- Department of Microbiology, School of Life Sciences, Pondicherry University, Puducherry, India
The prevalence of clinical resistance of P. falciparum towards artemisinin and its partner drugs has significantly hampered malarial chemotherapy. To circumvent this situation, identifying a new class of partner drugs with significant anti-malarial efficacy and multi-stage activity can slow the development of resistance. This study demonstrates the potential interactions of carboxylic ionophores such as monensin (MON), maduramicin (MAD) or salinomycin (SAL) with standard antimalarial drugs artemisinin (ART) or chloroquine (CQ). The in vitro drug interactions were studied in P. falciparum 3D7 strain by a growth inhibition SYBR green 1 assay. The asynchronized parasites were exposed for 48 h in the presence of varying proportions of two drug concentrations using the modified fixed-ratio isobologram method. We determined the growth inhibition response and the sums of the fractional inhibitory concentrations (ΣFICs) of the following drug combinations (4:1, 3:2, 2:3, 1:4) and (1:1, 1:3, 3:1) were calculated for 50% inhibitory concentrations (IC50s). Combining artemisinin with monensin, maduramicin, or salinomycin showed significant additive interaction. A combination of chloroquine with monensin, maduramicin, or salinomycin showed slight synergism to additive interaction. None of the drug combinations displayed an antagonistic effect indicating ionophores usage in combination therapy to treat drug-resistant malarial infections.
Introduction
Antimalarial chemotherapy has become more complex due to the emergence and spread of multidrug-resistant P. falciparum parasites. An estimated 247 million malaria cases were reported globally in 2021 (World Health Organization, 2022). Over the years of the COVID-19 pandemic, malaria service disruption led to the most significant annual increase of 13 million more malaria cases and 63,000 deaths (Weiss et al., 2021). P. falciparum fails to respond to monotherapy such as chloroquine, artemisinin, and other clinically important antimalarial drugs (Wellems and Plowe, 2001; Dondorp et al., 2009; Ashley et al., 2014). To circumvent this problem, artemisinin-based combination therapy (ACT) was advocated as the first line of treatment for P. falciparum infection in all malaria-endemic regions (Eastman and Fidock, 2009). Surprisingly, P. falciparum started showing decreased susceptibility towards artemisinin and its derivatives, along with its partner drugs (artemether-lumefantrine, artesunate-amodiaquine and artesunate-sulfadoxine-pyrimethamine) (World Health Organization, 2015; Nsanzabana, 2019; Ward et al., 2022). The subsequent treatment failure of ACT furthermore challenged the global effort to bring down the malaria burden. As a timely solution to counter antimalarial drug resistance, triple artemisinin-based combination therapy (TACT) has been implemented (van der Pluijm et al., 2021; Chen and Hsiang, 2022). Still, a robust search on identifying partner drugs for ACT and new drug combinations remains a high priority. Besides, currently available malarial vaccines such as RTSS, pfSPZ, ME-TARP and GMZ2 targeting different life stages of the parasites exhibit varied levels of protection having moderate efficacy. The P. falciparum surface antigens, which are highly polymorphic, present a significant challenge for vaccine design (Crompton et al., 2010; Stanisic and McCall, 2021). These situations demand novel or repurposed effective and safe partner drugs with unrelated modes of action in combination with chemotherapeutics, considered a potential alternative strategy (Aarestrup et al., 1998).
Ionophores, an FDA-approved veterinary antibiotic, display antiplasmodial activity by intercalating with parasite membrane and exchanging ions, leading to increased cytosolic ion concentration and alteration in pH, causing parasite death (Adovelande and Schrével, 1996; Aowicki and Huczynski, 2013). Interestingly, ionophores have been reported to exhibit multi-stage activity by targeting the blood stage, liver stage, gametocytes and sporozoites at sub-nanomolar levels both in vitro and in vivo conditions (Mahmoudi et al., 2008; D'Alessandro et al., 2015). Therefore, employing multi-stage antimalarial agents like ionophore combined with CQ or ART could tackle emerging drug-resistant parasites. The present findings demonstrate the seven different fixed-ratio drug combinations of selective ionophores (monensin, maduramicin, and salinomycin) with CQ or ART on the growth of Pf3D7 in cultures possessing either synergistic, additive or antagonistic effects.
Materials and methods
Materials
Powdered RPMI 1640 medium, AlbuMAX ІІ are Gibco products from Invitrogen Corporation. Monensin sodium salt, maduramycin, salinomycin, chloroquine, and artemisinin, gentamycin sulfate (cell-culture grade), Histopaque-1077 were purchased from Sigma-Aldrich. All other chemicals used were analytical-grade products.
In vitro culture of P. falciparum
The laboratory-adapted P. falciparum 3D7 strain was maintained in continuous culturing in human erythrocytes with 4%–5% hematocrit in RPMI 1640 medium supplemented with 5 g/L AlbuMAX (lipid-rich bovine albumin), 2 g/L glucose, 50 mg/L hypoxanthine, 2 g/L sodium bicarbonate, 10 mg/L gentamycin sulphate and incubated at 37°C in the low-oxygen environment by Candle jar method (Jensen and Trager, 1977). The daily changes of culture medium at 5% hematocrit and diluted with uninfected RBC when parasitemia exceeded 5%. The 100% packed RBC was obtained in sterile condition by removal of plasma and peripheral blood mononuclear cells (PBMCs) using Histopaque gradient, the RBCs were washed 2 to 3 times using RPMI 1640 medium without AlbuMAX (incomplete medium). Parasite stages were determined by Giemsa-stained blood smears. Parasitemia was routinely quantified by microscopic observation of blood smear using a cell counter.
In vitro drug inhibition assay
A stock solution of monensin, maduramicin, salinomycin and artemisinin was prepared in DMSO, whereas chloroquine was prepared in sterile distilled water and then diluted with RPMI 1640 medium to achieve the required concentration (final DMSO concentration of <1%, which is non-toxic to parasites). Drugs were two-fold serially diluted with a working concentration of 100 ng/mL in 96 well plates. Chloroquine (500 μg/mL) was used as a positive control. The different fixed-ratio drug combinations were exposed to asynchronous cultures with 1% parasitemia and 2% hematocrit and incubated for 48 h at 37°C in a candle jar. After 48 h of incubation, the parasite growth was determined by the SYBR Green-І assay (Smilkstein et al., 2004; Johnson et al., 2007). Freshly prepared 0.2 μL/mL of × 10,000 SYBR Green-І suspended in lysis buffer (20 mM Tris-HCl, 5 mM EDTA, 0.008% saponin and 0.08% Triton X) was subsequently added and incubated in the dark for 1 h at room temperature. The fluorescence emitted by DNA-bound dye was quantified using the multi-mode plate reader (Synergy Bioteck) with excitation and emission wavelength of 485 nm and 528 nm, respectively. The fluorescence readouts were plotted against drug concentrations and data processing of IC50 values, statistical analysis was performed using Microsoft Excel software.
Drug combination
A modified fixed-ratio isobologram method was used to assess drug interactions (Fivelman et al., 2004). For combination assays, the dosage of each drug dilution was prepared. The top concentration of each drug was 8 times higher than the respective IC50 values of individual drugs to fall at around the mid-point in a two-fold serial dilution. The individual and sum 50% fractional inhibitory concentration (FIC) of two drugs in fixed combination ratios (4:1, 3:2, 2:3, and 1:4) and (1:1, 1:3, and 3:1) was derived using the formula.
To obtain numeric values for the drug interactions, the results were expressed as the sum of two FICs (∑FIC) values as follows. ∑FIC<1 represents synergism, ∑FIC> =1 and <2 represent additive interactions, ∑FIC> =2 and <4 represent slight antagonism, while ∑FIC> =4 represents marked antagonism. The drug interaction analysis was employed based on the earlier reports (Agarwal et al., 2015).
Results
Determination of dose-response curves of individual drugs
Before embarking on the drug-interaction studies, the susceptibility profile of individual ionophores (monensin, maduramicin and salinomycin) and standard antimalarials (chloroquine and artemisinin) was performed using asynchronous P. falciparum 3D7 strain. The 50% inhibitory concentration (IC50) was determined and all three ionophores displayed pronounced antiplasmodial activity at sub-nanomolar concentration. Monensin displayed IC50 values 1.98 ng/mL, maduramicin displayed IC50 values 7.71 ng/mL and salinomycin indicated IC50 values 170 ng/mL. The reported IC50 values are in agreement with the previously reported values as shown in Table 1 (D'Alessandro et al., 2015; Rajendran et al., 2015; Raza et al., 2018).
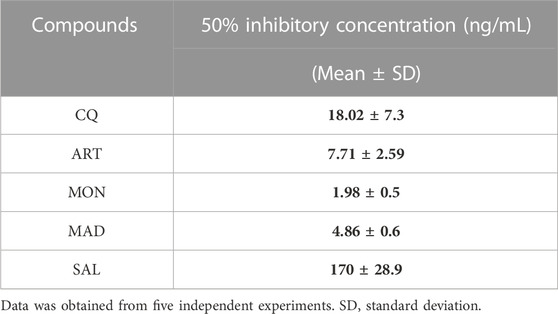
TABLE 1. In vitro antimalarial activity of standard antimalarials and ionophores against Plasmodium falciparum 3D7 strain.
Determination of drug interaction by modified fixed-ratio method
We then sought to investigate whether ionophores could potentiate the antiplasmodial action of CQ or ART. To this end, a fixed ratio (4:1, 3:2, 2:3, 1:4) drug combination assay was performed between three ionophores and standard antimalarials. To widen our understanding, we employed three other different drug ratios (1:1, 1:3, 3:1) to further investigate whether these interactions are additive or synergistic, as shown in Table 2.
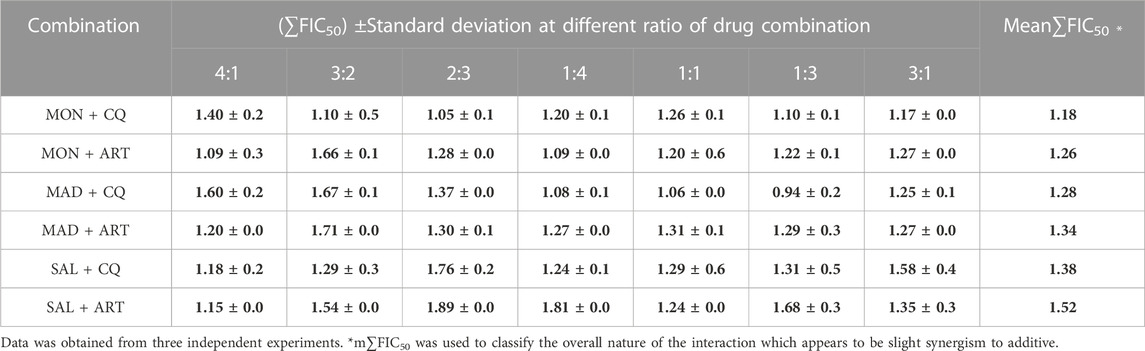
TABLE 2. In vitro interaction of ionophores with standard antimalarials against Plasmodium falciparum 3D7 strain blood-stage infection.
Among the ionophore interactions with CQ, MAD + CQ showed a significant interaction by exhibiting ∑FIC values ranging from 0.94 to 1.67, indicating a slight synergism to additive interactions. Followed by MON + CQ having a strong additive interaction (∑FIC ranging from 1.05 to 1.40) followed by SAL + CQ with weak additive interactions (∑FIC ranging from 1.18 to 1.76). All three combinations of ionophores with CQ failed to show any antagonistic effect.
Conversely, ionophore combined with ART, MON + ART showed strong additive interactions with ∑FIC values ranging from 1.09 to 1.66. Secondly, MAD + ART showed additive interaction with ∑FIC values ranging from 1.20 to 1.71 followed by SAL + ART with ∑FIC values ranging from 1.15 to 1.89. Therefore, ionophore combination with ART did not show any antagonistic effect. These results reveal that ionophore combination with CQ or ART could be considered a new combinatorial regimen.
Discussion
The present study demonstrates the carboxylic ionophores monensin, maduramicin, and salinomycin showed antimalarial activity at sub-nanomolar concentration. These ionophores displayed positive interactions (slight synergism to additive) with standard antimalarial drugs (CQ or ART) against the P. falciparum 3D7 line. Interestingly, ionophores (monensin and nigericin) exhibit antiplasmodial activity at the sub-nanomolar range and display 25 to 30,000-fold potential than CQ against Pf strains and a significant reduction in blood-parasite load in a murine model of malaria (Adovelande and Schrével, 1996). Likewise, ionophores display approximately >25-fold potential activity than ART against Pf3D7 (Rajendran et al., 2015). This fact led us to consider ionophores as a suitable partner drug along with clinical antimalarials (CQ or ART). Ionophores are reported to kill blood-stage (D'Alessandro et al., 2015), liver-stage (Mahmoudi et al., 2008), and sexual-stage parasites at sub-nanomolar concentrations. Surprisingly, mosquitoes ookinete and oocyst development are significantly hampered by ionophores acting as transmission-blocking agents (D'Alessandro et al., 2015). As a result, ionophores can simultaneously target asexual, sexual and liver-stage forms of parasites and possess multi-stage antimalarial potential. The ionophores cause minimal damage to the uninfected host erythrocytes >10 µM for monensin (Bhavsar et al., 2010), the release of hemoglobin in the supernatant after 7 days of incubation with monensin, salinomycin, or nigericin at 100 nM dose showed less than 3-fold increase (D'Alessandro et al., 2015), suggesting its non-toxic effect at therapeutic dose. Hepatocytes are primarily the host cells for sporozoite invasion. Monensin exposure at 1 µM showed no morphological defects or loss of viability (Leitao and Rodriguez, 2010) which further substantiates no toxic effects on the liver-host cells. This led us to evaluate the combinatorial impact of multi-stage antiplasmodial agents (ionophores) with CQ and ART. Therefore, the present study aimed to assess the combined effect of multistage potential ionophores with the blood-stage potential clinical antimalarial drugs.
Our results indicate that ionophores combined with CQ or ART at seven different drug ratios showed slight synergism to additive or indifferent interaction. Firstly, combining monensin, maduramicin or salinomycin with CQ showed varied drug interaction from slight synergism to strong to weak additivity. The combined detrimental effect is due to monensin mediated influx of Na+ ions causing alkalization of acidic food vacuole hampering hemoglobin degradation (Adovelande and Schrével, 1996). The influx of Na+ ions by maduramicin leads to alteration in parasite cytosolic pH resulting in cholesterol accumulation in the parasite plasma membrane and retards trophozoite to schizont development (Das et al., 2016). Salinomycin contributes towards the efflux of K+ ions from parasite cytosol and the subsequent influx of Na+ ions and water molecules in parasite cytosol, resulting in parasite cell swelling (Steverding and Sexton, 2013). As a result, ionophores tend to accumulate Na+ ions inside the parasite compartments by disturbing the ionic equilibrium and arresting parasite growth.
Clinically used antimalarial CQ mainly target heme polymerization (Coronado et al., 2014). Chloroquine entry into parasite acidic food vacuole leads to protonation of CQ2+ and subsequently interferes with heme polymerisation and fails to detoxify, leading to parasite death (Sullivan et al., 1998; Thomé et al., 2013). Mainly, artemisinin gets fully activated in the presence of parasite-derived heme (in the early ring stage) and hemoglobin-derived heme (in the trophozoite stage) which results in reactive oxygen species (ROS) generation and depolarisation of parasite mitochondria induces cell death via apoptosis (Wang et al., 2010; Mercer et al., 2011).
Therefore, these drugs display additive interactions by acting on different molecular targets of the parasite target organelles. Considering the fact, lipid-soluble fast-acting ionophores combined with water-soluble, fast-acting CQ showing differential pharmacokinetics may facilitate reducing clinical resistance and long-term efficacy. Similarly, both fast-acting and lipid-soluble antimalarials (ionophores and ART) having varied pharmacokinetics profiles, salinomycin (Qi et al., 2022), monensin (Atef et al., 1993), maduramicin (Raza et al., 2018) artemisinin and derivatives (Medhi et al., 2009; Birgersson et al., 2016) may significantly curb the parasite development at all stages with greater effectiveness. Intriguingly, ionophores display equipotency on CQ-susceptible and resistant strains of P. falciparum. Moreover, ionophores facilitate overcoming drug resistance by inhibiting drug-transporter proteins (Antoszczak et al., 2019). Furthermore, ionophores demonstrated slow-development of resistance in ruminal bacteria due to phenotypic selection rather than transmissible genetic factors (Russell and Houlihan, 2003). Intravenous administration of salinomycin (200–250 μg/kg) in metastatic cancer patients manifested only acute side effects without severe or long-term side effects indicating clinical safety (Naujokat and Steinhart, 2012).
Therefore, it seems reasonable to suggest that ionophores in combination with CQ or ART are worth serious consideration for clinical trials and employed in multidrug-resistant areas where malaria transmission is endemic.
Conclusion
In conclusion, our data suggest ionophores with clinical antimalarials CQ and ART displayed moderate synergism to additive interaction against P. falciparum 3D7 blood-stage infection. None of the drug combinations showed antagonistic interaction suggesting the utilization of such combinations in clinical settings. Under current recommendations, regarding combination therapies, ionophores could be partnered with standard antimalarials to combat severe complicated malaria in endemic regions.
Data availability statement
The original contributions presented in the study are included in the article/supplementary material, further inquiries can be directed to the corresponding author.
Author contributions
VR: Writing–original draft, Conceptualization, Funding acquisition, Methodology, Supervision. KG: Writing–original draft.
Funding
The author(s) declare financial support was received for the research, authorship, and/or publication of this article. The VR lab is supported by the research grant from Department of Science and Technology (DST), INSPIRE-Faculty Project (DST/INSPIRE/04/2018/003541), Ministry of Science and Technology, Government of India.
Acknowledgments
We thank Pondicherry Institute of Medical Sciences (PIMS) Blood Bank, Puducherry, India, for a continuous supply of blood for parasite culture.
Conflict of interest
The authors declare that the research was conducted in the absence of any commercial or financial relationships that could be construed as a potential conflict of interest.
Publisher’s note
All claims expressed in this article are solely those of the authors and do not necessarily represent those of their affiliated organizations, or those of the publisher, the editors and the reviewers. Any product that may be evaluated in this article, or claim that may be made by its manufacturer, is not guaranteed or endorsed by the publisher.
References
Aarestrup, F. M., Bager, F., Jensen, N. E., Madsen, M., Meyling, A., and Wegener, H. C. J. A. (1998). Surveillance of antimicrobial resistance in bacteria isolated from food animals to antimicrobial growth promoters and related therapeutic agents in Denmark. APMIS 106, 606–622. doi:10.1111/j.1699-0463.1998.tb01391.x
Adovelande, J., and Schrével, J. J. L. S. (1996). Carboxylic ionophores in malaria chemotherapy: the effects of monensin and nigericin on Plasmodium falciparum in vitro and Plasmodium vinckei petteri in vivo. Life Sci. 59, PL309–PL315. doi:10.1016/s0024-3205(96)00514-0
Agarwal, D., Sharma, M., Dixit, S. K., Dutta, R. K., Singh, A. K., Gupta, R. D., et al. (2015). In vitro synergistic effect of fluoroquinolone analogues in combination with artemisinin against Plasmodium falciparum; their antiplasmodial action in rodent malaria model. Malar. J. 14, 48–49. doi:10.1186/s12936-015-0561-2
Antoszczak, M., Steverding, D., and Huczynski, A. (2019). Anti-parasitic activity of polyether ionophores. Eur. J. Med. Chem. 166, 32–47. doi:10.1016/j.ejmech.2019.01.035
Aowicki, D., and Huczynski, A. (2013). Structure and antimicrobial properties of monensin A and its derivatives: summary of the achievements. Biomed. Res. Int. 2013, 742149. doi:10.1155/2013/742149
Ashley, E. A., Dhorda, M., Fairhurst, R. M., Amaratunga, C., Lim, P., Suon, S., et al. (2014). Spread of artemisinin resistance in Plasmodium falciparum malaria. N. Engl. J. Med. 371, 411–423. doi:10.1056/NEJMoa1314981
Atef, M., Ramadan, A., and Abo El-Sooud, K. (1993). Pharmacokinetic profile and tissue distribution of monensin in broiler chickens. Br. Poult. Sci. 34, 195–203. doi:10.1080/00071669308417575
Bhavsar, S. K., Eberhard, M., Bobbala, D., and Lang, F. J. C. P. (2010). Monensin induced suicidal erythrocyte death. Monensin Induc. suicidal erythrocyte death 25, 745–752. doi:10.1159/000315094
Birgersson, S., Van Toi, P., Truong, N. T., Dung, N. T., Ashton, M., Hien, T. T., et al. (2016). Population pharmacokinetic properties of artemisinin in healthy male Vietnamese volunteers. Malar. J. 15, 90. doi:10.1186/s12936-016-1134-8
Chen, I., and Hsiang, M. S. J. T. L. I. D. (2022). Triple artemisinin-based combination therapies for malaria: a timely solution to counter antimalarial drug resistance. Lancet. Infect. Dis. 22, 751–753. doi:10.1016/S1473-3099(21)00748-9
Coronado, L. M., Nadovich, C. T., and Spadafora, C. J. B. E. B. (2014). Malarial hemozoin: from target to tool. Biochim. Biophys. Acta 1840, 2032–2041. doi:10.1016/j.bbagen.2014.02.009
Crompton, P. D., Pierce, S. K., and Miller, L. H. J. T. J. O. C. I. (2010). Advances and challenges in malaria vaccine development. J. Clin. Invest. 120, 4168–4178. doi:10.1172/JCI44423
D'alessandro, S., Corbett, Y., Ilboudo, D. P., Misiano, P., Dahiya, N., Abay, S. M., et al. (2015). Salinomycin and other ionophores as a new class of antimalarial drugs with transmission-blocking activity. Antimicrob. Agents Chemother. 59, 5135–5144. doi:10.1128/AAC.04332-14
Das, S., Bhatanagar, S., Morrisey, J. M., Daly, T. M., Burns, J. M., Coppens, I., et al. (2016). Na+ influx induced by new antimalarials causes rapid alterations in the cholesterol content and morphology of Plasmodium falciparum. PLoS Pathog. 12, e1005647. doi:10.1371/journal.ppat.1005647
Dondorp, A. M., Nosten, F., Yi, P., Das, D., Phyo, A. P., Tarning, J., et al. (2009). Artemisinin resistance in Plasmodium falciparum malaria. N. Engl. J. Med. 361, 455–467. doi:10.1056/NEJMoa0808859
Eastman, R. T., and Fidock, D. A. J. N. R. M. (2009). Artemisinin-based combination therapies: a vital tool in efforts to eliminate malaria. Nat. Rev. Microbiol. 7, 864–874. doi:10.1038/nrmicro2239
Fivelman, Q. L., Adagu, I. S., and Warhurst, D. C. (2004). Modified fixed-ratio isobologram method for studying in vitro interactions between atovaquone and proguanil or dihydroartemisinin against drug-resistant strains of Plasmodium falciparum. Antimicrob. Agents Chemother. 48, 4097–4102. doi:10.1128/AAC.48.11.4097-4102.2004
Jensen, J. B., and Trager, W. J. T. J. O. P. (1977). Plasmodium falciparum in culture: use of outdated erythrocytes and description of the candle jar method. J. Parasitol. 63, 883–886. doi:10.2307/3279900
Johnson, J. D., Dennull, R. A., Gerena, L., Lopez-Sanchez, M., Roncal, N. E., and Waters, N. C. (2007). Assessment and continued validation of the malaria SYBR green I-based fluorescence assay for use in malaria drug screening. Drug Screen. 51, 1926–1933. doi:10.1128/AAC.01607-06
Leitao, R., and Rodriguez, A. J. E. P. (2010). Inhibition of Plasmodium sporozoites infection by targeting the host cell. Exp. Parasitol. 126, 273–277. doi:10.1016/j.exppara.2010.05.012
Mahmoudi, N., Garcia-Domenech, R., Galvez, J., Farhati, K., Franetich, J. F., Sauerwein, R., et al. (2008). New active drugs against liver stages of Plasmodium predicted by molecular topology. Antimicrob. Agents Chemother. 52, 1215–1220. doi:10.1128/AAC.01043-07
Medhi, B., Patyar, S., Rao, R. S., Byrav, D. S. P., and Prakash, A. (2009). Pharmacokinetic and toxicological profile of artemisinin compounds: an update. Pharmacology 84, 323–332. doi:10.1159/000252658
Mercer, A. E., Copple, I. M., Maggs, J. L., O'neill, P. M., and Park, B. K. J. J. O. B. C. (2011). The role of heme and the mitochondrion in the chemical and molecular mechanisms of mammalian cell death induced by the artemisinin antimalarials. J. Biol. Chem. 286, 987–996. doi:10.1074/jbc.M110.144188
Naujokat, C., and Steinhart, R. (2012). Salinomycin as a drug for targeting human cancer stem cells. J. Biomed. Biotechnol. 2012, 950658. doi:10.1155/2012/950658
Nsanzabana, C. (2019). Resistance to artemisinin combination therapies (ACTs): do not forget the partner drug. Trop. Med. Infect. Dis. 4, 26. doi:10.3390/tropicalmed4010026
Qi, D., Liu, Y., Li, J., Huang, J. H., Hu, X., and Wu, E. (2022). Salinomycin as a potent anticancer stem cell agent: state of the art and future directions. Med. Res. Rev. 42, 1037–1063. doi:10.1002/med.21870
Rajendran, V., Rohra, S., Raza, M., Hasan, G. M., Dutt, S., and Ghosh, P. C. (2015). Stearylamine liposomal delivery of monensin in combination with free artemisinin eliminates blood stages of Plasmodium falciparum in culture and P. Berghei infection in murine malaria. Antimicrob. Agents Chemother. 60, 1304–1318. doi:10.1128/AAC.01796-15
Raza, M., Bharti, H., Singal, A., Nag, A., and Ghosh, P. C. (2018). Long circulatory liposomal maduramicin inhibits the growth of Plasmodium falciparum blood stages in culture and cures murine models of experimental malaria. Nanoscale 10, 13773–13791. doi:10.1039/c8nr02442a
Russell, J. B., and Houlihan, A. J. (2003). Ionophore resistance of ruminal bacteria and its potential impact on human health. FEMS Microbiol. Rev. 27, 65–74. doi:10.1016/S0168-6445(03)00019-6
Smilkstein, M., Sriwilaijaroen, N., Kelly, J. X., Wilairat, P., and Riscoe, M. J. A. (2004). Simple and inexpensive fluorescence-based technique for high-throughput antimalarial drug screening. Antimicrob. Agents Chemother. 48, 1803–1806. doi:10.1128/AAC.48.5.1803-1806.2004
Stanisic, D. I., and Mccall, M. B. J. E. R. O. V. (2021). Correlates of malaria vaccine efficacy. Expert Rev. Vaccines 20, 143–161. doi:10.1080/14760584.2021.1882309
Steverding, D., and Sexton, D. W. J. P. (2013). Trypanocidal activity of salinomycin is due to sodium influx followed by cell swelling. Parasit. Vectors 6, 1–5. doi:10.1186/1756-3305-6-78
Sullivan, D. J., Matile, H., Ridley, R. G., and Goldberg, D. E. J. J. O. B. C. (1998). A common mechanism for blockade of heme polymerization by antimalarial quinolines. J. Biol. Chem. 273, 31103–31107. doi:10.1074/jbc.273.47.31103
Thomé, R., Lopes, S. C. P., Costa, F. T. M., and Verinaud, L. J. I. L. (2013). Chloroquine: modes of action of an undervalued drug. Immunol. Lett. 153, 50–57. doi:10.1016/j.imlet.2013.07.004
Van Der Pluijm, R. W., Amaratunga, C., Dhorda, M., and Dondorp, A. M. J. T. I. P. (2021). Triple artemisinin-based combination therapies for malaria–a new paradigm? Trends Parasitol. 37, 15–24. doi:10.1016/j.pt.2020.09.011
Wang, J., Huang, L., Li, J., Fan, Q., Long, Y., Li, Y., et al. (2010). Artemisinin directly targets malarial mitochondria through its specific mitochondrial activation. PLoS One 5, e9582. doi:10.1371/journal.pone.0009582
Ward, K. E., Fidock, D. A., and Bridgford, J. L. (2022). Plasmodium falciparum resistance to artemisinin-based combination therapies. Curr. Opin. Microbiol. 69, 102193. doi:10.1016/j.mib.2022.102193
Weiss, D. J., Bertozzi-Villa, A., Rumisha, S. F., Amratia, P., Arambepola, R., Battle, K. E., et al. (2021). Indirect effects of the COVID-19 pandemic on malaria intervention coverage, morbidity, and mortality in Africa: a geospatial modelling analysis. Lancet Infect. Dis. 21, 59–69. doi:10.1016/S1473-3099(20)30700-3
Wellems, T. E., and Plowe, C. V. J. T. J. O. I. D. (2001). Chloroquine-resistant malaria. Chloroquine-resistant Malar. 184, 770–776. doi:10.1086/322858
World Health Organization (2015). Global technical strategy for malaria 2016-2030. World Health Organization.
Keywords: ionophores, drug-interaction, isobologram, P. falciparum, antimalarials
Citation: Rajendran V and Gurukkalot K (2023) In vitro drug interaction of ionophores with artemisinin and chloroquine against Plasmodium falciparum 3D7 blood-stage infection. Front. Drug Discov. 3:1257698. doi: 10.3389/fddsv.2023.1257698
Received: 12 July 2023; Accepted: 02 October 2023;
Published: 14 November 2023.
Edited by:
Agam Prasad Singh, National Institute of Immunology (NII), IndiaReviewed by:
Ngozi J. Nwodo, University of Nigeria, NigeriaSatish Mishra, Central Drug Research Institute (CSIR), India
Copyright © 2023 Rajendran and Gurukkalot. This is an open-access article distributed under the terms of the Creative Commons Attribution License (CC BY). The use, distribution or reproduction in other forums is permitted, provided the original author(s) and the copyright owner(s) are credited and that the original publication in this journal is cited, in accordance with accepted academic practice. No use, distribution or reproduction is permitted which does not comply with these terms.
*Correspondence: Vinoth Rajendran, dmlub3RoLmF2akBnbWFpbC5jb20=
†These authors have contributed equally to this work and share first authorship
‡ORCID: Vinoth Rajendran, https://orcid.org/0000-0003-1453-1139