- 1Biolacuna Ltd., Oxford, United Kingdom
- 2Münster University Children’s Hospital and Center for Rare Diseases, Münster, Germany
- 3South Carolina Bioengineering Center for Regeneration and Formation of Tissues, Clemson University, Clemson, SC, United States
- 4Elastrin Therapeutics Inc., Greenville, SC, United States
- 5Department of Paediatrics, University of Oxford, Oxford, United Kingdom
Ectopic calcification disorders, including Generalized Arterial Calcification of Infancy (GACI) and Pseudoxanthoma Elasticum are rare but impactful on individuals, healthcare and society, with significant associated morbidity, mortality and healthcare costs. Available therapies are not curative and focus on reducing extracellular calcification to limit progression of the arteriopathy that is responsible for much of the morbidity and, in the case of GACI, significant early mortality (approximately 50% in infancy). In this article, current and emerging medical approaches are reviewed and critiqued, including dietary manipulation, phosphate binders, bisphosphonates, tissue nonspecific alkaline phosphatase inhibitors, ectonucleotide pyrophosphatase/phosphodiesterase 1 (ENPP1) enzyme replacement, allele-specific therapies, gene therapies, and antibody targeted treatment. Available therapies may limit further arterial calcification, but in GACI in particular, significant calcification can be present at birth, contributing to high infant mortality. This highlights the need for new approaches that aim to reverse established calcification, rather than merely slow its progression. Recently, a promising new class of antibody-targeted nanoparticle therapeutics has emerged that can reverse established arterial calcification in animals, restoring arterial elasticity. In one realization, nanoparticles carry established chelators, such as ethylenediaminetetraacetic disodium acid, to sites of arterial damage, concentrating the impact of the chelator where it is needed and limiting off-target effects. Such drugs would complement existing and emerging therapies, such as ENPP1 enzyme replacement, that slow or prevent progression of calcification, by offering an opportunity to “reset” arterial health in ectopic calcification disorders. At present, ectopic calcification disorders are challenging to treat effectively and carry a high burden of morbidity and mortality, particularly in GACI. Recent drug developments offer good reason to be hopeful for a new era of effective therapeutics that may reverse established arterial disease as well as halt its progression.
1 Introduction
Extra-skeletal, ectopic calcification of soft tissues is the pathological basis of a variety of disorders and may result from aging, injury, or disease. When the calcification affects the cardiovascular system, calcium phosphate crystals are deposited in the arteries, causing arterial inflammation and stenosis, reducing arterial compliance, and raising blood pressure. This accelerates other forms of vascular disease, such as atherosclerosis, transmits wide pulse pressures more effectively to vulnerable organ vascular beds, and increases cardiac afterload. The risk of adverse cardiovascular outcomes such as heart failure, myocardial infarction, stroke, and intestinal, limb, renal and retinal ischemia is increased.
Two rare syndromes, Generalized Arterial Calcification of Infancy (GACI) and Pseudoxanthoma Elasticum (PXE), form the archetype of heritable ectopic calcification disorders. They stem from similar, sometimes overlapping abnormalities in the metabolic processes responsible for regulating extracellular levels of inorganic phosphate, the adenosine phosphates, adenosine itself, and inorganic pyrophosphate. In addition to the significant morbidity and mortality associated with these conditions, they contribute to the major societal cost of rare diseases, estimated to be in the range of US$7.2—8.6 trillion per year, globally for all rare diseases (Andreu et al., 2022). Development of effective therapy for the ectopic calcification disorders would, therefore, be impactful at multiple levels, including substantial healthcare cost benefits.
2 Prevalence and etiology
On clinical criteria, GACI is estimated to affect 1 in 200,000 pregnancies worldwide (Ferreira et al., 2021a), with equal representation in all races/ethnicities and both males and females (Ziegler et al., 2020). Genetic screening studies suggest that this underestimates the prevalence of the responsible genetic abnormality (ectonucleotide pyrophosphatase/phosphodiesterase 1 [ENPP1] deficiency in most cases of GACI), which is estimated to occur globally in as many as 1 in 64,000 pregnancies (Chunn et al., 2022). The estimated prevalence of PXE is around 1 in 25,000 (Chassaing et al., 2005; Bartstra et al., 2021) to 1 in 56,000 (Kranenburg et al., 2019; Stumpf et al., 2021) with females being affected twice as often as males (Bercovitch and Terry, 2004). Using these prevalence estimates as a guide, Uitto and others have conservatively estimated that there may be as many as 7,000–8,000 individuals with PXE living in the United States, and as many as 150,000 persons worldwide (Uitto et al., 2014). Low clinical awareness of both conditions could account for some of the disparity between prevalence estimates and diagnosis rates (Rutsch et al., 2003; Li et al., 2014; Mastrolia et al., 2015). Clinical diagnosis may not be made in all cases of GACI due to the high fetal and infant mortality linked to the condition. Under-counting is a likely consequence of this, particularly in lower-to-middle income countries where infant mortality rates are higher and routine investigation of sudden infant deaths is less likely to occur (Chunn et al., 2022). As clinical signs of GACI are observable in utero as early as 18 weeks of gestation with the assistance of fetal ultrasound (Mastrolia et al., 2015), there may also be cases that are terminated before birth. Enhanced use of routine genetic screening would improve our knowledge of both of these syndromes (Chunn et al., 2022).
GACI and PXE are both genetic disorders that are typically autosomal recessive in their pattern of inheritance, with a mutated gene inherited from each parent, although spontaneous mutation can occur. The primary locus for GACI, found in around three-quarters of those diagnosed, results from mutation of the ENPP1 gene (6q23.2) (Rutsch et al., 2003; Rutsch et al., 2008; Li et al., 2014). This is known as GACI Type 1. The secondary locus for GACI, found in around 1 in 10 of those diagnosed, results from mutation of the ATP-Binding Cassette subfamily C member 6 (ABCC6) gene (16p13.11) (Rutsch et al., 2003). This is known as GACI Type 2. Other spontaneous causes of GACI have been described without mutation of either of these genes, but the cause in such cases is unknown (Kranenburg et al., 2018b).
The majority (∼80%) of PXE cases also result from mutations in ABCC6, but some cases have been described with mutations in ENPP1 (Bergen et al., 2000). Thus, there is overlap between genetic causes for PXE and GACI, with the clinical expression possibly dependent on other factors, such as the coinheritance of modifier genes that have yet to be discovered. The genetic and phenotypic overlap between these conditions has led some to suggest that they may represent two ends of a spectrum of disease, rather than distinct disorders (Van Dyck et al., 1989).
The protein encoded by ABCC6 is a membrane-bound, adenosine triphosphate (ATP)-dependent transporter. It is heavily expressed in hepatocytes and by proximal renal tubular cells, with limited expression in other tissues (Uhlén et al., 2015; Human Protein Atlas, 2023). Hepatic expression functions to excrete ATP into the circulation. Although it transports a variety of molecules out of cells, including organic anions, its ability to release ATP itself from cells into the extracellular space has been demonstrated as the mechanism by which ABCC6 mutations promote ectopic calcification (Jansen et al., 2013; Jansen et al., 2014). This extracellular ATP is the substrate for the extracellular enzymatic activity of the type II transmembrane protein encoded by ENPP1, which converts that ATP into inorganic pyrophosphate and adenosine monophosphate (AMP). ENPP1 is also expressed by hepatocytes and many other tissues, including arterial smooth muscle (Uhlén et al., 2015; Human Protein Atlas, 2023). AMP is further metabolized into inorganic phosphate and adenosine by the cell surface protein, CD73 (NT5E), which is expressed in most tissues (Uhlén et al., 2015; Human Protein Atlas, 2023). Inorganic pyrophosphate is metabolized by another cell surface protein, tissue-nonspecific alkaline phosphatase (TNAP) into inorganic phosphate, which is also widely expressed (Uhlén et al., 2015; Human Protein Atlas, 2023). This enzymatic cascade is illustrated in Figure 1.
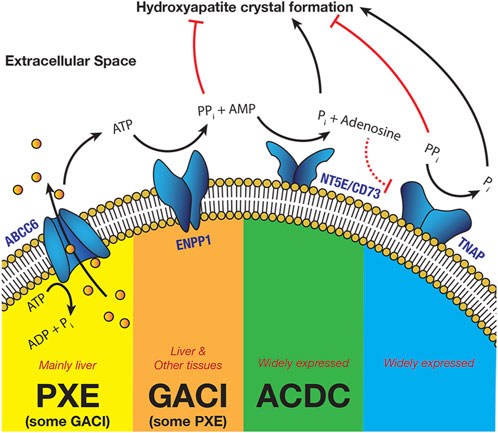
FIGURE 1. The Enzymatic Cascade of Pyrophosphate Metabolism (Schematic view—different steps can take place in different cell types) (PXE—Pseudoxanthoma elasticum, GACI—Generalized Arterial Calcification of Infancy, ACDC—Arterial Calcification due to Deficiency of CD73).
Inorganic pyrophosphate is a potent inhibitor of hydroxyapatite crystal formation, a hard mineral form of calcium phosphate. Such calcification occurs in a number of tissues, but is particularly common in the elastic tissues, including the arterial wall, the eyes and the skin. Reduced ENPP1 or ABCC6 expression both result in lower extracellular levels of inorganic pyrophosphate, promoting calcification, with levels being generally much lower in GACI than in PXE (Ralph et al., 2022). Importantly, reduced ENPP1 activity also lowers extracellular levels of AMP and of adenosine after conversion of AMP by CD73. Adenosine has at least two important functions: it is a potent inhibitor of TNAP, by downregulation of TNAP expression via the A3 Adenosine Receptor (Fujimoto et al., 2019) and it inhibits myointimal proliferation in the arterial wall (Dubey et al., 2015). Myointimal proliferation is an important element of the occlusive arteriopathy that characterizes GACI and is likely to be responsible for much of the early morbidity and mortality associated with the condition (Rutsch et al., 2008). This is the primary distinction between GACI and PXE, where such occlusive disease is not known to occur.
Mutations in other proteins in this metabolic cascade also occur and may result in similar, allied conditions. For example, Arterial Calcification Due to CD73 deficiency results, as the name suggests, from mutations in the NT5E gene which codes for CD73. Reduced CD73 activity reduces availability of extracellular adenosine, again promoting calcification through a lack of TNAP inhibition. These metabolic and phenotypic commonalities between the heritable ectopic calcification disorders suggest that many treatment innovations are likely to be beneficial across the range of these diseases (Van Dyck et al., 1989; Galletti et al., 2011; Ferreira et al., 2021a).
3 Clinical presentation and diagnosis
There are similarities in the clinical presentation of GACI and PXE, but most features in GACI tend to be more severe and to present earlier. GACI typically manifests in infants (Ziegler et al., 2020) with arterial calcification and intimal thickening, which causes lumen stenosis or obliteration. Patients also suffer osteomalacia, hearing loss, extravascular calcification, dermatological changes and eye changes such as angioid streaks (Ziegler et al., 2020). PXE is characterized by similar dermatological and ophthalmological manifestations, with arterial calcification not typically apparent until adulthood (Nitschke and Rutsch, 2017). Marked intimal thickening and resultant stenosis or obliteration of arteries has not been described in PXE. Although most patients without substantial eye involvement follow a relatively benign clinical course, some present acutely with gastrointestinal hemorrhage or ischaemic cardiovascular events (Naouri et al., 2009). This can occur at any age and sudden deaths in childhood have been reported (Sakata et al., 2006). There is also chronic associated morbidity, with symptoms such as angina, muscular cramps and claudication in all limbs. Arterial hypertension is very common in this population, affecting approximately 25% of cases, even when young. This can be related to a combination of renal arterial calcification and arterial stiffness, which is known to be significantly increased in PXE (Kranenburg et al., 2018b).
The affected vascular territories differ in these two conditions. In GACI, pathological examination has shown that the hepatic (81% of cases), aorta (80%), pulmonary (67%), coronary (53%) and renal (39%) arteries are most commonly affected in early-onset disease, with the coronary (88%), renal (55%), pulmonary (49%), aorta (36%), adrenal (34%), splenic (31%), pancreatic (28%), and mesenteric (26%) arteries being affected in late-onset disease (Chong and Hutchins, 2008). In one group of long-term survivors, arterial calcification was found in the aorta (14/16), and renal (11/16), mesenteric (11/16), coronary (10/16), iliac (10/16), and pulmonary (10/16) arteries (Ferreira et al., 2021a). The cerebral arteries are typically spared in GACI although there are reports of involvement, with consequent strokes or seizures (Van Dyck et al., 1989; Galletti et al., 2011). There are also reports of spontaneous resolution of calcification in some patients (Ciana et al., 2006) and generalized arterial stenosis can occur without calcification (Ferreira et al., 2021a). In PXE, by contrast, central arterial involvement is less common, with arteries affected by calcification tending to be peripheral (Leftheriotis et al., 2014). In one study, calcification was present in the sub-popliteal (85%), femoral-popliteal (74%), intracranial internal carotid (75%) arteries and the arteries of the arms (21%) in a population aged over 45 years (Leftheriotis et al., 2014). Such arm involvement is almost exclusively found in PXE.
Diagnosis of both GACI and PXE is made with reference to a set of criteria incorporating clinical observation, histopathological changes, and medical imaging. No consensus criteria have yet been formally accepted for GACI due to similarities with PXE (Ziegler et al., 2020; Kawai et al., 2022), but for PXE, consensus diagnostic criteria are used (Lebwohl et al., 1994). Updated criteria have been proposed that account for new understanding of the role of specific genetic mutations (Plomp et al., 2010). Nevertheless, as both GACI and PXE are rare, diagnosis remains challenging at least in part due to a lack of awareness and expertise among healthcare professionals.
4 Prognosis
Early diagnosis in both GACI and PXE is critical to optimum management and outcomes. Without timely and effective treatment, prognosis in GACI is poor. Around one-quarter of patients die in utero or are stillborn (Kawai et al., 2022), and half of surviving newborns die from acute cardiovascular events within 6 months of birth, despite medical care (Rutsch et al., 2008). Those who survive may live into adulthood, but often need intensive medical management of complications such as hearing loss, dental and bone abnormalities, including Rickets, stroke-induced cognitive impairment and cardiovascular disease (LaRusso et al., 2009; Jiang and Uitto, 2012).
By contrast, effective management of PXE can usually be achieved by avoidance of medications that worsen it, including non-steroidal anti-inflammatory analgesics, warfarin, and estrogens, by dietary changes to limit calcium intake and reduce blood pressure, and by regular low-impact exercise (Laube and Moss, 2005). Nevertheless, although the majority have a normal life expectancy (Chassaing et al., 2005), this depends on the extent of internal organ involvement (Laube and Moss, 2005; Kumar et al., 2012), with premature mortality typically being due to sudden cardiac failure, myocardial infarction, ischemic stroke, or gastrointestinal hemorrhage in the third to fifth decade of life (De Vilder and Vanakker, 2015).
5 Therapeutic approaches
There are no curative therapies available for ectopic calcification disorders currently. Existing therapies focus on reducing extracellular calcification to limit the impact of the arteriopathy and other consequences of such calcification. Most therapeutic approaches currently focus on addressing the low levels of inorganic pyrophosphate that are characteristic of ectopic calcification disorders. There have been few trials of novel therapies for GACI or PXE. The rarity of these conditions has meant that studies have usually had to rely on retrospective data without standardized protocols or diagnostic criteria, particularly in GACI (Boyce et al., 2020). This has contributed to a lack of consensus on optimum treatment protocols in both conditions.
5.1 Dietary management and supplementation
Few studies have explored the potential of dietary management and supplementation in GACI. Although increased dietary magnesium during pregnancy has been shown to prevent aortic mineralization in offspring of Enpp1asj mice that develop a GACI-like phenotype (Kingman et al., 2017), no trials of dietary manipulation in GACI have been registered with ClinicalTrials.gov as of 19 March 2023. By contrast, carotid artery wall thickness and connective tissue mineralization both improve with dietary magnesium supplementation in Abcc6−/− knockout mice that develop a PXE-like phenotype (LaRusso et al., 2009; Jiang and Uitto, 2012; Kupetsky-Rincon et al., 2012; Ibold et al., 2019).
A single randomized controlled trial (RCT) of magnesium supplementation in humans with PXE had promising results (Rose et al., 2019). Forty-four adults with PXE were randomized to take either 800 mg magnesium oxide (equivalent to 500 mg elemental magnesium) tablets or placebo twice-daily for 12 months. Decreased skin elastic fiber calcification was observed in the treatment group compared to the placebo group, but the difference was not statistically significant. Just over three-quarters of those in the treatment group had a clinically significant (30% or more) reduction in elastic fiber calcification. The precise mechanism of action for dietary magnesium supplementation is uncertain, but magnesium ions are known to be a direct competitive antagonist for calcium ions in many chemical interactions (Nitschke and Rutsch, 2017).
Direct oral supplementation using inorganic pyrophosphates has also been used since the first descriptions of its absorption in both mice and humans to treat GACI and PXE (Dedinszki et al., 2017). A single patient case report from Finland further demonstrated the potential of this treatment option when it was used to try to support re-vascularization of an ischemic leg (Väärämäki et al., 2019). Satisfactory uptake of orally delivered pyrophosphate has been further confirmed in a cohort study of both healthy volunteers and PXE patients (Kozák et al., 2022). A clinical trial (NCT04868578) is now underway to examine the effect of oral PPi supplementation on arterial calcification scores.
5.2 Phosphate binders
Increased dietary phosphate levels have been observed to accelerate mineralization in Abcc6−/− mice (Jiang and Uitto, 2012), suggesting a potential for phosphate binders to slow progression of PXE by limiting intestinal absorption of phosphate from the diet (Luo et al., 2020). A small case series in six people found that daily administration of 1,800 mg liquid aluminum hydroxide or 600 mg aluminum hydroxide tablets three times a day potentially improves the histological appearance of characteristic skin lesions in PXE and might prevent deterioration of eye disease (Sherer et al., 2005). A larger RCT later found no difference in calcification in those taking 800 mg sevelamer hydrochloride, another phosphate binder, three times a day for 2 years and those taking placebo (Yoo et al., 2011). No trials were registered on ClinicalTrials.gov as of 19 March 2023 that address the effects of phosphate binders in GACI.
5.3 Bisphosphonates
Bisphosphonates, pyrophosphate analogues which form a class of drugs usually used to prevent bone demineralization in osteoporosis and other bone disorders such as Paget’s disease or bone cancers, have been the first-line treatment for GACI for over 50 years (Nitschke and Rutsch, 2017). More recently, bisphosphonates have also been shown to be effective in reducing calcification in those with PXE caused by mutation of the ABCC6 gene (Bartstra et al., 2020). Some bisphosphonates can inhibit mineralization and calcification outside of areas of active bone deposition, thereby reducing calcification of soft tissues. High concentrations of etidronate, but not alendronate, reduced mineralization of hair follicles (Li et al., 2015), skin, and aortic tissue in Abcc6−/− mice (Li et al., 2016).
Most studies in patients with GACI have suggested benefits from bisphosphonate treatment, including improved survival rates (Meradji et al., 1978; Rutsch et al., 2008; Ramjan et al., 2009; Edouard et al., 2011). However, it has been suggested that these apparent benefits could result from selection bias in such studies, due to variations in treatment strategy according to disease severity. A recent study found that survival differences could not be demonstrated when treated and untreated cases were matched by age and compared from the age at which treated cases received bisphosphonates onwards (Ferreira et al., 2021b). Isolated case studies suggest that dietary magnesium supplementation might add to the effects of anti-phosphate therapy, particularly in cases of GACI who fail to respond to bisphosphonates alone (Dursun et al., 2019). However, much of the evidence underpinning the use of bisphosphonates in ectopic calcification disorders, particularly in GACI, is based on single case reports or case series. More recently, a single RCT, published as two separate papers, suggested that etidronate may reduce arterial calcification in adults with PXE (Kranenburg et al., 2018a; Bartstra et al., 2020). Specifically, in a pre-specified post hoc analysis the authors found that fortnightly treatment with 20 mg/kg etidronate over a period of 12 weeks was associated with cessation of calcification in the common carotid artery, the coronary arteries, the thoracic aorta, and a lower calcification rate in the abdominal aorta, the iliac arteries, and the arteries of the legs at 1 year. Results for the femoral arteries were published separately, showing similar benefits. To date (19 March 2023), no RCTs of bisphosphonates in GACI have been registered with ClinicalTrials.gov.
5.4 TNAP inhibitors
TNAP is an enzyme that facilitates degradation of inorganic pyrophosphate, a potent inhibitor of calcification (Luo et al., 2020). It has, therefore, been hypothesized that TNAP inhibition would raise inorganic pyrophosphate levels, counteracting the low levels that are typical in PXE. A study in mice found that a small molecule TNAP inhibitor decreased abnormal mineralization in a PXE mouse model, but not in a GACI mouse model (Ziegler et al., 2017; Li et al., 2019). In humans, a single small RCT found that 30 mg lansoprazole daily increased pyrophosphate levels over an 8-week period by 18% on average, although significant between-person variability was observed (−34% to +85%) (Murcia Casas et al., 2023). A second RCT of the TNAP inhibitor DS-1211b administered at low, medium, and high doses (undefined) for a period of 12-week is currently underway in adults with PXE (NCT05569252).
A recent study in a mouse model of Hutchinson-Gilford progeria syndrome, which also causes excessive ectopic vascular calcification, found that low levels of inorganic pyrophosphate were likely to be responsible for this (Villa-Bellosta, 2019). They found that combined use of levamisole, a TNAP inhibitor, with ARL67156, a CD39 (eNTPD) inhibitor increased the synthesis and reduced the degradation of pyrophosphate in aortas and blood ex vivo. CD39 promotes the degradation of extracellular ATP to AMP and inorganic phosphate. The apparent benefit of its inhibition in this study suggests it may also be a therapeutic target of interest in ectopic calcification disorders.
The development of new TNAP inhibitors has been further advanced by the use of computational studies that have sought to identify possible inhibitors and define their molecular architecture (Andleeb et al., 2020). These developments should facilitate faster and more cost effective candidate molecule identification and so hopefully speed up the progress of these treatments to clinical trials. However, no human trials of TNAP inhibitors in GACI had been registered on ClinicalTrials.gov as of 19 March 2023.
5.5 ENNP1 enzyme replacement therapy
ENPP1 is a key enzyme that promotes the conversion of adenosine triphosphate (ATP) into adenosine monophosphate (AMP) and inorganic pyrophosphate. ENPP1 mutations reduce this activity to very low or, in some cases, undetectable levels (Luo et al., 2020). Replacement therapy might address this, normalizing extracellular inorganic pyrophosphate levels. In studies of Enpp1asj mice that exhibit a GACI-like phenotype, administration of recombinant ENPP1 protein was found to reduce arterial calcification, thereby improving cardiovascular function and blood pressure, and reducing premature mortality (Albright et al., 2015; Khan et al., 2018; Nitschke et al., 2018). ENNP1 enzyme replacement therapy has also been shown to normalize plasma inorganic pyrophosphate levels and prevent mineralization in Abcc6−/− mice, suggesting a potential role in treating PXE as well (Zhao et al., 2017).
ENPP1 replacement should not only address the lack of pyrophosphate that drives ectopic calcification, but also address the lack of adenosine. Although calcification plays a significant role in the arteriopathy of GACI, myointimal proliferation resulting from a lack of adenosine is thought to be responsible for the obliterative arterial disease that causes accelerated heart failure and hypertension and much of the associated early morbidity and mortality. Importantly, all other therapeutic approaches address only the calcification element of GACI and do not address the myointimal proliferation. Nevertheless, although it may prevent worsening, ENPP1 replacement is unlikely to be able to reverse established, mature ectopic calcification whilst other therapies are being developed that might. Thus, a combination of such therapies may prove to be optimal in the treatment of GACI and possibly other ectopic calcification disorders.
A series of RCTs sponsored by the same pharmaceutical company is currently underway to investigate the safety and tolerability of ENPP1 enzyme replacement therapy in infants diagnosed with ENPP1 deficiency (NCT05734196), and in adults diagnosed with ENPP1 deficiency (NCT04686175) or ABCC6 deficiency (NCT05030831). These RCTs are Phase 1–2 first-in-human trials, and as such aim to investigate the safety, tolerability, pharmacokinetics, and pharmacodynamics of INZ-701 at doses of either 0.2 or 0.6 mg/kg, or for adults, 1.8 mg/kg. The latter of these three trials is not currently recruiting and the low incidence of these conditions means that results from the other trials may not be available for some time, possibly years.
5.6 Allele-specific therapies
Several treatments are being investigated to target specific allelic mutations. Premature termination codon suppressors, for example, act by inhibiting the effect of mutations that cause premature termination codons. They have been shown to have somewhat variable effects in animal models (Zhou et al., 2013), but have not yet entered clinical trials. The chemical chaperone 4-phenylbutyrate (4-PBA) has been investigated in PXE mutations where there is a lack of appropriate localization of the ABCC6 protein to the cell membrane (Pomozi et al., 2017). This has been shown to have some efficacy in specific mutations but has not yet been translated into clinical trials. The specific, small populations that some of these treatments could apply to presents a major challenge for their clinical development.
5.7 Gene therapies
Gene therapy, aimed at ABCC6 mutations has been shown to be effective in animal models (Huang et al., 2019), with significant reductions in ectopic mineralization. Another potential treatment using gene therapy to upregulate the expression of fetuin-A, a circulating anti-mineralization factor, has been investigated. Animal studies have shown this approach to be successful in reducing soft tissue mineralization in Abcc6−/−mice (Jiang et al., 2010). Unlike allele-specific therapies, gene therapies such as these would, usually, be applicable to a wider variety of individuals. However, a number of unaddressed issues still present obstacles in translating these developments into clinical use, such as the immunogenicity of the vector. No relevant clinical trials are currently registered on ClinicalTrials.gov.
5.8 Calcium crystallization inhibitors
SNF472, an intravenous formulation of myo-inositol hexaphosphate, is a calcification inhibitor with a novel mechanism of action, that is currently undergoing trials (NCT04195906). It acts by binding to hydroxyapatite crystals at their growth sites (Ferrer et al., 2018). Current phase III trials aim to test its ability to reduce the progression and painfulness of the ischaemic skin ulceration associated with calciphylaxis, or calcific uremic arteriolopathy, a complication in 4% of end-stage renal disease. The cause of such ulceration is progressive cardiovascular calcification, particularly of the arterioles, which is similar to that seen in the heritable ectopic calcification disorders. Thus, it is hoped that SNF472 may limit vascular calcification rates not only in calcific uremic arteriolopathy of renal dialysis patients, but also in disorders like GACI and PXE (Perelló et al., 2020).
5.9 Antibody-targeted treatment
Another novel treatment pathway being investigated is the use of antibody-targeted nanoparticles to deliver active payload substances directly to areas of elastin damage, which colocalize with ectopic mineralization. This approach could have a number of important advantages, including the ability to increase the localized concentrations of the payload substance and the duration of its exposure to the sites where it is needed, whilst minimizing off-target drug delivery. With this approach, payload substances can achieve localized concentrations that would not be possible with direct systemic delivery of the same substance without significant off-target side-effects. This should increase efficacy and the range of potential substances that can be used safely.
Such antibody-targeted drugs are typically delivered by intravenous administration and the safety and efficacy of a number of realizations of this technology is now well-established in preclinical studies of vascular damage and aneurysms, notably in rat (Nosoudi et al., 2016a; Nosoudi et al., 2016b) and mouse (Wang et al., 2021) models. The active substances delivered experimentally by this approach to date have included well-known chelation agents (Nosoudi et al., 2016a), novel, polyphenol-derived compounds (Nosoudi et al., 2016a; Nosoudi et al., 2016b; Wang et al., 2021), and a combination thereof (Nosoudi et al., 2016a). Chelating agents, such as EDTA and diethylenetriamine penta-acetic acid have demonstrated an ability to reduce arterial calcification in a variety of animal models and, in humans, as the active ingredient of a cocktail of substances routinely used in chelation therapy, in the Trial to Assess Chelation Therapy (Lei et al., 2013; Lei et al., 2014; Karamched et al., 2019; Keuth et al., 2020). Research is underway to combine these chelating agents with nanoparticles of human serum albumin to create a biodegradable and non-toxic matrix that can be linked with an antibody that binds specifically with epitopes that are only exposed when elastin is damaged. This should enable delivery of payload drugs to sites of arterial damage, as well as other areas of the body where elastic tissues have been damaged (Keuth et al., 2020). Ectopic calcification of the arterial wall and other elastic tissues is largely localized at sites of elastin damage and both processes have been shown to be mutually causative (Chen and Moe, 2012). Thus, such targeted nanoparticle drugs have the potential to specifically treat the arteriopathies associated with heritable ectopic calcification disorders and other conditions where arterial damage is associated with calcification whilst minimizing ectopic demineralization of tissues such as bone and other possible off-target effects. They also have potential benefits in other tissues where ectopic calcification occurs. An example of this would be calciphylaxis, where microvascular calcification can lead to tissue ischemia. This occurs predominantly in fat and skin tissue and can lead to skin necrosis (Rick et al., 2022).
The efficacy and safety of the chelation agents and polyphenolic compounds used to date as payloads in the development of this new class of drugs has been established in preclinical testing. The ability of these targeted drugs to demineralize established ectopic calcification, particularly in arteries, and promote elastin integrity has been demonstrated in both rats (Karamched et al., 2019) and mice (Crandall et al., 2023). In animal models, ethylenediaminetetraacetic disodium acid (EDTA)-loaded particles reverse established arterial calcification (Lei et al., 2014) and pentagalloylglucose (PGG), a polyphenolic compound related to tannic acid and a potent antioxidant, has been shown to restore arterial elasticity by repairing damaged elastin (Crandall et al., 2023). Their effects are likely to be complementary, as arterial calcification itself increases arterial stiffness and experimentally induced calcification through direct application of calcium chloride to arteries has been shown to promote elastin damage and arterial inflammation (Basalyga et al., 2004; Nosoudi et al., 2016a). Commensurately, elastin damage may be an essential precursor to medial arterial calcification. Vyavahare et al. were the first to show that elastin calcification was linked to increased matrix metalloproteinase (MMP) expression (Vyavahare et al., 2000) and that the process can be stopped using a synthetic MMP inhibitor, BB-1101. Mice where MMP genes have been knocked out do not develop such calcification in experiments where wild-type mice do (Basalyga et al., 2004). These experiments suggest that elastin degradation by MMPs, secreted by inflammatory cells or smooth muscle cells in the arterial wall, is required for medial arterial calcification to develop. Indeed MMPs may degrade medial elastin, resulting in the liberation of soluble elastin peptides, which bind the elastin-laminin receptor (ELR) present on most cells, stimulating MMP production and that of other serine elastases (Lee et al., 2006). This results in a positive feedback loop, with a cycle of MMP-mediated elastin degradation, inflammatory cell recruitment, and further MMP secretion. Soluble elastin peptides can also interact with the ELR to induce osteogenic changes in smooth muscle cells (Simionescu et al., 2005). Thus, arterial calcification and elastin damage are mutually-dependent processes and the treatment of either is likely to improve arterial elastic function, through reduced elastin damage, reduced arterial wall inflammation, and decreased calcification.
For example, in rats with moderate arterial aneurysms, EDTA-loaded particles, followed by particles loaded with PGG were found to infiltrate the aneurysms as early as 24 h after administration and deliver a number of benefits. There was a 4-fold decrease in mineral deposits (when compared to the natural course of disease), a 7-fold decrease in the external aneurysm diameter (a marker of aneurysm expansion), a 5-fold decrease in local elastin-degrading enzymatic activity, and a 50% increase in aortic circumferential strain—a proxy biomarker of elastin regeneration in the aorta. Overall, this antibody-targeted treatment restored the elastic lamina, reduced local inflammation, and improved vascular function (Nosoudi et al., 2016a) in a gene- and mutation-agnostic manner potentially applicable to all patients with heritable ectopic calcification disorders.
Crucially, the use of EDTA-loaded particles targeted to damaged elastin is the only one that has the potential to meaningfully reverse established arterial calcification. This might complement therapies such as ENPP1 replacement that prevent further calcification, by offering a “reset” of arterial health at the commencement of such treatment and periodically if ongoing calcification does occur despite preventive therapy. This developing technology holds great promise for the management of ectopic calcification disorders, but it has not yet reached the clinical trial stage.
5.10 Cell engineering
The modification of osteoclasts by tetracycline to improve their targeting to heterotopic ossification has been shown, both in vitro and in a mouse model to reduce heterotopic ossification when compared to non-modified osteoclasts (Jin et al., 2021). This form of treatment has the potential to be applicable in diseases such as PXE and GACI, but has not yet been demonstrated in appropriate animal models.
6 Conclusion
The majority of therapeutic approaches that are available or in development are focused on preventing further ectopic calcification. There is little evidence that current therapies are able to significantly reverse established calcification45. This is particularly important in GACI, where the associated arteriopathy can be advanced by the time of birth, contributing to a high infant mortality rate. It is clear, therefore, that there is a need to prioritize the development of therapies that not only halt future deposition of hydroxyapatite crystals, but also those that can dissolve existing deposits and restore arterial elastic function. In GACI, ENPP1 replacement therapy is the only approach currently that has the potential to limit the occlusive arteriopathy that characterizes this condition, as well as limiting further ectopic calcification. However, for many GACI patients such treatment might already be too late, even by the time of birth, due to the establishment of severe arterial calcification and associated loss of arterial elastic function, which lead to severe hypertension and heart failure. Reversal of established ectopic calcification could be the only way that these severely affected patients might avoid the major morbidity and mortality that is common in such cases. Ongoing treatment with both ENPP1 replacement and antibody-targeted nanoparticle chelation therapy is conceivably an approach that would be complementary. Together, these emerging drugs, if successfully brought to market could become the mainstays for treatment of ectopic calcification disorders that have, hitherto, lacked effective therapies.
There is reason to be hopeful for the future of treatments for GACI and PXE within the next decade with the advent of novel therapeutics that have the potential to not only halt, but also reverse their associated arterial damage that underpins much of their morbidity and mortality. This has the potential to significantly improve the life expectancy and quality of life for patients with these syndromes.
Author contributions
BD and AJ wrote the first draft of the manuscript. NV and FR contributed to sections of the manuscript. All authors contributed to manuscript design and revision, and read and approved the submitted version.
Funding
The authors declare that this study received funding from Elastrin Therapeutics Inc. The funder was not involved in the study design, collection, analysis, interpretation of data, the writing of this article or the decision to submit it for publication.
Conflict of interest
NV is a shareholder in Elastrin Therapeutics Inc. BD, FR, and AJ have provided consultancy services to Elastrin Therapeutics Inc.
Publisher’s note
All claims expressed in this article are solely those of the authors and do not necessarily represent those of their affiliated organizations, or those of the publisher, the editors and the reviewers. Any product that may be evaluated in this article, or claim that may be made by its manufacturer, is not guaranteed or endorsed by the publisher.
References
Albright, R. A., Stabach, P., Cao, W., Kavanagh, D., Mullen, I., Braddock, A. A., et al. (2015). ENPP1-Fc prevents mortality and vascular calcifications in rodent model of generalized arterial calcification of infancy. Nat. Commun. 6, 10006. doi:10.1038/ncomms10006
Andleeb, H., Hussain, M., Abida Ejaz, S., Sevigny, J., Farman, M., Yasinzai, M., et al. (2020). Synthesis and computational studies of highly selective inhibitors of human recombinant tissue non-specific alkaline phosphatase (h-TNAP): a therapeutic target against vascular calcification. Bioorg Chem. 101, 103999. doi:10.1016/j.bioorg.2020.103999
Andreu, P., Karam, J., Child, C., Chiesi, G., and Cioffi, G. (2022). The burden of rare diseases: an economic evaluation. Available at: https://chiesirarediseases.com/assets/pdf/chiesiglobalrarediseases.whitepaper-feb.-2022_production-proof.pdf (Accessed April 18, 2023).
Bartstra, J. W., de Jong, P. A., Kranenburg, G., Wolterink, J. M., Isgum, I., Wijsman, A., et al. (2020). Etidronate halts systemic arterial calcification in pseudoxanthoma elasticum. Atherosclerosis 292, 37–41. doi:10.1016/j.atherosclerosis.2019.10.004
Bartstra, J. W., Risseeuw, S., de Jong, P. A., van Os, B., Kalsbeek, L., Mol, C., et al. (2021). Genotype-phenotype correlation in pseudoxanthoma elasticum. Atherosclerosis 324, 18–26. doi:10.1016/j.atherosclerosis.2021.03.012
Basalyga, D. M., Simionescu, D. T., Xiong, W., Baxter, B. T., Starcher, B. C., and Vyavahare, N. R. (2004). Elastin degradation and calcification in an abdominal aorta injury model: role of matrix metalloproteinases. Circulation 110, 3480–3487. doi:10.1161/01.CIR.0000148367.08413.E9
Bercovitch, L., and Terry, P. (2004). Pseudoxanthoma elasticum 2004. J. Am. Acad. Dermatol 51, S13–S14. doi:10.1016/j.jaad.2004.01.015
Bergen, A. A., Plomp, A. S., Schuurman, E. J., Terry, S., Breuning, M., Dauwerse, H., et al. (2000). Mutations in ABCC6 cause pseudoxanthoma elasticum. Nat. Genet. 25, 228–231. doi:10.1038/76109
Boyce, A. M., Gafni, R. I., and Ferreira, C. R. (2020). Generalized arterial calcification of infancy: new insights, controversies, and approach to management. Curr. Osteoporos. Rep. 18, 232–241. doi:10.1007/s11914-020-00577-4
Chassaing, N., Martin, L., Calvas, P., Le Bert, M., and Hovnanian, A. (2005). Pseudoxanthoma elasticum: a clinical, pathophysiological and genetic update including 11 novel ABCC6 mutations. J. Med. Genet. 42, 881–892. doi:10.1136/jmg.2004.030171
Chen, N. X., and Moe, S. M. (2012). Vascular calcification: pathophysiology and risk factors. Curr. Hypertens. Rep. 14, 228–237. doi:10.1007/s11906-012-0265-8
Chong, C. R., and Hutchins, G. M. (2008). Idiopathic infantile arterial calcification: the spectrum of clinical presentations. Pediatr. Dev. Pathology 11, 405–415. doi:10.2350/07-06-0297.1
Chunn, L. M., Bissonnette, J., Heinrich, S. V., Mercurio, S. A., Kiel, M. J., Rutsch, F., et al. (2022). Estimation of ENPP1 deficiency genetic prevalence using a comprehensive literature review and population databases. Orphanet J. Rare Dis. 17, 421. doi:10.1186/s13023-022-02577-2
Ciana, G., Trappan, A., Bembi, B., Benettoni, A., Maso, G., Zennaro, F., et al. (2006). Generalized arterial calcification of infancy: two siblings with prolonged survival. Eur. J. Pediatr. 165, 258–263. doi:10.1007/s00431-005-0035-6
Crandall, C. L., Caballero, B., Viso, M. E., Vyavahare, N. R., and Wagenseil, J. E. (2023). Pentagalloyl glucose (PGG) prevents and restores mechanical changes caused by elastic fiber fragmentation in the mouse ascending aorta. Ann. Biomed. Eng. 51, 806–819. doi:10.1007/s10439-022-03093-x
Dedinszki, D., Szeri, F., Kozak, E., Pomozi, V., Tokesi, N., Mezei, T. R., et al. (2017). Oral administration of pyrophosphate inhibits connective tissue calcification. EMBO Mol. Med. 9, 1463–1470. doi:10.15252/emmm.201707532
De Vilder, E. Y., and Vanakker, O. M. (2015). From variome to phenome: pathogenesis, diagnosis and management of ectopic mineralization disorders. World J. Clin. Cases 3, 556–574. doi:10.12998/wjcc.v3.i7.556
Dubey, R. K., Fingerle, J., Gillespie, D. G., Mi, Z., Rosselli, M., Imthurn, B., et al. (2015). Adenosine attenuates human coronary artery smooth muscle cell proliferation by inhibiting multiple signaling pathways that converge on cyclin D. Hypertension 66, 1207–1219. doi:10.1161/HYPERTENSIONAHA.115.05912
Dursun, F., Atasoy Öztürk, T., Güven, S., Kırmızıbekmez, H., Seymen Karabulut, G., Kalın, S., et al. (2019). Magnesium and anti-phosphate treatment with bisphosphonates for generalised arterial calcification of infancy: a case report. J. Clin. Res. Pediatr. Endocrinol. 11, 311–318. doi:10.4274/jcrpe.galenos.2018.2018.0204
Edouard, T., Chabot, G., Miro, J., Buhas, D. C., Nitschke, Y., Lapierre, C., et al. (2011). Efficacy and safety of 2-year etidronate treatment in a child with generalized arterial calcification of infancy. Eur. J. Pediatr. 170, 1585–1590. doi:10.1007/s00431-011-1572-9
Ferreira, C. R., Hackbarth, M. E., Ziegler, S. G., Kristen, S., Roberts, M. S., Rosing, D. R., et al. (2021a). Prospective phenotyping of long-term survivors of generalized arterial calcification of infancy (GACI). Genet. Med. 23, 396–407. doi:10.1038/s41436-020-00983-0
Ferreira, C. R., Kintzinger, K., Hackbarth, M. E., Botschen, U., Nitschke, Y., Mughal, M. Z., et al. (2021b). Ectopic calcification and hypophosphatemic Rickets: natural history of ENPP1 and ABCC6 deficiencies. J. Bone Mineral Res. 36, 2193–2202. doi:10.1002/jbmr.4418
Ferrer, M. D., Ketteler, M., Tur, F., Tur, E., Isern, B., Salcedo, C., et al. (2018). Characterization of SNF472 pharmacokinetics and efficacy in uremic and non-uremic rats models of cardiovascular calcification. PLoS One 13, e0197061. doi:10.1371/journal.pone.0197061
Fujimoto, K., Shioi, A., Miki, Y., Kakutani, Y., Morioka, T., Shoji, T., et al. (2019). Adenosine attenuates aortic smooth muscle cell calcification through A3 adenosine receptor. Tohoku J. Exp. Med. 249, 275–283. doi:10.1620/tjem.249.275
Galletti, S., Nitschke, Y., Malavolti, A. M., Aquilano, G., Faldella, G., Corvaglia, L., et al. (2011). Generalized arterial calcification of infancy: fatal clinical course associated with a novel mutation in ENPP1. JIMD Rep. 1, 23–27. doi:10.1007/8904_2011_11
Huang, J., Snook, A. E., Uitto, J., and Li, Q. (2019). Adenovirus-mediated ABCC6 gene therapy for heritable ectopic mineralization disorders. J. Invest. Dermatol 139, 1254–1263. doi:10.1016/j.jid.2018.12.017
Human Protein Atlas (2023). The human protein Atlas. Available at: www.proteinatlas.org (Accessed October 24, 2023).
Ibold, B., Faust, I., Tiemann, J., Gorgels, T. G. M. F., Bergen, A. A. B., Knabbe, C., et al. (2019). Abcc6 deficiency in mice leads to altered ABC transporter gene expression in metabolic active tissues. Lipids Health Dis. 18, 2. doi:10.1186/s12944-018-0943-x
Jansen, R. S., Duijst, S., Mahakena, S., Sommer, D., Szeri, F., Váradi, A., et al. (2014). ABCC6-mediated ATP secretion by the liver is the main source of the mineralization inhibitor inorganic pyrophosphate in the systemic circulation - brief report. Arterioscler. Thromb. Vasc. Biol. 34, 1985–1989. doi:10.1161/ATVBAHA.114.304017
Jansen, R. S., Küçükosmanoglu, A., de Haas, M., Sapthu, S., Otero, J. A., Hegman, I. E. M., et al. (2013). ABCC6 prevents ectopic mineralization seen in pseudoxanthoma elasticum by inducing cellular nucleotide release. Proc. Natl. Acad. Sci. U. S. A. 110, 20206–20211. doi:10.1073/pnas.1319582110
Jiang, Q., Dibra, F., Lee, M. D., Oldenburg, R., and Uitto, J. (2010). Overexpression of fetuin-a counteracts ectopic mineralization in a mouse model of pseudoxanthoma elasticum (abcc6(-/-)). J. Invest. Dermatol 130, 1288–1296. doi:10.1038/jid.2009.423
Jiang, Q., and Uitto, J. (2012). Restricting dietary magnesium accelerates ectopic connective tissue mineralization in a mouse model of pseudoxanthoma elasticum (Abcc6(-/-)). Exp. Dermatol 21, 694–699. doi:10.1111/j.1600-0625.2012.01553.x
Jin, W., Lin, X., Pan, H., Zhao, C., Qiu, P., Zhao, R., et al. (2021). Engineered osteoclasts as living treatment materials for heterotopic ossification therapy. Nat. Commun. 12, 6327. doi:10.1038/s41467-021-26593-1
Karamched, S. R., Nosoudi, N., Moreland, H. E., Chowdhury, A., and Vyavahare, N. R. (2019). Site-specific chelation therapy with EDTA-loaded albumin nanoparticles reverses arterial calcification in a rat model of chronic kidney disease. Sci. Rep. 9, 2629–2711. doi:10.1038/s41598-019-39639-8
Kawai, K., Sato, Y., Kawakami, R., Sakamoto, A., Cornelissen, A., Mori, M., et al. (2022). Generalized arterial calcification of infancy (GACI): optimizing care with a multidisciplinary approach. J. Multidiscip. Healthc. 15, 1261–1276. doi:10.2147/JMDH.S251861
Keuth, J., Nitschke, Y., Mulac, D., Riehemann, K., Rutsch, F., and Langer, K. (2020). Reversion of arterial calcification by elastin-targeted DTPA-HSA nanoparticles. Eur. J. Pharm. Biopharm. 150, 108–119. doi:10.1016/j.ejpb.2020.03.007
Khan, T., Sinkevicius, K. W., Vong, S., Avakian, A., Leavitt, M. C., Malanson, H., et al. (2018). ENPP1 enzyme replacement therapy improves blood pressure and cardiovascular function in a mouse model of generalized arterial calcification of infancy. Dis. Model Mech. 11, dmm035691. doi:10.1242/dmm.035691
Kingman, J., Uitto, J., and Li, Q. (2017). Elevated dietary magnesium during pregnancy and postnatal life prevents ectopic mineralization in Enpp1asj mice, a model for generalized arterial calcification of infancy. Oncotarget 8, 38152–38160. doi:10.18632/oncotarget.16687
Kozák, E., Fülöp, K., Tőkési, N., Rao, N., Li, Q., Terry, S. F., et al. (2022). Oral supplementation of inorganic pyrophosphate in pseudoxanthoma elasticum. Exp. Dermatol 31, 548–555. doi:10.1111/exd.14498
Kranenburg, G., Baas, A. F., de Jong, P. A., Asselbergs, F. W., Visseren, F. L. J., Spiering, W., et al. (2019). The prevalence of pseudoxanthoma elasticum: revised estimations based on genotyping in a high vascular risk cohort. Eur. J. Med. Genet. 62, 90–92. doi:10.1016/j.ejmg.2018.05.020
Kranenburg, G., de Jong, P. A., Bartstra, J. W., Lagerweij, S. J., Lam, M. G., Ossewaarde-van Norel, J., et al. (2018a). Etidronate for prevention of ectopic mineralization in patients with pseudoxanthoma elasticum. J. Am. Coll. Cardiol. 71, 1117–1126. doi:10.1016/j.jacc.2017.12.062
Kranenburg, G., Visseren, F. L. J., de Borst, G. J., de Jong, P. A., and Spiering, W.SMART studygroup (2018b). Arterial stiffening and thickening in patients with pseudoxanthoma elasticum. Atherosclerosis 270, 160–165. doi:10.1016/j.atherosclerosis.2018.02.006
Kumar, P., Sivasubramanian, A., Vadivel, S., and Krishnaswamy, M. (2012). Pseudoxanthoma elasticum with periumbilical perforation in a nullipara. Indian J. Dermatol 57, 210–212. doi:10.4103/0019-5154.96195
Kupetsky-Rincon, E. A., Li, Q., and Uitto, J. (2012). Magnesium reduces carotid intima-media thickness in a mouse model of pseudoxanthoma elasticum: a novel treatment biomarker. Clin. Transl. Sci. 5, 259–264. doi:10.1111/j.1752-8062.2011.00390.x
LaRusso, J., Li, Q., Jiang, Q., and Uitto, J. (2009). Elevated dietary magnesium prevents connective tissue mineralization in a mouse model of pseudoxanthoma elasticum (Abcc6(-/-)). J. Invest. Dermatol 129, 1388–1394. doi:10.1038/jid.2008.391
Laube, S., and Moss, C. (2005). Pseudoxanthoma elasticum. Arch. Dis. Child. 90, 754–756. doi:10.1136/adc.2004.062075
Lebwohl, M., Neldner, K., Pope, F. M., De Paepe, A., Christiano, A. M., Boyd, C. D., et al. (1994). Classification of pseudoxanthoma elasticum: report of a consensus conference. J. Am. Acad. Dermatol 30, 103–107. doi:10.1016/s0190-9622(08)81894-4
Lee, J. S., Basalyga, D. M., Simionescu, A., Isenburg, J. C., Simionescu, D. T., and Vyavahare, N. R. (2006). Elastin calcification in the rat subdermal model is accompanied by up-regulation of degradative and osteogenic cellular responses. Am. J. Pathol. 168, 490–498. doi:10.2353/ajpath.2006.050338
Leftheriotis, G., Kauffenstein, G., Hamel, J. F., Abraham, P., Le Saux, O., Willoteaux, S., et al. (2014). The contribution of arterial calcification to peripheral arterial disease in pseudoxanthoma elasticum. PLoS One 9, e96003. doi:10.1371/journal.pone.0096003
Lei, Y., Grover, A., Sinha, A., and Vyavahare, N. (2013). Efficacy of reversal of aortic calcification by chelating agents. Calcif. Tissue Int. 93, 426–435. doi:10.1007/s00223-013-9780-0
Lei, Y., Nosoudi, N., and Vyavahare, N. (2014). Targeted chelation therapy with EDTA-loaded albumin nanoparticles regresses arterial calcification without causing systemic side effects. J. Control. Release 196, 79–86. doi:10.1016/j.jconrel.2014.09.029
Li, Q., Brodsky, J. L., Conlin, L. K., Pawel, B., Glatz, A. C., Gafni, R. I., et al. (2014). Mutations in the ABCC6 gene as a cause of generalized arterial calcification of infancy: genotypic overlap with pseudoxanthoma elasticum. J. Invest. Dermatol 134, 658–665. doi:10.1038/jid.2013.370
Li, Q., Huang, J., Pinkerton, A. B., Millan, J. L., van Zelst, B. D., Levine, M. A., et al. (2019). Inhibition of tissue-nonspecific alkaline phosphatase attenuates ectopic mineralization in the Abcc6-/- mouse model of PXE but not in the Enpp1 mutant mouse models of GACI. J. Invest. Dermatol 139, 360–368. doi:10.1016/j.jid.2018.07.030
Li, Q., Kingman, J., Sundberg, J. P., Levine, M. A., and Uitto, J. (2016). Dual effects of bisphosphonates on ectopic skin and vascular soft tissue mineralization versus bone microarchitecture in a mouse model of generalized arterial calcification of infancy. J. Invest. Dermatol 136, 275–283. doi:10.1038/JID.2015.377
Li, Q., Sundberg, J. P., Levine, M. A., Terry, S. F., and Uitto, J. (2015). The effects of bisphosphonates on ectopic soft tissue mineralization caused by mutations in the ABCC6 gene. Cell Cycle 14, 1082–1089. doi:10.1080/15384101.2015.1007809
Luo, H., Li, Q., Cao, Y., and Uitto, J. (2020). Therapeutics development for pseudoxanthoma elasticum and related ectopic mineralization disorders: update 2020. J. Clin. Med. 10, 114. doi:10.3390/jcm10010114
Mastrolia, S. A., Weintraub, A. Y., Baron, J., Sciaky-Tamir, Y., Koifman, A., Loverro, G., et al. (2015). Antenatal diagnosis of idiopathic arterial calcification: a systematic review with a report of two cases. Arch. Gynecol. Obstet. 291, 977–986. doi:10.1007/s00404-014-3567-z
Meradji, M., de Villeneuve, V. H., Huber, J., de Bruijn, W. C., and Pearse, R. G. (1978). Idiopathic infantile arterial calcification in siblings: radiologic diagnosis and successful treatment. J. Pediatr. 92, 401–405. doi:10.1016/s0022-3476(78)80427-2
Murcia Casas, B., Carrillo Linares, J. L., Baquero Aranda, I., Rioja Villodres, J., Merino Bohórquez, V., González Jiménez, A., et al. (2023). Lansoprazole increases inorganic pyrophosphate in patients with pseudoxanthoma elasticum: a double-blind, randomized, placebo-controlled crossover trial. Int. J. Mol. Sci. 24, 4899. doi:10.3390/ijms24054899
Naouri, M., Boisseau, C., Bonicel, P., Daudon, P., Bonneau, D., Chassaing, N., et al. (2009). Manifestations of pseudoxanthoma elasticum in childhood. Br. J. Dermatology 161, 635–639. doi:10.1111/j.1365-2133.2009.09298.x
Nitschke, Y., and Rutsch, F. (2017). Inherited arterial calcification syndromes: etiologies and treatment concepts. Curr. Osteoporos. Rep. 15, 255–270. doi:10.1007/s11914-017-0370-3
Nitschke, Y., Yan, Y., Buers, I., Kintziger, K., Askew, K., and Rutsch, F. (2018). ENPP1-Fc prevents neointima formation in generalized arterial calcification of infancy through the generation of AMP. Exp. Mol. Med. 50, 1–12. doi:10.1038/s12276-018-0163-5
Nosoudi, N., Chowdhury, A., Siclari, S., Karamched, S., Parasaram, V., Parrish, J., et al. (2016a). Reversal of vascular calcification and aneurysms in a rat model using dual targeted therapy with EDTA-and PGG-loaded nanoparticles. Theranostics 6, 1975–1987. doi:10.7150/thno.16547
Nosoudi, N., Chowdhury, A., Siclari, S., Parasaram, V., Karamched, S., and Vyavahare, N. (2016b). Systemic delivery of nanoparticles loaded with pentagalloyl glucose protects elastic lamina and prevents abdominal aortic aneurysm in rats. J. Cardiovasc Transl. Res. 9, 445–455. doi:10.1007/s12265-016-9709-x
Perelló, J., Ferrer, M. D., Del Mar Pérez, M., Kaesler, N., Brandenburg, V. M., Behets, G. J., et al. (2020). Mechanism of action of SNF472, a novel calcification inhibitor to treat vascular calcification and calciphylaxis. Br. J. Pharmacol. 177, 4400–4415. doi:10.1111/bph.15163
Plomp, A. S., Toonstra, J., Bergen, A. A. B., van Dijk, M. R., and de Jong, P. T. V. M. (2010). Proposal for updating the pseudoxanthoma elasticum classification system and a review of the clinical findings. Am. J. Med. Genet. A 152A, 1049–1058. doi:10.1002/ajmg.a.33329
Pomozi, V., Brampton, C., Szeri, F., Dedinszki, D., Kozák, E., van de Wetering, K., et al. (2017). Functional rescue of ABCC6 deficiency by 4-phenylbutyrate therapy reduces dystrophic calcification in Abcc6-/- mice. J. Invest. Dermatol 137, 595–602. doi:10.1016/j.jid.2016.10.035
Ralph, D., Nitschke, Y., Levine, M. A., Caffet, M., Wurst, T., Saeidian, A. H., et al. (2022). ENPP1 variants in patients with GACI and PXE expand the clinical and genetic heterogeneity of heritable disorders of ectopic calcification. PLoS Genet. 18, e1010192. doi:10.1371/journal.pgen.1010192
Ramjan, K. A., Roscioli, T., Rutsch, F., Sillence, D., and Munns, C. F. J. (2009). Generalized arterial calcification of infancy: treatment with bisphosphonates. Nat. Clin. Pract. Endrocrinol Metab. 5, 167–172. doi:10.1038/ncpendmet1067
Rick, J., Strowd, L., Pasieka, H. B., Saardi, K., Micheletti, R., Zhao, M., et al. (2022). Calciphylaxis: Part I. Diagnosis and pathology. J. Am. Acad. Dermatol 86, 973–982. doi:10.1016/j.jaad.2021.10.064
Rose, S., On, S. J., Fuchs, W., Chen, C., Phelps, R., Kornreich, D., et al. (2019). Magnesium supplementation in the treatment of pseudoxanthoma elasticum: a randomized trial. J. Am. Acad. Dermatol 81, 263–265. doi:10.1016/j.jaad.2019.02.055
Rutsch, F., Böyer, P., Nitschke, Y., Ruf, N., Lorenz-Depierieux, B., Wittkampf, T., et al. (2008). Hypophosphatemia, hyperphosphaturia, and bisphosphonate treatment are associated with survival beyond infancy in generalized arterial calcification of infancy. Circ. Cardiovasc Genet. 1, 133–140. doi:10.1161/CIRCGENETICS.108.797704
Rutsch, F., Ruf, N., Vaingankar, S., Toliat, M. R., Suk, A., Höhne, W., et al. (2003). Mutations in ENPP1 are associated with “idiopathic” infantile arterial calcification. Nat. Genet. 34, 379–381. doi:10.1038/ng1221
Sakata, S., Su, J. C., Robertson, S., Yin, M., and Chow, C. (2006). Varied presentations of pseudoxanthoma elasticum in a family. J. Paediatr. Child. Health 42, 817–820. doi:10.1111/j.1440-1754.2006.00984.x
Sherer, D. W., Singer, G., Uribarri, J., Phelps, R. G., Sapadin, A. N., Freund, K. B., et al. (2005). Oral phosphate binders in the treatment of pseudoxanthoma elasticum. J. Am. Acad. Dermatol 53, 610–615. doi:10.1016/j.jaad.2004.11.066
Simionescu, A., Philips, K., and Vyavahare, N. (2005). Elastin-derived peptides and TGF-beta1 induce osteogenic responses in smooth muscle cells. Biochem. Biophys. Res. Commun. 334, 524–532. doi:10.1016/j.bbrc.2005.06.119
Stumpf, M. J., Schahab, N., Nickenig, G., Skowasch, D., and Schaefer, C. A. (2021). Therapy of pseudoxanthoma elasticum: current knowledge and future perspectives. Biomedicines 9, 1895. doi:10.3390/biomedicines9121895
Uhlén, M., Fagerberg, L., Hallström, B. M., Lindskog, C., Oksvold, P., Mardinoglu, A., et al. (2015). Proteomics. Tissue-based map of the human proteome. Sci. (1979) 347, 1260419. doi:10.1126/science.1260419
Uitto, J., Jiang, Q., Váradi, A., Bercovitch, L. G., and Terry, S. F. (2014). Pseudoxanthoma elasticum: diagnostic features, classification, and treatment options. Expert Opin. Orphan Drugs 2, 567–577. doi:10.1517/21678707.2014.908702
Väärämäki, S., Pelttari, S., Uusitalo, H., Tőkési, N., Váradi, A., and Nevalainen, P. I. (2019). Pyrophosphate treatment in pseudoxanthoma elasticum (PXE)-Preventing ReOcclusion after surgery for critical limb ischaemia. Surg. Case Rep. 2, 1–3. doi:10.31487/j.scr.2019.04.02
Van Dyck, M., Proesmans, W., Van Hollebeke, E., Marchal, G., and Moerman, P. (1989). Idiopathic infantile arterial calcification with cardiac, renal and central nervous system involvement. Eur. J. Pediatr. 148, 374–377. doi:10.1007/BF00444138
Villa-Bellosta, R. (2019). ATP-based therapy prevents vascular calcification and extends longevity in a mouse model of Hutchinson–Gilford progeria syndrome. Proc. Natl. Acad. Sci. 116, 23698–23704. doi:10.1073/pnas.1910972116
Vyavahare, N., Jones, P. L., Tallapragada, S., and Levy, R. J. (2000). Inhibition of matrix metalloproteinase activity attenuates tenascin-C production and calcification of implanted purified elastin in rats. Am. J. Pathol. 157, 885–893. doi:10.1016/S0002-9440(10)64602-0
Wang, X., Parasaram, V., Dhital, S., Nosoudi, N., Hasanain, S., Lane, B. A., et al. (2021). Systemic delivery of targeted nanotherapeutic reverses angiotensin II-induced abdominal aortic aneurysms in mice. Sci. Rep. 11, 8584. doi:10.1038/s41598-021-88017-w
Yoo, J. Y., Blum, R. R., Singer, G. K., Stern, D. K., Emanuel, P. O., Fuchs, W., et al. (2011). A randomized controlled trial of oral phosphate binders in the treatment of pseudoxanthoma elasticum. J. Am. Acad. Dermatol 65, 341–348. doi:10.1016/j.jaad.2010.05.023
Zhao, J., Kingman, J., Sundberg, J. P., Uitto, J., and Li, Q. (2017). Plasma PPi deficiency is the major, but not the exclusive, cause of ectopic mineralization in an Abcc6-/- mouse model of PXE. J. Invest. Dermatol 137, 2336–2343. doi:10.1016/j.jid.2017.06.006
Zhou, Y., Jiang, Q., Takahagi, S., Shao, C., and Uitto, J. (2013). Premature termination codon read-through in the ABCC6 gene: potential treatment for pseudoxanthoma elasticum. J. Invest. Dermatol 133, 2672–2677. doi:10.1038/jid.2013.234
Ziegler, S. G., Ferreira, C. R., MacFarlane, E. G., Riddle, R. C., Tomlinson, R. E., Chew, E. Y., et al. (2017). Ectopic calcification in pseudoxanthoma elasticum responds to inhibition of tissue-nonspecific alkaline phosphatase. Sci. Transl. Med. 9, eaal1669. doi:10.1126/scitranslmed.aal1669
Ziegler, S. G., Gahl, W. A., and Ferreira, C. R. (2020). Generalized arterial calcification of infancy. GeneReviews. Available at: https://www.ncbi.nlm.nih.gov/books/NBK253403/ (Accessed September 25, 2023).
Keywords: generalized arterial calcification of infancy, Pseudoxanthoma elasticum, arterial calcification, therapeutics, ectopic calcification disorders
Citation: Davies BM, Rutsch F, Vyavahare N and Jones A (2023) Future treatments for the arteriopathy of ectopic calcification disorders. Front. Drug Discov. 3:1249966. doi: 10.3389/fddsv.2023.1249966
Received: 29 June 2023; Accepted: 07 November 2023;
Published: 21 November 2023.
Edited by:
Aloke Finn, CVPath Institute, United StatesReviewed by:
Fernando Prieto-Martínez, National Autonomous University of Mexico, MexicoAndras Varadi, Hungarian Academy of Sciences (MTA), Hungary
Copyright © 2023 Davies, Rutsch, Vyavahare and Jones. This is an open-access article distributed under the terms of the Creative Commons Attribution License (CC BY). The use, distribution or reproduction in other forums is permitted, provided the original author(s) and the copyright owner(s) are credited and that the original publication in this journal is cited, in accordance with accepted academic practice. No use, distribution or reproduction is permitted which does not comply with these terms.
*Correspondence: Alexander Jones, QWxleGFuZGVyLkpvbmVzQGJpb2xhY3VuYS5jb20=