black/elemental) carbon in soils and sediments using reference materials from soil, water, sediment and the atmosphere. Glob. Biogeochem. Cycle 21:GB3016. doi: 10.1029/2006GB002914
Hartman, B. E., Chen, H. M., and Hatcher, P. G. (2015). A non-thermogenic source of black carbon in peat and coal. Int. J. Coal Geol. 144, 15–22. doi: 10.1016/j.coal.2015.03.011
Hedges, J. I., Eglinton, G., Hatcher, P. G., Kirchman, D. L., Arnosti, C., Derenne, S., et al. (2000). The molecularly-uncharacterized component of nonliving organci matter in natural environments. Org. Geochem. 31, 945–958. doi: 10.1016/S0146-6380(00)00096-6
Hedin, S. G. (1907). On extraction by casein of trypsin, adsorbed by charcoal. Biochem. J. 2, 81–88. doi: 10.1042/bj0020081
Heer, O. (1866). “Die Pflanzen der Pfahlbauten,” in Neujahrsblatt, Herausgegeben von der Naturforschende Gesellschaft Zürich, 1–54.
Hernes, P. J., and Hedges, J. I. (2000). Determination of condensed tannin monomers in environmental samples by capillary gas chromatography of acid depolymerization extracts. Anal. Chem. 72, 5115–5124. doi: 10.1021/ac991301y
Herring, J. R. (1976). Cenozoic charcoal flux increase to North Pacific sediments. Trans. Am. Geophys. Union 57, 259–259.
Hilscher, A., and Knicker, H. (2011). Degradation of grass-derived pyrogenic organic material, transport of the residues within a soil column and distribution in soil organic matter fractions during a 28 month microcosm experiment. Org. Geochem. 42, 42–54. doi: 10.1016/j.orggeochem.2010.10.005
Hitzenberger, R., Jennings, S. G., Larson, S. M., Dillner, A., Cachier, H., Galambos, Z., et al. (1999). Intercomparison of measurement methods for black carbon aerosols. Atmos. Environ. 33, 2823–2833. doi: 10.1016/S1352-2310(98)00360-4
Hitzenberger, R., Petzold, A., Bauer, H., Ctyroky, P., Pouresmaeil, P., Laskus, L., et al. (2006). Intercomparison of thermal and optical measurement methods for elemental carbon and black carbon at an urban location. Environ. Sci. Technol. 40, 6377–6383. doi: 10.1021/es051228v
Homfray, I. F. (1910). On the absorption of gases by charcoal. Proc. Royal Soc. Lond. Ser. A Contain. Pap. Math. Phys. Character 84, 99–106. doi: 10.1098/rspa.1910.0060
Jaffe, R., Ding, Y., Niggemann, J., Vahatalo, A. V., Stubbins, A., Spencer, R. G. M., et al. (2013). Global charcoal mobilization from soils via dissolution and riverine transport to the oceans. Science 340, 345–347. doi: 10.1126/science.1231476
Janik, L. J., Skjemstad, J. O., Shepherd, K. D., and Spouncer, L. R. (2007). The prediction of soil carbon fractions using mid-infrared-partial least square analysis. Aust. J. Soil Res. 45, 73–81. doi: 10.1071/SR06083
Jauss, V., Johnson, M., Krull, E., Daub, M., and Lehmann, J. (2015). Pyrogenic carbon controls across a soil catena in the Pacific Northwest. Catena 124, 53–59. doi: 10.1016/j.catena.2014.09.001
Jien, S.-H., and Wang, C.-S. (2013). Effects of biochar on soil properties and erosion potential in a highly weathered soil. Catena 110, 225–233. doi: 10.1016/j.catena.2013.06.021
Kappenberg, A., Blaesing, M., Lehndorff, E., and Amelung, W. (2016). Black carbon assessment using benzene polycarboxylic acids: limitations for organic-rich matrices. Org. Geochem. 94, 47–51. doi: 10.1016/j.orggeochem.2016.01.009
Kasozi, G. N., Zimmerman, A. R., Nkedi-Kizza, P., and Gao, B. (2010). Catechol and humic acid sorption onto a range of laboratory-produced black carbons (biochars). Environ. Sci. Technol. 44, 6189–6195. doi: 10.1021/es1014423
Kirchstetter, T. W., and Novakov, T. (2007). Controlled generation of black carbon particles from a diffusion flame and applications in evaluating black carbon measurement methods. Atmos. Environ. 41, 1874–1888. doi: 10.1016/j.atmosenv.2006.10.067
Knicker, H., Hilscher, A., Gonzalez-Vila, F. J., and Almendros, G. (2008). A new conceptual model for the structural properties of char produced during vegetation fires. Org. Geochem. 39, 935–939. doi: 10.1016/j.orggeochem.2008.03.021
Knicker, H., Muffler, P., and Hilscher, A. (2007). How useful is chemical oxidation with dichromate for the determination of “black carbon” in fire-affected soils? Geoderma 142, 178–196. doi: 10.1016/j.geoderma.2007.08.010
Knicker, H., Totsche, K. U., Almendros, G., and Gonzalez-Vila, F. J. (2005). Condensation degree of burnt peat and plant residues and the reliability of solid-state VACP MAS C-13 NMR spectra obtained from pyrogenic humic material. Org. Geochem. 36, 1359–1377. doi: 10.1016/j.orggeochem.2005.06.006
Kuhlbusch, T., and Crutzen, P. (1996). “Black carbon, the global carbon cycle, and atmospheric carbon dioxide,” in Biomass Burning and Global Change, ed J. Levine (Cambridge, MA: The MIT Press), Chapter 16, 160–169.
Kumari, K., Moldrup, P., Paradelo, M., Elsgaard, L., and de Jonge, L. W. (2017). Effects of biochar on dispersibility of colloids in agricultural soils. J. Environ. Qual. 46, 143–152. doi: 10.2134/jeq2016.08.0290
Kuo, L. J., Herbert, B. E., and Louchouarn, P. (2008). Can levoglucosan be used to characterize and quantify char/charcoal black carbon in environmental media? Org. Geochem. 39, 1466–1478. doi: 10.1016/j.orggeochem.2008.04.026
Lehmann, J. (2007a). Bio-energy in the black. Front. Ecol. Environ. 5, 381–387. doi: 10.1890/1540-9295(2007)5[381:BITB]2.0.CO;2
Lehmann, J., and Joseph, S. (2015). “Biochar for environmental management: an introduction,” in Environmental Management: Science, Technology and Implementation, eds J. Lehmann, and S. Joseph (London: Earthscan), 235.
Lehmann, J., da Silva, J. P. Jr., Rondon, M., Cravo, M., Greenwood, J., Hehls, B., et al. (2002). “Slash and char: a feasible alternative for soil fertility management in the central amazon?” in 17th World Conference of Soil Science, ed S. T1UoS (Bangkok: International Union of Soil Sciences), 449, 1–12.
Li, W. B., Hou, Y. C., Yang, F., and Wu, W. Z. (2017). Production of benzene carboxylic acids and small-molecule fatty acids from lignite by two-stage alkali-oxygen. Ind. Eng. Chem. Res. 56, 1971–1978. doi: 10.1021/acs.iecr.6b04562
Lim, B., and Cachier, H. (1996). Determination of black carbon by chemical oxidation and thermal treatment in recent marine and lake sediments and cretaceous-tertiary clays. Chem. Geol. 131, 143–154. doi: 10.1016/0009-2541(96)00031-9
Masiello, C. A. (2004). New directions in black carbon organic geochemistry. Mar. Chem. 92, 201–213. doi: 10.1016/j.marchem.2004.06.043
Mayol-Bracero, O. L., Gabriel, R., Andreae, M. O., Kirchstetter, T. W., Novakov, T., Ogren, J., et al. (2002). Carbonaceous aerosols over the Indian ocean during the Indian ocean experiment (INDOEX): chemical characterization, optical properties, and probable sources. J. Geophys. Res. 107, 8030. doi: 10.1029/2000JD000039
McBeath, A. V., Smernik, R. J., Schneider, M. P. W., Schmidt, M. W. I., and Plant, E. L. (2011). Determination of the aromaticity and the degree of aromatic condensation of a thermosequence of wood charcoal using NMR. Org. Geochem. 42, 1194–1202. doi: 10.1016/j.orggeochem.2011.08.008
Meredith, W., Ascough, P. L., Bird, M. I., Large, D. J., Snape, C. E., Sun, Y., et al. (2012). Assessment of hydropyrolysis as a method for the quantification of black carbon using standard reference materials. Geochim. Cosmochim. Acta 97, 131–147. doi: 10.1016/j.gca.2012.08.037
Nelson, P. N., and Baldock, J. A. (2005). Estimating the molecular composition of a diverse range of natural organic materials from solid-state 13C NMR and elemental analyses. Biogeochemistry 72, 1–34. doi: 10.1007/s10533-004-0076-3
Nguyen, B. T., and Lehmann, J. (2009). Black carbon decomposition under varying water regimes. Org. Geochem. 40, 846–853. doi: 10.1016/j.orggeochem.2009.05.004
Novakov, T. (1981). “Microchemical characterization of aerosols,” in Proceedings of the 8th International Microchemical Symposium, eds H. Malissa, M. Grasserbauer, and R. Belcher (Graz: Springer), 141–165.
Novakov, T. (1984). The role of soot and primary oxidants in atmospheric chemistry. Sci. Total Environ. 36, 1–10. doi: 10.1016/0048-9697(84)90241-9
Novakov, T., and Rosen, H. (2013). The black carbon story: early history and new perspectives. Ambio 42, 840–851. doi: 10.1007/s13280-013-0392-8
Paetsch, L., Mueller, C. W., Rumpel, C., Angst, Š., Wiesheu, A. C., Girardin, C., et al. (2017). A multi-technique approach to assess the fate of biochar in soil and to quantify its effect on soil organic matter composition. Organ. Geochem. 112, 177–186. doi: 10.1016/j.orggeochem.2017.06.012
Preston, C. M., and Schmidt, M. W. I. (2006). Black (pyrogenic) carbon: a synthesis of current knowledge and uncertainties with special consideration of boreal regions. Biogeosciences 3, 397–420. doi: 10.5194/bg-3-397-2006
Qi, F. J., Naidu, R., Bolan, N. S., Dong, Z. M., Yan, Y. B., Lamb, D., et al. (2017). Pyrogenic carbon in Australian soils. Sci. Total Environ. 586, 849–857. doi: 10.1016/j.scitotenv.2017.02.064
Reisser, M., Purves, R. S., Schmidt, M. W. I., and Abiven, S. (2016). Pyrogenic carbon in soils: a literature-based inventory and a global estimation of its content in soil organic carbon and stocks. Front. Earth Sci. 4:80. doi: 10.3389/feart.2016.00080
Rosen, H., and Novakov, T. (1977). Raman scattering and the characterisation of atmospheric aerosol particles. Nature 266, 708–710. doi: 10.1038/266708a0
Rumpel, C., Ba, A., Darboux, F., Chaplot, V., and Planchon, O. (2009). Erosion budget and process selectivity of black carbon at meter scale. Geoderma 154, 131–137. doi: 10.1016/j.geoderma.2009.10.006
Santin, C., Doerr, S. H., Kane, E. S., Masiello, C. A., Ohlson, M., de la Rosa, J. M., et al. (2016). Towards a global assessment of pyrogenic carbon from vegetation fires. Glob. Chang. Biol. 22, 76–91. doi: 10.1111/gcb.12985
Schmid, H., Laskus, L., Abraham, H. J., Baltensperger, U., Lavanchy, V., Bizjak, M., et al. (2001). Results of the “carbon conference” international aerosol carbon round robin test stage I. Atmos. Environ. 35, 2111–2121. doi: 10.1016/S1352-2310(00)00493-3
Schmidt, M. W. I., Masiello, C. A., and Skjemstad, J. O. (2003). Final recommendations for reference materials in black carbon analysis. Eos Trans. AGU 84, 582–583. doi: 10.1029/2003EO520006
Schmidt, M. W. I., and Noack, A. G. (2000). Black carbon in soils and sediments: analysis, distribution, implications, and current challenges. Glob. Biogeochem. Cycle 14, 777–793. doi: 10.1029/1999GB001208
Schmidt, M. W. I., Skjemstad, J. O., Czimczik, C. I., Glaser, B., Prentice, K. M., Gelinas, Y., et al. (2001). Comparative analysis of black carbon in soils. Glob. Biogeochem. Cycle 15, 163–167. doi: 10.1029/2000GB001284
Schopf, J. M. (1975). Modes of fossil preservation. Rev. Palaeobot. Palynol. 20, 27–53. doi: 10.1016/0034-6667(75)90005-6
Scott, A. C., and Damblon, F. (2010). Charcoal: taphonomy and significance in geology, botany and archaeology. Palaeogeogr. Palaeoclimatol. Palaeoecol. 291, 1–10. doi: 10.1016/j.palaeo.2010.03.044
Seiler, W., and Crutzen, P. J. (1980). Estimates of gross and net fluxes of carbon between the biosphere and the atmosphere from biomass burning. Clim. Change 2, 207–247. doi: 10.1007/BF00137988
Simpson, M. J., and Hatcher, P. G. (2004). Determination of black carbon in natural organic matter by chemical oxidation and solid-state 13C nuclear magnetic resonance spectroscopy. Org. Geochem. 35, 923–935. doi: 10.1016/j.orggeochem.2004.04.004
Singh, N., Abiven, S., Torn, M. S., and Schmidt, M. W. I. (2012). Fire-derived organic carbon in soil turns over on a centennial scale. Biogeosciences 9, 2847–2857. doi: 10.5194/bg-9-2847-2012
Smith, D. M., Griffin, J. J., and Goldberg, E. D. (1973). Elemental carbon in marine sediments - baseline for burning. Nature 241, 268–270. doi: 10.1038/241268a0
Smith, D. M., Griffin, J. J., and Goldberg, E. D. (1975). Spectrometric method for the quantitative determination of elemental carbon. Anal. Chem. 47, 233–238. doi: 10.1021/ac60352a032
Stubbins, A., Hood, E., Raymond, P. A., Aiken, G. R., Sleighter, R. L., Hernes, P. J., et al. (2012). Anthropogenic aerosols as a source of ancient dissolved organic matter in glaciers. Nat. Geosci. 5, 198–201. doi: 10.1038/ngeo1403
Stubbins, A., Spencer, R. G. M., Chen, H. M., Hatcher, P. G., Mopper, K., Hernes, P. J., et al. (2010). Illuminated darkness: molecular signatures of Congo river dissolved organic matter and its photochemical alteration as revealed by ultrahigh precision mass spectrometry. Limnol. Oceanogr. 55, 1467–1477. doi: 10.4319/lo.2010.55.4.1467
Swain, A. M. (1978). Environmental changes during the past 2000 years in north-central Wisconsin: analysis of pollen, charcoal, and seeds from varved lake sediments. Quat. Res. 10, 55–68. doi: 10.1016/0033-5894(78)90013-3
Sweetser, R. H. (1908). Charcoal and coke as blast-furnace fuels. Trans. Am. Inst. Min. Metall. Eng. 39, 228–235.
ten Brink, H., Maenhaut, W., Hitzenberger, R., Gnauk, T., Spindler, G., Even, A., et al. (2004). INTERCOMP2000: the comparability of methods in use in Europe for measuring the carbon content of aerosol. Atmos. Environ. 38, 6507–6519. doi: 10.1016/j.atmosenv.2004.08.027
Thevenon, F., Williamson, D., Bard, E., Anselmetti, F. S., Beaufort, L., and Cachier, H. (2010). Combining charcoal and elemental black carbon analysis in sedimentary archives: implications for past fire regimes, the pyrogenic carbon cycle, and the human-climate interactions. Glob. Planet. Change 72, 381–389. doi: 10.1016/j.gloplacha.2010.01.014
Thomas, M. D. (1952). The present status of the development of instrumentation from the study of air pollution. Proc. Natl. Air Pollut. Sympos. 2, 16–23.
van Leeuwen, T. T., van der Werf, G. R., Hoffmann, A. A., Detmers, R. G., Rucker, G., French, N. H. F., et al. (2014). Biomass burning fuel consumption rates: a field measurement database. Biogeosciences 11, 7305–7329. doi: 10.5194/bg-11-7305-2014
Waggoner, D. C., Chen, H. M., Willoughby, A. S., and Hatcher, P. G. (2015). Formation of black carbon-like and alicyclic aliphatic compounds by hydroxyl radical initiated degradation of lignin. Org. Geochem. 82, 69–76. doi: 10.1016/j.orggeochem.2015.02.007
Wagner, S., Ding, Y., and Jaffé, R. (2017). A new perspective on the apparent solubility of dissolved black carbon. Front. Earth Sci. 5:75. doi: 10.3389/feart.2017.00075
Wagner, S., Riedel, T., Niggemann, J., Vähätalo, A. V., Dittmar, T., and Jaffé, R. (2015). Linking the molecular signature of heteroatomic dissolved organic matter to watershed characteristics in world rivers. Environ. Sci. Technol. 49, 13798–13806. doi: 10.1021/acs.est.5b00525
Wang, D. Y., Fonte, S. J., Parikh, S. J., Six, J., and Scow, K. M. (2017). Biochar additions can enhance soil structure and the physical stabilization of C in aggregates. Geoderma 303, 110–117. doi: 10.1016/j.geoderma.2017.05.027
Wang, W. H., Hou, Y. C., Wu, W. Z., Niu, M. G., and Liu, W. N. (2012). Production of benzene polycarboxylic acids from lignite by alkali-oxygen oxidation. Ind. Eng. Chem. Res. 51, 14994–15003. doi: 10.1021/ie3021297
Watson, J. G., Chow, J. C., and Chen, L. W. A. (2005). Summary of organic and elemental carbon/black carbon analysis methods and intercomparisons. Aerosol Air Qual. Res. 5, 65–102. doi: 10.4209/aaqr.2005.06.0006
Western, A. C. (1963). “Wood and charcoal in archaeology,” in Science in Archaeology: A Comprehensive Survey of Progress and Research, eds D. Brothwell and E. Higgs (London: Thames and Hudson), 150–158.
Wilkins, E. T. (1954). Air pollution aspects of the London fog of december 1952-discussion. Q. J. Royal Meteorol. Soc. 80, 267–271. doi: 10.1002/qj.49708034420
Yoshioka, H., and Ishiwatari, R. (2005). An improved ruthenium tetroxide oxidation of marine and lacustrine kerogens: possible origin of low molecular weight acids and benzenecarboxylic acids. Org. Geochem. 36, 83–94. doi: 10.1016/j.orggeochem.2004.07.002
Zand, A. D., and Grathwohl, P. (2016). Enhanced immobilization of polycyclic aromatic hydrocarbons in contaminated soil using forest wood-derived biochar and activated carbon under saturated conditions, and the importance of biochar particle size. Pol. J. Environ. Stud. 25, 427–441. doi: 10.15244/pjoes/60160
Zimmerman, A. (2010). Abiotic and microbial oxidation of laboratory-produced black carbon (biochar). Environ. Sci. Technol. 44, 1295–1301. doi: 10.1021/es903140c
Zimmerman, A. R., Gao, B., and Ahn, M.-Y. (2011). Positive and negative carbon mineralization priming effects among a variety of biochar-amended soils. Soil Biol. Biochem. 43, 1169–1179. doi: 10.1016/j.soilbio.2011.02.005
Ziolkowski, L. A., Chamberlin, A. R., Greaves, J., and Druffel, E. R. M. (2011). Quantification of black carbon in marine systems using the benzene polycarboxylic acid method: a mechanistic and yield study. Limnol. Oceanogr. Methods 9, 140–149. doi: 10.4319/lom.2011.9.140
Keywords: pyrogenic carbon, black carbon, quantification, artifacts, biochar, ring trial
Citation: Zimmerman AR and Mitra S (2017) Trial by Fire: On the Terminology and Methods Used in Pyrogenic Organic Carbon Research. Front. Earth Sci. 5:95. doi: 10.3389/feart.2017.00095
Received: 10 August 2017; Accepted: 06 November 2017;
Published: 17 November 2017.
Edited by:
Cristina Santin, Swansea University, United KingdomReviewed by:
Michael W. I. Schmidt, University of Zurich, SwitzerlandPhilippa Louise Ascough, Scottish Universities Environmental Research Centre, United Kingdom
Michael Ian Bird, James Cook University Cairns, Australia
Copyright © 2017 Zimmerman and Mitra. This is an open-access article distributed under the terms of the Creative Commons Attribution License (CC BY). The use, distribution or reproduction in other forums is permitted, provided the original author(s) or licensor are credited and that the original publication in this journal is cited, in accordance with accepted academic practice. No use, distribution or reproduction is permitted which does not comply with these terms.
*Correspondence: Andrew R. Zimmerman, YXppbW1lckB1ZmwuZWR1