- 1Department of Pharmacy, School of Medicine, University of Naples “Federico II”, Napoli, Italy
- 2Department of Agricultural Science, University of Naples “Federico II”, Portici, Italy
Antimicrobial resistance has significantly increased over the last 30 years, prompting scientists to continuously look for novel, effective ways to combat drug-resistant bacteria and fungi. Due to their broad range of effectiveness, ease of synthesis, and ability to avoid resistance, antimicrobial peptides (AMPs) represent a potential approach. The direct investigation of metal effects on peptide activity has not received much attention. Divalent metal ions such as Zn(II), Cu(II), Ni(II), and Fe(II) do, in fact, influence some AMPs, producing an effect on their mode of action or function. Although the precise process by which metals are involved in microbial death is not well understood, this review goes into detail on several potential strategies to enhance AMPs activity through the synergy with metals. Important variables in these interactions include the individual peptide sequence, the type of metal ion, the capacity of metal to form chelates, and the softness of the ligand/metal complex. This article offers a thorough summary of the ways in which metal ions alter the structure of AMPs to boost their activity or sequester metal to impact on bacteria function.
1 Introduction
Metal ions can interact with therapeutic peptides through various mechanisms. Among these, they have the capability to augment the biological efficacy of antimicrobial peptides (AMPs), suggesting potential for the creation of new antibiotics and antifungals (Galdiero et al., 2015; Mulukutla et al., 2024).
AMPs, integral components of the innate immune system, exhibit diverse actions and minimal tendencies to induce antibiotic resistance. They exhibit broad-spectrum activity against various microorganisms, including Gram-positive and Gram-negative bacteria, fungi, viruses and can be used alone or in combination with antibiotics (Galdiero et al., 2015). There are also some encouraging examples of AMPs in clinical trials or already introduced into the market (Biswas et al., 2024; Cresti et al., 2024; Xiang et al., 2024; Lesiuk et al., 2022). Found across a spectrum of organisms including plants, insects, mammals, and invertebrates, they typically feature a significant proportion of hydrophobic and positively charged residues arranged amphipathically; while most AMPs are positively charged, some anionic examples have also been identified (Galdiero et al., 2015; Mulukutla et al., 2024). Classification of AMPs can be based on their biological origin (bacterial, plant, or animal), secondary structure (helical, β-sheet, extended, or random), biophysical characteristics (charge, hydrophobicity, length), molecular targets (cell surface, cell-penetrating), and functions (antibacterial, antifungal, antiviral, anticancer, wound healing, and immune modulation).
A crucial aspect to consider is how AMPs interact with cell membranes, with proposed mechanisms including membrane permeabilization (Bellavita et al., 2022; Bellavita et al., 2023a), disruption of ion transmembrane gradients, or interference with cell membrane biosynthesis (Galdiero et al., 2015). These mechanisms are heavily influenced by the structural arrangement of AMPs. In particular, AMPs primarily operate through two main mechanisms: a) interaction with phospholipids to disrupt membrane bilayer (Bellavita et al., 2023b); b) targeting of intracellular components to inhibit nucleic acid and protein synthesis (Le et al., 2017). The former mechanism involves either pore formation or membrane disruption, with some peptides accumulating on the membrane and altering its shape to create transitional pores. Recent studies indicate that many AMPs utilize the latter mechanism by binding to intracellular targets such as proteins, RNA, and DNA within various cellular compartments (Le et al., 2017). In some cases, AMPs exploit both mechanisms simultaneously.
Over the past three decades, antimicrobial resistance has seen a significant increase, prompting researchers to constantly seek out new, effective therapies and targeted delivery methods to combat drug-resistant bacteria and fungi. Indeed, AMPs have emerged as a promising class of therapeutics in this pursuit due to their overall minimal tendency to induce resistance, facile synthesis and broad-spectrum activity, notwithstanding the shortcoming of peptides as drugs (Galdiero et al., 2015). In fact, peptides are prone to enzyme degradation which may limit their effectiveness; they have poor oral bioavailability, they may have a short half-life in the body and in some cases they can elicit an immune response leading to potential side effects. Despite facing these challenges in clinical development, many synthetic AMPs are presently undergoing clinical trials for treating various diseases like diabetic foot ulcers and infections associated with chemotherapy (Da Silva et al., 2021). Although bacteria can diminish the effectiveness of AMPs through mechanisms like altering membrane composition, efflux via energy-dependent pumps, and protease cleavage, the development of peptidomimetics has shown promise in overcoming these limitations (Bellavita et al., 2023c; Roscetto et al., 2021). Thus, while AMPs hold great potential for drug development, further efforts are necessary to ensure successful delivery, appropriate targeting, and enhanced efficacy in vivo. To facilitate delivery and mitigate the risk of resistance development, researchers have explored conjugating AMPs and their derivatives to nanoparticles composed of metals such as nickel, silver, or gold (Teixeira et al., 2020). Additionally, AMPs can be attached to metal surfaces to prevent infections associated with implants (Biswaro et al., 2018).
Indeed, while there is extensive literature on the role and mechanisms of action of AMPs and their delivery, relatively few studies have directly examined the influence of metals on peptide activity (Sóvágó et al., 2012). Given that numerous proteins depend on metal ions for their functionality, it is reasonable to assume that metals could also regulate the activity of AMPs (Figure 1). Some AMPs are indeed affected in their activity or mechanism of action by the presence of divalent metal ions like Zn (II) and Cu(II), Ni(II), Fe(II). The precise mechanism by which metals induce microbial death is not fully elucidated, but several plausible mechanisms exist: a) as metal ions are required by bacteria to survive and infect host cells, these last may compete for metal supply a phenomenon known as nutritional immunity; thus, the binding of metal ions by host proteins prevents their availability to bacteria; b) metal ions can alter the charge and/or structure of peptides, thereby boosting their antibacterial properties; c) metal ions may generate reactive oxygen species (ROS), leading to oxidative damage involving lipid peroxidation, protein oxidation, and DNA damage (Libardo et al., 2014). The specific peptide sequence and the type of metal ion are critical determinants of these interactions. Other key factors include the preferred geometry of the metal, its ability to form chelates, and the softness of the ligand/metal complex. Numerous examples exist where AMPs employ different modes of action, either naturally or in response to external factors (Donaghy et al., 2023).
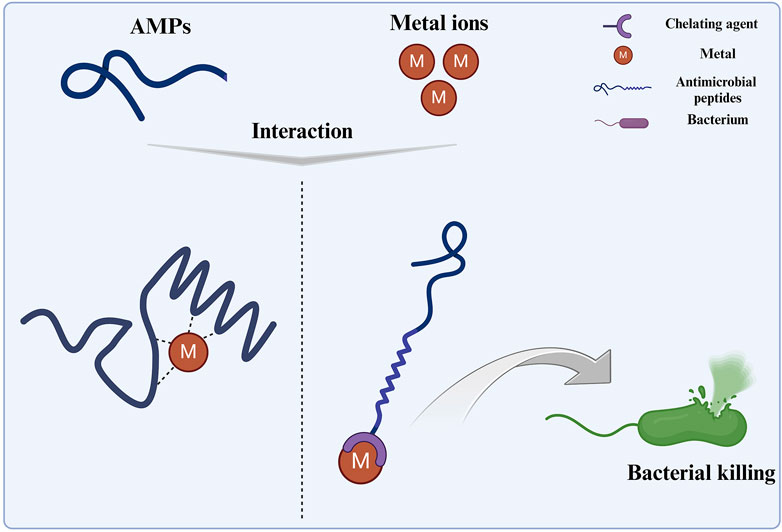
Figure 1. The impact of metal ions on the activity of AMPs: on the left side metals determine structural modifications of the peptide that enhance antimicrobial activity, while on the right side a metal chelating unit naturally present or engineered with the AMP causes metal sequestration and microorganism starvation.
Here, we present a comprehensive overview of how metal ions impact antimicrobial peptide function through structural modifications and metal sequestration and report some examples of the literature.
2 Peptide-metal coordination
Iron stands as a necessary trace metal for the majority of living organisms, serving as a prosthetic group for proteins involved in critical physiological functions such as respiration, oxygen transport, and DNA synthesis (Garstka et al., 2023). Zinc places as the second most crucial trace element in the human body after iron, essential for cell division and growth across all forms of life, including humans. Unlike elements like copper or iron, zinc maintains a stable oxidation state in solution due to its electron configuration, [Ar]3d10, which precludes it from undergoing d-d transitions and renders it undetectable in absorption spectroscopy. The preferred donor atoms for Zn(II) typically include nitrogen, sulfur, and oxygen, which, for instance, are present in the side chains of amino acid residues containing histidine, cysteine, glutamic acid, or aspartic acid. Nickel is another biologically relevant metal ion, vital for numerous metabolic processes such as metabolism and pathogenicity in fungi, plants, eubacteria, and archaebacteria (Żamojć et al., 2021). While trace amounts of nickel are present in the human body due to its necessity for commensal bacteria, prolonged or extensive exposure to nickel may lead to genotoxic, hemotoxic, teratogenic, carcinogenic, or immunotoxic effects. Ni(II) and Fe(II) are particularly intriguing from a chemical standpoint as they can ideally compete with Zn(II) for binding sites. In aerobic organisms, copper is present in various metal proteins/enzymes that play vital roles for many different organisms, from plants to fungi and vertebrates. It can play both a structural role, as in the case of copper-activated metal regulated transcription factors of fungi, and a functional role, as in the case of nucleophilic catalysis and electron transport (Turski and Thiele, 2009). In humans, copper is especially important in Cu-Zn superoxide dismutase (catalyzes the disproportion of the superoxide ion into O2 and peroxide ion) and in the cytochrome c oxidase enzyme, active in the respiratory chain. Of the two forms Cu(I) and Cu(II), the first prevails in the intracellular reducing environment, the second in the extracellular oxidizing one. The stability of Cu(I), almost insoluble in water at physiological pH, is ensured by complexation with sulfur or azole ligands coming from the protein environment. The fact that the Cu(II)/Cu(I) reduction potential is tuned by coordination geometries and the type of binding sites makes copper an ideal metal center for electron transfer processes.
The polarizability of an atom is a fundamental concept in the Hard and Soft Acids and Bases (HSAB) theory. According to this theory, hard acids and bases are non-polarizable and typically small, while soft acids and bases are polarizable and generally larger with lower charge density. Borderline acids and bases have properties that lie between those of hard and soft ligands (Asensio-Calavia et al., 2023). The HSAB principle dictates that hard acids prefer hard bases, soft acids prefer soft bases, and borderline acids prefer borderline bases (Asensio-Calavia et al., 2023). Fe(III), Cu(I), and Zn(II) can be categorized as hard, soft, and borderline Lewis acids, respectively. The chelate effect, observed when polydentate ligands with multiple donor atoms form more stable complexes compared to their monodentate counterparts, further stabilizes metal-peptide combinations. Thermodynamically, 5 and 6-membered rings are the most stable in Cu(II)-peptide complexes, where Cu(II) is coordinated to three amide nitrogen atoms from the peptide backbone preceding it and a histidine imidazole nitrogen. Complex shape, governed by Ligand Field Stabilization Energy (LFSE), also influences metal-peptide complex formation. Fe(III) and Fe(II) typically adopt octahedral or distorted octahedral geometries when bound to peptides. Cu(II) prefers square planar, square pyramidal, or axially distorted octahedral geometries, while Cu(I) favors geometries with 2, 3, or 4-coordinate sites. Zn(II) tends to form distorted tetrahedral complexes (Dudev and Lim, 2000).
Peptides have a strong ability to efficiently bind metal ions because of the participation of the amino group in N-terminal position and subsequently of deprotonated nitrogen atoms along the peptide backbone. This leads to the formation of highly stable complexes between peptides and metal ions. These complexes exhibit a wide spectrum of possible donor atoms and can exist in various conformations (Bal et al., 1997). The selective binding of metal ions by amino acids is dependent upon the hard/soft character of the metal ion with key metal anchoring sites including the side chains of histidine (imidazole) or cysteine (thiolate) residues, as well as the carboxyl groups of glutamic and aspartic acids (Garstka et al., 2023; Lihi et al., 2017). Histidine and cysteine residues are particularly effective in enhancing the stability and altering the structure of peptide-metal complexes (Sóvágó et al., 2012). Specifically, amino acids like histidine, aspartic acid, and glutamic acid demonstrate pH-sensitive behaviours largely due to their protonation in acidic settings. This protonation is crucial as it enhances the overall cationic nature of the AMP molecule, influencing its interaction with the mainly anionic microbial membrane. The outcome of this interaction hinges on whether these residues are protonated, which in turn improves the molecule cationic properties. Oligoalanine is an example of a ligand with non-coordinating side chains that possesses amino and amide nitrogen atoms, as well as carbonyl and carboxyl oxygens as donor sites. Residues with aromatic rings, like tyrosine or phenylalanine, can contribute to complex stability or structure through hydrophobic interactions or ring stacking. Motifs such as Cys–His and His–Cys are prevalent in AMPs and serve as highly attractive metal binding sites for ions like Zn(II), Fe(II), or Ni(II), which are essential to the function of bacterial and fungal cells (Garstka et al., 2023). Thus, sequences abundant in histidine and cysteine residues serve as ideal ligands and are exploited as effective metal chelators by natural processes and for scientific development. The diversity of physiologically significant amino acid residues allows for a wide range of intramolecular interactions in metal-peptide systems, leading to structurally specific complexes that could modulate biological activity through metal binding (Kozłowski et al., 1999). Therefore, utilizing our comprehension of the coordination mode, structure, and function of individual binding sites, we can advance the development of new materials and applications.
Cysteine has a reactive sulfhydryl group, and a disulfide bond, which plays a structural role in protein/peptide folding, may form when two cysteines come together (Muskal et al., 1990). In particular, two cysteine residues that are adjacent to one another or separated by just one residue cannot interact because of the peptide bond’s rigid planar structure and as a matter of fact, the closest proximity between two cysteine residues in the α-helix and β-turn results from their separation by two amino acid residues. Furthermore, the C-terminal donor group serves as the major anchor group for peptides containing both cysteine and histidine residues, such as Ac-HAAC-NH2 or Ac-CGAH-NH2 (Garstka et al., 2023; Muskal et al., 1990).
Histidine side chain is certainly the most environment tunable metal ligand. Its plasticity in molecular interactions can be attributed to its structure and acid-base characteristics (Rowinska-Zyrek et al., 2013). The aromatic imidazole ring, which has two nitrogen atoms and a pKa slightly above 6, is partially deprotonated at physiological pH and can be seen in both basic and acidic forms. The positive charge in the acidic imidazolium cation is split equally between the two nitrogen atoms. Each of these has the ability to release a proton in response to a change in pH, forming the equivalent tautomer forms 1N or 3N, which can bind metal ions (Rowinska-Zyrek et al., 2013). The development of two fused chelate rings, the six-membered (Libardo et al., 2014) (histamine-like) and the five-membered (NH2, COO−) (amino acid-like), allows all three donor groups of this amino acid to cooperate in metal binding. The 1N imidazole nitrogen is preferred compared to the 3N because of the higher thermodynamic stability of five- and six-membered rings compared to bigger ones. Thus, in biological systems, histidine serves as the main low molecular weight chelator.
However, the number and location of histidine residues within the peptide chain have a significant impact on the capacity of His residues to coordinate within a peptide sequence. One of the nitrogen atoms of the histidine imidazole ring and the nitrogen atom of the N-terminal amino group can form complexes with peptides that have a histidine residue in the first position (Garstka et al., 2023; Migliorini et al., 2010). The creation of a five-membered ring is the defining hallmark of this coordination mechanism, which is also known as the histamine-like model. As for Zn(II), it exhibits a histamine coordination mode over the entire pH range with atoms of oxygen, nitrogen, and sulphur originating from side chains of amino acids and water molecules filling the zinc coordination sphere. Cu(II), Ni(II), and Fe(II) are examples of compounds that can deprotonate the amide bond at basic pH. As a result, amide nitrogen atoms may be included in the metal coordination sphere, forming stable, six-membered rings with a (NH2, 3N) mode (Sóvágó et al., 2016).
The simultaneous engagement of the amine, imidazole, and intervening histidine amide nitrogen atoms in binding is made possible by the inclusion of histidine at position two of a peptide chain. This configuration promotes stable five- or six-membered rings, which improve Ni(II) and Cu(II) coordination. The predominant complex observed is CuH-1L detected at pH as low as 4 and remaining the most significant species up to pH 10. The exceptional stability of this complex arises from the formation of another pair of fused chelate rings: a five-membered ring involving the NH2 and N-atoms, and a six-membered ring involving the N- and imidazole nitrogen (Nim). X-ray structures of Cu(II) complexes corroborate the 3N coordination in the CuH-1L complex. Clearly, the amino acid at position one plays a crucial role in fine-tuning the formation of the complex (Kozłowski et al., 1999).
The coordination plane can be saturated when three fused chelate rings form simultaneously due to the presence of a His residue in position three of the peptide chain. This motif is known as the ATCUN (amino terminal Cu(II) and Ni(II) binding site) motif or albumin-like coordination site (Garstka et al., 2023).
The 4N complexes resulting from this binding mechanism represent the most stable peptide-metal ion complexes. Due to the cooperative nature of binding, the intermediate complexes with 1N, 2N, and 3N coordination are less prevalent. These intermediate complexes are typically identified through computer analysis of potentiometric titrations, as their inclusion in the complexation model enhances numerical fitting accuracy. Nevertheless, despite the improved fit, a thorough spectroscopic analysis showed that no such species existed (Garstka et al., 2023). This result suggests that the observed existence of these small complexes might simply be a potentiometric technique artefact. The cooperative formation of fused chelate rings leads to a transition from octahedral and high spin geometry to square-planar and low spin geometry (Kozłowski et al., 1999).
When the N-terminal amine and the imidazole donors are separated by two or more intervening amino acid residues, the possibility of concerted formation of the fused chelate system is lost. This is because the situation is more complicated due to the competition from five or more possible nitrogen donors for the four equatorial locations surrounding the metal ion. Similarly, introducing more than one histidine residue in the oligopeptide can yield different outcomes depending on the other amino acids present and the distance between the histidine residues (Kozłowski et al., 1999).
Apart from the aforementioned coordination mechanisms, it is also important to note that a particular amino acid sequence –HEXXH– with a strong affinity for Zn(II) may exist at any point in the chain (Wątły et al., 2016). This sequence has been discovered in metalloproteinases that are zinc ion-dependent (Jongeneel et al., 1989). In this instance, the coordination sphere contains two imidazole nitrogen atoms. Water molecules or oxygen atoms from the Glu carboxyl group may occupy the coordination sphere (Hooper, 1994).
3 Metal induced structural modifications for antimicrobial activity
Numerous AMPs rely on metal ions like Zn(II), Cu(II), and Fe(II) to exert their antimicrobial activity. Interactions between metal ions and ligands can facilitate the establishment of a precise configuration of AMPs; it is plausible that these peptides only bind to bacteria when metals are present, which leads to the cell wall permeabilization or instability. As discussed above, often metal coordination involves residues such as histidine, aspartic acid, and glutamic acid which, being pH-sensitive, undergo protonation enhancing the overall cationic nature of the AMP molecule and modifying its membrane interaction. Thus, AMPs often exhibit higher effectiveness upon coordination with metal ions, since these ions are likely altering their physicochemical properties, including local charge and/or structure (Łoboda et al., 2018), consequently emerging as promising candidates for antimicrobial therapies (Dudek et al., 2023; Miller et al., 2021).
Among metal-activated AMPs, certainly we have to note those that possess the amino terminal Cu(II) and Ni(II) binding motif, known as the ATCUN motif, which consists of two amide groups positioned between a free amine group at the N-terminus and a His residue at the third position and has a strong affinity for binding specific transition metal ions such as Cu(II), Ni(II), and Pd(II). The formation of a favourable 6-membered chelate ring, involving the deprotonated peptide nitrogen of histidine, rather than a 7-membered ring, supports the view that metal ion coordination predominantly occurs at the π nitrogen, rather than the τ nitrogen, of the imidazole ring. Thus, these peptides combine to produce metal complexes that are anchored at the free N-terminus and comprise three fused chelate rings (5-, 5-, and 6-membered ones), two intervening peptide nitrogen atoms and the histidine imidazole π nitrogen (4N). These complexes are the most stable peptide ligands due to the chelating effect and in fact at physiological pH, they reach femtomolar dissociation constants.
Here we report a selection of AMPs that rely on metal ions for their activity.
Bacitracin A is a cyclic peptide produced by Bacillus subtilis and Bacillus licheniformis, binding to a variety of divalent metals, which is widely used in animal feed as well as in human and veterinary medicine (Johnson et al., 1945). Bacitracin binds to undecaprenyl pyrophosphate, a key molecule for bacterial cell wall synthesis (Storm and Strominger, 1973). In detail, bacitracin causes bacterial death by blocking the movement of peptidoglycan precursors to the site of cell-wall formation by sequestering the lipid carrier (Toscano and Storm, 1982); to this aim, bacitracin needs to bind also a divalent metal ion creating a ternary 1:1:1 antibiotic:metal:lipid complex (Stone and Strominger, 1971; Economou et al., 2013). Although bacitracin is able to bind a variety of metals, its antibacterial activity is maximal when it is coupled to Zn(II). In fact, the zinc form of bacitracin is the one that is most frequently used in antibiotic formulations. A 1.10 Å x-ray structure of the complex Zn-bacitracin and undecaprenyl pyrophosphate (PDB code 4K7T) shows the coordination to both zinc and sodium (Economou et al., 2013). The crystal structure of the Zn-bacitracin-undecaprenyl pyrophosphate complex reveals a compact arrangement where bacitracin wraps around the lipid and metal ions, facilitating interaction with bacterial membranes (Economou et al., 2013). Bacitracin adopts a highly amphipathic structure, acting as a clasp to keep the pyrophosphate in a closed state with less than 2% of its surface area solvent accessible. The majority of the polar side chains of the bacitracin are directly involved in recognizing the metal or the pyrophosphate group, whilst its nonpolar side chains form a hydrophobic band surrounding the exit channel via which the lipid chain extends out of the binding site (Economou et al., 2013). Additionally, the amphipathic structure of the complex is probably helping in the association with the lipid membrane. Likely, in the presence of a bacterial membrane, the hydrophobic face will interact with the lipid membrane, favoring the crossing of the lipid undecaprenyl tail towards the membrane interior (Stone and Strominger, 1971). Zn(II) coordinates with an octahedral shape; donor atoms originate from the side chain of d-Glu (Cresti et al., 2024), the lipid pyrophosphate, the nitrogen of the thiazoline ring, the N-terminal amine, and a water molecule. (Figure 2A, gray sphere). Furthermore, none of the His side chains, which usually are critical for Zn(II) complexation, are involved. The coordination geometry of the sodium ion is somewhat distorted square pyramidal; five ligands, comprising two oxygen atoms from the pyrophosphate group, the side chain of d-Asp (Da Silva et al., 2021), and the carbonyl oxygens at the backbone of residues 5 and 8, coordinate the metal (Economou et al., 2013) (Figure 2A, green sphere).
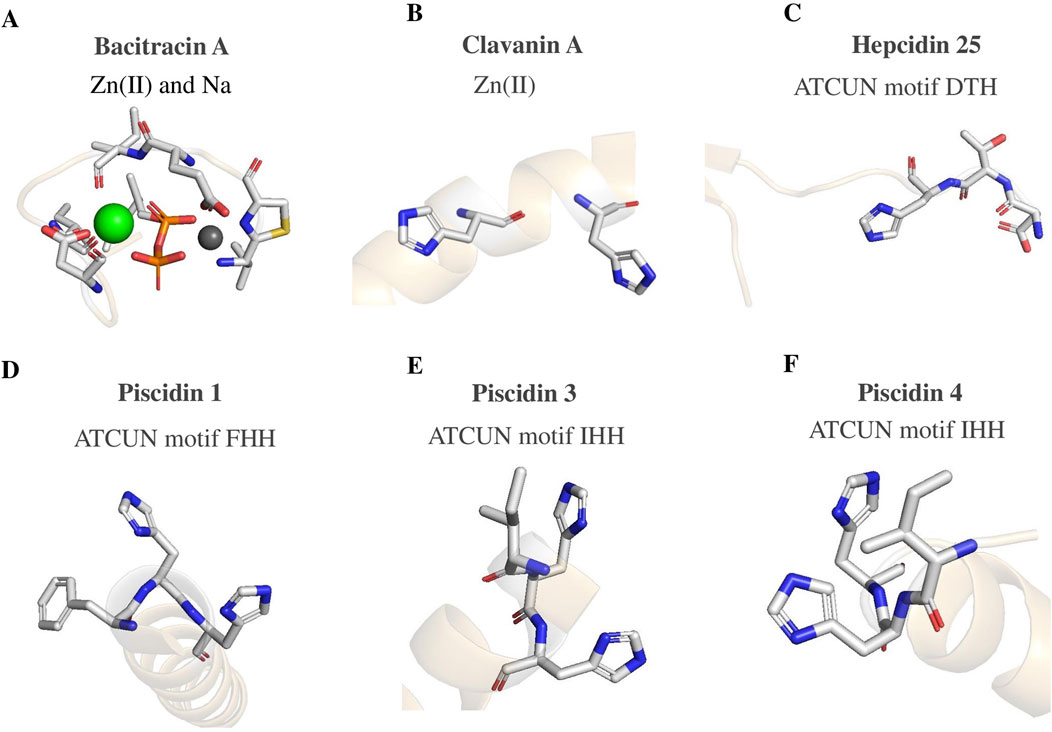
Figure 2. Metal coordination sites of: (A) Bacitracin A, PDB code 4K7T (Economou et al., 2013); (B) Clavanin A PDB code 6C41 (Silva et al., 2016); (C) Hepcidin25, PDB 4QAE; (D) Piscidin 1 PDB 6PF0 (Comert et al., 2019); (E) Piscidin 3 PDB 6PEZ (Comert et al., 2019); (F) Piscidin 4 PDB 5H2S. Coordinating residues are shown as sticks, metal ions as spheres.
Clavanin A, a 23-residue α-helical AMP derived from Styela clava, demonstrates broad-spectrum antimicrobial properties against both Gram-positive and Gram-negative bacteria, effective even in high ionic strength conditions (0.3 M NaCl). Unlike many AMPs, whose activities are restricted by pH, ClavA maintains activity across a wide range of pH, from 5.5 to 7.4, hinting at potentially diverse mechanisms of action that remain still to be elucidated. Remarkably, the presence of Zn(II) augments its antimicrobial efficacy by 16-fold, attributed to the chelation with His17 and His21, though the specific coordination complex has yet to be defined (Juliano et al., 2017). In fact, while the peptide structure in several environments is known (PDB code 6C41) (Silva et al., 2016), no coordination complex between ClavA and Zn(II) has been characterized. These histidine residues, positioned in an i, i + 4 arrangement (Figure 2B), may facilitate the peptide contact to the cell membrane by increasing the net positive charge at the C-terminal, thereby drawing ClavA closer for interaction. Consequently, the Phe residues initiate membrane penetration and ClavA aligns its hydrophilic and hydrophobic residues with the corresponding regions of the lipid bilayer, improving its chances of adopting an α-helical structure. Zn(II) acts as a molecular staple, stabilizing the α-helix by reducing conformational flexibility and promoting proper folding. Once the helix is stabilized, Zn(II) might detach from ClavA, interact with the membrane, and contribute to its deformation, thereby enhancing lipid reorganization beneficial to ClavA integration into the bilayer and its transition to a transmembrane form (Alexander et al., 2018).
Shepherin I and II are extracted from the roots of shepherd’s purse, showing efficacy against Gram-negative bacteria and fungi. The presence of Zn(II) enhances the antimicrobial activity of shepherin I (Wątły et al., 2023). Interestingly, Zn(II) binds to shepherin I, leading to the formation of fibrils with exceptional antifungal properties. This phenomenon is intriguing since the peptide alone lacks potent anticandidal action or fibril formation which are observed in the Zn(II)-shepherin I complex, highlighting how metal binding induces structural rearrangements impacting both morphology and biological activity. Shepherin II consists of 38 amino acid residues, eight of which are histidine residues, making it an ideal anchor for Cu(II) and Zn(II). The regular arrangement of six GGH repeats may enable the peptide to function as a metal-sponge (Park C. J. et al., 2000). Shepherin II exhibits robust antifungal activity against various fungi such as Candida albicans, Saccharomyces cerevisiae, and Cryptococcus neoformans along with activity against diverse Gram-negative bacteria (Park C. J. et al., 2000). The presence of the ATCUN motif is a distinct feature of Shepherin II. Thus, Zn(II) is crucial for Shepherin II to undergo a morphological transformation leading to fibril formation and enhanced antifungal activity (Alexander et al., 2018; Szarszoń et al., 2024).
Calcitermin, a 15-amino acid residue AMP present in the human airway fluid, exhibits activity primarily in acidic pH environments (pH 5.4), commonly observed during inflammation. It possesses an effective metal-binding domain consisting of residues His9, His11, and His13, along with the free terminal amino and carboxyl groups (Bellotti et al., 2019). Calcitermin adopts a helical conformation in membranes, and its antimicrobial activity against C. albicans and bacteria such as Staphylococcus aureus and Enterococcus faecalis is enhanced by Zn(II) and Cu(II) (Cole et al., 2001; Bellotti et al., 2023). Although it is not completely ruled out that the C-terminal carboxyl group, rather than Glu15, is involved in this coordination, comparative studies with other His-rich AMPs indicate that the Glu15 residue may further stabilize the Zn(II)-calcitermin complex in acidic conditions.
Histatins are His-rich peptides with antibacterial and antifungal properties secreted by human salivary glands secrete. Both Histatin 5 (Hst5) and Histatin 3 (Hst3) have ATCUN sequences and have a high affinity for Cu(II) and Ni(II), and a lower affinity for Zn(II). Specifically, they have four binding sites for nickel and copper but only one binding site for zinc, which is associated with the HEKHH sequence. The ATCUN motif of Hst5 in C. albicans, is likely responsible of ROS production, which is linked to both the inhibition of respiration and of cell development. Hst5 also contains two potential Zn(II) binding sites, HxxxHH and HExHH, and both sites follow the i, i + 4 motif that is also present in ClavA. A Zn(II)-bridged dimer is thought to be formed when Hst5 binds to Zn(II) with a stoichiometry of 2:2 Zn(II):Hst5, in which each Zn(II) coordinates to four His residues, two from each of the two Hst5 monomers. Indeed, the binding of Zn(II) to Hst5 appears to stabilize the peptide and when Zn(II) is present, Hst5 is also able to fuse negatively charged vesicles. Only at neutral and basic pH, where the imidazole side chains are deprotonated, the binding is observed, indicating the importance of His side chains in coordinating Zn(II) (Garstka et al., 2023).
Hepcidin-25 is a peptide that binds to the ferroportin protein and controls its expression, which is crucial for maintaining iron homeostasis (Loréal et al., 2014). Hepcidin is available in three different forms: hepcidin-25 with the ATCUN motif (Figure 2C, PDB code 4QAE), hepcidin-22 and hepcidin-20 without the motif. Hepcidin-25 can bind Cu(II), Ni(II), and Zn(II) (Tselepis et al., 2010). Unlike hepcidin-22 and hepcidin-20, binding of the copper reduces its tendency to aggregate at high concentrations. Numerous studies have shown that the ATCUN motif in hepcidin-25 inhibits peptide aggregation and contributes to its action. Interestingly, in the presence of reducing and oxidizing agents, plasmid DNA could be cleaved by an N-terminal derivative of hepcidin-25, but not by a derivative lacking the ATCUN motif. The addition of copper enhanced the antibacterial activity of hepcidin-25, indicating that the ATCUN motif might be involved in its capacity to inhibit bacteria (Maisetta et al., 2010). Hepcidin function in maintaining iron homeostasis may also be influenced by the ATCUN motif. In mouse models, substituting alanine for the third histidine led to a decrease in activity and an increase in serum iron levels.
Ixosin is released by ticks salivary glands of Ixodes sinensis and is a 23 amino acid AMP with antibacterial and antifungal properties (Libardo et al., 2016). The complex, after linking copper, is located into the bacterial membrane where it can cause local perturbations and rupture of the bilayer. The ATCUN motif (GLH) is required to produce ROS. Cu-ixosin produces ROS with the help of dioxygen. These ROSs then diffuse to and react with adjacent bilayer unsaturations, producing (per)oxidation of lipids. Other AMPs, such as ixosin B, target the (per)oxidized lipids, which results in a synergistic impact. Since they have a longer residence time in the membrane, they may produce non-transient pores, which although may not directly cause cell death, allow other antimicrobial effectors to continue their access to the bacterium, making it nearly hard for the bacteria to recover. It is worth noting that Cu−ATCUN complexes are highly catalytic in the generation of ROS. This implies that the same biological impact could be produced by even minute amounts of copper-bound ixosin (Alexander et al., 2018; Libardo et al., 2016).
Piscidins, are marine AMPs with broad-spectrum activity against viruses, fungi, and bacteria (Asensio-Calavia et al., 2023; Raju et al., 2021). The high ionic concentrations in marine environments pose no challenge to marine AMPs due to their evolutionary adaptations. The piscidin family includes members like epinecidin-1, myxinidin, chrysophin, diacentracin, pleurocidins, and moronecidins, and these peptides play critical roles in the innate immunity of fish (Asensio-Calavia et al., 2023; Raju et al., 2021). They are characterized by an abundance of cysteine and arginine and their relatively short length. Although their precise mechanism of action remains unclear, it is likely that they possess properties that enable them to bind to and disrupt membranes, leading to the formation of temporary pores or channels that result in microbial death. Additionally, emerging evidence suggests that these AMPs may also disrupt cellular processes such as protein and nucleic acid synthesis and enzymatic reactions (Asensio-Calavia et al., 2023). The antimicrobial efficacy of piscidin peptides, along with their resilience to variations in pH and ionic strength, are significantly impacted by the positioning and quantity of histidines. Specifically, histidine protonation is crucial as it enhances the overall cationic nature of the AMP molecule, influencing its interaction with the mainly anionic microbial membrane. Additionally, the capacity of these AMPs to bind with metals, such as Zn(II), and to form salt bridges either between peptide and membrane or among peptides themselves, plays a pivotal role in their ability to disrupt microbial membranes (Alexander et al., 2018). The ATCUN motif present in certain piscidins significantly enhances their antimicrobial effectiveness by enabling the coordination of Cu(II) (Figures 2D–F, PDB codes 6PF0, 6PEZ, 5H2S) (Paredes et al., 2020; Comert et al., 2019). For instance, peptides such as mononecidin and piscidin-3 feature these ATCUN motifs (Alexander et al., 2018). When these peptides are in presence of oxygen, their ATCUN motifs bind to Cu(II) with picomolar affinity. This Cu(II)-ATCUN complex formation under aerobic conditions can lead to increased oxidative stress, boosting the peptide bactericidal activity (Oludiran et al., 2019). Thus, by generating ROSs, the ATCUN-AMP sequence is believed to represent complex yet effective antibacterial machinery in vertebrates (Fu et al., 2021; Portelinha et al., 2021). It is interesting to note that when the histidine residue is substituted with alanine, myxinidin an AMP obtained from the epidermal mucus of hagfish, loses some of its effectiveness against Escherichia coli, Klebsiella pneumoniae, and S. aureus (Cantisani et al., 2014). Remarkably, using the bioinformatics tool MeBiPred (Aptekmann et al., 2022), further piscidin peptides have been identified as capable of binding Zn(II) [such as Pleurocidin-like WF4, from Pluteus americanus, and Chrysophsin-3, from Chrysophrys (Pagrus) major]. Other two piscidins peptides Chrysophsin-2 and Chrysophsin-1 (from P. major) were predicted and demonstrated to bind Cu(II) utilizing their ATCUN motifs. In aerobic environments, these Cu(II)-ATCUN complexes serve as sources of oxidative stress, thereby enhancing the peptides’ lethality against bacteria (Asensio-Calavia et al., 2023).
4 Host-guest competition for metal capture
4.1 Metal sequestering antimicrobial peptides
First row transition metals are an essential food for both host cells and infectious agents, as they serve to stabilize molecular structures, tune regulatory activity, or work as redox sites in respiratory chain enzymes. Bio-invaders are armed with a redundant arsenal of molecules, like siderophores, useful for stealing metals from host cells; in parallel, host cells have their own strategy to counteract enemies. Among other devices, mammals have developed defence mechanisms called nutritional immunity that limit the availability of specific metals to infectious agents and prevent the invasion of pathogens (Hood and Skaar, 2012; Murdoch and Skaar, 2022; Damo et al., 2016). Hosts can hinder the growth of bacteria by releasing AMPs responsible for sequestering metals. Among these we mention here lactoferrin (Damo et al., 2016; Kell et al., 2020), some specific proteins S100 and micropulsin (Alexander et al., 2018).
Lactoferrin is a protein belonging to the innate immune system of vertebrates. It is capable of reversibly binding two iron ions. In the early stage of infection, lactoferrin moves from special leukocyte granules to the site of infection to sequester iron and thereby block the uptake of Fe(III) by the bacteria. The protein is made up of two domains, the N-terminal one and the C-terminal one [PDB structure 1LFG (Haridas et al., 1995), 1B0L (Sun et al., 1999)]. Each domain has two subdomains of structure α and β, at the interface of which is the iron coordination pocket. The metal ligands, highly conserved in the transferrin family to which lactoferrin belongs, are composed of Tyr92, Tyr192, Asp60, His253 and two oxygen atoms from a carbonate ion (Mizutani et al., 2012) (Figure 3A). As expected for a protein responsible for the binding and controlled release of iron, the α and β portions of each subdomain can change their relative arrangement. The α and β portions are close or far from each other depending on whether the iron is bound or released. Only the close state, by recruiting Asp60 and His253, guarantees the exa-coordination required by the iron ion.
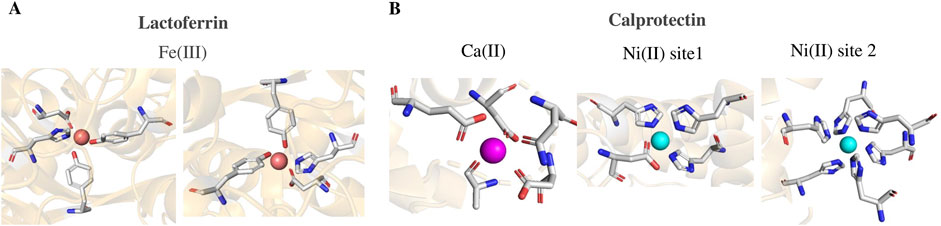
Figure 3. (A) Metal coordination sites of lactoferrin, PDB code 1LFG (Hood and Skaar, 2012); each metal pocket is due to Tyr92, Tyr192, Asp60, His53 (shown in the figure) plus a carboxylate ion (not shown). (B) Coordination sites of calprotectin, PDB code 5W1F (Mizutani et al., 2012); on the left the structure of one of the four sites for calcium, on the right the structures of sites 1 and 2 intended for transition metal ions and here occupied by Ni(II). Coordinating residues are shown as rods, metal ions as spheres.
The S100 protein subfamily is exclusive to vertebrates and is characterized by a distinctive conformational structure. These proteins play diverse roles in physiological processes, including calcium homeostasis, the elimination of reactive oxygen species (ROS) and nitric oxide, and various immune responses. The S100 multi-functionalities are extensively treated in a recent review by Singh and Ali (Singh and Ali, 2022). Although typically dimeric, S100 proteins can also form higher oligomeric structures. Each S100 monomer consists of two alpha helices connected by a flexible loop, known as the EF-hand motif (E helix - loop - F helix). Proper folding requires the binding of metal ions such as Ca(II), Zn(II), Cu(II), or Mn(II) (Gilston et al., 2016; Wheeler et al., 2016), which enables the proteins to dimerize or oligomerize effectively.
Calprotectin belongs to the S100 family. The role in health and disease of over twenty S100 proteins was recently reported by L.L. Gonzalez and co-authors (Gonzalez et al., 2020). Calprotectin is a multi-metal sequestering protein that is capable of coordinating Mn(II), Fe(II), Zn(II) and even Ni(II) (Nakashige et al., 2017). By removing these ions from the environment, this protein helps starve microbes. Calprotectin belongs to the innate immune system; it lives in neutrophils and epithelial cells from where it moves to reach sites of infection. Calprotectin has 4 EF domains, each coordinating a calcium ion (Figure 3B). For each domain, calcium presents a hepta-coordination with pentagonal bipyramidal geometry assisted by 5 binding sites coming from the loop and by a bidentate carboxylate ligand of a side chain of Asp or Glu belonging to the F helix (Gifford et al., 2007). This antimicrobial protein is composed of heterodimers formed by the S100 proteins called S100A8 (α) and S100A9 (β). Binding to calcium ions promotes the self-association of two αβ dimers to form the α2β2 tetramer. The tetramer, in turn, exhibits two extra coordination sites for transition metals of the first row. These, called site 1 (named His3Asp) and site 2 (named His6) are located at the interface between the two dimers. Site 1 binds Zn(II) with high affinity, but also Mn(II) and Fe(II) although with lower affinity. Site 2 binds Mn(II), Fe(II) and Zn(II) with increasing affinity (Nakashige et al., 2017). The calcium dependence of calprotectin implies that when present in the extracellular space at infection sites, where calcium levels are higher, it transits from a low-affinity state to a high-affinity state, allowing for tighter binding of zinc or manganese ions. Numerous studies have shown that calprotectin employs a sequestration mechanism to inhibit bacteria by binding zinc and manganese ions. Abscesses caused by S. aureus have been found to contain calprotectin, and animals deficient in calprotectin exhibited higher concentrations of S. aureus and metals. Treatment of media with calprotectin prior to inoculation led to decreased growth of S. aureus, an effect that was reversed upon addition of manganese and zinc, indicating that calprotectin suppresses bacterial growth. Although calprotectin antibacterial activity depends on zinc binding, its broad-spectrum activity is eliminated when the manganese-binding site is mutated, indicating that manganese sequestration may be more crucial for host defense against pathogens than zinc sequestration. Depending on the type of infection, calprotectin may bind both manganese and zinc to chelate both metals since various infections have varying needs for transition metals (Alexander et al., 2018). Finally, the X-ray structure [PDB code 5W1F (Nakashige et al., 2017)] shows that both sites 1 and 2 can be occupied by Ni(II) (Figure 3B). Site 2 has a great coordination affinity for Ni(II) even greater than that for zinc ion. These experimental results demonstrate that calprotectin represents a multi-metal scavenger with a broad range of antimicrobial action.
Psoriasin is an S100 family protein that binds Ca(II) and is activated in psoriatic skin and developing keratinocytes in the presence of calcium. It plays a role in the immune response by attracting CD4+ T-lymphocytes and neutrophils. The crystal structure of Ca(II)-bound psoriasin (PDB code 1PSR) reveals a Zn(II)-binding site formed by three histidine residues and one aspartate residue, similar to metalloproteases (Brodersen et al., 1998). Psoriasin exhibits antibacterial activity specifically against E. coli, which decreases when zinc is prebound, suggesting it removes zinc from bacteria to exert its effects. Moreover, keratinocytes release psoriasin, perhaps for skin protection (Alexander et al., 2018; Brodersen et al., 1998).
Microplusin is an AMP detected in the hemocytes, ovaries, and fat bodies of ticks. It comprises six cysteine residues that form three disulfide bonds and five α-helices, with N- and C-termini that are disordered. Besides its activity against S. aureus, microplusin exhibits antibacterial effects against various Gram-positive bacteria but not Gram-negative ones (Alexander et al., 2018). This disparity in activity could be attributed to the divergent physiological concentrations of copper found in each microorganism. The addition of copper reverses the inhibitory impact of microplusin on Micrococcus luteus and C. neoformans, implying its role in sequestering copper from pathogens. In C. neoformans, microplusin impedes melanization and the formation of the polysaccharide capsule, processes reliant on copper-dependent enzymes.
4.2 Metal sequestering by siderophores
Iron is an essential element for all living organisms (Correnti and Strong, 2012), involved in a variety of metabolic activities in aerobically growing microorganisms, including the reduction of oxygen for ATP generation, the functionalization of heme, and the reduction of DNA precursors. Despite its abundance in nature, ferric iron is not easily accessible in a dissolved form. This is caused by the precipitation of ferric hydroxide and its severe insolubility (Ksp = 10−38), which lowers the concentration of free iron at pH 7 to 10−8 M (Swayambhu et al., 2021). Thus living organisms have striven to acquire, transport and store iron in aerobic, Fe(III), as well as in reducing, Fe(II), conditions. Whilst animals use iron-binding proteins to deal with iron, bacteria use siderophores (iron carriers) to acquire iron from the environment and from the host cells (Correnti and Strong, 2012). Siderophores are low-molecular-weight secondary compounds (500–1,500 Da) indeed with high specificity and affinity towards Fe(III), produced by bacteria, fungi and plants under iron deficient conditions (Hider and Kong, 2010). Siderophores can also bind Fe(II), as well as other metals (zinc, molybdenum, vanadium, copper, etc.), but their affinity for Fe(III) is generally much higher (Johnstone and Nolan, 2015; Helman and Lawrence, 1989; Khan et al., 2018). Due to their strong ability to chelate metals, siderophores have raised a lot of interest from the medical and pharmaceutical scientific community (Ribeiro and Simões, 2019). Microbes have developed smart strategies for iron uptake. Once bound the iron free or coordinated to the host proteins, the iron-loaded siderophores are recognized by the receptors of the bacterial membrane and translocated into the cytoplasm (Swayambhu et al., 2021).
Apart from using their own siderophores to attack and destroy other species, certain microbes can exploit siderophores belonging to other microbe species (xenosiderophores). For example, microcins (Mcc) are AMPs produced and post-translationally modified with a siderophore moiety by Gram-negative bacteria such as E. coli and K. pneumoniae. Equipped in this way, these siderophore-AMPs can bind to the catecholate receptors of phylogenetically similar bacteria, enter and destroy them thus eliminating competitors for the same resources (Massip and Oswald, 2020). In the never-ending host-infectious agent battle, the host’s immune system has proteins, such as siderocalins, capable of sequestering the entire Fe(III)-siderophore complex when far away from its bacterial receptor (Khan et al., 2018). The ability of these host proteins to recognize foreign siderophores has led to investigate on possible endogenous siderophores and their role in intracellular iron homeostasis (Correnti and Strong, 2012; Devireddy et al., 2010; Kramer et al., 2020). The iron-binding moiety of a lipocalin(24p3)-associated mammalian siderophore 2,5-dihydroxybenzoic acid (2,5-DHBA) has been identified and is similar to the iron-binding component of bacterial enterobactin (2,3-DHBA) (Devireddy et al., 2010). Anyway, the whole mammalian siderophore structure remains unknown.
The principal iron-binding motifs of the more than 500 distinct siderophores that have been discovered and characterized thus far, include catecholate, hydroxamate, phenolate, carboxylate, and mixed, i.e., systems having several different types of iron-binding motifs (Hider and Kong, 2010; Kramer et al., 2020; Timofeeva et al., 2022; Wilson et al., 2016; Rayner et al., 2023; Ustiatik et al., 2021; Butler and Theisen, 2010). The crystal structures of several siderophores in apo form, loaded with metals or complexed with their receptors, reported in the literature so far, have greatly aided in the design of new artificial molecules for therapeutic purposes. Some examples of the above-mentioned classes are reported in Table 1.
Typically, natural siderophores coordinate Fe(III) through oxygen atoms, resulting in the formation of octahedral complexes. These complexes feature six electron-donating atoms symmetrically and spatially distributed around the central iron atom. The highest binding affinity is obtained when all six atoms interacting with iron are located within the same molecule, underscoring the chelate effect and the significance of multiple denticity. The octahedral field contributes to the formation of thermodynamically stable, high-spin Fe(III) particles (Timofeeva et al., 2022). Based on their chemical nature, siderophores can bind the Fe(III) through bidentate (for example, catecholate, hydroxamate) or polydentate (phenolates, nitrogen heterocycles, etc.) functional groups.
Catecholate siderophores show two ortho-phenolate groups and bind with high affinity to Fe(III) forming a hexadentate octahedral complex supplying two oxygen atoms for each catechol group (Ghosh et al., 2020). For example, Enterobactin, produced by E. coli, comprises three catecholamide groups linked by a trilactone ring and shows a very high affinity for Fe(III). In the crystal structure of the ferric enterobactin receptor from Pseudomonas aeruginosa (PfeA) in complex with enterobactin (PDB code 6Q5E), this last coordinated Fe(III) in an octahedral manner by the six hydroxyls of the three catecholates (Moynié et al., 2019). Azotochelin and Protochelin are other catecholate siderophores produced by the Gram-negative Azotobacter vinelandii. They are a bis-catechol and a tris-catechol siderophore, respectively (Cornish and Page, 1998). The crystal structures of PfeA-protochelin (PDB code 5NC4) and PfeA-azotochelin (PDB code 5NR2) show that protokelin always uses three catecholate groups for iron hexa-coordination, while azotokelin uses only two catecholate groups, so extra oxygen groups are needed to complete the iron coordination shell (Moynié et al., 2019). Phenolate siderophores are often included in catecholate groups, as they show only one phenolate group instead of two (Ghosh et al., 2020).
Hydroxamates are another important class of siderophores, showing a higher metal-chelating ability (Al Shaer et al., 2020). Hydroxamate siderophore mostly contains C (=O) N-(OH) R, where R is an amino acid or a derivative of it. Two oxygen atoms provided by each hydroxamate group form a bidentate ligand. Each hydroxamate can form a hexadentate octahedral complex with ferric ion (Khan et al., 2018; Ghosh et al., 2020). Ferrioxamine B and other hydroxamate siderophores, produced by several Streptomyces species, are used by pathogenic microorganisms, such as P. aeruginosa or S. aureus, to scavenge iron from plant and animal hosts. Structural studies, combining X-ray crystallography and other biophysical methods, have been carried out to elucidate how siderophore uptake across the bacterial membranes happens and to exploit this information for the uploading of drugs bound to siderophores (PDB codes 6I96, 6ENK) (Josts et al., 2019; Podkowa et al., 2014; Ronan et al., 2018).
Carboxyl and hydroxyl groups are present in carboxylate siderophores and bind to iron through carboxyl and hydroxyl groups (Timofeeva et al., 2022; Ghosh et al., 2020). Staphyloferrin A and Staphyloferrin B from S. aureus are an example of highly hydrophilic carboxylate-type siderophore. The X-ray structure of staphyloferrin A (SA) in complex with its HtsA receptor (PDB code 3LHS) shows the SA coordinates Fe(III) with distorted octahedral geometry where ferric iron is coordinated by an oxygen atom from each of β-hydroxy, β-carboxyl-substituted carboxylates groups (Grigg et al., 2010).
Mixed type siderophores contain both catecholate and hydroxamate group and/or other donor groups.
Yersiniabactin (Ybt) is a 5-member heterocycle siderophore, which is made up of one salicylate, one malonate, and three cyclized cysteine residues, produced by the Gram-negative Yersinia pestis. In ferric-Ybt (PDB code 6P6J), the iron atom is coordinated by three pairs of electrons from nitrogen and three negatively charged oxygen atoms, including a phenolate oxygen, a carboxylate oxygen, and the oxygen from a secondary alcohol. These six ligands are arranged in a distorted octahedral configuration (Miller et al., 2006).
Also, Pyochelin (Pch), from P. aeruginosa, contains five-member heterocycles as iron coordinating functional group. It is composed of two thiazoline and thiozolidine heterocyclic rings and binds Fe(III) by two nitrogen atoms of the thiazolin and thiazolidin rings, and two oxygen atoms of the phenolate and carboxylate groups. Two oxygen atoms of ethylene glycol bind to Fe(III) leading together with Pch to a hexa-coordinated state of iron (Cobessi et al., 2005) (PDB code 1XKW). Differently from Pch, Pyoverdin (Pvd) another siderophore from P. aeruginosa, does contain cathecolate/hydroxamate as iron chelating groups. Pvd chelates iron by using three bi-dentate groups: the catecholate of the chromophore and two hydroxamates from two modified amino acids on the peptide chain (PDB code 2W6U) (Greenwald et al., 2009).
5 Engineered metal-AMPs
The overuse and/or misuse of antibiotics for the treatment of bacterial infections has caused an increasing bacterial resistance to almost all known antibiotics so pushing the research community to develop new approaches to fight bacterial infections and to increase the permeability of the drug into the multi-drug-resistant cells. In this perspective, siderophores can be redirected to beneficial applications such as the selective administration of antibiotics/antimicrobial peptides, which can circumvent the problem of resistance development. Siderophore conjugation is mainly used for two applications in antimicrobial therapy: a) siderophore-mediated drug delivery known as Trojan Horse strategy, b) iron starvation by competitive chelation (Ribeiro and Simões, 2019) (Figure 4).
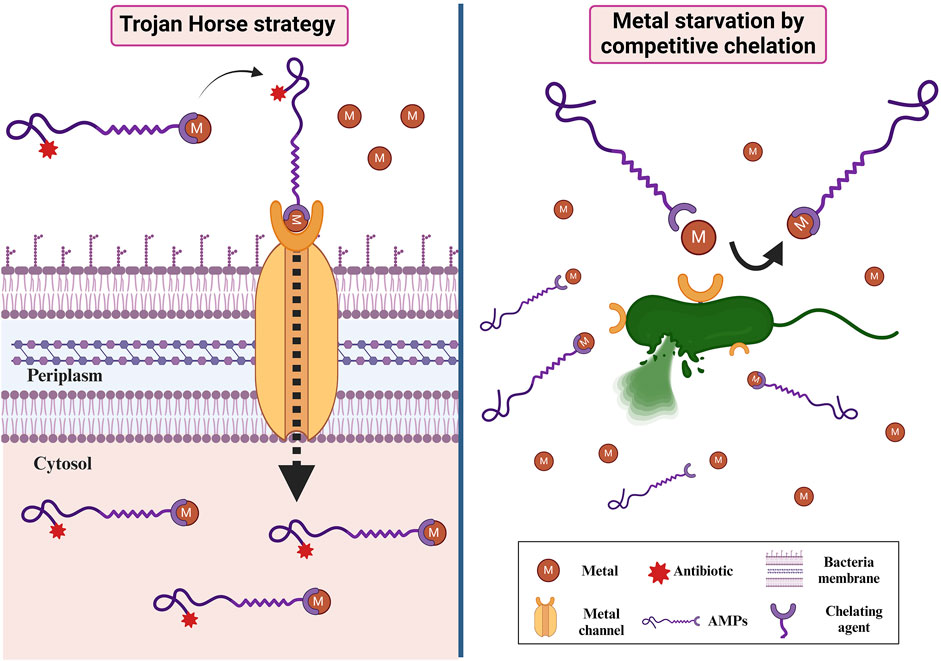
Figure 4. Applications in antimicrobial therapy exploiting conjugation of metal chelating agents: in the left side is reported siderophore-mediated drug delivery known as Trojan Horse strategy, in the right side is reported iron starvation by competitive chelation.
The Trojan Horse strategy utilizes siderophores as vectors for delivery of antimicrobial agents inside the bacterial cells (Ribeiro and Simões, 2019; Frei et al., 2023). This approach offers a notable advantage by enabling precise targeting of specific bacteria, as mammalian cells lack siderophore transporters. This allows the technique to take advantage of iron uptake pathways to enter bacteria (Olshvang et al., 2023). Antibiotics/AMPs have the potential to be linked with either natural or synthetic siderophores. This conjugation allows them to be recognized by outer membrane receptors present in pathogens, thereby providing an alternative cellular entry pathway. Exploiting this strategy can significantly decrease the minimum inhibitory concentration (MIC) of the antibiotic, sometimes by nearly 100-fold. Numerous artificial hybrids containing hydroxamate chelators have been reported thus far, with cargos ranging from cephalosporines to fluoroquinolones; in 2019, cefiderocol (Shionogi & Co.) was the first metallophore–antibiotic conjugate approved for clinical use by the US Food and Drug Administration (FDA) and later by the European Medicines Agency (2020) (McCreary et al., 2021; Choi and McCarthy, 2018). This drug is made up of a cephalosporin antibiotic cargo and a catechol chelating unit. In addition to antibiotics, siderophores can be used to transport toxic metals (such as gallium, aluminum, and cadmium) to specific microorganisms (Frei et al., 2023). The Trojan Horse strategy is also used for increasing the activity of AMPs facilitating their crossing through the outer-membrane barrier of Gram-negative pathogens. For example, Olshvang et al. (2023) conjugated different kinds of siderophores to a short AMP [K(RW)3] to overcome the outer-membrane barrier of P. aeruginosa PAO1 exploiting the transport through the siderophore-transporters. They linked to the AMP, both deferoxamine and bidentate hydroxamate chelator as natural and synthetic siderophore, respectively, and displayed that these siderophore-vectors increased the activity of K(RW)3 against P. aeruginosa ATCC and genetically modified strains unable to synthesize siderophores (Olshvang et al., 2023).
Siderophores can successfully inhibit microorganism growth by starving target cells through removal of iron from the surrounding environment. Microorganisms and other undesirable cells (such as cancer cells) may be among these. Siderophores not recognized by microorganisms (i.e., those lacking required production and transport mechanisms) are excellent options for inducing death of pathogens caused by iron deficit, since the target cells would not be able to use or transport the siderophore that is sequestering environmental iron.
The unravelling of natural sideromycins activity opened the door for research into new conjugates. This led to the focus on adjusting the chelators’ structures and changing the antibacterial moiety, which produced effective tools for combating microorganisms that are resistant to multiple drugs.
For example, Bellavita et al. (2023d) showed that the introduction of a hydroxamate-based siderophore increased the activity of a cyclic-temporin-like derivative against Gram-negative superbugs, such as K. pneumoniae and E. coli. The hydroxamate chelator can sequestrate Fe(III) that is essential for the bacterial growth and to induce the bacterial death. In this work, the authors covalently linked the chelator to the temporin-derivative through a long linker (16 atoms) to guarantee the exposure of the hydroxamate unit to chelate Fe(III) present in the cell-culture medium, causing an inhibition of the bacterial growth at very low MIC values (3.12 μM and 6.25 μM) (Bellavita et al., 2023d). The same strategy was also used by Oh et al. (2021), which have incorporated the catecholate moiety as Fe(III) chelator in the sequence of an antimicrobial macrocyclic peptoid. Through different techniques including UV-vis, fluorescence, and EPR spectroscopies, they showed a strong ability of the catecholate–AMP conjugate to bind Fe(III) with the affinity similar to the natural deferoxamine.
A similar approach was used by Libardo et al. (2014) introducing ATCUN motifs to AMPs able to form Cu(II)–complex and induce the ROS formation. Remarkably, Libardo et al. (2014) and Libardo et al. (2015) chose three ATCUN binding motifs (two highly active sequences and a weakly active ATCUN sequence as a control) to conjugate to the amino terminus of three AMPs with distinct modes of action: sh-buforin (Park C. B. et al., 2000), a non-membrane-active peptide, and the membrane-disrupting peptides, anoplin (Dos Santos Cabrera et al., 2008) and PAP (Javadpour et al., 1996). The objective was to create dual-activity compounds that combine the oxidative stress caused by the reactivity of the ATCUN motif with the potency and selectivity of AMPs to produce a novel class of metal-binding AMPs with enhanced efficacies. Interestingly, the molecular mode of action of ATCUN–AMPs varies among peptides and is primarily dependent on the parent peptide, even though they can stimulate the generation of ROS. Buforin II targets nucleic acids by localizing in the cytoplasm of the cell to carry out its action. When the ATCUN motif is added to sh-buforin, the cell produces more ROS and has lower MICs. The ATCUN derivatives of buforin appear to have the same target as the parent peptide because they oxidatively cleaved DNA rather than permeabilizing the membrane. The two AMPs targeting the cell membrane of bacteria, anoplin and PAP, have broad-spectrum efficacy against them. The ATCUN derivatives of these two peptides also target the cell membrane but exhibit higher antibacterial activity than the PAP derivatives, whose action is associated only with an increase in amphipathicity. In the case of anoplin, Libardo et al. (2014) designed and synthesized two new CuII-binding peptides, RTH–and LKH–Anoplin, that resulted to be more potent than Anoplin, showing a good ability to form Cu(II) complex and induce the ROS formation by causing a lipid damage in the bacterial membrane.
Boyce et al. (2020) recently reported an intriguing study in which they designed conjugates with an amine-containing antibiotic, a protease-cleavable linker, and an N-terminal siderophore to facilitate uptake. By employing this method, scientists were able to convert daptomycin, which is often only effective against Gram-positive bacteria, so that it could also target Acinetobacter species that are Gram-negative. Similarly, they demonstrated the delivery of macrolides and oxazolidinone antibiotics into several Gram-negative bacteria with the help of siderophores.
Recently, Stepanova et al. (2024) reported about the synthesis of novel complex polymeric conjugates of the peptide antibiotic colistin. In particular, they used a biocompatible and water-soluble synthetic glycopolymer as the carrier and they linked the siderophore deferoxamine (DFOA) and vitamin B12. Conjugates containing DFOA complexed with Fe3+ showed increased antimicrobial activity against P. aeruginosa compared to other conjugates.
An intriguing approach that has recently been published consists of the titanium immobilization of AMPs, possibly preventing implant failure and the development of sepsis (Skerlavaj and Boix-Lemonche, 2023; Drexelius and Neundorf, 2021). There are two different strategies for titanium coatings to AMPs; the first one consists of the incorporation of titanium-binding moieties into AMPs and then they coat onto the specific substrates; the second one consists of binding the AMP to a titanium-based layer featured by a functional group that links covalently AMPs. In both cases, this functionalization does not induce a loss of the antimicrobial activity but can improve issues related to their clinical use, such as stability. For example, Yazici et al. (2016) used the first strategy by attaching two different titanium-binding sequences, TiBP1 (RPRENRGRERGL) and TiBP2 (SRPNGYGGSESS) via a triple-glycine linker, to the AMP E14LKK. Both peptides were then coated on titanium surfaces and they showed a significant reduction in the S. epidermis and E. coli colonization. For instance, a polydopamine (PD) linker-based layer is commonly used to coat an AMP on the titanium surface (Ryu et al., 2018). For example, Trzcińska et al. have used this strategy to link three LL-37-derived peptides (more active than LL-37), to a titanium alloy, Ti6Al4V (Trzcińska et al., 2020). After the coating, AMPs showed long-term stability and approximately 40%–50% of all peptides were released from the surface after 30 days. These results demonstrated that all peptides could provide long-term antimicrobial activity when coated on metal surfaces.
6 Concluding remarks
AMPs are attractive molecules for drug development, but before they can be regarded seriously as viable treatments, additional work needs to be done to make sure they are successfully delivered, suitably targeted, and exhibit enhanced efficacy in vivo. Certain AMPs can also increase their activity by coordinating metals. These AMPs sequester vital metals from bacteria and/or change their structure in response to metals. Therefore, there has been growing interest in enhancing the activity of AMPs by incorporating a metal-binding unit. This approach aims to exploit one of the above reported mechanisms of action involving metals.
In drug design, it is widely recognized that developing new antibiotics with enhanced and broad-spectrum antimicrobial activity is more achievable when targeting multiple pathways in microorganisms. Siderophores that are not recognized by microorganisms lacking the appropriate production and transport mechanisms, emerge as prime candidates for inducing pathogen death due to iron deficiency. Many studies have evaluated the effect of conjugating the ATCUN motif to AMPs. Therefore, agents like the ATCUN–AMPs discussed in this review are promising lead compounds that deserve further investigation to fully understand their mechanisms of action and potential therapeutic applications. Furthermore, literature data clearly show that metals play a pivotal role in many pathologies and understanding peptide metal complex formation is key not only for antimicrobial therapeutical approaches. As an example, cancer cells often increase the expression of iron-importing receptors while reducing the expression of iron-exporting machinery. This strategy enhances their ability to acquire iron, facilitating their growth and proliferation. Siderophores possess the ability to either outcompete cancer cells for iron or transfer harmful metals (such as gallium and copper) to the cancer cell proximity, which can result in decreased cell proliferation or even death. The use of peptide binding metals represents an avenue to explore in this scenario.
Metal ions have the power to dramatically alter the redox activity and aggregation of the proteins and peptides involved; for instance, dyshomeostasis of metal ions has been linked to type 2 diabetes (T2D), Alzheimer’s disease (AD), and Parkinson’s disease (PD). Copper imbalance has been detected in several illnesses involving the central nervous system, including AD. For instance, amyloidogenic peptides may interact with metal ions like copper and zinc, and this interaction may have a significant effect on the aggregation and toxicity of the resulting compounds. This suggests that metal ions may be a key factor in the development of these diseases (Barnham and Bush, 2008; DeToma et al., 2012; Atrián-Blasco et al., 2018). A number of investigations have discovered a comparatively elevated concentration of metal ions (Zn, Cu, and Fe) in aggregates that are responsible of these pathologies. One factor to consider is that metal binding in the cytosol is a low probability scenario due to the comparatively low binding constant for these peptides and proteins. On the other hand, it is possible that Cu(II) and Zn(II) interact with intrinsically disordered proteins or peptides (IDPs) in the extracellular environment. For AD, the concept of a labile Cu(II) pool has been suggested. Likewise, areas with elevated concentrations of metal ions, particularly those that are loosely bound, such as in beta-cells within the pancreas, could play a crucial role in the interaction between IDPs and these metal ions. Furthermore, certain alterations in the concentration of metal ions may be necessary, potentially increasing the pool of available metal ions and facilitating their binding (Kowalik-Jankowska et al., 2003; Syme et al., 2004; Karr et al., 2004). The kinetics of the process or the morphologies of the aggregates formed when peptides with intrinsic disorder assemble can be influenced by metal ions, such as zinc and copper. Cu(II) has the potential to be a highly effective catalysts in the reduction of dioxygen, which produces ROS. Diabetes, PD, AD, and other amyloidogenic illnesses have all been linked to oxidative stress. The degree to which Cu combines with amyloidogenic peptides and proteins directly contributes to the observed oxidative stress is unknown.
Interestingly, metals also play a pivotal role in viral diseases. For example, zinc finger domains are commonly found in antiviral proteins, and FDA-approved drugs designed to eject zinc from these domains are currently available on the market (Sargsyan et al., 2020). By attaching to cysteine, these zinc-ejecting drugs break the zinc-cysteine tetrahedral complex and release the zinc. One of the two cysteine proteases that cause SARS-CoV-2 to mature and become infectious, papain-like protease (PLpro), is a promising target for COVID-19 treatment. PLpro contains a zinc-finger domain crucial for substrate binding and structural stability. Drugs like disulfiram and ebselen, known as zinc ejectors, destabilize PLpro, thereby reducing its catalytic activity. They could be utilized alongside other antiviral drugs as part of a multi-target approach to treating COVID-19 (Shetler et al., 2022).
A recent alternative approach for inhibiting NS2B-NS3 from Zika involves designing specific metallo- peptide inactivators. These inactivators consist of a catalytic amino-terminal copper and nickel motif along with a target-specific binding motif (Pinkham et al., 2018; da Silva-Júnior and de Araújo-Júnior, 2019). Incorporating metals such as Cu, Co, Fe, and Ni has been reported in studies involving peptidomimetic compounds with antiviral activity. Additionally, these metallo-peptidomimetic compounds possess the ability to deactivate certain proteins by generating ROS catalyzed by metals.
The fight against antibiotic resistance and other human diseases involves the combined use of AMP and metals. Based on the historical background of peptide-metal interaction, scientists face a promising challenge for the future.
Author contributions
LF: Writing–review and editing, Writing–original draft, Validation, Supervision, Project administration, Conceptualization. SB: Writing–review and editing, Writing–original draft, Methodology, Formal Analysis, Data curation. RB: Writing–review and editing, Writing–original draft, Methodology, Investigation, Formal Analysis, Data curation. GD’A: Writing–review and editing, Writing–original draft, Validation, Supervision, Project administration, Investigation, Conceptualization. AF: Writing–review and editing, Writing–original draft, Investigation, Data curation, Conceptualization. SG: Writing–review and editing, Writing–original draft, Validation, Project administration, Investigation, Data curation, Conceptualization.
Funding
The author(s) declare that no financial support was received for the research, authorship, and/or publication of this article.
Conflict of interest
The authors declare that the research was conducted in the absence of any commercial or financial relationships that could be construed as a potential conflict of interest.
The author(s) declared that they were an editorial board member of Frontiers, at the time of submission. This had no impact on the peer review process and the final decision.
Publisher’s note
All claims expressed in this article are solely those of the authors and do not necessarily represent those of their affiliated organizations, or those of the publisher, the editors and the reviewers. Any product that may be evaluated in this article, or claim that may be made by its manufacturer, is not guaranteed or endorsed by the publisher.
References
Alexander, J. L., Thompson, Z., and Cowan, J. A. (2018). Antimicrobial metallopeptides. ACS Chem. Biol. 13 (4), 844–853. doi:10.1021/acschembio.7b00989
Al Shaer, D., Al Musaimi, O., de la Torre, B. G., and Albericio, F. (2020). Hydroxamate siderophores: natural occurrence, chemical synthesis, iron binding affinity and use as Trojan horses against pathogens. Eur. J. Med. Chem. 208, 112791. doi:10.1016/j.ejmech.2020.112791
Aptekmann, A. A., Buongiorno, J., Giovannelli, D., Glamoclija, M., Ferreiro, D. U., and Bromberg, Y. (2022). mebipred: identifying metal-binding potential in protein sequence. Bioinformatics 38 (14), 3532–3540. doi:10.1093/bioinformatics/btac358
Asensio-Calavia, P., González-Acosta, S., Otazo-Pérez, A., López, M. R., Morales-delaNuez, A., and Pérez de la Lastra, J. M. (2023). Teleost piscidins-in silico perspective of natural peptide antibiotics from marine sources. Antibiot. (Basel, Switzerland) 12 (5), 855. doi:10.3390/antibiotics12050855
Atrián-Blasco, E., Gonzalez, P., Santoro, A., Alies, B., Faller, P., and Hureau, C. (2018). Cu and Zn coordination to amyloid peptides: from fascinating chemistry to debated pathological relevance. Coord. Chem. Rev. 375, 38–55. doi:10.1016/j.ccr.2018.04.007
Bal, W., Dyba, M., and Kozłowski, H. (1997). The impact of the amino-acid sequence on the specificity of copper(II) interactions with peptides having nonco-ordinating side-chains. Acta biochim. Pol. 44 (3), 467–476. doi:10.18388/abp.1997_4397
Barnham, K. J., and Bush, A. I. (2008). Metals in Alzheimer’s and Parkinson’s diseases. Curr. Opin. Chem. Biol. 12 (2), 222–228. doi:10.1016/j.cbpa.2008.02.019
Bellavita, R., Braccia, S., Galdiero, S., and Falanga, A. (2023c). Glycosylation and lipidation strategies: approaches for improving antimicrobial peptide efficacy. Pharm. (Basel) 16 (3), 439. doi:10.3390/ph16030439
Bellavita, R., Buommino, E., Casciaro, B., Merlino, F., Cappiello, F., Marigliano, N., et al. (2022). Synthetic amphipathic β-sheet temporin-derived peptide with dual antibacterial and anti-inflammatory activities. Antibiot. (Basel, Switzerland) 11 (10), 1285. doi:10.3390/antibiotics11101285
Bellavita, R., Falanga, A., Merlino, F., D’Auria, G., Molfetta, N., Saviano, A., et al. (2023a). Unveiling the mechanism of action of acylated temporin L analogues against multidrug-resistant Candida albicans. J. Enzyme Inhib. Med. Chem. 38 (1), 36–50. doi:10.1080/14756366.2022.2134359
Bellavita, R., Leone, L., Maione, A., Falcigno, L., D’Auria, G., Merlino, F., et al. (2023d). Synthesis of temporin L hydroxamate-based peptides and evaluation of their coordination properties with iron(III). Dalton Trans. (Cambridge, England : 2003) 52 (13), 3954–3963. doi:10.1039/d2dt04099a
Bellavita, R., Maione, A., Braccia, S., Sinoca, M., Galdiero, S., Galdiero, E., et al. (2023b). Myxinidin-derived peptide against biofilms caused by cystic fibrosis emerging pathogens. Int. J. Mol. Sci. 24 (4), 3092. doi:10.3390/ijms24043092
Bellotti, D., D’Accolti, M., Pula, W., Huang, N., Simeliere, F., Caselli, E., et al. (2023). Calcitermin-loaded smart gels activity against Candida albicans: a preliminary in vitro study. Gels 9 (2), 165. doi:10.3390/gels9020165
Bellotti, D., Toniolo, M., Dudek, D., Mikołajczyk, A., Guerrini, R., Matera-Witkiewicz, A., et al. (2019). Bioinorganic chemistry of calcitermin - the picklock of its antimicrobial activity. Dalton Trans. 48 (36), 13740–13752. doi:10.1039/c9dt02869b
Biswaro, L. S., da Costa Sousa, M. G., Rezende, T. M. B., Dias, S. C., and Franco, O. L. (2018). Antimicrobial peptides and nanotechnology, recent advances and challenges. Front. Microbiol. 9, 855. doi:10.3389/fmicb.2018.00855
Biswas, R., Jangra, B., Ashok, G., Ravichandiran, V., and Mohan, U. (2024). Current strategies for combating biofilm-forming pathogens in clinical healthcare-associated infections. Indian J. Microbiol. 64 (3), 781–796. doi:10.1007/s12088-024-01221-w
Boyce, J. H., Dang, B., Ary, B., Edmondson, Q., Craik, C. S., DeGrado, W. F., et al. (2020). Platform to discover protease-activated antibiotics and application to siderophore-antibiotic conjugates. J. Am. Chem. Soc. 142 (51), 21310–21321. doi:10.1021/jacs.0c06987
Brodersen, D. E., Etzerodt, M., Madsen, P., Celis, J. E., Thøgersen, H. C., Nyborg, J., et al. (1998). EF-hands at atomic resolution: the structure of human psoriasin (S100A7) solved by MAD phasing. Structure 6 (4), 477–489. doi:10.1016/s0969-2126(98)00049-5
Butler, A., and Theisen, R. M. (2010). Iron(III)-siderophore coordination chemistry: reactivity of marine siderophores. Coord. Chem. Rev. 254 (3-4), 288–296. doi:10.1016/j.ccr.2009.09.010
Cantisani, M., Finamore, E., Mignogna, E., Falanga, A., Nicoletti, G. F., Pedone, C., et al. (2014). Structural insights into and activity analysis of the antimicrobial peptide myxinidin. Antimicrob. agents Chemother. 58 (9), 5280–5290. doi:10.1128/AAC.02395-14
Choi, J. J., and McCarthy, M. W. (2018). Cefiderocol: a novel siderophore cephalosporin. Expert Opin. Investig. Drugs 27 (2), 193–197. doi:10.1080/13543784.2018.1426745
Cobessi, D., Celia, H., and Pattus, F. (2005). Crystal structure at high resolution of ferric-pyochelin and its membrane receptor FptA from Pseudomonas aeruginosa. J. Mol. Biol. 352 (4), 893–904. doi:10.1016/j.jmb.2005.08.004
Cole, A. M., Kim, Y. H., Tahk, S., Hong, T., Weis, P., Waring, A. J., et al. (2001). Calcitermin, a novel antimicrobial peptide isolated from human airway secretions. FEBS Lett. 504 (1-2), 5–10. doi:10.1016/s0014-5793(01)02731-4
Comert, F., Greenwood, A., Maramba, J., Acevedo, R., Lucas, L., Kulasinghe, T., et al. (2019). The host-defense peptide piscidin P1 reorganizes lipid domains in membranes and decreases activation energies in mechanosensitive ion channels. J. Biol. Chem. 294 (49), 18557–18570. doi:10.1074/jbc.RA119.010232
Cornish, A. S., and Page, W. J. (1998). The catecholate siderophores of Azotobacter vinelandii: their affinity for iron and role in oxygen stress management. Microbiology 144 (7), 1747–1754. doi:10.1099/00221287-144-7-1747
Correnti, C., and Strong, R. K. (2012). Mammalian siderophores, siderophore-binding lipocalins, and the labile iron pool. J. Biol. Chem. 287 (17), 13524–13531. doi:10.1074/jbc.R111.311829
Cresti, L., Cappello, G., and Pini, A. (2024). Antimicrobial peptides towards clinical application-A long history to Be concluded. Int. J. Mol. Sci. 25 (9), 4870. doi:10.3390/ijms25094870
Damo, S., and Kehl-Fie, T. E. (2016). “Metal sequestration: an important contribution of antimicrobial peptides to nutritional immunity,” in Antimicrobial peptides: role in human health and disease. Editors J. Harder, and J.-M. Schröder (Cham: Springer International Publishing), 89–100.
Da Silva, J., Leal, E. C., and Carvalho, E. (2021). Bioactive antimicrobial peptides as therapeutic agents for infected diabetic foot ulcers. Biomolecules 11 (12), 1894. doi:10.3390/biom11121894
da Silva-Júnior, E. F., and de Araújo-Júnior, J. X. (2019). Peptide derivatives as inhibitors of NS2B-NS3 protease from dengue, West Nile, and Zika flaviviruses. Bioorg. Med. Chem. 27 (18), 3963–3978. doi:10.1016/j.bmc.2019.07.038
DeToma, A. S., Salamekh, S., Ramamoorthy, A., and Lim, M. H. (2012). Misfolded proteins in Alzheimer's disease and type II diabetes. Chem. Soc. Rev. 41 (2), 608–621. doi:10.1039/c1cs15112f
Devireddy, L. R., Hart, D. O., Goetz, D. H., and Green, M. R. (2010). A mammalian siderophore synthesized by an enzyme with a bacterial homolog involved in enterobactin production. Cell 141 (6), 1006–1017. doi:10.1016/j.cell.2010.04.040
Donaghy, C., Javellana, J. G., Hong, Y. J., Djoko, K., and Angeles-Boza, A. M. (2023). The synergy between zinc and antimicrobial peptides: an insight into unique bioinorganic interactions. Molecules 28 (5), 2156. doi:10.3390/molecules28052156
Dos Santos Cabrera, M. P., Arcisio-Miranda, M., Broggio Costa, S. T., Konno, K., Ruggiero, J. R., Procopio, J., et al. (2008). Study of the mechanism of action of anoplin, a helical antimicrobial decapeptide with ion channel-like activity, and the role of the amidated C-terminus. J. Pept. Sci. 14 (6), 661–669. doi:10.1002/psc.960
Drexelius, M. G., and Neundorf, I. (2021). Application of antimicrobial peptides on biomedical implants: three ways to pursue peptide coatings. Int. J. Mol. Sci. 22 (24), 13212. doi:10.3390/ijms222413212
Dudek, D., Miller, A., Hecel, A., Kola, A., Valensin, D., Mikołajczyk, A., et al. (2023). Semenogelins armed in Zn(II) and Cu(II): may bioinorganic chemistry help nature to cope with Enterococcus faecalis? Inorg. Chem. 62 (34), 14103–14115. doi:10.1021/acs.inorgchem.3c02390
Dudev, T., and Lim, C. (2000). Tetrahedral vs. octahedral zinc complexes with ligands of biological interest: a DFT/CDM study. J. Am. Chem. Soc. 122 (45), 11146–11153. doi:10.1021/ja0010296
Economou, N. J., Cocklin, S., and Loll, P. J. (2013). High-resolution crystal structure reveals molecular details of target recognition by bacitracin. Proc. Natl. Acad. Sci. U S A. 110 (35), 14207–14212. doi:10.1073/pnas.1308268110
Frei, A., Verderosa, A. D., Elliott, A. G., Zuegg, J., and Blaskovich, M. A. T. (2023). Metals to combat antimicrobial resistance. Nat. Rev. Chem. 7 (3), 202–224. doi:10.1038/s41570-023-00463-4
Fu, R., Rooney, M. T., Zhang, R., and Cotten, M. L. (2021). Coordination of redox ions within a membrane-binding peptide: a tale of aromatic rings. J. Phys. Chem. Lett. 12 (18), 4392–4399. doi:10.1021/acs.jpclett.1c00636
Galdiero, S., Falanga, A., Berisio, R., Grieco, P., Morelli, G., and Galdiero, M. (2015). Antimicrobial peptides as an opportunity against bacterial diseases. Curr. Med. Chem. 22 (14), 1665–1677. doi:10.2174/0929867322666150311145632
Garstka, K., Dzyhovskyi, V., Wątły, J., Stokowa-Sołtys, K., Świątek-Kozłowska, J., Kozłowski, H., et al. (2023). CH vs. HC-promiscuous metal sponges in antimicrobial peptides and metallophores. Molecules 28 (10), 3985. doi:10.3390/molecules28103985
Ghosh, S. K., Bera, T., and Chakrabarty, A. M. (2020). Microbial siderophore – a boon to agricultural sciences. Biol. Control 144, 104214. doi:10.1016/j.biocontrol.2020.104214
Gifford, J. L., Walsh, M. P., and Vogel, H. J. (2007). Structures and metal-ion-binding properties of the Ca2+-binding helix-loop-helix EF-hand motifs. Biochem. J. 405 (2), 199–221. doi:10.1042/BJ20070255
Gilston, B. A., Skaar, E. P., and Chazin, W. J. (2016). Binding of transition metals to S100 proteins. Sci. China Life Sci. 59 (8), 792–801. doi:10.1007/s11427-016-5088-4
Gonzalez, L. L., Garrie, K., and Turner, M. D. (2020). Role of S100 proteins in health and disease. Biochimica biophysica acta. Mol. cell Res. 1867 (6), 118677. doi:10.1016/j.bbamcr.2020.118677
Greenwald, J., Nader, M., Celia, H., Gruffaz, C., Geoffroy, V., Meyer, J. M., et al. (2009). FpvA bound to non-cognate pyoverdines: molecular basis of siderophore recognition by an iron transporter. Mol. Microbiol. 72 (5), 1246–1259. doi:10.1111/j.1365-2958.2009.06721.x
Grigg, J. C., Cooper, J. D., Cheung, J., Heinrichs, D. E., and Murphy, M. E. P. (2010). The Staphylococcus aureus siderophore receptor HtsA undergoes localized conformational changes to enclose staphyloferrin A in an arginine-rich binding pocket. J. Biol. Chem. 285 (15), 11162–11171. doi:10.1074/jbc.M109.097865
Haridas, M., Anderson, B. F., and Baker, E. N. (1995). Structure of human diferric lactoferrin refined at 2.2 A resolution. Acta Crystallogr. D. Biol. Crystallogr. 51 (Pt 5), 629–646. doi:10.1107/S0907444994013521
Helman, R., and Lawrence, G. D. (1989). The increase in ferrioxamine B reduction potential with increasing acidity of the medium. Bioelectrochemistry Bioenergetics 22 (3), 187–196. doi:10.1016/0022-0728(89)87263-8
Hider, R. C., and Kong, X. (2010). Chemistry and biology of siderophores. Nat. Prod. Rep. 27 (5), 637–657. doi:10.1039/b906679a
Hood, M. I., and Skaar, E. P. (2012). Nutritional immunity: transition metals at the pathogen-host interface. Nat. Rev. Microbiol. 10 (8), 525–537. doi:10.1038/nrmicro2836
Hooper, N. M. (1994). Families of zinc metalloproteases. FEBS Lett. 354 (1), 1–6. doi:10.1016/0014-5793(94)01079-x
Javadpour, M. M., Juban, M. M., Lo, W. C., Bishop, S. M., Alberty, J. B., Cowell, S. M., et al. (1996). De novo antimicrobial peptides with low mammalian cell toxicity. J. Med. Chem. 39 (16), 3107–3113. doi:10.1021/jm9509410
Johnson, B. A., Anker, H., and Meleney, F. L. (1945). Bacitracin: a new antibiotic produced by a member of the B. subtilis group. Science 102 (2650), 376–377. doi:10.1126/science.102.2650.376
Johnstone, T. C., and Nolan, E. M. (2015). Beyond iron: non-classical biological functions of bacterial siderophores. Dalton Trans. (Cambridge, England : 2003) 44 (14), 6320–6339. doi:10.1039/c4dt03559c
Jongeneel, C. V., Bouvier, J., and Bairoch, A. (1989). A unique signature identifies a family of zinc-dependent metallopeptidases. FEBS Lett. 242 (2), 211–214. doi:10.1016/0014-5793(89)80471-5
Josts, I., Veith, K., and Tidow, H. (2019). Ternary structure of the outer membrane transporter FoxA with resolved signalling domain provides insights into TonB-mediated siderophore uptake. Elife 8, e48528. doi:10.7554/eLife.48528
Juliano, S. A., Pierce, S., deMayo, J. A., Balunas, M. J., and Angeles-Boza, A. M. (2017). Exploration of the innate immune system of Styela clava: Zn(2+) binding enhances the antimicrobial activity of the tunicate peptide Clavanin A. Biochemistry 56 (10), 1403–1414. doi:10.1021/acs.biochem.6b01046
Karr, J. W., Kaupp, L. J., and Szalai, V. A. (2004). Amyloid-beta binds Cu2+ in a mononuclear metal ion binding site. J. Am. Chem. Soc. 126 (41), 13534–13538. doi:10.1021/ja0488028
Kell, D. B., Heyden, E. L., and Pretorius, E. (2020). The biology of lactoferrin, an iron-binding protein that can help defend against viruses and bacteria. Front. Immunol. 11, 1221. doi:10.3389/fimmu.2020.01221
Khan, A., Singh, P., and Srivastava, A. (2018). Synthesis, nature and utility of universal iron chelator - siderophore: A review. Microbiol. Res. 212-213, 103–111. doi:10.1016/j.micres.2017.10.012
Kowalik-Jankowska, T., Ruta, M., Wiśniewska, K., and Lankiewicz, L. (2003). Coordination abilities of the 1-16 and 1-28 fragments of beta-amyloid peptide towards copper(II) ions: a combined potentiometric and spectroscopic study. J. Inorg. Biochem. 95 (4), 270–282. doi:10.1016/s0162-0134(03)00128-4
Kozłowski, H., Bal, W., Dyba, M., and Kowalik-Jankowska, T. (1999). Specific structure–stability relations in metallopeptides. Coord. Chem. Rev. 184 (1), 319–346. doi:10.1016/s0010-8545(98)00261-6
Kramer, J., Özkaya, Ö., and Kümmerli, R. (2020). Bacterial siderophores in community and host interactions. Nat. Rev. Microbiol. 18 (3), 152–163. doi:10.1038/s41579-019-0284-4
Le, C. F., Fang, C. M., and Sekaran, S. D. (2017). Intracellular targeting mechanisms by antimicrobial peptides. Antimicrob. Agents Chemother. 61 (4), e02340–16. doi:10.1128/AAC.02340-16
Lesiuk, M., Paduszyńska, M., and Greber, K. E. (2022). Synthetic antimicrobial immunomodulatory peptides: ongoing studies and clinical trials. Antibiot. (Basel, Switzerland) 11 (8), 1062. doi:10.3390/antibiotics11081062
Libardo, M. D., Cervantes, J. L., Salazar, J. C., and Angeles-Boza, A. M. (2014). Improved bioactivity of antimicrobial peptides by addition of amino-terminal copper and nickel (ATCUN) binding motifs. ChemMedChem 9 (8), 1892–1901. doi:10.1002/cmdc.201402033
Libardo, M. D., Gorbatyuk, V. Y., and Angeles-Boza, A. M. (2016). Central role of the copper-binding motif in the complex mechanism of action of ixosin: enhancing oxidative damage and promoting synergy with ixosin B. ACS Infect. Dis. 2 (1), 71–81. doi:10.1021/acsinfecdis.5b00140
Libardo, M. D., Paul, T. J., Prabhakar, R., and Angeles-Boza, A. M. (2015). Hybrid peptide ATCUN-sh-Buforin: influence of the ATCUN charge and stereochemistry on antimicrobial activity. Biochimie 113, 143–155. doi:10.1016/j.biochi.2015.04.008
Lihi, N., Lukács, M., Szűcs, D., Várnagy, K., and Sóvágó, I. (2017). Nickel(II), zinc(II) and cadmium(II) complexes of peptides containing separate aspartyl and cysteinyl residues. Polyhedron 133, 364–373. doi:10.1016/j.poly.2017.05.044
Łoboda, D., Kozłowski, H., and Rowińska-Żyrek, M. (2018). Antimicrobial peptide–metal ion interactions – a potential way of activity enhancement. New J. Chem. 42 (10), 7560–7568. doi:10.1039/c7nj04709f
Loréal, O., Cavey, T., Bardou-Jacquet, E., Guggenbuhl, P., Ropert, M., and Brissot, P. (2014). Iron, hepcidin, and the metal connection. Front. Pharmacol. 5, 128. doi:10.3389/fphar.2014.00128
Maisetta, G., Petruzzelli, R., Brancatisano, F. L., Esin, S., Vitali, A., Campa, M., et al. (2010). Antimicrobial activity of human hepcidin 20 and 25 against clinically relevant bacterial strains: effect of copper and acidic pH. Peptides 31 (11), 1995–2002. doi:10.1016/j.peptides.2010.08.007
Massip, C., and Oswald, E. (2020). Siderophore-microcins in Escherichia coli: determinants of digestive colonization, the first step toward virulence. Front. Cell Infect. Microbiol. 10, 381. doi:10.3389/fcimb.2020.00381
McCreary, E. K., Heil, E. L., and Tamma, P. D. (2021). New perspectives on antimicrobial agents: cefiderocol. Antimicrob. agents Chemother. 65 (8), e0217120. doi:10.1128/AAC.02171-20
Migliorini, C., Witkowska, D., Valensin, D., Kamysz, W., and Kozlowski, H. (2010). Competition between histamine-like and poly-imidazole coordination sites for Cu(2+) and Zn(2+) ions in zebra-fish peptide of prion-like protein. Dalton Trans. (Cambridge, England : 2003) 39 (37), 8663–8670. doi:10.1039/c0dt00137f
Miller, A., Matera-Witkiewicz, A., Mikołajczyk, A., Wieczorek, R., and Rowińska-Żyrek, M. (2021). Chemical “butterfly effect” explaining the coordination chemistry and antimicrobial properties of Clavanin complexes. Inorg. Chem. 60 (17), 12730–12734. doi:10.1021/acs.inorgchem.1c02101
Miller, M. C., Parkin, S., Fetherston, J. D., Perry, R. D., and DeMoll, E. (2006). Crystal structure of ferric-yersiniabactin, a virulence factor of Yersinia pestis. J. Inorg. Biochem. 100 (9), 1495–1500. doi:10.1016/j.jinorgbio.2006.04.007
Mizutani, K., Toyoda, M., and Mikami, B. (2012). X-ray structures of transferrins and related proteins. Biochim. Biophys. Acta 1820 (3), 203–211. doi:10.1016/j.bbagen.2011.08.003
Moynié, L., Milenkovic, S., Mislin, G. L. A., Gasser, V., Malloci, G., Baco, E., et al. (2019). The complex of ferric-enterobactin with its transporter from Pseudomonas aeruginosa suggests a two-site model. Nat. Commun. 10 (1), 3673. doi:10.1038/s41467-019-11508-y
Mulukutla, A., Shreshtha, R., Kumar Deb, V., Chatterjee, P., Jain, U., and Chauhan, N. (2024). Recent advances in antimicrobial peptide-based therapy. Bioorg Chem. 145, 107151. doi:10.1016/j.bioorg.2024.107151
Murdoch, C. C., and Skaar, E. P. (2022). Nutritional immunity: the battle for nutrient metals at the host-pathogen interface. Nat. Rev. Microbiol. 20 (11), 657–670. doi:10.1038/s41579-022-00745-6
Muskal, S. M., Holbrook, S. R., and Kim, S. H. (1990). Prediction of the disulfide-bonding state of cysteine in proteins. Protein Eng. 3 (8), 667–672. doi:10.1093/protein/3.8.667
Nakashige, T. G., Zygiel, E. M., Drennan, C. L., and Nolan, E. M. (2017). Nickel sequestration by the host-defense protein human calprotectin. J. Am. Chem. Soc. 139 (26), 8828–8836. doi:10.1021/jacs.7b01212
Oh, J., Kang, D., Hong, S., Kim, S. H., Choi, J. H., and Seo, J. (2021). Formation of a tris(catecholato) iron(III) complex with a nature-inspired cyclic peptoid ligand. Dalton Trans. 50 (10), 3459–3463. doi:10.1039/d1dt00091h
Olshvang, E., Fritsch, S., Scholtyssek, O. C., Schalk, I. J., and Metzler-Nolte, N. (2023). Vectorization via siderophores increases antibacterial activity of K(RW)(3) peptides against Pseudomonas aeruginosa. Chemistry 29 (50), e202300364. doi:10.1002/chem.202300364
Oludiran, A., Courson, D. S., Stuart, M. D., Radwan, A. R., Poutsma, J. C., Cotten, M. L., et al. (2019). How oxygen availability affects the antimicrobial efficacy of host defense peptides: lessons learned from studying the copper-binding peptides piscidins 1 and 3. Int. J. Mol. Sci. 20 (21), 5289. doi:10.3390/ijms20215289
Paredes, S. D., Kim, S., Rooney, M. T., Greenwood, A. I., Hristova, K., and Cotten, M. L. (2020). Enhancing the membrane activity of Piscidin 1 through peptide metallation and the presence of oxidized lipid species: implications for the unification of host defense mechanisms at lipid membranes. Biochim. Biophys. Acta Biomembr. 1862 (7), 183236. doi:10.1016/j.bbamem.2020.183236
Park, C. B., Yi, K. S., Matsuzaki, K., Kim, M. S., and Kim, S. C. (2000). Structure-activity analysis of buforin II, a histone H2A-derived antimicrobial peptide: the proline hinge is responsible for the cell-penetrating ability of buforin II. Proc. Natl. Acad. Sci. U S A. 97 (15), 8245–8250. doi:10.1073/pnas.150518097
Park, C. J., Park, C. B., Hong, S. S., Lee, H. S., Lee, S. Y., and Kim, S. C. (2000). Characterization and cDNA cloning of two glycine- and histidine-rich antimicrobial peptides from the roots of shepherd’s purse, Capsella bursa-pastoris. Plant Mol. Biol. 44 (2), 187–197. doi:10.1023/a:1006431320677
Pinkham, A. M., Yu, Z., and Cowan, J. A. (2018). Broad-spectrum catalytic metallopeptide inactivators of Zika and West Nile virus NS2B/NS3 proteases. Chem. Commun. (Camb) 54 (87), 12357–12360. doi:10.1039/c8cc07448h
Podkowa, K. J., Briere, L. A., Heinrichs, D. E., and Shilton, B. H. (2014). Crystal and solution structure analysis of FhuD2 from Staphylococcus aureus in multiple unliganded conformations and bound to ferrioxamine-B. Biochemistry 53 (12), 2017–2031. doi:10.1021/bi401349d
Portelinha, J., Duay, S. S., Yu, S. I., Heilemann, K., Libardo, M. D. J., Juliano, S. A., et al. (2021). Antimicrobial peptides and copper(II) ions: novel therapeutic opportunities. Chem. Rev. 121 (4), 2648–2712. doi:10.1021/acs.chemrev.0c00921
Raju, S. V., Sarkar, P., Kumar, P., and Arockiaraj, J. (2021). Piscidin, fish antimicrobial peptide: structure, classification, properties, mechanism, gene regulation and therapeutical importance. Int. J. Peptide Res. Ther. 27 (1), 91–107. doi:10.1007/s10989-020-10068-w
Rayner, B., Verderosa, A. D., Ferro, V., and Blaskovich, M. A. T. (2023). Siderophore conjugates to combat antibiotic-resistant bacteria. RSC Med. Chem. 14 (5), 800–822. doi:10.1039/d2md00465h
Ribeiro, M., and Simões, M. (2019). Advances in the antimicrobial and therapeutic potential of siderophores. Environ. Chem. Lett. 17 (4), 1485–1494. doi:10.1007/s10311-019-00887-9
Ronan, J. L., Kadi, N., McMahon, S. A., Naismith, J. H., Alkhalaf, L. M., and Challis, G. L. (2018). Desferrioxamine biosynthesis: diverse hydroxamate assembly by substrate-tolerant acyl transferase DesC. Philosophical Trans. R. Soc. B Biol. Sci. 373 (1748), 20170068. doi:10.1098/rstb.2017.0068
Roscetto, E., Bellavita, R., Paolillo, R., Merlino, F., Molfetta, N., Grieco, P., et al. (2021). Antimicrobial activity of a lipidated temporin L analogue against carbapenemase-producing Klebsiella pneumoniae clinical isolates. Antibiot. (Basel, Switzerland) 10 (11), 1312. doi:10.3390/antibiotics10111312
Rowinska-Zyrek, M., Witkowska, D., Potocki, S., Remelli, M., and Kozlowski, H. (2013). His-rich sequences – is plagiarism from nature a good idea? New J. Chem. 37 (1), 58–70. doi:10.1039/c2nj40558j
Ryu, J. H., Messersmith, P. B., and Lee, H. (2018). Polydopamine surface chemistry: a decade of discovery. ACS Appl. Mater Interfaces 10 (9), 7523–7540. doi:10.1021/acsami.7b19865
Sargsyan, K., Lin, C. C., Chen, T., Grauffel, C., Chen, Y. P., Yang, W. Z., et al. (2020). Multi-targeting of functional cysteines in multiple conserved SARS-CoV-2 domains by clinically safe Zn-ejectors. Chem. Sci. 11 (36), 9904–9909. doi:10.1039/d0sc02646h
Shetler, C. L., Ferreira, J. C., Cardoso, T. H. S., Silva, E. M. A., Saksena, N. K., and Rabeh, W. M. (2022). Therapeutic potential of metal ions for COVID-19: insights from the papain-like protease of SARS-CoV-2. Biochem. J. 479 (20), 2175–2193. doi:10.1042/BCJ20220380
Silva, O. N., Alves, E. S., de la Fuente-Núñez, C., Ribeiro, S. M., Mandal, S. M., Gaspar, D., et al. (2016). Structural studies of a lipid-binding peptide from tunicate hemocytes with anti-biofilm activity. Sci. Rep. 6, 27128. doi:10.1038/srep27128
Singh, P., and Ali, S. A. (2022). Multifunctional role of S100 protein family in the immune system: an update. Cells 11 (15), 2274. doi:10.3390/cells11152274
Skerlavaj, B., and Boix-Lemonche, G. (2023). The potential of surface-immobilized antimicrobial peptides for the enhancement of orthopaedic medical devices: a review. Antibiot. (Basel, Switzerland) 12 (2), 211. doi:10.3390/antibiotics12020211
Sóvágó, I., Kállay, C., and Várnagy, K. (2012). Peptides as complexing agents: factors influencing the structure and thermodynamic stability of peptide complexes. Coord. Chem. Rev. 256 (19), 2225–2233. doi:10.1016/j.ccr.2012.02.026
Sóvágó, I., Várnagy, K., Lihi, N., and Grenács, Á. (2016). Coordinating properties of peptides containing histidyl residues. Coord. Chem. Rev. 327-328, 43–54. doi:10.1016/j.ccr.2016.04.015
Stepanova, M., Levit, M., Egorova, T., Nashchekina, Y., Sall, T., Demyanova, E., et al. (2024). Poly(2-Deoxy-2-Methacrylamido-D-Glucose)-Based complex conjugates of colistin, deferoxamine and vitamin B12: synthesis and biological evaluation. Pharmaceutics 16 (8), 1080. doi:10.3390/pharmaceutics16081080
Stone, K. J., and Strominger, J. L. (1971). Mechanism of action of bacitracin: complexation with metal ion and C 55 -isoprenyl pyrophosphate. Proc. Natl. Acad. Sci. U S A. 68 (12), 3223–3227. doi:10.1073/pnas.68.12.3223
Storm, D. R., and Strominger, J. L. (1973). Complex Formation between bacitracin peptides and isoprenyl pyrophosphates. J. Biol. Chem. 248 (11), 3940–3945. doi:10.1016/s0021-9258(19)43823-4
Sun, X. L., Baker, H. M., Shewry, S. C., Jameson, G. B., and Baker, E. N. (1999). Structure of recombinant human lactoferrin expressed in Aspergillus awamori. Acta Crystallogr. D. Biol. Crystallogr. 55 (Pt 2), 403–407. doi:10.1107/s0907444998011226
Swayambhu, G., Bruno, M., Gulick, A. M., and Pfeifer, B. A. (2021). Siderophore natural products as pharmaceutical agents. Curr. Opin. Biotechnol. 69, 242–251. doi:10.1016/j.copbio.2021.01.021
Syme, C. D., Nadal, R. C., Rigby, S. E., and Viles, J. H. (2004). Copper binding to the amyloid-beta (Abeta) peptide associated with Alzheimer's disease: folding, coordination geometry, pH dependence, stoichiometry, and affinity of Abeta-(1-28): insights from a range of complementary spectroscopic techniques. J. Biol. Chem. 279 (18), 18169–18177. doi:10.1074/jbc.M313572200
Szarszoń, K., Mikołajczyk, A., Grelich-Mucha, M., Wieczorek, R., Matera-Witkiewicz, A., Olesiak-Bańska, J., et al. (2024). Bioinorganic chemistry of shepherin II complexes helps to fight Candida albicans? J. Inorg. Biochem. 253, 112476. doi:10.1016/j.jinorgbio.2023.112476
Teixeira, M. C., Carbone, C., Sousa, M. C., Espina, M., Garcia, M. L., Sanchez-Lopez, E., et al. (2020). Nanomedicines for the delivery of antimicrobial peptides (AMPs). Nanomater. (Basel) 10 (3), 560. doi:10.3390/nano10030560
Timofeeva, A. M., Galyamova, M. R., and Sedykh, S. E. (2022). Bacterial siderophores: classification, biosynthesis, perspectives of use in agriculture. Plants (Basel) 11 (22), 3065. doi:10.3390/plants11223065
Toscano, W. A., and Storm, D. R. (1982). Bacitracin. Pharmacol. Ther. 16 (2), 199–210. doi:10.1016/0163-7258(82)90054-7
Trzcińska, Z., Bruggeman, M., Ijakipour, H., Hodges, N. J., Bowen, J., and Stamboulis, A. (2020). Polydopamine linking substrate for AMPs: characterisation and stability on Ti6Al4V. Materials 13, 3714. doi:10.3390/ma13173714
Tselepis, C., Ford, S. J., McKie, A. T., Vogel, W., Zoller, H., Simpson, R. J., et al. (2010). Characterization of the transition-metal-binding properties of hepcidin. Biochem. J. 427 (2), 289–296. doi:10.1042/BJ20091521
Turski, M. L., and Thiele, D. J. (2009). New roles for copper metabolism in cell proliferation, signaling, and disease. J. Biol. Chem. 284 (2), 717–721. doi:10.1074/jbc.R800055200
Ustiatik, R., Nuraini, Y., Suharjono, S., and Handayanto, E. (2021). Siderophore production of the Hg-resistant endophytic bacteria isolated from local grass in the Hg-contaminated soil. J. Ecol. Eng. 22, 129–138. doi:10.12911/22998993/135861
Wątły, J., Potocki, S., and Rowińska-Żyrek, M. (2016). Zinc homeostasis at the bacteria/host interface-from coordination chemistry to nutritional immunity. Chem. (Weinheim an der Bergstrasse, Germany) 22 (45), 15992–16010. doi:10.1002/chem.201602376
Wątły, J., Szarszoń, K., Mikołajczyk, A., Grelich-Mucha, M., Matera-Witkiewicz, A., Olesiak-Bańska, J., et al. (2023). Zn(II) induces fibril formation and antifungal activity in shepherin I, an antimicrobial peptide from capsella bursa-pastoris. Inorg. Chem. 62 (48), 19786–19794. doi:10.1021/acs.inorgchem.3c03409
Wheeler, L. C., Donor, M. T., Prell, J. S., and Harms, M. J. (2016). Multiple evolutionary origins of ubiquitous Cu2+ and Zn2+ binding in the S100 protein family. PLoS One 11 (10), e0164740. doi:10.1371/journal.pone.0164740
Wilson, B. R., Bogdan, A. R., Miyazawa, M., Hashimoto, K., and Tsuji, Y. (2016). Siderophores in iron metabolism: from mechanism to therapy potential. Trends Mol. Med. 22 (12), 1077–1090. doi:10.1016/j.molmed.2016.10.005
Xiang, S., Han, N., Xie, Y., Du, J., Luo, Z., Xu, J., et al. (2024). Antimicrobial peptides in treatment of Stage III Grade B periodontitis: a randomized clinical trial. Oral Dis. 30 (5), 3376–3385. doi:10.1111/odi.14786
Yazici, H., O'Neill, M. B., Kacar, T., Wilson, B. R., Oren, E. E., Sarikaya, M., et al. (2016). Engineered chimeric peptides as antimicrobial surface coating agents toward infection-free implants. ACS Appl. Mater Interfaces 8 (8), 5070–5081. doi:10.1021/acsami.5b03697
Żamojć, K., Wyrzykowski, D., Sabatino, G., Papini, A. M., Wieczorek, R., Chmurzyński, L., et al. (2021). Key role of histidine residues orientation in affinity binding of model pentapeptides with Ni2+ ions: a theoretical supported experimental study. J. Mol. Liq. 341, 117414. doi:10.1016/j.molliq.2021.117414
Keywords: transition metal, antimicrobial peptides, siderophores, coordination, structural modifications
Citation: Falcigno L, Braccia S, Bellavita R, D’Auria G, Falanga A and Galdiero S (2024) Metal-antimicrobial peptides combo: promising weapons to combat bacteria invaders. Front. Drug Discov. 4:1440378. doi: 10.3389/fddsv.2024.1440378
Received: 29 May 2024; Accepted: 02 October 2024;
Published: 16 October 2024.
Edited by:
Tânia Martins Silva, Universidade do Porto, PortugalReviewed by:
Cesar Augusto Roque-Borda, São Paulo State University, BrazilSlawomir Potocki, University of Wrocław, Poland
Copyright © 2024 Falcigno, Braccia, Bellavita, D’Auria, Falanga and Galdiero. This is an open-access article distributed under the terms of the Creative Commons Attribution License (CC BY). The use, distribution or reproduction in other forums is permitted, provided the original author(s) and the copyright owner(s) are credited and that the original publication in this journal is cited, in accordance with accepted academic practice. No use, distribution or reproduction is permitted which does not comply with these terms.
*Correspondence: Stefania Galdiero, c3RlZmFuaWEuZ2FsZGllcm9AdW5pbmEuaXQ=