- 1Department of Forestry, Michigan State University, East Lansing, MI, United States
- 2Department of Chemistry and Biochemistry, Southeast Environmental Research Center, Florida International University, North Miami, FL, United States
Inventories of fire-derived (pyrogenic) C (PyC) stocks in soils remain incomplete for many parts of the world, yet are critical to reduce uncertainties in global PyC estimates. Additionally, PyC dynamics in soils remain poorly understood. For example, dissolved PyC (DPyC) fluxes from soil horizons, as well as the influence of historical fire events on these fluxes and soil PyC stocks remain poorly quantified. In this study, we examined stock and concentration differences in soil PyC and leached DPyC, respectively, between two forest types in the Great Lakes region (USA): (1) a red pine (Pinus resinosa) forest planted after the site had experienced post-logging slash burning in the late nineteenth century (100 year-burned site), and (2) a sugar maple (Acer saccharum) forest that showed no evidence of burning in the past 250 years (unburned site). We hypothesized that the 100 year-burned site would have greater PyC stocks and concentrations of DPyC compared to the unburned site. We measured PyC in soil, as well as DPyC in soil water leaching from O and E horizons following a spring snowmelt event in both 100 year-burned and unburned sites. Additionally, we measured DPyC drained from B horizons in 100 year-burned site. In organic horizons, PyC stocks were 1.8 (Oi) and 2.3 (Oe) times greater in the 100 year-burned site than in the unburned site. Contrary to our initial hypothesis, DPyC concentrations did not differ between sites. On average, DPyC leached from all sites contributed 3.11 ± 0.27% of the total dissolved organic carbon pool. In the 100 year-burned site, a significant decline in concentrations of DPyC leaving the B horizon was attributed to the immobilization of this C pool in the Al and Fe oxides-rich subsoil. Even though PyC stock in O horizons was higher in 100 year-burned than in unburned site, our results did not support our initial hypothesis that the 100 year-burned site would have greater DPyC concentrations than the unburned site, suggesting that any differences in DPyC resulting from a single fire event are either not detectable after >100 years post-burn, and/or that the release of DPyC is a continuous, long-term process resulting from the degradation of historically accumulated PyC.
Introduction
Pyrogenic carbon (PyC) is a thermally-altered form of organic carbon (C) produced during fires (Goldberg, 1985; Bird et al., 2015). Interest in understanding the importance of PyC to the global soil C dynamics has increased in part because PyC is thought to contribute to long-term C sequestration in soils, owed to its apparent stability in soils on decades to millennial residence time scales (Santos et al., 2012; Singh et al., 2012; Fang et al., 2014). Global storage of soil PyC has been estimated at 200 Pg (uppermost 2 m), with PyC accounting for up to 60% of soil organic C worldwide (Forbes et al., 2006; Reisser et al., 2016). Limited data on PyC stocks and dynamics in many ecosystems and subsoil horizons, however, still hinder a better understanding of PyC stabilization processes and accurate estimations of the global PyC budget. Despite the apparent stability of PyC in soils, there is growing evidence that a fraction of PyC is subjected to redistribution in the landscape and lost from soils via erosion (Rumpel et al., 2006, 2009; Boot et al., 2015; Güereña et al., 2015; Cotrufo et al., 2016) and can be vertically transported to lower soil depths via translocation (Dai et al., 2005; Leifeld et al., 2007) and leaching (Hockaday et al., 2006, 2007; Major et al., 2010). This suggests that PyC is much more dynamic in soils than previously thought. For example, large fluxes of dissolved PyC (DPyC, <0.7 μm particle size) in a tropical river were reported to increase during heavy rain and derive from charcoal remaining in surface soils from historical fires rather than from recent burning (Marques et al., 2017). Thus, quantifying the relative impact of historical fires on PyC stocks and export from soils is essential to understand the impact of fires on PyC stabilization in and mobilization mechanisms from soils.
Although much of the existing soil PyC stocks data reported in the literature is restricted to the uppermost 30 cm depth (Bird et al., 2015), a significant proportion of PyC could be stored below 30 cm depth (subsoils) in forest ecosystems. Subsoils have been reported to store up to 63% of the total C stored in soils worldwide (Batjes, 1996; Jobbagy and Jackson, 2000), but only few studies have reported PyC stocks in lower soil horizons of fire-impacted forest ecosystems (Czimczik et al., 2005; Soucémarianadin et al., 2014; Jauss et al., 2017; Koele et al., 2017). For example, in a Canadian boreal forest, 12–46% of the total PyC stock in podzols was found in the entire mineral horizon (Ae, Bf, Bfc, BC, and C) (Soucémarianadin et al., 2014). In a study conducted in a boreal forest in Siberia, on the other hand, PyC in mineral soils (depth >1 m) contributed very little (<1%) to the total stocks in podzols (Czimczik et al., 2005). In a recent inventory of PyC in Amazon basin forest soils, 50% of PyC in the soil profile was measured at depths ranging from 44 to 71 cm (Koele et al., 2017). Incomplete available datasets on PyC pools for the entire soil profile limit accurate estimations of the global PyC budget and the assessment of how fire impacts PyC storage in deep soils.
PyC loss from soils via leaching is one of the major gaps in PyC research (Bird et al., 2015; Santín et al., 2016), limiting our understanding of PyC transport pathways across ecosystems and the role of PyC in the global C cycle (Santín et al., 2016). In aquatic systems, DPyC is typically reported as a measure of dissolved poly-condensed aromatic C (Dittmar, 2008) and has been shown to correlate with dissolved organic carbon (DOC) (Ding et al., 2013, 2014; Jaffé et al., 2013; Güereña et al., 2015). Few studies have examined the export pathways for the transport of DPyC from terrestrial to aquatic ecosystems (Güereña et al., 2015; Cotrufo et al., 2016; Marques et al., 2017). While a study conducted in mixed tropical highland forest and agricultural catchments dominated by Nitisols showed that subsurface transport was more important than erosion in driving the movement of DPyC from soils to streams (Güereña et al., 2015), a study conducted in a savanna Oxisol showed that leaching accounted for less than 1% of the annual DPyC losses (detected based on 13C stable isotope approaches) one and two years after the addition of charcoal to tropical soil (Major et al., 2010). No study, however, has reported fluxes of DPyC from organic and mineral soil horizons in temperate ecosystems, limiting our understanding of PyC contribution to C stabilization in subsoils and mobilization of DPyC to aquatic systems. Moreover, the role of historical fire events in controlling the fluxes of terrestrially-derived DPyC to aquatic ecosystems remains unclear. Accurate estimations of DPyC fluxes from soils and a clearer picture of the role of historical fires in driving these fluxes are essential to understand PyC dynamics in soils, and to predict the impact of fires on PyC export from soils to aquatic systems.
Although it would be plausible to expect that recent fires would be the major factor driving the export of DPyC from land to aquatic systems, recent studies do not support this hypothesis (Dittmar et al., 2012; Ding et al., 2013; Myers-Pigg et al., 2015; Wagner et al., 2015). For example, there was no correlation between DPyC concentrations in streams and fire frequency in the grassland ecosystem watersheds in Kansas (Ding et al., 2013). In another study, DPyC fluxes in burned watersheds were not greater than those in unburned sites when measured 1 year after a wildfire event in Colorado (Wagner et al., 2015). The difficulty in linking DPyC with fire events in watersheds suggests that other variables interact to drive the transport of DPyC from soils to fluvial systems, including fire characteristics (i.e., size, severity, and intensity), soil physical and chemical properties, landscape geomorphology, ecosystem type, the time elapsed since the fire event, and hydrology (Cotrufo et al., 2016; Reisser et al., 2016). Despite evidence for the uncoupled relationship between DPyC in rivers and forest fires, no study has yet investigated the direct impact of a single fire event on DPyC leached from different soil horizons in temperate forest ecosystems.
The concentration of DPyC in soil solution percolating through the soil system could be influenced by the amount of PyC stored in organic horizons and formation of chemical associations with reactive minerals in subsoils. In forest soils, organic horizons are the major source of DOC moving downward to lower soil depths, where part of the DOC pool can be retained due to its chemical associations with mineral surfaces (Kaiser and Guggenberger, 2000; Kalbitz et al., 2000; Kleber et al., 2015). Whether PyC stocks in topsoil (above 30 cm depth) influences DPyC concentrations in forest soils impacted by a historic fire remains largely unknown, but could provide insights on the mechanisms driving the vertical downward mobilization of DPyC.
Here, we investigated how a historical fire in the Upper Peninsula of Michigan (USA) influenced stocks of PyC and its downward movement through the soil profile as DPyC. In this study, we report PyC stocks in the area of the Upper Peninsula of Michigan that either burned, or did not burn, in the extensive post-logging fires associated with Euro-American settlement of this region in the late nineteenth century (Whitney, 1987). Additionally, we explored the relative importance of charcoal stocks on the fluxes of DPyC leached from soils by taking advantage of the experimental sites mentioned above. These site characteristics present unique opportunities to: (1) quantify the stocks of PyC, as well as concentrations of DPyC leached through different soil horizons and (2) determine whether soils with a charcoal layer >100 years old would (a) result in higher PyC stocks in both organic and mineral soils; and (b) release more DPyC into soil leachates than forest soils that had not burned for at least the past 250 years. We hypothesized that PyC stocks and DPyC concentrations would be greater in sites with a history of more recent fire (hereafter referred to as 100 year-burned sites), compared to sites that have remained unburned for at least two centuries (hereafter referred to as unburned sites).
Materials and Methods
Field Sites and Experimental Design
Our study was located in the eastern Upper Peninsula of Michigan (USA, 46°19′N, 85°02′W, Figure 1A), in adjacent areas representing two contrasting forest cover types: red pine (Pinus resinosa) forests planted in the 1930s, and naturally regenerated (i.e., unplanted) sugar-maple (Acer saccharum) forests. The study area has been described in detail by Schaetzl and Rothstein (2016). General Land Office (GLO) survey data from 1850 show that pre-settlement vegetation of all our study sites consisted of mature beech (Fagus grandifolia)—sugar maple—hemlock (Tsuga canadensis) forest, which was harvested during the late nineteenth to early twentieth century (Comer et al., 1995). GLO survey records of the same area from 1938, together with the presence of a distinct charcoal layer (<0.5 cm thick) in the red pine forest sites, clearly indicate that areas planted to red pine in the 1930s experienced severe slash fires during the logging era, prior to the establishment of the red pine plantations. In contrast, there is no visible charcoal in the sugar maple sites, and no indication of burning in the GLO records for at least the past 250 years. Thus, we highlight that these two different forest types (planted and unplanted) developed after the time of these major disturbances. Three distinct stands were selected for each forest type. In this paper, we refer to red pine and sugar maple sites as “100 year-burned” and “unburned,” respectively. The sites are characterized by a mean annual temperature and precipitation of 4.7°C and 812 mm, respectively. The soils are strongly developed, well-drained spodosols, developed on deep outwash sands and classified as sandy, isotic, frigid Typic Haplorthods (Schaetzl et al., 2015; Schaetzl and Rothstein, 2016). Pictures of the vertical soil profiles typically found in the sites are shown in Figures 1B,C.
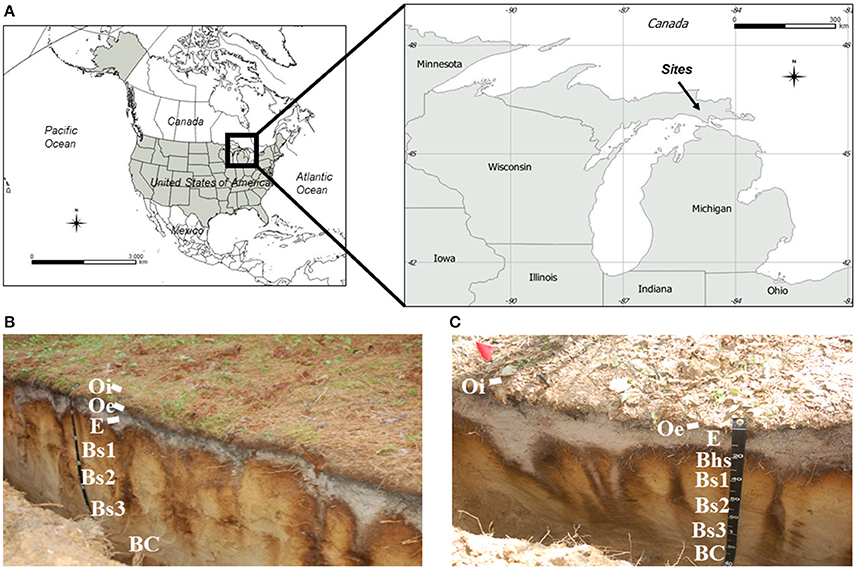
Figure 1. Map showing the location of the sites in the eastern Upper Peninsula of Michigan (USA), (A) and schematic figures of soil vertical profiles typically found in 100-year burned (B) and unburned sites (C). Spodic horizons: Bs, sesquioxides of Al and Fe; and Bhs, mixture of Bs and Bh (organic matter accumulation).
PyC Measurements
PyC in Organic and Mineral Soils
Zero-tension lysimeters (ZTLs) were installed underneath each soil horizon (O, E, and B), as described in Schaetzl and Rothstein (2016). Organic (Oi and Oe) and mineral (E and B) soil horizons overlying the ZTLs were sampled from nine pits per forest type, totaling 18 pits. Organic horizons were collected within a 930 cm2 diameter sampling frame. All soil samples were air-dried, and mineral soils were sieved to 2-mm particle-size. Prior to analyses, samples were dried to constant mass at 60°C and ball milled. Total C content was determined by dry combustion using an elemental analyzer (Costech Analytical Technologies, Inc., Valencia, CA).
To examine changes in PyC stocks in soils with depth, PyC in soil horizons overlying and underneath the ZTLs was measured using a thermo-chemical digestion method (Kurth, MacKenzie and DeLuca, KMD) described by Kurth et al. (2006) and later modified for organic samples (Maestrini and Miesel, 2016). Briefly, 1 g of soil along with 10 mL of 1 M nitric acid and 20 mL of 30% hydrogen peroxide were added to glass tubes and digested in an aluminum block heater at 100°C for 16 h. For organic samples, pulverized quartz sand was mixed with the sample to achieve a 10% mass ratio (Maestrini and Miesel, 2016). After the digestion, the samples were cooled and vacuum-filtered through glass funnels lined with pre-weighed Whatman filter paper (grade 2) to recover the post-digested fine soil material. Filters with digested materials were dried at 60°C for 24 h and weighed to determine dry mass. The digested materials were separated from the filters and re-homogenized prior to analyzing for total C by dry combustion. PyC was assumed to comprise the total OC in the digested material, although we acknowledge that the KMD method can overestimate PyC in non-pyrolyzed organic samples by ~5% (Maestrini and Miesel, 2016). We used PyC concentration (Table S1), litter mass, and sampling area data to calculate PyC stocks (g m−2) in the O horizon. In the unburned site, one of the Oe horizons was not measured for PyC (missing sample). Additionally, in most cases PyC in BC horizons was not reported due to low C detection. To calculate stocks (g PyC m−2) in the mineral horizon, we used PyC concentration, bulk density, and thickness of the soil horizon. In the 100 year-burned site, one sample from the E horizon was identified as an outlier and removed from the analysis. Because lysimeters were specifically placed underneath the upper B horizon, we report soil PyC stocks for both upper and lower B horizons in the two sites.
To provide information on the quality of PyC in Oi and Oe horizons, which we assume to be the main sources of DPyC entering in the soil system, we limited our sample size to three pits per forest type (totaling 6 pits). We used benzene polycarboxylic acids (BPCAs) as specific markers for PyC as described by Wiedemeier et al. (2013), and used the equivalent of 0.5 mg OC per sample as recommended by Kappenberg et al. (2016). Briefly, the ball-milled sample was digested at 170°C under pressure for 8 h in 70% nitric acid. The digested solution was filtered and phthalic acid was added to the solution as an internal check standard. The filtrate was cleaned in cation exchange resins, freeze-dried and subsequently redissolved in HPLC-grade water. These clean-up steps are required to remove metals and other interfering compounds from soil-derived BPCAs prior to analysis (Wiedemeier et al., 2013). The cation exchange procedure results in very small losses of BPCAs which do not impact overall data trends (Wiedemeier et al., 2013). Individual BPCAs were separated from the redissolved solution using a reversed stationary phase column using standard gradient conditions and quantified using multiple-point calibration with external standard solutions of BPCAs. PyC contents in these samples are reported as a sum of individual BPCA monomers (B6CA, B5CA, B4CA, and B3CA), each representing a BPCA with a particular number of carboxylic groups (e.g., B3CA represents BPCA with three carboxylic groups). The relative proportion of each individual BPCA reflects the level of PyC aromatic condensation, which mirrors the size of the original polycyclic structures (Schmidt et al., 2017). The amount of PyC as BPCAs in those soils (Table S2), as well as the distribution of each individual BPCA in soil PyC (Figure S1) are reported in the Supplemental Material.
PyC in Soil Solution
On April 28th, 2014, we sampled soil solution from three stands per forest type, within an area of approximately 17 km2. Leachates drained from the O, E, and B horizons were collected in pre-cleaned bottles and stored at −20°C until further analysis. Although the entire site had 54 ZTLs installed, we collected soil leachates from 18 ZTLs that had not been affected by a manipulation experiment out of the scope of this study. The concentration of dissolved organic C (DOC, mg L−1) was measured on a TOC-Vcpn analyzer (Shimadzu Corp., Kyoto, Japan). PyC in leachate was measured using the BPCA method (Dittmar, 2008; Ding et al., 2013), which may slightly overestimate DPyC (Wagner et al., 2017). Here, we report DPyC data in O and E horizons for both 100 year-burned and unburned sites. Due to lack of replication, we only report DPyC data in the B horizon for the 100 year-burned site. Briefly, we filtered leachates through pre-combusted (450°C, 5 h) fiberglass filters (Whatman GF/F 0.7 μm pore size) and extracted dissolved organic matter (DOM) by solid-phase extraction (SPE, 5 g Bond Elut PPL, Agilent, Dittmar, 2008; Dittmar et al., 2008) by passing samples through pre-rinsed (with MeOH) SPE cartridges. The cartridges were dried under N2, after which DOM was eluted with 20 mL MeOH. Aliquots of the MeOH-extracted DOM were transferred to 2 mL glass ampules and dried under N2 until MeOH was completely evaporated. Each ampule received 0.5 mL of concentrated HNO3 and was then flame-sealed. DOM was oxidized at 160°C for 6 h (Ding et al., 2013). Since BPCA oxidation conditions were optimized in each laboratory to maximize the greatest percent carbon recovery, oxidation times and temperatures are different for the analysis of DPyC (at Florida International University) and soil PyC (at Colorado State University). After oxidation, the concentrated HNO3 was dried under N2 at 50°C. The solid residue remaining in the ampules was re-dissolved in mobile phase for high pressure liquid chromatography (HPLC) analysis of BPCAs (Dittmar, 2008) using an HPLC system coupled with a diode array detector (Surveyor, Thermo Scientific) and a Sunfire C18 reversed phase column (3.5 μm, 2.1 × 150 mm; Waters Corporation). BPCAs generated from DPyC were separated and quantified using a gradient elution method with a mobile phase A (4 mM tetrabutylammonium bromide, 50 mM sodium acetate, 10% MeOH) and mobile phase B (100% MeOH) (Dittmar, 2008). Non-pyrogenic organic materials do not produce BPCAs following the procedure described in the current study (Dittmar, 2008; Kappenberg et al., 2016), therefore we presume the DPyC reported in our study to be fire-derived. To calculate flux (g m−2), DPyC concentration (mg L−1) was multiplied by the total volume per unit area (L m−2) of leachate collected in one single snowmelt event (Figures 3A,B). The distribution of each individual BPCA in DPyC (Figure S2) is reported in the Supplemental Material.
Statistical Analyses
Data were transformed (log10) to normality and homogeneity of variance when necessary, and outliers were identified using Grubb's test. Comparisons between 100 year-burned and unburned sites for DPyC concentrations and fluxes, as well as PyC stocks in soils were performed using t-test at p < 0.05 significance level. Analyses were conducted using SigmaPlot v.12 (Systat Software, Inc., Chicago, IL), SPSS v.24 (IBM SPSS statistics for Windows), and software R 3.4.0 (R Development Core Team; http://www.R-project.org/).
Results
PyC Stocks in 100 Year-Burned and Unburned Sites
One hundred year-burned sites had significantly greater PyC stocks in the Oi (17.0 ± 2.1 g m−2) and Oe (39.8 ± 6.1 g m−2) horizons compared to unburned sites (Oi: 9.7 ± 0.8 g m−2, Oe: 17.0 ± 0.8 g m−2, P < 0.01, Figure 2). In the 100 year-burned site, PyC stocks were significantly greater in the Oe horizon (39.8 ± 6.1 g m−2) than in Oi horizon (17.0 ± 2.1 g m−2, P = 0.002, Figure 2). In E and B horizons, total stocks averaged 83.8 ± 6.7 and 400 ± 33 g m−2, respectively, across both sites. Differences in mineral soil PyC stocks between the two sites were not statistically significant (P = 0.296 and 0.364 for E and B horizon, respectively), even though the average PyC stocks in E and B soil horizons were greater in the 100 year-burned site than in the unburned site (Figure 2).
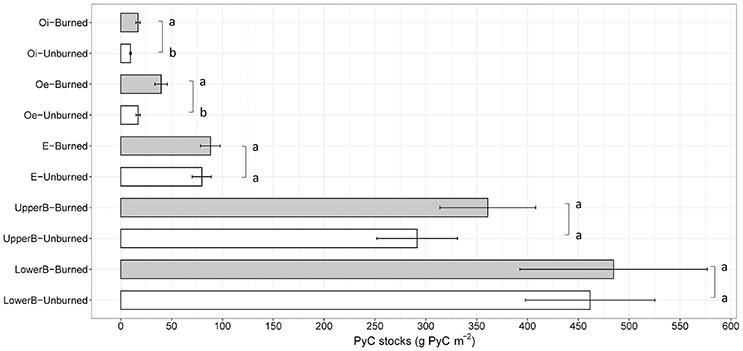
Figure 2. Pyrogenic carbon (PyC) stocks (g m−2) in organic (Oi and Oe) and mineral horizons E and B in burned and unburned sites, determined by the KMD method. Values shown are means ± standard error. Similar letters denote no statistically significant differences (P < 0.05). Number of replicates: Oi-Burned and -Unburned (n = 9), Oe-Burned and -Unburned (n = 8), E-Burned (n = 8), and -Unburned (n = 9), UpperB-Burned and -Unburned (n = 9), and LowerB-Burned and -Unburned (n = 9).
Dissolved PyC Leached from Organic and Mineral Soil Horizons
DPyC concentrations averaged 0.75 ± 0.13 and 1.24 ± 0.33 mg L−1 in 100 year-burned and unburned sites, respectively; however, there was no statistically significant difference between 100 year-burned and unburned sites (Figure 3A). Differences between horizons were only statistically significant between E and B horizons in the 100 year-burned site, with the E horizon yielding greater DPyC concentrations than the B horizon (P = 0.013, Figure 3A). In both sites, DPyC fluxes averaged 0.081 ± 0.032 and 0.052 ± 0.020 g m−2 in the O and E horizons, respectively (Figure 3B). For flux values, we found no significant difference between E and B horizons in the 100 year-burned site. Differences in DPyC fluxes and proportion of DPyC in DOC leached from O and E horizons between 100 year-burned and unburned sites were not statistically significant; however, we noted that the proportion of DPyC in DOC in E horizon was slightly higher in the 100 year-burned site than in unburned site and this difference was marginally significant (Figure 3C, P = 0.057).
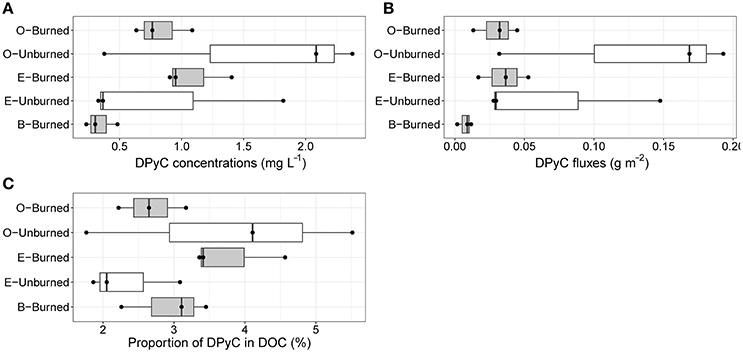
Figure 3. Box-plot of DPyC concentration (A, mg L−1), flux (B, g m−2), and proportion of DPyC in total DOC (C, %) in soil solution drained from the O, E, and B horizons in burned sites and from the O and E horizons in unburned sites. DPyC was determined by the BPCA-DOC method. BPCA-based DPyC data for the B horizon in unburned sites are missing in (A–C) due to lack of replication. Dark circles are individual data points. Statistically significant differences in (A) between E and B horizons in burned sites (P = 0.013).
Discussion
Our results provided evidence for a persistence of PyC input in soils caused by a single fire event in the 100 year-burned red pine sites. The PyC stocks measured in the O horizons were within the range of those reported for a conifer forest soil sampled 1 year after burning (Pingree et al., 2012), but lower than those reported for unburned sites in subalpine forest soil in Colorado (Buma et al., 2014). PyC stocks reported in this study are also lower than those reported for southern boreal forest soils in Minnesota sampled 1–2 months after burning and measured using solid-state 13C NMR (Miesel et al., 2015). Although the study by Miesel et al. (2015) used a different technique to measure soil PyC stocks, making direct comparisons of soil PyC stocks difficult, this is the only publication that reports PyC stock data for soils within the same region as our study site. PyC stocks presented here for E horizons are lower than those reported for the top 30 cm of sandy podzols (which included both Ae and B horizons) under a black spruce forest (Soucémarianadin et al., 2014) and the uppermost 10 cm of a subalpine (Buma et al., 2014) and a southern boreal forest soil (Miesel et al., 2015). PyC stocks measured for B horizon in our study were 3.1- to 3.5-fold greater than those reported by Soucémarianadin et al. (2014) 3–5 years after the fire. It is possible that (1) these stocks in the B horizons result from great amounts of PyC generated immediately after fire in the 100-year burned site, and that (2) that particulate PyC vertically moved to lower soil dephs via translocation over time (Dai et al., 2005; Leifeld et al., 2007; Koele et al., 2017). Further studies are needed to determine the major factors driving the stocks and transport rates of PyC to lower soil depths. Currently, little PyC stock data exists for B horizons in forest soils (Bird et al., 2015). As such, our study contributes to the fill this gap in PyC literature by providing a complete PyC stock dataset for a temperate forest soil.
Because our data show that a single fire event that occurred >100 years prior to sample collection significantly increased PyC stocks in organic horizons, we expected the increased soil PyC content to translate to higher concentrations and fluxes of DPyC in melt waters leached from the same fire-impacted soils. However, contrary to our hypothesis, concentrations and fluxes of DPyC mobilized from the O and E horizons did not reflect differences in fire recent history between the two sites studied here, even though a visible PyC layer was retained in the 100 year-burned site soils for approximately 100 years, and as evidenced by greater PyC stocks in the Oe horizon of the 100 year-burned site relative to the unburned site (Figure 2). The contribution of DPyC to total DOC reported in this study was much greater (from 1.77 to 5.51% across sites) than that reported for DPyC leached from the first 15 cm (0.02%) and 30 cm (0.017%) depths of a savanna oxisol 2 years after PyC application as charcoal (Major et al., 2010). We speculate that these differences are likely due to the timing of the leachate sampling, soil properties, and the physicochemical characteristics of PyC. Interestingly, our values are within the observed range of % DPyC reported in riverine systems (from 0.1 to 17.5%, Jaffé et al. (2013)).
We offer two explanations for the apparent uncoupling between DPyC concentrations and the abundance of PyC produced in the logging-era fire: First, DPyC concentrations and fluxes were likely influenced by the time and duration of our single sampling event, as soil leachates were sampled at a time when snowmelt occurred earlier at the unburned site because of the absence of leaves in the deciduous-dominated forest. As a result, more melt water moved through the unburned sites compared to the 100 year-burned sites. It is also possible that we may have missed the peak flux of DPyC associated with spring snowmelt. In-stream concentrations of DPyC can vary significantly with discharge in response to spring snow melt, likely driven by variations in hydrological flow paths which connect different soil horizons to the stream (Guggenberger et al., 2008; Stubbins et al., 2015; Wagner et al., 2015). Therefore, concentrations of DPyC mobilized from different soil horizons likely also undergo similar hysteresis patterns during a flushing event. Although capturing variations in DPyC concentrations leached from each soil horizon over the entire duration of spring snow melt at these sites was outside the scope of the current study, our results highlight the importance of closing this knowledge gap in future research endeavors. Second, it is possible that much of the easily-mobilized forms of PyC were lost to leaching during the first stages of PyC decomposition in soils (Hammes et al., 2008). As such, we may have missed the initial pulse of DPyC derived from fresh charcoal deposited in soils immediately after the original burn event some 100 years prior. Since soil DPyC did not vary predictably between 100 year-burned and unburned sites, this observation is in agreement with previous suggestions (Dittmar et al., 2012; Ding et al., 2013), that concentrations of DPyC alone may not be a suitable proxy for the occurrence of historic fire events.
In the current study, B soil horizons exhibited greater Fe and Al oxide contents compared to the E soil horizons (Schaetzl and Rothstein, 2016). PyC has been shown to interact with, and possibly bind to, Fe and Al hydroxides (Brodowski et al., 2005; Santos et al., 2012; Solomon et al., 2012; Soucémarianadin et al., 2014). Therefore, the Fe and Al concentrations in the B soil horizons likely influenced the amount of soil PyC stored in those horizons, consistent with previous studies (see above). It is also possible that DPyC was in part retained in the B horizons via sorption to Fe and Al hydroxides, as concentrations decreased significantly from the E to the B horizons for DPyC measured in soil solutions leached from 100 year-burned site soils. This sharp decrease in DPyC concentrations has been also observed by previous studies for DOC (Kaiser and Guggenberger, 2000). Taken together, our observations are consistent with previous studies that propose mineral adsorption as a stabilization mechanism for PyC (Czimczik and Masiello, 2007; Cusack et al., 2012).
Although further studies are needed to determine the mechanisms driving this relationship, we found similarities in the relative proportion of BPCAs between DPyC in soil solution leached from the O and E horizons (Figure S2) and in the soluble fraction of aged charcoal leached in laboratory (Abiven et al., 2011). This finding suggests that DPyC released from spodosols is influenced by aging and oxidation of soil PyC remaining in soil on a timescale of 100 years, which is also consistent with previous observations of PyC-like compounds in leachates sampled from a spodosol affected by a historic fire in northern Michigan (Hockaday et al., 2006).
It is possible that atmospheric PyC deposition from recent and historical fire events also contributed to: (a) the amount of PyC observed in the forest floor of both forest cover types, and (b) the concentration of DPyC in soil solution. Although annual PyC deposition fluxes (19.3–24.4 mg m−2 year−1) estimated for a single site in northern Michigan were considered small relative to soil PyC stock estimated for that site, these flux estimations did not take into account wet deposition of PyC during wintertime (Santos et al., 2014). A significant proportion of atmospheric PyC can be deposited onto snow during the winter and released during snowmelt (Lazarcik et al., 2017), becoming part of the soil DOC pool. For instance, results from a study conducted in New Hampshire, US, reported up to 62% losses of atmospheric PyC from snowpack within the first 24% of the snowmelt (Lazarcik et al., 2017). Over time, some of this atmospheric PyC could have migrated vertically to lower soil depths, becoming either retained in mineral soils or part of the DPyC pool. Thus, it may be possible that a proportion of the DPyC we observed in this study was derived from a pulse of atmospheric PyC that percolated into the soil with snowmelt, especially in the unburned site. Because wet deposition can be a significant source of DOC in watersheds, with annual wet deposition of DOC ranging between 3 and 13 kg C ha−1 year−1 (Iavorivska et al., 2017), the role of atmospheric deposition as an input of DPyC mobilized in soils warrants further research.
We cannot exclude the possibility that an ancient forest fire event (>250 years ago) may have contributed to the PyC and DPyC values we reported for the unburned sites. This would be consistent with observations of a continuous, long-term release of DPyC resulting from the degradation of historically accumulated PyC (Dittmar et al., 2012; Marques et al., 2017).
Finally, we acknowledge the limitations of this study, which prevents us from extrapolating current results to different field conditions. Due to budget limitations, the DPyC data reported here was collected from a single sampling date during spring snowmelt at our study site; therefore, variability in DPyC fluxes from different soil horizons across larger spatial and temporal scales between these contrasting forest types is still not understood. Future studies should focus on the collection of soil water samples at different times of the year and under different flow regimes to provide a more comprehensive dataset across which mobilization of DPyC from 100 year-burned and unburned landscapes can be robustly compared.
Conclusion
This study provides a preliminary look at the relationship between PyC stored in soils and the soluble fraction of DPyC, which is mobilized from different soil horizons during flushing events. Our results suggest that a single major fire event that occurred >100 years ago, which generated a substantial visible charcoal layer in the Oe horizon, had little to no detectable effect on concentrations of DPyC leached from soils relative to soils that have not experienced a similar fire event for the past 250 years. Based on the results of this study, we recommend three research directions that should be further explored to close critical knowledge gaps in our understanding of the PyC cycle this and similar systems: (1) dominant processes that constrain the links between soil PyC and DPyC; (2) the role of biotic and abiotic processes in facilitating the mobilization of PyC to lower soil depths; (3) the contribution of reactive Al and Fe oxides and oxyhydroxides in the retention of DPyC in subsoils.
Author Contributions
FS, DR, and JM led efforts in devising the study objectives and approach, and in writing the manuscript. FS and SW conducted DPyC measurements and analyzed DPyC data. DR established the original study site, provided soil and leachate samples, and provided the background data. RJ provided support with DPyC and soil PyC measurements, and data interpretation. JM provided support for DOC-SPE extraction and soil PyC measurements. All authors provided input and revisions in constructing the final version of the manuscript.
Conflict of Interest Statement
The authors declare that the research was conducted in the absence of any commercial or financial relationships that could be construed as a potential conflict of interest.
The reviewer, YY, declared a past co-authorship with one of the authors, RJ, to the handling editor.
Acknowledgments
We thank two reviewers for their constructive and helpful comments. Rodney Simpson and the EcoCore Analytical Facility (Colorado State University) for soil BPCA analysis, Dominic Uhelski for assistance with KMD digestions and data, as well as Mike Cook and Mike Luehmann for collecting the soil leachates. This project was supported by Michigan State University, the Department of Forestry Fred Arnold fund (awarded to JM), with partial support provided through the Scientist Exchange Program of the Novus Research Coordination Network NSF DEB-1145815 (awarded to FS). Establishment of the original study site, lysimeter installation and soil sample collection was supported by National Science Foundation (grant EAR1053373), Michigan State University AgBioResearch, and USDA National Institute of Food and Agriculture (McIntire Stennis Project MICL06006) awards to DR. Additional support was provided through Florida International University for the BPCA measurements for both DPyC and PyC, funded through the George Barley Fund (awarded to RJ). SW and RJ thank the NSF through the FCE LTER (DEB-1237517) for additional financial support of this work. This is contribution number 846 from the Southeast Environmental Research Center in the Institute of Water & Environment at Florida International University.
Supplementary Material
The Supplementary Material for this article can be found online at: https://www.frontiersin.org/articles/10.3389/feart.2017.00080/full#supplementary-material
References
Abiven, S., Hengartner, P., Schneider, M. P., Singh, N., and Schmidt, M. W. (2011). Pyrogenic carbon soluble fraction is larger and more aromatic in aged charcoal than in fresh charcoal. Soil Biol. Biochem. 43, 1615–1617. doi: 10.1016/j.soilbio.2011.03.027
Batjes, N. H. (1996). Total carbon and nitrogen in the soils of the world. Eur. J. Soil Sci. 47, 151–163. doi: 10.1111/j.1365-2389.1996.tb01386.x
Bird, M. I., Wynn, J. G., Saiz, G., Wurster, C. M., and McBeath, A. (2015). The pyrogenic carbon cycle. Annu. Rev. Earth Planet. Sci. 43:273–298. doi: 10.1146/annurev-earth-060614-105038
Boot, C. M., Haddix, M., Paustian, K., and Cotrufo, M. F. (2015). Distribution of black carbon in ponderosa pine forest floor and soils following the high park wildfire. Biogeosciences 12:3029–3039. doi: 10.5194/bg-12-3029-2015
Brodowski, S., Amelung, W., Haumaier, L., Abetz, C., and Zech, W. (2005). Morphological and chemical properties of black carbon in physical soil fractions as revealed by scanning electron microscopy and energy-dispersive X-ray spectroscopy. Geoderma 128, 116–129.doi: 10.1016/j.geoderma.2004.12.019
Buma, B., Poore, R. E., and Wessman, C. A. (2014). Disturbances, their interactions, and cumulative effects on carbon and charcoal stocks in a forested ecosystem. Ecosystems 17, 947–959. doi: 10.1007/s10021-014-9770-8
Comer, P. J., Albert, D. A., Wells, H. A., Hart, B. L., Raab, J. B., Price, D. L., et al. (1995). Michigan's presettlement vegetation, as interpreted from the general land office Surveys 1816–1856. Michigan Natural Feature Inventory, Lansing
Cotrufo, M. F., Boot, C. M., Kampf, S., Nelson, P. A., Brogan, D. J., Covino, T., et al. (2016). Redistribution of pyrogenic carbon from hillslopes to stream corridors following a large montane wildfire. Glob. Biogeochem. Cycles 30, 1348–1355. doi: 10.1002/2016GB005467
Cusack, D. F., Chadwick, O. A., Hockaday, W. C., and Vitousek, P. M. (2012). Mineralogical controls on soil black carbon preservation. Glob. Biogeochem. Cycles 26:GB2019. doi: 10.1029/2011GB004109
Czimczik, C. I., and Masiello, C. A. (2007). Controls on black carbon storage in soils. Glob. Biogeochem. Cycles 21, 1–8. doi: 10.1029/2006GB002798
Czimczik, C. I., Schmidt, M. W. I., and Schulze, E. D. (2005). Effects of increasing fire frequency on black carbon and organic matter in Podzols of Siberian Scots pine forests. Eur. J. Soil Sci. 56, 417–428. doi: 10.1111/j.1365-2389.2004.00665.x
Dai, X., Boutton, T. W., Glaser, B., Ansley, R. J., and Zech, W. (2005). Black carbon in a temperate mixed-grass savanna. Soil Biol. Biochem. 37, 1879–1881. doi: 10.1016/j.soilbio.2005.02.021
Ding, Y., Cawley, K. M., da Cunha, C. N., and Jaffé, R. (2014). Environmental dynamics of dissolved black carbon in wetlands. Biogeochemistry 119, 259–273. doi: 10.1007/s10533-014-9964-3
Ding, Y., Yamashita, Y., Dodds, W. K., and Jaffé, R. (2013). Dissolved black carbon in grassland streams: is there an effect of recent fire history? Chemosphere 90, 2557–2562. doi: 10.1016/j.chemosphere.2012.10.098
Dittmar, T. (2008). The molecular level determination of black carbon in marine dissolved organic matter. Org. Geochem. 39, 396–407. doi: 10.1016/j.orggeochem.2008.01.015
Dittmar, T., De Rezende, C. E., Manecki, M., Niggemann, J., Ovalle, A. R. C., Stubbins, A., et al. (2012). Continuous flux of dissolved black carbon from a vanished tropical forest biome. Nat. Geosci. 5:618–622. doi: 10.1038/ngeo1541
Dittmar, T., Koch, B., Hertkorn, N., and Kattner, G. (2008). A simple and efficient method for the solid-phase extraction of dissolved organic matter (SPE-DOM) from seawater. Limnol. Oceanogr. Methods 6, 230–235. doi: 10.4319/lom.2008.6.230
Fang, Y., Singh, B., Singh, B. P., and Krull, E. (2014). Biochar carbon stability in four contrasting soils. Eur. J. Soil Sci. 65, 60–71. doi: 10.1111/ejss.12094
Forbes, M. S., Raison, R. J., and Skjemstad, J. O. (2006). Formation, transformation and transport of black carbon (charcoal) in terrestrial and aquatic ecosystems. Sci. Total Environ. 370, 190–206. doi: 10.1016/j.scitotenv.2006.06.007
Goldberg, E. D. (1985). Black Carbon in the Environment: Properties and Distribution. New York, NY: John Wiley & Sons.
Güereña, D. T., Lehmann, J., Walter, T., Enders, A., Neufeldt, H., Odiwour, H., et al. (2015). Terrestrial pyrogenic carbon export to fluvial ecosystems: lessons learned from the white Nile watershed of East Africa. Glob. Biogeochem. Cycles 29, 1911–1928. doi: 10.1002/2015GB005095
Guggenberger, G., Rodionov, A., Shibistova, O., Grabe, M., Kasansky, O. A., Fuchs, H., et al. (2008). Storage and mobility of black carbon in permafrost soils of the forest tundra ecotone in Northern Siberia. Glob. Chang. Biol. 14, 1367–1381. doi: 10.1111/j.1365-2486.2008.01568.x
Hammes, K., Torn, M. S., Lapenas, A. G., and Schmidt, M. W. I. (2008). Centennial black carbon turnover observed in a Russian steppe soil. Biogeosciences 5, 1339–1350. doi: 10.5194/bg-5-1339-2008
Hockaday, W. C., Grannas, A. M., Kim, S., and Hatcher, P. G. (2006). Direct molecular evidence for the degradation and mobility of black carbon in soils from ultrahigh-resolution mass spectral analysis of dissolved organic matter from a fire-impacted forest soil. Org. Geochem. 37, 501–510. doi: 10.1016/j.orggeochem.2005.11.003
Hockaday, W. C., Grannas, A. M., Kim, S., and Hatcher, P. G. (2007). The transformation and mobility of charcoal in a fire-impacted watershed. Geochim. Cosmochim. Acta 71:3432–3445. doi: 10.1016/j.gca.2007.02.023
Iavorivska, L., Boyer, E. W., and Grimm, J. W. (2017). Wet atmospheric deposition of organic carbon: an underreported source of carbon to watersheds in the northeastern United States. J. Geophys. Res. Atmos. 122, 3104–3115. doi: 10.1002/2016JD026027
Jaffé, R., Ding, Y., Niggemann, J., Vähätalo, A. V., Stubbins, A., Spencer, R. G. Dittmar, et al. (2013). Global charcoal mobilization from soils via dissolution and riverine transport to the oceans. Science 340, 345–347. doi: 10.1126/science.1231476
Jauss, V., Sullivan, P. J., Sanderman, J., Smith, D. B., and Lehmann, J. (2017). Pyrogenic carbon distribution in mineral topsoils of the northeastern United States. Geoderma 296, 69–78. doi: 10.1016/j.geoderma.2017.02.022
Jobbagy, E. G., and Jackson, R. B. (2000). The vertical distribution of soil organic carbon and its relation to climate and vegetation. Ecol. Appl. 10, 423–436. doi: 10.1890/1051-0761(2000)010[0423:TVDOSO]2.0.CO;2
Kaiser, K., and Guggenberger, G. (2000). The role of DOM sorption to mineral surfaces in the preservation of organic matter in soils. Org. Geochem. 31, 711–725. doi: 10.1016/S0146-6380(00)00046-2
Kalbitz, K., Solinger, S., Park, J. H., Michalzik, B., and Matzner, E. (2000). Controls on the dynamics of dissolved organic matter in soils: a review. Soil Sci. 165, 277–304. doi: 10.1097/00010694-200004000-00001
Kappenberg, A., Bläsing, M., Lehndorff, E., and Amelung, W. (2016). Black carbon assessment using benzene polycarboxylic acids: limitations for organic-rich matrices. Org. Geochem. 94, 47–51. doi: 10.1016/j.orggeochem.2016.01.009
Kleber, M., Eusterhues, K., Keiluweit, M., Mikutta, C., Mikutta, R., and Nico, P. S. (2015). Mineral-organic associations: formation, properties, and relevance in soil environments. Adv. Agron. 130, 1–140. doi: 10.1016/bs.agron.2014.10.005
Koele, N., Bird, M., Haig, J., Marimon-Junior, B. H., Marimon, B. S., Phillips, O. L., et al. (2017). Amazon Basin forest pyrogenic carbon stocks: first estimate of deep storage. Geoderma 306, 237–243. doi: 10.1016/j.geoderma.2017.07.029
Kurth, V. J., Mackenzie, M. D., and Deluca, T. H. (2006). Estimating charcoal content in forest mineral Soils 137, 135–139. doi: 10.1016/j.geoderma.2006.08.003
Lazarcik, J., Dibb, J. E., Adolph, A. C., Amante, J. M., Wake, C. P., Scheuer, E., et al. (2017). Major fraction of black carbon is flushed from the melting New Hampshire snowpack nearly as quickly as soluble impurities. J. Geophys. Res. Atmos. 122, 537–553. doi: 10.1002/2016JD025351
Leifeld, J., Fenner, S., and Müller, M. (2007). Mobility of black carbon in drained peatland soils. Biogeosciences 4, 425–432. doi: 10.5194/bg-4-425-2007
Maestrini, B., and Miesel, J. R. (2016). Modification of the weak nitric acid digestion method for the quantification of black carbon in organic matrices. Org. Geochem. 103, 1–4. doi: 10.1016/j.orggeochem.2016.10.010
Major, J., Lehmann, J., Rondon, M., and Goodale, C. (2010). Fate of soil-applied black carbon: downward migration, leaching and soil respiration. Glob. Chang. Biol. 16, 1366–1379. doi: 10.1111/j.1365-2486.2009.02044.x
Marques, J. S. J., Dittmar, T., Niggemann, J., Almeida, M. G., Gomez-Saez, G. V., and Rezende, C. E. (2017). Dissolved Black Carbon in the Headwaters-to-Ocean Continuum of Paraíba do Sul River. Front. Earth Sci. 5:11. doi: 10.3389/feart.2017.00011
Miesel, J. R., Hockaday, W. C., Kolka, R. K., and Townsend, P. A. (2015). Soil organic matter composition and quality across fire severity gradients in coniferous and deciduous forests of the southern boreal region. J. Geophys. Res. Biogeosci. 120, 1124–1141. doi: 10.1002/2015JG002959
Myers-Pigg, A. N., Louchouarn, P., Amon, R. M., Prokushkin, A., Pierce, K., and Rubtsov, A. (2015). Labile pyrogenic dissolved organic carbon in major Siberian Arctic rivers: implications for wildfire-stream metabolic linkages. Geophys. Res. Lett. 42, 377–385. doi: 10.1002/2014GL062762
Pingree, M. R. A., Homann, P. S., Morrissette, B., and Darbyshire, R. (2012). Long and short-term effects of fire on soil charcoal of a conifer forest in Southwest Oregon. Forests 3, 353–369. doi: 10.3390/f3020353
Reisser, M., Purves, R. S., Schmidt, M. W. I., and Abiven, S. (2016). Pyrogenic carbon in soils: a literature-based inventory and a global estimation of its content in soil organic carbon and stocks. Front. Earth Sci. 4, 1–14. doi: 10.3389/feart.2016.00080
Rumpel, C., Alexis, M., Chabbi, A., Chaplot, V., Rasse, D. P., Valentin, C., et al. (2006). Black carbon contribution to soil organic matter composition in tropical sloping land under slash and burn agriculture. Geoderma 130, 35–46. doi: 10.1016/j.geoderma.2005.01.007
Rumpel, C., Ba, A., Darboux, F., Chaplot, V., and Planchon, O. (2009). Erosion budget and process selectivity of black carbon at meter scale. Geoderma 154, 131–137. doi: 10.1016/j.geoderma.2009.10.006
Santín, C., Doerr, S. H., Kane, E. S., Masiello, C. A., Ohlson, M., de la Rosa, J. M., et al. (2016). Towards a global assessment of pyrogenic carbon from vegetation fires. Glob. Chang. Biol. 22, 76–91. doi: 10.1111/gcb.12985
Santos, F., Fraser, M. P., and Bird, J. A. (2014). Atmospheric black carbon deposition and characterization of biomass burning tracers in a northern temperate forest. Atmos. Environ. 95, 383–390. doi: 10.1016/j.atmosenv.2014.06.038
Santos, F., Torn, M. S., and Bird, J. A. (2012). Biological degradation of pyrogenic organic matter in temperate forest soils. Soil Biol. Biochem. 51, 115–124. doi: 10.1016/j.soilbio.2012.04.005
Schaetzl, R. J., and Rothstein, D. E. (2016). Temporal variation in the strength of podzolization as indicated by lysimeter data. Geoderma 282:26–36. doi: 10.1016/j.geoderma.2016.07.005
Schaetzl, R. J., Luehmann, M. D., and Rothstein, D. (2015). Pulses of podzolization: the relative Importance of spring snowmelt, summer storms, and fall rains on spodosol development. Soil Sci. Soc. Am. J. 79, 117–131. doi: 10.2136/sssaj2014.06.0239
Schmidt, M. W. I., Hilf, M. D., and Wiesenberg, G. L. B. (2017). “15 Analysis of biochars using benzene polycarboxylic acids,” in Biochar A Guide to Analytical Methods, eds B. Singh, M. Camps-Arbestain, and J. Lehmann (Boca Raton, FL: CSIRO Publishing), 162.
Singh, N., Abiven, S., Torn, M. S., and Schmidt, M. W. I. (2012). Fire-derived organic carbon in soil turns over on a centennial scale. Biogeosciences 9, 2847–2857. doi: 10.5194/bg-9-2847-2012
Solomon, D., Lehmann, J., Wang, J., Kinyangi, J., Heymann, K., Lu, Y., et al. (2012). Micro- and nano-environments of C sequestration in soil: a multi-elemental STXM-NEXAFS assessment of black C and organomineral associations. Sci. Total Environ. 438, 372–388. doi: 10.1016/j.scitotenv.2012.08.071
Soucémarianadin, L. N., Quideau, S. A., and MacKenzie, M. D. (2014). Pyrogenic carbon stocks and storage mechanisms in podzolic soils of fire-affected Quebec black spruce forests. Geoderma 217–218, 118–128. doi: 10.1016/j.geoderma.2013.11.010
Stubbins, A., Spencer, R. G., Mann, P. J., Holmes, R. M., McClelland, J. W., Niggemann, J., et al. (2015). Utilizing colored dissolved organic matter to derive dissolved black carbon export by arctic rivers. Front. Earth Sci. 3:63. doi: 10.3389/feart.2015.00063
Wagner, S., Cawley, K. M., Rosario-Ortiz, F. L., and Jaffé, R. (2015). In-stream sources and links between particulate and dissolved black carbon following a wildfire. Biogeochemistry 124, 145–161. doi: 10.1007/s10533-015-0088-1
Wagner, S., Ding, Y., and Jaffé, R. (2017). A new perspective on the apparent solubility of dissolved black carbon. Front. Earth Sci. 5:75. doi: 10.3389/feart.2017.00075
Whitney, G. G. (1987). An ecological history of the Great Lakes forest of Michigan. J. Ecol. 75, 667–684. doi: 10.2307/2260198
Keywords: dissolved pyrogenic carbon, pyrogenic carbon stock, carbon leaching, spodosols, historical fire, Michigan, temperate forest
Citation: Santos F, Wagner S, Rothstein D, Jaffe R and Miesel JR (2017) Impact of a Historical Fire Event on Pyrogenic Carbon Stocks and Dissolved Pyrogenic Carbon in Spodosols in Northern Michigan. Front. Earth Sci. 5:80. doi: 10.3389/feart.2017.00080
Received: 29 May 2017; Accepted: 29 September 2017;
Published: 16 October 2017.
Edited by:
Cristina Santin, Swansea University, United KingdomReviewed by:
Youhei Yamashita, Hokkaido University, JapanDerrick Y. F. Lai, The Chinese University of Hong Kong, Hong Kong
Copyright © 2017 Santos, Wagner, Rothstein, Jaffe and Miesel. This is an open-access article distributed under the terms of the Creative Commons Attribution License (CC BY). The use, distribution or reproduction in other forums is permitted, provided the original author(s) or licensor are credited and that the original publication in this journal is cited, in accordance with accepted academic practice. No use, distribution or reproduction is permitted which does not comply with these terms.
*Correspondence: Fernanda Santos, ZnNhbnRvcy5zb2lsc0BnbWFpbC5jb20=
†Present Address: Fernanda Santos, School of Natural Sciences, University of California-Merced, Merced, CA, United States
Sasha Wagner, Marine Sciences Department, Skidaway Institute of Oceanography, University of Georgia, Savannah, GA, United States