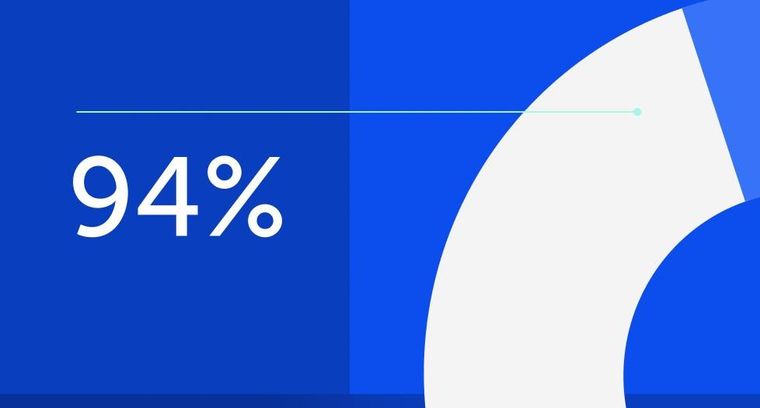
94% of researchers rate our articles as excellent or good
Learn more about the work of our research integrity team to safeguard the quality of each article we publish.
Find out more
REVIEW article
Front. Drug Discov., 01 June 2023
Sec. Anti-Infective Agents
Volume 3 - 2023 | https://doi.org/10.3389/fddsv.2023.1176768
This article is part of the Research TopicDrug Discovery for Emerging and Neglected Tropical Diseases: Advances, Challenges and PerspectivesView all 5 articles
In the 21st Century, emergence and re-emergence of infectious diseases is significant and has an increasing importance in global concern of public health. Based on the COVID-19 pandemic and recently reported epidemics, most human pathogens originate in zoonosis. Many of such pathogens are related to viruses that have RNA genomes, which can be presented structurally as a single-strand or double-strand. During the last two decades, a timeline of major RNA viruses emergencies can be exemplified, such as Severe Acute Respiratory Syndrome Coronavirus (SARS-CoV) in 2003, influenza A virus (H1N1) pdm09 in 2009, Middle East respiratory syndrome coronavirus (MERS-CoV) in 2012, Ebola virus (EBOV) in 2013–2016, Zika virus (ZIKV) in 2015 and the SARS-CoV-2 pdm19 in 2019. Even so, prophylactic or therapeutic drugs are unavailable for many RNA viruses circulating. Nonetheless, the COVID-19 pandemic brought considerable scientific advances in accelerating progress regarding prophylaxis, antiviral and drug development, and novel treatments. Regarding RNA virus diseases for humans, arboviruses play an essential and neglected role, constantly reemerging and affecting almost half of the human population, for which no drug has been licensed. Here we review the consolidated RNA viruses’ emergence and re-emergence in the 21st Century through available data. Then, we explored valuable lessons gained during the SARS-CoV-2 pandemic and focused on potential epidemiologic updates, prophylaxis, available treatments, and viral drug inhibitors. Finally, we explore arbovirus’s significance and the ongoing development of effective vaccines, antiviral drugs, and novel therapeutic approaches as strategies to control these neglected tropical diseases (NTD).
Newly emerging and re-emerging infectious diseases (EID) are becoming a more common and significant threat to human and animal health (Plowright et al., 2017; Plowright et al., 2021). Over the twenty-first century, humankind has witnessed the emergence of several zoonotic viruses, such as severe acute respiratory syndrome coronavirus (SARS-CoV) in 2003, influenza A virus (H1N1) pdm09 in 2009, Middle East respiratory syndrome coronavirus (MERS-CoV) in 2012, Ebola virus (EBOV) in 2013–2016, Zika virus (ZIKV) in 2015 and the SARS-CoV-2 pdm19 in 2019 (Table 1). Like other microorganisms, viruses can evolve to exploit new niches and adapt to expand the host range, thus crossing species boundaries and infecting new hosts through a well-known viral spillover event (Hui, 2006; Ellwanger & Chies, 2021; Spernovasilis et al., 2022). In the last 2 decades, most outbreaks, epidemics, and pandemics events of spillover were frequently related to zoonotic viruses, already have been circulating before in non-human wild or domestic animal reservoirs (Ellwanger & Chies, 2021). These events significantly challenged global health, security, and economic growth during human history, causing immeasurable cases of morbidity and mortality cases each year (Morens et al., 2004; Wolfe et al., 2007; Sakai and Morimoto, 2022). After the SARS-CoV-2 pandemic, some of the major concerns worldwide are to predict and prevent future events of viral emergence and to know where they will take place in order to apply quick actions to minimize viral spread as a public health priority (Heymann et al., 2015; Becker et al., 2019; Bernstein et al., 2022).
However, actions to reduce such events are yet to be fully established due to the different factors driving the dynamics of spillover, such as climate and environmental changes, global air travel network, population growth and urbanization, anthropogenic activities, demographic changes and migration, and agricultural expansion (Jones et al., 2013; Allen et al., 2017; Wilcox & Steele, 2021; Baker et al., 2022; Mitman, 2022). Based on the SARS-CoV-2 pandemic and what was recently reported in the past outbreaks, most of these viruses have RNA as genetic material as a single-strand or double-strand. This review study aims to explore and consolidate the emergence and re-emergence of RNA viruses in the 21st century through available data. Then, we will explore valuable lessons gained during the SARS-CoV-2 pandemic and focus on potential epidemiologic updates, available treatments, and virus-inhibiting drugs. Finally, we demonstrate the great importance of genomic surveillance of NTD and explore the ongoing development of antiviral drugs, novel therapeutic approaches and strategies for NTD.
Historically, epidemics and pandemics of RNA viruses have challenged and directly impacted public health in several countries, raising concerns about the importance of a global epidemiological surveillance system (Devaux, 2012; Khan et al., 2021). It is essential to point out that the origin of RNA viruses is still widely debated as some authors have explained that there might be a possible ancestral origin from a polyphyletic group (Wolfe et al., 2007) evolving from an “ancient virus” (Koonin et al., 2006). RNA viruses generally have small genomes (average size of ∼9 kb) with a higher mutation rate per replication cycle than any other organism (Woolhouse, 2002; Domingo, 2010; Holmes, 2010). Viral genetic variation is an important characteristic providing them with an enhanced capability of rapid viral evolution, adaptation, and host range tropism (Moya et al., 2004). Indeed, this higher mutation rate of RNA viruses is a combination of different mechanisms, such as unique point mutations, recombination, and reassortment (Hui, 2006), which together form the viral genetic variation and help shed light on the importance of these organisms in emerging NTD (Hui, 2006; Dolan et al., 2018; Malecela & Ducker, 2021).
Based on the Baltimore classification system, RNA viruses are composed of four main viral categories: double-stranded (dsRNA viruses), positive-sense single-stranded (+ssRNA viruses), negative-sense single-stranded (−ssRNA viruses) and single positive-stranded RNA with DNA intermediate in life-cycle (ssRNA-RT viruses) (Baltimore, 1971; Koonin et al., 2021). Several viruses of NTD have positive single-stranded RNA (+ssRNA) genomes and are divided into distinct viral families with remarkable differences in genomic composition, RNA replication genes, and structural viral particles. However, the steps and mechanisms of +ssRNA virus replication are sufficiently shared within this viral group. Additionally, viral replication happens in the cytoplasm in which the host machinery is used in multiple stages. These common steps of +ssRNA virus replication bring lessons and strategies which can be applied to different viruses in this group, such as antiviral inhibitors and vaccines (Ahlquist et al., 2003). Some examples of viruses of NTD belonging to this group are SARS-CoV, SARS-CoV-2 and many of the arboviruses (ARthropod-BOrne viruses).
In January 2023, according to the list of NTD provided by the World Health Organization (WHO), only Dengue virus (DENV) and chikungunya virus (CHIKV) can be highlighted as single positive-sense RNA ones (Table 1) (Desiree LaBeaud, 2008; Martins-Melo et al., 2018). Nevertheless, many other viruses could be tracked in the category of NTD, as reviewed previously (Maslow, 2019), thus highlighting the importance of these viruses to provide a comprehensive overview of NTD. A summary of the emergence and re-emergence of RNA viruses in the 21st century is listed in Table 1.
Coronaviridae is a family of viruses which are initially aimed at epithelial cells from the respiratory and digestive systems. Their genome consists of a single-stranded RNA of positive polarity (+ssRNA), the largest + ssRNA viruses described so far, with sizes ranging from 26 to 32 kilobases (kb). Viral proteases are processed into polyproteins, which encodes 16 non-structural proteins (NsP) to drive essential functions, such as genome replication, inhibition of cellular functions and synthesis of sub-genomic RNA (sg mRNA) (Su et al., 2016). The last part of the viral genome encodes for ORFs responsible for structural proteins, including spike (S), envelope (E), membrane (M) and nucleoprotein (N). Many diseases caused by human coronaviruses (HCoVs) are widely dispersed worldwide. Most of the HCoVs known until the 20th century were related to mild respiratory diseases, such as common flu symptoms (Lai & Cavanagh, 1997; McIntosh & Perlman, 2015; Su et al., 2016).
Based on the sequences available in the public databases and protein conservation, the Coronaviridae family (CoVs) can be divided into four genera: alpha-CoV, beta-CoV, gamma-CoV, and delta-CoV (Lai & Cavanagh, 1997; Su et al., 2016). Currently, seven different strains of HCoVs can cause respiratory tract infections, namely,: two alphaCoV strains (HCoV_229 E and HCoV_NL63) and five betaCoV strains, of which two are from group A (HCoV_HKU1 and HCoV_OC43), two from group B (SARS-CoV and SARS-CoV-2) and one from group C (MERS-CoV) (Jacobs et al., 2020). Fortunately, many HCoVs are seasonal viruses circulating endemically in the human population for several decades, thus commonly causing mild respiratory infections depending on each individual’s immune response (Su et al., 2016). However, prior to SARS-COV-2, we had the emergence of two highly pathogenic coronaviruses, that is, SARS-CoV and MERS-CoV (Ksiazek et al., 2003; de Wit et al., 2016).
The first cases of SARS-CoV were identified in Foshan, a city in Guangdong Province, China. The disease was characterized by high fever, cough, myalgia, headache, shortness of breath and respiratory distress as late symptoms of the disease progressing quickly to an “atypical pneumonia” (de Wit et al., 2016; Lu et al., 2020; Paules et al., 2020). The outbreak spread rapidly to 29 countries in different continents. The pandemic ended in July 2003, with 8,096 reported cases and 774 deaths, notably being the first pandemic of this century (de Haan & Rottier, 2005; de Wit et al., 2016; Hua et al., 2020). Other highly pathogenic coronavirus emerged after almost 10 years, the MERS-CoV in 2012, which caused a cluster of severe cases of pneumonia in Jeddah, Saudi Arabia (Hijawi et al., 2013). Similarly, to SARS-CoV, the MERS-CoV infection can be asymptomatic or evolve into a wide range of symptomatic clinical cases. The most significant clinical symptoms are fever, abdominal pain, and in some cases, diarrhea, whereas the most severe cases are characterized by progressive acute pneumonia (Hilgenfeld & Peiris, 2013; Zhu et al., 2020; Cascella et al., 2023). MERS-CoV spread from the Middle East to twenty new countries in distant continents. In 2016, 2,521 cases and 624 deaths were reported, with the death toll recently increasing to 866 deaths (Alyami et al., 2020; Dhama et al., 2020; Ahmad, 2022).
By the end of December 2019, the third highly pathogenic coronavirus was identified in the central hospital of Wuhan, a densely populated city in the central province of Hubei, China. The new HCoV was named severe acute respiratory syndrome coronavirus 2 (SARS-CoV-2) due to its phylogenetic proximity to SARS-CoV (Zhou et al., 2020; Santacroce et al., 2021). This new HCoV could induce systemic symptoms in the severe cases, such as dry cough, fever, fatigue, respiratory pneumonia, and potentially fatal cardiorespiratory failure (Hong et al., 2020; Shi et al., 2020; Zhou et al., 2020; Hay et al., 2022). The disease caused by SARS-CoV-2 was subsequently named coronavirus disease 2019 (COVID-19). Due to the rapid spread of SARS-CoV-2, WHO declared a new pandemic in March 2020. In March 2023, there were 680 million reported cases with 6.8 million deaths, thus being the most significant pandemic of the 21st century (Dorsett, 2020; Bigoni et al., 2022; Cascella et al., 2023).
Much of what has been observed in the scientific efforts and advances for the new pandemic coronavirus was not observed during the last decades for a considerable number of viral outbreaks of NTD occurring each year in tropical and subtropical regions (Wilder-Smith et al., 2017). Over 500 arboviruses have been identified, with 150 viruses being known to cause human disease (Young, 2018), and belonging to families such as Flaviviridae (genus Flavivirus) and Togaviridae (genus Alphavirus), including the order of Bunyaviridae (families of Nairoviridae, Peribunyaviridae and Phenuiridae) (Simmonds et al., 2017; Chen et al., 2018; Young, 2018; Abudurexiti et al., 2019). Most of those outbreaks are related to arboviruses which are cyclically transmitted to a mammalian host through the bite of hematophagous insects (Go et al., 2014; Weaver et al., 2018; Young, 2018). The geographic distribution of these arboviruses is restricted to the ecological parameters related to their transmission cycles (Gubler, 2001; Tajudeen et al., 2022), but a considerable increase of cases was observed in endemic regions as most countries are middle-income ones and have low investments in research (Tajudeen et al., 2022).
The genera Flavivirus and Alphavirus are arboviruses most known for causing disabling fever syndromes worldwide, which significantly burden worldwide public health and the global economy as well (Go et al., 2014). Several studies highlight the importance of DENV as the most prevalent arbovirus disease, which can be found in the co-circulation of multiple serotypes (i.e., hyperendemic) in several tropical countries of South America, Asia and Africa. In fact, CHIKV and ZIKV have recently expanded their geographical boundaries into the New World (Marcondes & Ximenes, 2016; Rodrigues Faria et al., 2016). In the last decade, South America and Caribbean regions reported endemic infections of CHIKV (2013–2014) (Rodrigues Faria et al., 2016) and epidemic infections of ZIKV (2015–2016) (Marcondes & Ximenes, 2016). Additionally, sporadic outbreaks of Mayaro virus (MAYV) were reported in rural areas of Central and South America (Figueiredo & Figueiredo, 2014; Acosta-Ampudia et al., 2018), including a recently reported re-emergence of yellow fever virus (YFV) in Brazil (2016–2019) (Cunhados et al., 2019).
Other studies have pointed out the possibility of the geographical spread of West Nile virus (WNV), Japanese encephalitis virus (JEV) (Simon-Loriere et al., 2017), and Rift Valley fever virus (RVFV) (Freire et al., 2015), as well as several human cases of Saint Louis encephalitis virus (SLEV) (Heinen et al., 2015) and Oropouche virus (OROV) (Mourãão et al., 2009). Unfortunately, in contrast to SARS-CoV-2, a few licensed vaccines are available, and still today, there is no specific antiviral drug to treat infections caused by arboviruses. Figure 1 highlights the global spread of emerging and re-emerging arboviruses in the 21st century (Figure 1A) and lists the number of reported cases in the world (Figure 1B) and in America (Figure 1C).
FIGURE 1. Global burden of Flavivirus and Alphavirus infections (A) Map showing arbovirus-reported outbreaks in the 21st century (B) The number of cases of some arbovirus diseases worldwide (data provided from WHO) (C) The number of cases of some arbovirus diseases in America (data provided from PAHO). CCHFV: Crimean-Congo hemorrhagic fever virus. CHIKV: Chikungunya virus. DENV: Dengue virus. JEV: Japanese encephalitis virus. KFDV: Kyasanur forest disease virus. RVFV: Rift Valley fever virus. YFV: Yellow fever virus. ZIKV: Zika virus.
Flaviviruses comprise the epidemic viruses DENV, ZIKV, WNV, YFV, and JEV (Simon-Loriere et al., 2017), all belonging to a diverse viral genus with close phylogenetic relationships and similar biological cycles (i.e., virus-vector-host interaction). The viral particles of Flaviviruses are enveloped and share an icosahedral structure of 40–60 nm (Zhang, 2003; Mackenzie, 2005). These viruses have a genome size of approximately 11 kb, which encodes a single polyprotein by its ORF and is flanked by two non-coding regions (5′and 3′) (Chambers et al., 1990). The polypeptide consists of approximately 3,400 amino acids, which are further cleaved into three structural proteins, namely, capsid protein (C), precursor membrane protein (prM) and envelope protein (E), and into seven non-structural proteins (NS1-NS5) (Chambers et al., 1990).
Togaviridae is a smaller family comprising only one genus, Alphavirus, and also includes the epidemic viruses CHIKV and MAYV (Chen et al., 2018). This genus comprises a small group of viruses with diversity in molecular and antigenic classification (Strauss & Strauss, 1994). Alphaviruses are enveloped viruses with a diameter ranging from 65–70 nm and an icosahedral capsid. Their genome is approximately 10–12 kb in length (Azar et al., 2020) and has an architecture organized into two ORFs. The first ORF encodes for four non-structural proteins (nsPs) accounting for the replication of the virus’ genetic material through the formation of RNA replicase complexes, which act in the evasion of the immune responses. The genes of the second ORF encode for structural proteins named C (capsid), E1 and E2 (envelope glycoproteins 1 and 2) as well as E3 and 6 K peptides (Kril et al., 2021).
During the SARS-CoV-2 pandemic, unprecedented efforts and studies performed by the international scientific community and health agencies generated public knowledge on SARS-CoV- 2. Consequently, numerous sequenced viral genomes became available in public databases, such as GenBank and GISAID. In fact, more than 14 million genetic sequence data (GSD) are deposited in the GISAID database (Khare et al., 2021), and this number continues to increase. Analysis of GSD was performed almost in real-time, which has profoundly affected the management of the SARS-CoV-2 pandemic. Additionally, it made it possible to generate epidemiological (Faria et al., 2021; Naveca et al., 2022), genetic, and phylogenetic studies (Cunhados et al., 2019; Nunes D. A. F. et al., 2022), with enhancing precision to provide a wide range of information, which in turn enabled more effective actions to face the pandemic (Chen et al., 2022). In such a scenario, the importance of WHO was also highlighted as a reference center providing a genomic guide to help laboratories worldwide carry out DNA sequencing given the extreme relevance of these data and possible impact on public health (Jacot et al., 2021; Brito et al., 2022).
Currently, the genomic databases of SARS-CoV-2 GSD can be classified into public-domain and public-access databases, which differ from each other in terms of access restrictions. In the public-domain database, GSD can be accessed without registration or identification (Cochrane et al., 2016). On the other hand, identification is needed to access a public-access database, such as GISAID, and manipulate GSD in order to supervise the use of information. Moreover, it is possible to credit researchers who contributed by depositing GSD into databases (Elbe & Buckland-Merrett, 2017; Shu & McCauley, 2017; Shu & McCauley, 2017; Khare et al., 2021).
Analysis of GSD made it possible to infer that SARS-CoV-2 originated from a zoonotic source, like most viruses affecting humans (Holmes et al., 2021), and similarly to SARS-CoV, had its pandemic epicenter traced to a wild animal market (Guan et al., 2003). However, despite all efforts, the specific intermediate host of SARS-CoV-2 still needs to be determined. Its genetic proximity to the bat SARS-CoV-like coronavirus (BatCoVRaTG13), with a nucleotide similarity of ∼96%, is still the closest one to that of zoonotic coronaviruses reported in the first outbreak region (Paraskevis et al., 2020). Nevertheless, despite the high percentage of genetic similarity between SARS-CoV2 and RaTG13, there is a divergence in part of the genome encoding for the receptor-binding domain (RBD), which is an essential role in the connection between spike protein and angiotensin-converting enzyme 2 (ACE2), the receptor of human cells where the virus binds (Andersen et al., 2020).
Many studies have suggested that pangolins (Manis javanica) are possible intermediate hosts of SARS-CoV-2 because of the high sequence similarity with the viral RBD (∼97.4%) found in coronaviruses isolated from these animals (Zhang et al., 2003; Lam et al., 2012; Zhou et al., 2020; Malik et al., 2023), implying a possible origin of SARS-CoV-2 through recombination (Wong et al., 2020). However, the hypothesis of homoplasy, which suggests that similarities between SARS-CoV-2 and other SARS-CoV-2-like coronaviruses have arisen independently through convergent evolution rather than ancestry, has yet to be fully discarded (Tang et al., 2020).
SARS-CoV-2 has an evolutionary rate of 1.15 × 10−3 substitutions/site/year, which differs slightly from the substitution rates of other highly pathogenic hCoVs, such as SARS-CoV (0.80–2.38 × 10−3) and MERS-CoV (0.63–1.12 × 10−3). However, the two viral strains of SARS-CoV-2, A and B lineages, left China and diversified into several new variants, possibly due to deletion events (Candido et al., 2020; Tang et al., 2020; Rambaut et al., 2021; McCarthy et al., 2021). SARS-CoV-2 variants have been identified and named in different ways by GISAID, Nextstrain and Pango (Alm et al., 2020). As a standardization attempt, in May 2021, WHO suggested a new nomenclature based on the Greek alphabet, which covers some of the main variants and has been extensively used ever since (Papanikolaou et al., 2022). Among the new variants, the most worrying ones have been classified by the Centers for Disease Control and Prevention (CDC) as well as by WHO based on their mutational characteristics, transmissibility, pathogenicity, and ability to evade the immune system, the so-called variants of concern (VOC) (CDC, 2021).
In December 2022, the latest emerging VOC was named Omicron, which appeared in South Africa (Kirola, 2021; Tegally et al., 2022) and spread worldwide (Mohapatra et al., 2022). Evidence of an increased transmission of Omicron variant, including risk or mortality, pointed to mutations in RBD and nucleocapsid (N) regions (Andreano et al., 2020; Plante et al., 2020; Tegally et al., 2020; Xie et al., 2021; Andreata-Santos et al., 2022). The Omicron-related strains (BA.2, BA.4 and BA.5) carry numerous mutations, particularly in the S gene, which encodes for spike protein (Hussain et al., 2020; Wu et al., 2020; Zhou et al., 2020; Hanifa et al., 2022; Papanikolaou et al., 2022; Takashita et al., 2022; Tegally et al., 2022). Although this is already well-established in the literature, the mechanisms underlying these mutations have not been fully characterized. It is established, however, that directional positive selection pressure drives the accumulation of mutations in the SARS-CoV-2 genome, resulting in more non-synonymous mutations affecting the virus’ adaptability (Martin et al., 2021; Andreata-Santos et al., 2022; Nunes D. R. et al., 2022). The spike protein plays a crucial role in the virus tropism and its entry into host cells by binding to the ACE2 receptor, thus also being the most immunogenic viral protein (Li 2016; Hoffmann et al., 2020).
As a result, the spike protein has been used as the central target region in many vaccines and serological tests (Galipeau et al., 2020; Sandbrink & Shattock, 2020; Infantino et al., 2021; Kyriakidis et al., 2021). Thus, the emergence of new variants carrying mutations throughout the genome, but with greater intensity in the spike region, has raised the question of how it would impact the efficiency and effectiveness of vaccines and serological tests. This issue is still being carefully evaluated.
Tested therapeutic options are available to date for SARS-CoV-2, including antiviral drugs. The National Institutes for Health (NIH) in the United States has frequently published guidelines for treating COVID-19, including a classification of drugs for use by different individuals as authorized by the Food and Drug Administration (FDA). Based on a wide range of clinical manifestations and considering the patient’s age, some medicines were recommended, such as ritonavir-boosted nirmatrelvir (Paxlovid), remdesivir, molnupiravir and bebtelovimab (Murakami et al., 2022; Niknam et al., 2022).
One of the most evident lessons from the COVID-19 pandemic is the extensive and rapid antiviral drug repurposing by using high-throughput screening methods with numerous research groups (Chen, 2020; Rodon et al., 2021; Hamed et al., 2022). The RNA-dependent RNA polymerase (RdRp) enzyme is one of the main therapeutic targets of viral drugs (Wang et al., 2020). Two drugs aimed at RdRp are currently used to control SARS-CoV-2 infection, namely, remdesivir and molnupiravir (Kabinger et al., 2021). As the first drug to be approved for the treatment of COVID-19, remdesivir was also endorsed for children’s treatment (Young, 2021; Chera & Tanca, 2022), whereas the above-mentioned drugs are only authorized for emergency use (Atluri et al., 2022). Remdesivir is a nucleotide analogue which prevents the synthesis of viral RNA in coronavirus infection when incorporated as a substrate by RdRp (Gordon et al., 2020; Kokic et al., 2021).
As a result of a broad collaborative effort, the US Food and Drug Administration (FDA) approved the brand-name drug of Veklury (remdesivir) on 22 October 2020, as being the first drug for the treatment of COVID-19 (Al-Ardhi et al., 2022; Niknam et al., 2022). Despite its approval, there are controversies regarding its efficacy. The Solidarity Therapeutics Trial, conducted by WHO, showed no improvement in the overall mortality rate of hospitalized COVID-19 patients treated with remdesivir compared to standard care. In contrast, another study on adaptive COVID-19 treatment trial (ACTT-1), sponsored by the National Institute of Allergy and Infectious Diseases, demonstrated that remdesivir reduced recovery time by 5 days in hospitalized COVID-19 patients compared to standard care, but did not significantly reduce mortality (Beigel et al., 2020). Thus, the use of remdesivir for COVID-19 treatment remains controversial, but it has undoubtedly played an essential role in the fight against the pandemic (Okoli et al., 2021).
As for molnupiravir, a slightly different mechanism of action is observed. Molnupiravir is first converted into β-D-N4-hydroxycytidine ribonucleoside and subsequently into ribonucleoside triphosphate (NHC-TP) through the catalytic activity of the enzyme kinase. When incorporated by RNA polymerase, NHC-TP leads to a significantly increased number of errors in the viral genetic material, thus generating an erroneous mechanism (Donovan-Banfield et al., 2022), and consequently making viral replication unfeasible (Agostini et al., 2019; Kabinger et al., 2021; Kamal et al., 2022).
Other antiviral drugs have been used to inhibit viral proteases, thereby interfering with the SARS-CoV-2 replication cycle. For example, Paxlovid inhibits viral proteases, which are crucial for the replicative cycle of SARS-CoV-2 (Cannalire et al., 2022;; Marzi et al., 2022), by combining the action of two drugs: Nirmatrelvir and Ritonavir. Nirmatrelvir is a newly developed drug by Pfizer which can bind to the viral protease and prevent its catalytic activity. On the other hand, Ritonavir functions by slowing down the metabolism of Nirmatrelvir to increase its efficiency (Ahmad et al., 2021; Marzi et al., 2022).
Other available treatments for COVID-19 are the anti-SARS-CoV-2 monoclonal antibodies, which can be used depending on the severity and risk factors. Tixagevimab and cilgavimab (AZD7442), two human monoclonal antibodies (mAbs), have been designated for complement blockade of SARS-CoV-2 infection by inhibiting the attachment of the SARS-CoV-2 spike protein. These antibodies have the ability to prevent the virus from attaching to the cell surface receptor (ACE2) by binding to two distinct regions of the spike protein, namely, tixagevimab, which recognizes the receptor-binding domain (RBD) and cilgavimab, which targets the N-terminal domain (NTD) (Stuver et al., 2022; Focosi et al., 2023). FDA approved this pre-exposure prophylactic drug in October 2022, given the continuous emergence of new SARS-CoV-2 variants. After previously being tested in a phase III double-blind trial involving 5,197 participants during the emergence of VOCs, the promise of reducing the incidence of symptomatic COVID-19 was demonstrated (Kertes et al., 2023).
AZD7442 was originally indicated for individuals who did not have an adequate immune response or who had contraindications to vaccination (Akinosoglou et al., 2022). However, the neutralizing ability as a consequence of escape mutations acquired by the new SARS-CoV-2 variants led to its removal from the recommended drugs by the FDA (Focosi et al., 2023; Suribhatla et al., 2023).
Despite the importance of the epidemics of arboviruses regarding severe illnesses, no specific drugs or therapies are currently approved for their treatment. However, some strategies in the development and reposition of drugs for the treatment of SARS-CoV-2 can be used for arboviral infections. These main strategies can be classified into the following categories: antiviral drugs, vaccine development and gene therapies (Idrees & Ashfaq, 2013; Bishop, 2015; Gao et al., 2019; Poland et al., 2019; Dong & Dimopoulos, 2021).
Viral protease inhibitors represent a promising class of drugs for controlling and treating arboviral infections. Drugs such as atazanavir, boceprevir, and lopinavir/ritonavir can inhibit viral protease activity, thus preventing the cleavage of proteins essential for maturation of virions, leading to a significant reduction in viral load (Anderson et al., 2009). These inhibitors have a well-established history of use against human immunodeficiency virus (HIV) and hepatitis C virus (HCV), being further approved for treating SARS-CoV-2 infections, although only ritonavir and Paxlovid (nirmatrelvir and ritonavir) remain in use by FDA (11 May 2023) (Lv et al., 2015; Wang et al., 2021; Narayanan et al., 2022).
In recent years, much information on the structure and function of proteins has been generated, such as NS2, NS3, and nsP3, which are essential proteases for cleavage and maturation of virions for many arboviruses belonging to the Flavivirus and Alphavirus genera, respectively (Nitsche, 2019; Hucke & Bugert, 2020). Thanks to these collaborative efforts, it has been possible not only to design molecules capable of inhibiting the proteolytic activity of these enzymes (Ivanova et al., 2021;; Nunes D. A. F. et al., 2022), but also to investigate drug replacement for arboviruses (Ding et al., 2022).
Indeed, the use of nelfinavir as an inhibitor of HIV and HCV proteases in the replication of DENV and CHIKV has been previously described. In the study by Bhakat et al., the drugs used as inhibitors of HIV and HCV proteases were screened based on their structural similarity to compounds of interest. Although the transition from in silico to in-vivo studies revealed promising initial results, such inhibition was accompanied by high levels of toxicity (Bhakat et al., 2015; Boldescu et al., 2017).
A more recent screening of FDA-approved drugs identified telmisartan and novobiocin as promising candidates, and they were used as protease inhibitors against chikungunya virus. Telmisartan is an antihypertensive drug aimed at blocking the angiotensin receptor, whereas novobiocin is an approved antibiotic aimed at inhibiting the ATPase activity of bacterial DNA gyrase. By using molecular modeling was demonstrated that these drugs interact with the chikungunya virus nsP2 protein and confirmed in vitro their inhibitory activity (Figure 2) (Tripathi et al., 2020). Although these findings demonstrate the potential of drug replacement strategies and exploration of protease inhibitors for possible treatments for arboviruses, no protease inhibitor has been approved yet for these viruses.
FIGURE 2. Structural representation of CHIKV nsP2 bound to the protease inhibitor. CHIKV nsP2 protein (cyan cartoon) highlighting the active site of novobiocin (green stick). The figures of protein structure were made with the PyMOL Molecular Graphics System, Version 2.0 Schrödinger, LLC.
Another promising drug class for antiviral therapy is the nucleoside/nucleotide analogs, with remdesivir being the most recent example (Kokic et al., 2021). Sofosbuvir was the first drug of this class and was initially developed for the treatment of HCV. It is orally administered and consists of a uridine molecule connected to a phosphoramidite group through an ester bond. After absorption, sofosbuvir undergoes hepatic metabolism to be converted into an active form through hydrolysis and phosphorylation (Figure 3A). This active form is a nucleotide analog aimed at inhibiting the HCV RNA-dependent RNA polymerase NS5B, ultimately blocking the viral RNA synthesis (Figure 3B) (Bhatia et al., 2014; Keating, 2014).
FIGURE 3. Structural representation of Flavivirus (HCV, DENV) proteins, highlighting the site of binding of select drugs that illustrate one of the most effective strategies against arboviruses (A) HCV NS5-RNA-dependent-RNA-polymerase domain (teal cartoon) structure interacting with sofosbuvir (multicolored stick), depicted in greater detail in (B) highlighting critical amino acid residues (K469, R486, D553) forming polar contacts with sofosbuvir, as well as the G681 residue which is the starting of GDD motif, the drug’s active site (C) Amino acid sequence alignment of NS5 region from flaviviruses (WNV, JEV, ZIKV, DENV2, YEV, TBEV, and HCV) highlighting some of the most conserved protein regions, including the GDD motif and sites of polar contacts with sofosbuvir/NS5 (D) Structural overlay of NS5 from HCV (palecyan cartoon) and DENV (teal cyan cartoon) with remdesivir (multicolored stick) (E) Detail of structural similarity between DENV and HCV virus proteins. (F) CHIKV nsP2 protein (cyan cartoon) highlighting the active site of novobiocin (green stick).
Indeed, the GDD (Gly-Asp-Asp) motif is a highly conserved site found in flaviviruses and which has been identified as the binding site for sofosbuvir (Figure 3C) (Yap et al., 2007). This drug has shown success in both in vitro studies with dengue virus and clinical trials with yellow fever virus, thus highlighting its potential for therapeutic applications (Figure 3D) (Gan et al., 2018; Mendes et al., 2019; Siqueira-Batista et al., 2019).
This class of drug was quickly recognized as a promising tool against epidemic viruses, and the subsequent refining of the most efficient nucleotides led to the identification of remdesivir, later described as the most effective drug against emerging viruses such as SARS-CoV, MERS-CoV and Ebola virus (Malin et al., 2020). As for SARS-CoV-2, this strategy has also shown promising results against arboviruses, as already demonstrated in preclinical studies on remdesivir and molnupiravir (Konkolova et al., 2020; Chera & Tanca, 2022; Extance, 2022; Vangeel et al., 2022). However, further studies are required to establish these drugs’ efficacy in treating patients with arboviral infections.
In recent years there has been a marked shift regarding vaccines, treatments and control of arbovirus-borne diseases, as demonstrated by the launch of the Global Arbovirus Initiative (Simpson, 1972; Rocha et al., 2018; Balakrishnan, 2022), due, in part, to the growing emergence of these viruses. Arboviruses are responsible for roughly 30% of all emerging infectious diseases (Jones et al., 2008; Soldan & González-Scarano, 2014). Despite their significance, there are still limited options of vaccines or effective antiviral therapies for most arboviruses, including flavivirus and alphavirus genera (Carvalho & Long, 2021).
Only four arbovirus vaccines have been currently approved by WHO and FDA for use, namely, dengue virus vaccine (Tully & Griffiths, 2021), Japanese encephalitis virus vaccine (Amicizia et al., 2018), yellow fever virus vaccine (Gordon Frierson, 2010), and tick-borne encephalitis virus vaccine (Wikel, 2018; Nygren et al., 2022). The available vaccines can be categorized into inactivated (TBEV) and live attenuated (YFV, JEV, DENV) ones. The latter category may use chimeric viruses, as demonstrated by the DENGVAXIA vaccine for DENV and one of the three vaccines available for JEV (Collins & Metz, 2017). However, the COVID-19 pandemic has presented the opportunity to use a new technology (Klein, 2022), which has been under development over the last 30 years, the so-called mRNA vaccines (Verbeke et al., 2019). The mRNA-based vaccines are usually encapsulated by a lipid particle to allow the delivery of coding RNA into the cytoplasm, where the ribosomes will translate it into a protein. As demonstrated in the case of SARS-CoV-2, mRNA vaccines elicit both humoral and cellular responses. Host cells express viral proteins which are recognized by B-cell-produced antibodies, thus preventing viral entry into the cell. Furthermore, these vaccines may also induce robust CD8+ T-cell-based immune responses involving apoptosis in the infected cells (Pardi et al., 2018; Rijkers et al., 2021). There is also the possibility of modifying the mRNA to increase stability (Sahin et al., 2014) or prevent immune response to the exogenous RNA molecule, as in the case of the SARS-CoV-2 Pfizer-BioTech vaccine, where uridine was modified into a pseudo-uridine (Dolgin, 2021).
This type of vaccine has several benefits, such as excellent safety, better stability and the possibility of increased absorption. Indeed, this platform allows vaccines to be optimally developed and produced at a shorter time than any other platform (Pardi et al., 2018; Chaudhary et al., 2021; Dolgin, 2021). The mRNA vaccines have immense potential for use in combating NTD. This technology is already being applied in preclinical studies for vaccines against several arboviruses, including Zika, chikungunya and dengue viruses (Chaudhary et al., 2021; Wollner et al., 2021; Ge et al., 2022). Moderna has developed an mRNA sequence by using the mRNA platform which encodes a membrane protein fused to the envelope protein (prM-E) (Richner et al., 2017). The mRNA sequence encoding the prM-E protein is an immunogenic strategy successfully explored for fighting ZIKV, thus demonstrating the induction of high levels of specific and neutralizing antibodies (Larocca et al., 2016; Pardi et al., 2017; Richner et al., 2017).
Surface proteins, such as spike of SARS-CoV-2, E2-E1 of chikungunya and prM-E of Zika and dengue viruses, are commonly chosen as targets for mRNA vaccines not only because they are the surface protein allowing the entry of the virus, but also because they elicit a robust immune response. Proteins E and prM are the two main targets of monoclonal antibodies in Flaviviruses (Rey et al., 2018; Slon-Campos et al., 2018; Chaudhary et al., 2021; Wollner et al., 2021; Ge et al., 2022).
The RNA interference (RNAi) is a cellular mechanism present in several eukaryotic organisms aimed at decreasing the expression of specific genes by the use of molds of small non-coding RNAs (sncRNAs) with approximately 20–30 nucleotides as a template complementary to mRNA. This pathway is specific and conserved between species and, in theory, can be used to silence the expression of any gene of interest (Agrawal et al., 2003). Physiologically, RNAi acts in biological processes such as cell development and differentiation, and in some animals (e.g., mosquitoes), it participates in the immune response against viral genetic material (Siomi & Siomi, 2009; Wang, 2021). In fact, RNAi has been studied as a therapeutic platform for treating various genetic diseases, cancers and antiviral therapy (Sidahmed et al., 2014; Kristen et al., 2019; Kara et al., 2022). These molecules can be quickly designed, synthesized and tested in several in vitro and in vivo trials (Agarwal et al., 2022).
By recognizing specific sequences of the viral genome, these molecules can prevent the replication of the virus and the translation of viral proteins without affecting the host’s gene expression (Ketzinel-Gilad et al., 2006). Considering their therapeutic applications, three types of RNAi stand out, namely, microRNAs (miRNAs), small interfering RNAs (siRNAs) and short hairpin RNAs (shRNAs) (Agarwal et al., 2022). Although the miRNA pathway occurs through the expression and processing of non-coding regions of the cell’s genome, the siRNA pathway relies on detecting double-stranded RNAs inside the cell. There are currently several tools and techniques allowing the design and insertion of artificial RNAs (e.g., amiRNAs and siRNAs) into the cell environment (Agarwal et al., 2022). Also, shRNAs are synthetically designed and delivered as expression plasmids or bacterial/viral vectors.
COVID-19 pandemic has made coronaviruses the most urgent targets for developing antiviral drugs, including exploring the RNAi platform. This methodology was used in vivo and in vitro to prevent SARS-CoV-2 dissemination (Table 2) (Donia & Bokhari, 2021; Talukder & Chanda, 2021). Studies with the development of RNAi directed against the 5′UTR region of the viral genome (Idris, 2021; Tolksdorf et al., 2021), including other genome regions such as RNA polymerase (RdRp) and Helicase (Hel) proteins (Idris, 2021) were rapidly conducted (Table 2). They reported a reduction in viral loads in vitro and in vivo, showing that this technique can be a good strategy for arbovirus infections and considering the complex host-pathogen and vector-pathogen interactions (Gubler, 2002). Several in vitro and in vivo studies with different flavivirus and alphavirus genera (Table 2) have demonstrated a significant viral load reduction by several RNAi designed against non-structural and structural protein genome regions (Pacca et al., 2009; Parashar et al., 2013; Haikerwal, 2022) or even terminal genome regions (Stein et al., 2011). These studies develop and test approximately 6–10 RNAi aimed at various genome locations, with 2-3 molecules having a significant inhibitory activity (Table 2) ranging from 80%–90%. A common point in many of these studies is the use of tests combining siRNAs, which showed an additive effect on the inhibition of replication of different viral strains (O’Brien, 2007).
Nowadays, no clinical trials use RNAi against SARS-CoV2 or flavivirus and alphavirus genera. However, the use of this technology in treating other emerging viruses, such as HIV and EBOV, has succeeded in different stages of clinical trials, demonstrating its potential in treating viral diseases (Setten et al., 2019). Although there is still much to be done in the development of these therapeutic approaches, mainly regarding cost and time of action of the molecules in the organism (which can also influence the price of the treatment), the promising results presented by these studies show that this new therapeutic approach is excellent in the treatment of these diseases.
Herein, we reviewed the literature regarding the research for prophylaxis and treatment development for SARS-CoV-2 and Neglected Tropical Diseases (NTD), focusing mainly on arboviruses (Figure 4). Efforts to combat the SARS-CoV-2 pandemic have opened new perspectives regarding strategies that can be used to expand the treatment, therapy, and immunization for other RNA viruses. The review also indicates which approaches were used and currently appear to be the most promising ones—for example, inhibitory replication drugs like nucleoside analogs and protease inhibitors. Moreover, developing mRNA vaccines against the SARS-CoV-2 virus demonstrates enormous efficacy and great vaccine candidates. Since few vaccines can suppress most epidemic arboviruses, this would be an excellent strategy as it has already started on clinical trials for zika, chikungunya, and dengue viruses. Also, the recent design and development of new therapy using RNAi for the most critical arboviruses was reviewed, some of it within promising results targeting distinct flavivirus and alphavirus RNA conserved regions. Ultimately, antiviral treatments and vaccines are crucial for improving public health and preventing future arbovirus epidemics in tropical and subtropical areas.
FIGURE 4. Schematic representation of genome organization of Flavivirus (A) and Alphavirus (B), and summary of new therapeutic approaches against Flavivirus and Alphavirus diseases (C). The viral genome of Flavivirus consists of an open reading frame (ORF) between two untranslated regions (UTR). From the ORF, all viral proteins are generated, which are non-structural (NS1-5) and structural (C, M, and E) proteins. The viral genome of Alphavirus consists of two ORFs separated by a subgenomic promoter (SP). From the first ORF, the non-structural proteins (nsP1-4) are generated, while the structural proteins (C, E1 and E2) are generated by the second ORF. In addition, a summary of viral infection cycle of Flaviviruses and Alphaviruses in eukaryotic cells is represented. The first step involves the virion particles containing structural proteins and RNA genomic material. The box highlights the possibility of blocking viral entrance by using neutralizing IgM and IgG antibodies induced by mRNA vaccines. The second step involves the release of viral genome traffic from early endosomes. Next, RNA replication can be blocked either by using analogue nucleoside, the growing such as remdesevir and sofosbovir, to be incorporated by the RNA-dependent RNA polymerase (RdRp) enzyme into which is then incorporated into the growing RNA strand or using RNA interference (RNAi) specific for target locations of the viral genome, leading to genome degradation. The following steps, such as translation, can be inhibited by blocking both structure and function of nonstructural proteins, which are essential proteases for virion cleavage and maturation. Examples of such protease inhibitors include novobicin and telmisartan. Created with Biorender.com.
DRN and CTB conceptualized the work. DRN and DBML performed the graphical analysis, Table and Figures. DRN, DBML, RAS, LMRJ and CTB wrote the manuscript and corrected the final version. All authors have read, edited and agreed to the published version of the manuscript.
This work was supported by the São Paulo Research Foundation (FAPESP) according to grant numbers 2020/089435 (CB and LJ) and 2021/05661-1 (RA-S), the National Council for Scientific and Technological Development (CNPq) according to grant number 405691/2018-1 (CB) and Coordination of Improvement of Higher Education Personnel (CAPES) according to grant numbers 8888.506234/2020-00 (DR-N) and 88887.822311/2023-00 (DL). The funders had no role in study design, data collection and analysis, decision to publish the manuscript or preparation of the manuscript.
We would like to thank Beatriz Amaral de Castilho and Beatriz Ernestina Cabilio Guth (Department of Microbiology, Immunology and Parasitology of the Federal University of São Paulo School of Medicine (UNIFESP) for kindly donating supplies and equipment for the Laboratory of Arbovirus Research (LARCH) as well as Fernando M. Antonelli for his computing support. The authors would also like to thank FAPESP and CNPq for their financial support.
The authors declare that the research was conducted in the absence of any commercial or financial relationships that could be construed as a potential conflict of interest.
All claims expressed in this article are solely those of the authors and do not necessarily represent those of their affiliated organizations, or those of the publisher, the editors and the reviewers. Any product that may be evaluated in this article, or claim that may be made by its manufacturer, is not guaranteed or endorsed by the publisher.
Aaron, L., McMahon, J., Taylor, C., Pyke, A. T., Brischetto, A., Aminzadeh, Z., et al. (2022). Locally acquired lymphocytic choriomeningitis virus infections in South-East queensland: An outbreak of a pathogen rarely described in Australia. Intern. Med. J. 52 (8), 1415–1418. doi:10.1111/imj.15878
Abudurexiti, A., Adkins, S., Alioto, D., Alkhovsky, S. V., Avšič-Županc, T., Ballinger, M. J., et al. (2019). Taxonomy of the order bunyavirales: Update 2019. Archives virology 164 (7), 1949–1965. doi:10.1007/s00705-019-04253-6
Acosta-Ampudia, Y., Monsalve, D. M., Rodríguez, Y., Pacheco, Y., Anaya, J. M., and Ramírez-Santana, C. (2018). Mayaro: An emerging viral threat? Emerg. microbes Infect. 7 (1), 163. doi:10.1038/s41426-018-0163-5
Agarwal, A., Sarma, D. K., Chaurasia, D., and Maan, H. S. (2022). Novel molecular approaches to combat vectors and vector-borne viruses: Special focus on RNA interference (RNAi) mechanisms. Acta trop. 233, 106539. doi:10.1016/j.actatropica.2022.106539
Agostini, M. L., Pruijssers, A. J., Chappell, J. D., Gribble, J., Lu, X., Andres, E. L., et al. (2019). Small-molecule antiviral β-d-N4-hydroxycytidine inhibits a proofreading-intact coronavirus with a high genetic barrier to resistance. J. virology 93 (24), 013488–e1419. doi:10.1128/JVI.01348-19
Agrawal, N., Dasaradhi, P. V. N., Mohmmed, A., Malhotra, P., K Bhatnagar, R., and Mukherjee, S. K. (2003). RNA interference: Biology, mechanism, and applications. Microbiol. Mol. Biol. Rev. 67, 657–685. doi:10.1128/mmbr.67.4.657-685.2003
Ahlquist, P., Noueiry, A. O., Lee, W. M., Kushner, D. B., and Dye, B. T. (2003). Host factors in positive-strand RNA virus genome replication. J. virology 77 (15), 8181–8186. doi:10.1128/jvi.77.15.8181-8186.2003
Ahmad, B., Batool, M., Ain, Q. U., Kim, M. S., and Choi, S. (2021). Exploring the binding mechanism of PF-07321332 SARS-CoV-2 protease inhibitor through molecular dynamics and binding free energy simulations. Int. J. Mol. Sci. 22 (17), 9124. doi:10.3390/ijms22179124
Ahmad, T. (2022). Global research trends in MERS-CoV: A comprehensive bibliometric analysis from 2012 to 2021. Front. public health 10, 933333. doi:10.3389/fpubh.2022.933333
Akinosoglou, K., Rigopoulos, E. A., Kaiafa, G., Daios, S., Karlafti, E., Ztriva, E., et al. (2022). Tixagevimab/cilgavimab in SARS-CoV-2 prophylaxis and therapy: A comprehensive review of clinical experience. Viruses 15 (1), 118. doi:10.3390/v15010118
Al-Ardhi, F. M., Novotny, L., Alhunayan, A., and Al-Tannak, N. F. (2022). Comparison of remdesivir and favipiravir - the anti-Covid-19 agents mimicking purine RNA constituents. Biomed. Pap. Med. Fac. Univ. Palacky 166 (1), 12–20. doi:10.5507/bp.2021.063
Allen, T., Murray, K. A., Zambrana-Torrelio, C., Morse, S. S., Rondinini, C., Di Marco, M., et al. (2017). Global hotspots and correlates of emerging zoonotic diseases. Nat. Commun. 8 (1), 1124. doi:10.1038/s41467-017-00923-8
Alm, E., Broberg, E. K., Connor, T., Hodcroft, E. B., Komissarov, A. B., Maurer-Stroh, S., et al. (2020). Geographical and temporal distribution of SARS-CoV-2 clades in the WHO European region, january to june 2020. Eurosurveillance 25 (32), 2001410. doi:10.2807/1560-7917.ES.2020.25.32.2001410
Alyami, M. H., Alyami, H. S., and Warraich, A. (2020). Middle East Respiratory Syndrome (MERS) and novel coronavirus disease-2019 (COVID-19): From causes to preventions in Saudi Arabia. Saudi Pharm. J. SPJ 28 (11), 1481–1491. doi:10.1016/j.jsps.2020.09.014
Amicizia, D., Zangrillo, F., Lai, P. L., Iovine, M., and Panatto, D. (2018). Overview of Japanese encephalitis disease and its prevention. Focus on IC51 vaccine (IXIARO®). J. Prev. Med. Hyg. 59 (1), E99–E107. doi:10.15167/2421-4248/jpmh2018.59.1.962
Anantpadma, M., and Vrati, S. (2012). siRNA-mediated suppression of Japanese encephalitis virus replication in cultured cells and mice. J. Antimicrob. Chemother. 67 (2), 444–451. doi:10.1093/jac/dkr487
Andersen, K. G., Rambaut, A., Lipkin, W. I., Holmes, E. C., and Garry, R. F. (2020). The proximal origin of SARS-CoV-2. Nat. Med. 26 (4), 450–452. doi:10.1038/s41591-020-0820-9
Anderson, J., Schiffer, C., Lee, S. K., and Swanstrom, R. (2009). Viral protease inhibitors. Antivir. Strateg. 189, 85–110. doi:10.1007/978-3-540-79086-0_4
Andreano, E., Piccini, G., Licastro, D., Casalino, L., Johnson, N. V., Paciello, I., et al. (2020). SARS-CoV-2 escape in vitro from a highly neutralizing COVID-19 convalescent plasma. Cold Spring Harb. Lab. 2020, 424451.
Andreata-Santos, R., Janini, L. M. R., and Durães-Carvalho, R. (2022). From Alpha to Omicron SARS-CoV-2 variants: What their evolutionary signatures can tell us? J. Med. virology 94 (5), 1773–1776. doi:10.1002/jmv.27555
Anthony, K. G., Bai, F., Krishnan, M. N., Fikrig, E., and Koski, R. A. (2009). Effective siRNA targeting of the 3’ untranslated region of the West Nile virus genome. Antivir. Res. 82 (3), 166–168. doi:10.1016/j.antiviral.2008.12.007
Araf, Y., Maliha, S. T., Zhai, J., and Zheng, C. (2023). Marburg virus outbreak in 2022: A public health concern. Lancet. Microbe 4 (1), e9. doi:10.1016/S2666-5247(22)00258-0
Aslan, A. T., and Balaban, H. Y. (2020). Hepatitis E virus: Epidemiology, diagnosis, clinical manifestations, and treatment. World J. gastroenterology WJG 26 (37), 5543–5560. doi:10.3748/wjg.v26.i37.5543
Atluri, K., Aimlin, I., and Arora, S. (2022). Current effective therapeutics in management of COVID-19. J. Clin. Med. Res. 11 (13), 3838. doi:10.3390/jcm11133838
Ayyagari, V. S. (2022). Design of siRNA molecules for silencing of membrane glycoprotein, nucleocapsid phosphoprotein, and surface glycoprotein genes of SARS-CoV2. J. Genet. Eng. Biotechnol. 20 (1), 65. doi:10.1186/s43141-022-00346-z
Azar, S. R., Campos, R. K., Bergren, N. A., Camargos, V. N., and Rossi, S. L. (2020). Epidemic alphaviruses: Ecology, emergence and outbreaks. Microorganisms 8 (8), 1167. doi:10.3390/microorganisms8081167
Bai, F., Wang, T., Pal, U., Bao, F., Gould, L. H., and Fikrig, E. (2005). Use of RNA interference to prevent lethal murine west nile virus infection. J. Infect. Dis. 191 (7), 1148–1154. doi:10.1086/428507
Baker, R. E., Mahmud, A. S., Miller, I. F., Rajeev, M., Rasambainarivo, F., Rice, B. L., et al. (2022). Infectious disease in an era of global change. Nat. Rev. Microbiol. 20 (4), 193–205. doi:10.1038/s41579-021-00639-z
Balakrishnan, V. S. (2022). WHO launches global initiative for arboviral diseases. Lancet. Microbe 3 (6), e407. doi:10.1016/S2666-5247(22)00130-6
Baltimore, D. (1971). Viral genetic systems. Trans. N. Y. Acad. Sci. 33 (3), 327–332. doi:10.1111/j.2164-0947.1971.tb02600.x
Becker, D. J., Washburne, A. D., Faust, C. L., Mordecai, E. A., and Plowright, R. K. (2019). The problem of scale in the prediction and management of pathogen spillover. Philosophical Trans. R. Soc. Lond. Ser. B, Biol. Sci. 374, 20190224. doi:10.1098/rstb.2019.0224
Becker-Dreps, S., González, F., and Bucardo, F. (2020). Sapovirus: An emerging cause of childhood diarrhea. Curr. Opin. Infect. Dis. 33 (5), 388–397. doi:10.1097/QCO.0000000000000671
Beer, E. M., and Rao, V. B. (2019). A systematic review of the epidemiology of human monkeypox outbreaks and implications for outbreak strategy. PLoS neglected Trop. Dis. 13 (10), e0007791. doi:10.1371/journal.pntd.0007791
Beigel, J. H., Tomashek, K. M., Dodd, L. E., Mehta, A. K., Zingman, B. S., Kalil, A. C., et al. (2020). Remdesivir for the treatment of covid-19 - final report. N. Engl. J. Med. 383 (19), 1813–1826. doi:10.1056/NEJMoa2007764
Bellomo, C., Alonso, D. O., Ricardo, T., Coelho, R., Kehl, S., Periolo, N., et al. (2021). Emerging hantaviruses in Central Argentina: First case of Hantavirus Pulmonary Syndrome caused by Alto Paraguay virus, and a novel orthohantavirus in Scapteromys aquaticus rodent. PLoS neglected Trop. Dis. 15 (11), e0009842. doi:10.1371/journal.pntd.0009842
Bernstein, A. S., Ando, A. W., Loch-Temzelides, T., Vale, M. M., Li, B. V., Li, H., et al. (2022). The costs and benefits of primary prevention of zoonotic pandemics. Sci. Adv. 8 (5), eabl4183. doi:10.1126/sciadv.abl4183
Bhakat, S., Delang, L., Kaptein, S., Neyts, J., Leyssen, P., and Jayaprakash, V. (2015). Reaching beyond HIV/HCV: Nelfinavir as a potential starting point for broad-spectrum protease inhibitors against dengue and chikungunya virus. RSC Adv. 5, 85938–85949. doi:10.1039/c5ra14469h
Bhatia, H. K., Singh, H., Grewal, N., and Natt, N. K. (2014). Sofosbuvir: A novel treatment option for chronic hepatitis C infection. J. Pharmacol. Pharmacother. 5 (4), 278–284. doi:10.4103/0976-500X.142464
Bhomia, M., Sharma, A., Gayen, M., Gupta, P., and Maheshwari, R. K. (2013). Artificial microRNAs can effectively inhibit replication of Venezuelan equine encephalitis virus. Antivir. Res. 100 (2), 429–434. doi:10.1016/j.antiviral.2013.08.010
Bigoni, A., Malik, A. M., Tasca, R., Carrera, M. B. M., Schiesari, L. M. C., Gambardella, D. D., et al. (2022). Brazil’s health system functionality amidst of the COVID-19 pandemic: An analysis of resilience. Lancet Regional Health – Am. 10, 100222. doi:10.1016/j.lana.2022.100222
Bishop, B. M. (2015). Potential and emerging treatment options for Ebola virus disease. Ann. Pharmacother. 49, 196–206. doi:10.1177/1060028014561227
Boldescu, V., Behnam, M. A. M., Vasilakis, N., and Klein, C. D. (2017). Broad-spectrum agents for flaviviral infections: Dengue, Zika and beyond. Nat. Rev. Drug Discov. 16 (8), 565–586. doi:10.1038/nrd.2017.33
Brazier, E., Maruri, F., Wester, C. W., Musick, B., Freeman, A., Parcesepe, A., et al. (2023). Design and implementation of a global site assessment survey among HIV clinics participating in the International epidemiology Databases to Evaluate AIDS (IeDEA) research consortium. PloS one 18 (3), e0268167. doi:10.1371/journal.pone.0268167
Brito, A. F., Semenova, E., Dudas, G., Hassler, G. W., Kalinich, C. C., Kraemer, M. U. G., et al. (2022). Global disparities in SARS-CoV-2 genomic surveillance. Nat. Commun. 13 (1), 7003–7013. doi:10.1038/s41467-022-33713-y
Candido, D. S., Ingra, M. C., Jaqueline, G. D. J., William, M. S., Filipe, R. R. M., Simon, D., et al. (2020). Evolution and epidemic spread of SARS-CoV-2 in Brazil. Science 369 (6508), 1255–1260. doi:10.1126/science.abd2161
Cannalire, R., Cerchia, C., Beccari, A. R., Di Leva, F. S., and Summa, V. (2022). Targeting SARS-CoV-2 proteases and polymerase for COVID-19 treatment: State of the art and future opportunities. J. Med. Chem. 65 (4), 2716–2746. doi:10.1021/acs.jmedchem.0c01140
Carvalho, V. L., and Long, M. T. (2021). Perspectives on new vaccines against arboviruses using insect-specific viruses as platforms. Vaccines 9 (3), 263. doi:10.3390/vaccines9030263
Cascella, M., Rajnik, M., Aleem, A., Dulebohn, S. C., and Napoli, R. D. (2023). “Features, evaluation, and treatment of coronavirus (COVID-19),” in StatPearls [internet] (FL, United States of America: StatPearls Publishing).
Chambers, T. J., Hahn, C. S., Galler, R., and Rice, C. M. (1990). Flavivirus genome organization, expression, and replication. Annu. Rev. Microbiol. 44, 649–688. doi:10.1146/annurev.mi.44.100190.003245
Chancey, C. (2015). The global ecology and epidemiology of West Nile virus. BioMed Res. Int. 2015, 376230. doi:10.1155/2015/376230
Chaudhary, N., Weissman, D., and Whitehead, K. A. (2021). mRNA vaccines for infectious diseases: Principles, delivery and clinical translation. Nat. Rev. Drug Discov. 20 (11), 817–838. doi:10.1038/s41573-021-00283-5
Chen, C. (2020) ‘Favipiravir versus arbidol for COVID-19: A randomized clinical trial’, medRxiv. doi:10.1101/2020.03.17.20037432
Chen, R., Mukhopadhyay, S., Merits, A., Bolling, B., Nasar, F., Coffey, L. L., et al. (2018). ICTV virus taxonomy profile: Togaviridae. J. general virology 99 (6), 761–762. doi:10.1099/jgv.0.001072
Chen, Z., Azman, A. S., Chen, X., Zou, J., Tian, Y., Sun, R., et al. (2022). Global landscape of SARS-CoV-2 genomic surveillance and data sharing. Nat. Genet. 54 (4), 499–507. doi:10.1038/s41588-022-01033-y
Chera, A., and Tanca, A. (2022). Remdesivir: The first FDA-approved anti-COVID-19 treatment for Young children. Discov. (Craiova, Rom. 10 (2), E151. doi:10.15190/d.2022.10
Chippaux, J.-P., and Chippaux, A. (2018). Yellow fever in Africa and the americas: A historical and epidemiological perspective. J. Venom. animals toxins Incl. Trop. Dis. 24, 20. doi:10.1186/s40409-018-0162-y
Cochrane, G., Karsch-Mizrachi, I., and Takagi, T., and International Nucleotide Sequence Database Collaboration (2016). The international nucleotide sequence database collaboration. Nucleic acids Res. 44, D48–D50. doi:10.1093/nar/gkv1323
Collins, M. H., and Metz, S. W. (2017). Progress and works in progress: Update on flavivirus vaccine development. Clin. Ther. 39 (8), 1519–1536. doi:10.1016/j.clinthera.2017.07.001
Corrin, T., Ackford, R., Mascarenhas, M., Greig, J., and Waddell, L. A. (2021). Eastern equine encephalitis virus: A scoping review of the global evidence. Vector borne zoonotic Dis. 21 (5), 305–320. doi:10.1089/vbz.2020.2671
Cunha, M., dos, P., Pour, S. Z., Ortiz-Baez, A. S., Černý, J., Pereira, B. B. d. S., et al. (2019). Origin of the São Paulo Yellow Fever epidemic of 2017–2018 revealed through molecular epidemiological analysis of fatal cases. Sci. Rep. 9, 20418. doi:10.1038/s41598-019-56650-1
da Costa, V. G., Moreli, M. L., and Saivish, M. V. (2020). The emergence of SARS, MERS and novel SARS-2 coronaviruses in the 21st century. Archives virology 165 (7), 1517–1526. doi:10.1007/s00705-020-04628-0
Dash, P. K., Tiwari, M., Santhosh, S. R., Parida, M., and Lakshmana Rao, P. V. (2008). RNA interference mediated inhibition of Chikungunya virus replication in mammalian cells. Biochem. biophysical Res. Commun. 376 (4), 718–722. doi:10.1016/j.bbrc.2008.09.040
de Haan, C. A., and Rottier, P. J. (2005). Molecular interactions in the assembly of coronaviruses. Adv. virus Res. 64, 165–230. doi:10.1016/S0065-3527(05)64006-7
de Souza, W. M. (2023). Spatiotemporal dynamics and recurrence of chikungunya virus in Brazil: An epidemiological study. Lancet. Microbe 4, E319–E329. Preprint. doi:10.1016/S2666-5247(23)00033-2
de Wit, E., van Doremalen, N., Falzarano, D., and Munster, V. J. (2016). SARS and MERS: Recent insights into emerging coronaviruses. Nat. Rev. Microbiol. 14 (8), 523–534. doi:10.1038/nrmicro.2016.81
Dekker, S. E., Green, E. W., and Ahn, J. (2021). Treatment and prevention of acute hepatitis B virus. Clin. liver Dis. 25 (4), 711–724. doi:10.1016/j.cld.2021.06.002
Delgado, S., Erickson, B. R., Agudo, R., Blair, P. J., Vallejo, E., Albariño, C. G., et al. (2008). Chapare virus, a newly discovered arenavirus isolated from a fatal hemorrhagic fever case in Bolivia. PLoS Pathog. 4 (4), e1000047. doi:10.1371/journal.ppat.1000047
Dennehy, J. J. (2017). Evolutionary ecology of virus emergence. Ann. N. Y. Acad. Sci. 1389 (1), 124–146. doi:10.1111/nyas.13304
Desiree LaBeaud, A. (2008). Why arboviruses can Be neglected tropical diseases. PLoS neglected Trop. Dis. 2 (6), e247. doi:10.1371/journal.pntd.0000247
Devaux, C. A. (2012). Emerging and re-emerging viruses: A global challenge illustrated by chikungunya virus outbreaks. World J. virology 1 (1), 11–22. doi:10.5501/wjv.v1.i1.11
Dhama, K., Patel, S. K., Sharun, K., Pathak, M., Tiwari, R., Yatoo, M. I., et al. (2020). SARS-CoV-2 jumping the species barrier: Zoonotic lessons from SARS, MERS and recent advances to combat this pandemic virus. Travel Med. Infect. Dis. 37, 101830. doi:10.1016/j.tmaid.2020.101830
Diaz, A., Coffey, L. L., Burkett-Cadena, N., and Day, J. F. (2018). Reemergence of st. Louis encephalitis virus in the americas. Emerg. Infect. Dis. 24 (12), 2150–2157. doi:10.3201/eid2412.180372
Ding, C., Tang, W., Xia, B., Peng, H., Liu, Y., Wang, J., et al. (2022). High-throughput screening of FDA-approved drug library reveals ixazomib is a broad-spectrum antiviral agent against arboviruses. Viruses 14 (7), 1381. doi:10.3390/v14071381
Dolan, P. T., Whitfield, Z. J., and Andino, R. (2018). Mapping the evolutionary potential of RNA viruses. Cell host microbe 23 (4), 435–446. doi:10.1016/j.chom.2018.03.012
Dolgin, E. (2021). The tangled history of mRNA vaccines. Berlin, Germany: Nature Publishing Group. doi:10.1038/d41586-021-02483-w
Domingo, E. (2010). Mechanisms of viral emergence. Veterinary Res. 41 (6), 38. doi:10.1051/vetres/2010010
Dong, S., and Dimopoulos, G. (2021). Antiviral compounds for blocking arboviral transmission in mosquitoes. Viruses 13 (1), 108. doi:10.3390/v13010108
Donia, A., and Bokhari, H. (2021). RNA interference as a promising treatment against SARS-CoV-2. Int. Microbiol. official J. Span. Soc. Microbiol. 24 (1), 123–124. doi:10.1007/s10123-020-00146-w
Donovan-Banfield, I., Penrice-Randal, R., Goldswain, H., Rzeszutek, A. M., Pilgrim, J., Bullock, K., et al. (2022). Characterisation of SARS-CoV-2 genomic variation in response to molnupiravir treatment in the AGILE Phase IIa clinical trial. Nat. Commun. 13, 7284. doi:10.1038/s41467-022-34839-9
Dorsett, M. (2020). Point of no return: COVID-19 and the U.S. Healthcare system: An emergency physician’s perspective. Sci. Adv. 6, eabc5354. doi:10.1126/sciadv.abc5354
Drebot, M. A., Jones, S., Grolla, A., Safronetz, D., Strong, J. E., Kobinger, G., et al. (2015). Hantavirus pulmonary syndrome in Canada: An overview of clinical features, diagnostics, epidemiology and prevention. Can. Commun. Dis. Rep. = Releve Des. Mal. Transm. au Can. 41 (6), 124–131. doi:10.14745/ccdr.v41i06a02
Elbe, S., and Buckland-Merrett, G. (2017). Data, disease and diplomacy: GISAID’s innovative contribution to global health. Glob. challenges Hob. NJ) 1 (1), 33–46. doi:10.1002/gch2.1018
Ellwanger, J. H., and Chies, J. A. B. (2021). Zoonotic spillover: Understanding basic aspects for better prevention. Genet. Mol. Biol. 44, e20200355. doi:10.1590/1678-4685-GMB-2020-0355
Enria, D. A., Briggiler, A. M., and Sánchez, Z. (2008). Treatment of Argentine hemorrhagic fever. Antivir. Res. 78 (1), 132–139. doi:10.1016/j.antiviral.2007.10.010
Erlanger, T. E., Weiss, S., Keiser, J., Utzinger, J., and Wiedenmayer, K. (2009). Past, present, and future of Japanese encephalitis. Emerg. Infect. Dis. 15 (1), 1–7. doi:10.3201/eid1501.080311
Extance, A. (2022)., 377. BMJ, o926. Covid-19: What is the evidence for the antiviral molnupiravir? doi:10.1136/bmj.o926
Faria, N. R., Mellan, T. A., Whittaker, C., Claro, I. M., Candido, D. d. S., Mishra, S., et al. (2021). Genomics and epidemiology of the P.1 SARS-CoV-2 lineage in Manaus, Brazil. Science 372 (6544), 815–821. doi:10.1126/science.abh2644
Fichet-Calvet, E., and Rogers, D. J. (2009). Risk maps of Lassa fever in west Africa. PLoS neglected Trop. Dis. 3 (3), e388. doi:10.1371/journal.pntd.0000388
Figueiredo, M. L. G., and Figueiredo, L. T. M. (2014). Emerging alphaviruses in the americas: Chikungunya and Mayaro. Rev. Soc. Bras. Med. Trop. 47 (6), 677–683. doi:10.1590/0037-8682-0246-2014
Focosi, D., Maggi, F., Shoham, S., and Casadevall, A. (2023). Discriminating endogenous vaccine-elicited anti-Spike antibody responses from exogenous anti-Spike monoclonal antibodies: The case of Evusheld. J. Med. virology 95 (1), e28277. doi:10.1002/jmv.28277
Freire, C. C. M., Iamarino, A., Soumaré, P. O. L., Faye, O., Sall, A. A., and Zanotto, P. M. A. (2015). Reassortment and distinct evolutionary dynamics of Rift Valley Fever virus genomic segments. Sci. Rep. 5, 11353. doi:10.1038/srep11353
Fulhorst, C. F., Cajimat, M. N. B., Milazzo, M. L., Paredes, H., de Manzione, N. M. C., Salas, R. A., et al. (2008). Genetic diversity between and within the arenavirus species indigenous to Western Venezuela. Virology 378 (2), 205–213. doi:10.1016/j.virol.2008.05.014
Galipeau, Y., Greig, M., Liu, G., Driedger, M., and Langlois, M. A. (2020). Humoral responses and serological assays in SARS-CoV-2 infections. Front. Immunol. 11, 610688. doi:10.3389/fimmu.2020.610688
Gallo, G. L., López, N., and Loureiro, M. E. (2022). The virus-host interplay in junín mammarenavirus infection. Viruses 14 (6), 1134. doi:10.3390/v14061134
Gan, C. S., Lim, S. K., Chee, C. F., Yusof, R., and Heh, C. H. (2018). Sofosbuvir as treatment against dengue? Chem. Biol. drug Des. 91 (2), 448–455. doi:10.1111/cbdd.13091
Gao, S., Song, S., and Zhang, L. (2019). Recent progress in vaccine development against chikungunya virus. Front. Microbiol. 10, 2881. doi:10.3389/fmicb.2019.02881
Ge, N., Sun, J., Liu, Z., Shu, J., Yan, H., Kou, Z., et al. (2022). An mRNA vaccine encoding Chikungunya virus E2-E1 protein elicits robust neutralizing antibody responses and CTL immune responses. Virol. Sin. 37 (2), 266–276. doi:10.1016/j.virs.2022.01.032
Giulietti, M., Righetti, A., Cianfruglia, L., Šabanović, B., Armeni, T., Principato, G., et al. (2018). To accelerate the Zika beat: Candidate design for RNA interference-based therapy. Virus Res. 255, 133–140. doi:10.1016/j.virusres.2018.07.010
Go, Y. Y., Balasuriya, U. B. R., and Lee, C.-K. (2014). Zoonotic encephalitides caused by arboviruses: Transmission and epidemiology of alphaviruses and flaviviruses. Clin. Exp. vaccine Res. 3 (1), 58–77. doi:10.7774/cevr.2014.3.1.58
Gómez, R. M., Jaquenod de Giusti, C., Sanchez Vallduvi, M. M., Frik, J., Ferrer, M. F., and Schattner, M. (2011). Junín virus. A XXI century update. Microbes Infect. / Institut Pasteur 13 (4), 303–311. doi:10.1016/j.micinf.2010.12.006
Gordon, C. J., Tchesnokov, E. P., Feng, J. Y., Porter, D. P., and Götte, M. (2020). The antiviral compound remdesivir potently inhibits RNA-dependent RNA polymerase from Middle East respiratory syndrome coronavirus. J. Biol. Chem. 295 (15), 4773–4779. doi:10.1074/jbc.AC120.013056
Guan, Y., Zheng, B. J., He, Y. Q., Liu, X. L., Zhuang, Z. X., Cheung, C. L., et al. (2003). Isolation and characterization of viruses related to the SARS coronavirus from animals in southern China. Science 87, 276–278. doi:10.1126/science.1087139
Gubler, D. J. (2001). Human arbovirus infections worldwide. Ann. N. Y. Acad. Sci. 951, 13–24. doi:10.1111/j.1749-6632.2001.tb02681.x
Gubler, D. J. (2002). The global emergence/resurgence of arboviral diseases as public health problems. Archives Med. Res. 33 (4), 330–342. doi:10.1016/s0188-4409(02)00378-8
Haikerwal, A. (2022)., 14. Viruses, 1628. Inhibition of Venezuelan equine encephalitis virus using small interfering RNAs doi:10.3390/v14081628
Hamed, A. A., Fandy, T. E., Tkaczuk, K. L., Verspoor, K., and Lee, B. S. (2022). COVID-19 drug repurposing: A network-based framework for exploring biomedical literature and clinical trials for possible treatments. Pharmaceutics 14 (3), 567. doi:10.3390/pharmaceutics14030567
Hanifa, M., Salman, M., Fatima, M., Mukhtar, N., Almajhdi, F. N., Zaman, N., et al. (2022). Mutational analysis of the spike protein of SARS-COV-2 isolates revealed atomistic features responsible for higher binding and infectivity. Front. Cell Dev. Biol. 10, 940863. doi:10.3389/fcell.2022.940863
Hay, J., Essbauer, S. S., Fair, J. M., and Hewson, R. (2022). Infectious disease surveillance: Applying cooperative research to recent outbreaks including COVID-19. Lausanne, Switzerland: Frontiers Media SA.
Heinen, L. B., Chen, S., Serra, O. P., Cardoso, B. F., Gondim, B. H. F., Dos Santos, M. A. M., et al. (2015). Saint Louis encephalitis virus in mato grosso, central-western Brazil. Rev. do Inst. Med. Trop. Sao Paulo 57 (3), 215–220. doi:10.1590/S0036-46652015000300006
Heymann, D. L., Chen, L., Takemi, K., Fidler, D. P., Tappero, J. W., Thomas, M. J., et al. (2015). Global health security: The wider lessons from the west african Ebola virus disease epidemic. Lancet 385, 1884–1901. doi:10.1016/s0140-6736(15)60858-3
Hijawi, B., Abdallat, M., Sayaydeh, A., Alqasrawi, S., Haddadin, A., Jaarour, N., et al. (2013). Novel coronavirus infections in Jordan, april 2012: Epidemiological findings from a retrospective investigation. East. Mediterr. health J. = La revue de sante de Mediterr. Orient. = al-Majallah al-sihhiyah li-sharq al-mutawassit 19, S12–S18. doi:10.26719/2013.19.supp1.s12
Hilgenfeld, R., and Peiris, M. (2013). From SARS to MERS: 10 years of research on highly pathogenic human coronaviruses. Antivir. Res. 100 (1), 286–295. doi:10.1016/j.antiviral.2013.08.015
Hoffmann, M., Kleine-Weber, H., Schroeder, S., Krüger, N., Herrler, T., Erichsen, S., et al. (2020). SARS-CoV-2 cell entry depends on ACE2 and TMPRSS2 and is blocked by a clinically proven protease inhibitor. Cell 181 (2), 271–280. doi:10.1016/j.cell.2020.02.052
Holmes, E. C. (2010). Evolution in health and medicine Sackler colloquium: The comparative genomics of viral emergence. Proc. Natl. Acad. Sci. U. S. A. 107, 1742–1746. doi:10.1073/pnas.0906193106
Holmes, E. C., Goldstein, S. A., Rasmussen, A. L., Robertson, D. L., Crits-Christoph, A., Wertheim, J. O., et al. (2021). The origins of SARS-CoV-2: A critical review. Cell 184 (19), 4848–4856. doi:10.1016/j.cell.2021.08.017
Hong, J.-M., Hu, L. H., Zhong, Q. S., Zhu, L. C., Hang, Y. P., Fang, X. Y., et al. (2020). Epidemiological characteristics and clinical features of patients infected with the COVID-19 virus in nanchang, jiangxi, China. Front. Med. 7, 571069. doi:10.3389/fmed.2020.571069
Howard-Jones, A. R., Pham, D., Sparks, R., Maddocks, S., Dwyer, D. E., Kok, J., et al. (2023). Arthropod-borne flaviviruses in pregnancy. Microorganisms 11 (2), 433. doi:10.3390/microorganisms11020433
Hua, J., Wang, G., Huang, M., Hua, S., and Yang, S. (2020). A visual approach for the SARS (severe acute respiratory syndrome) outbreak data analysis. Int. J. Environ. Res. public health 17 (11), 3973. doi:10.3390/ijerph17113973
Hübschen, J. M., Gouandjika-Vasilache, I., and Dina, J. (2022). Measles. Lancet 399 (10325), 678–690. doi:10.1016/S0140-6736(21)02004-3
Hucke, F. I. L., and Bugert, J. J. (2020). Current and promising antivirals against chikungunya virus. Front. Public Health 8, 618624. doi:10.3389/fpubh.2020.618624
Hui, E. K.-W. (2006). Reasons for the increase in emerging and re-emerging viral infectious diseases. Microbes Infect./Institut Pasteur 8 (3), 905–916. doi:10.1016/j.micinf.2005.06.032
Hussain, I., Pervaiz, N., Khan, A., Saleem, S., Shireen, H., Wei, D. Q., et al. (2020). Evolutionary and structural analysis of SARS-CoV-2 specific evasion of host immunity. Genes and Immun. 21 (6), 409–419. doi:10.1038/s41435-020-00120-6
Idrees, S., and Ashfaq, U. A. (2013). RNAi: Antiviral therapy against dengue virus. Asian Pac. J. Trop. Biomed. 3 (3), 232–236. doi:10.1016/S2221-1691(13)60057-X
Idris, A., Davis, A., Supramaniam, A., Acharya, D., Kelly, G., Tayyar, Y., et al. (2021). A SARS-CoV-2 targeted siRNA-nanoparticle therapy for COVID-19. Mol. Ther. J. Am. Soc. Gene Ther. 29 (7), 2219–2226. doi:10.1016/j.ymthe.2021.05.004
Infantino, M., Pieri, M., Nuccetelli, M., Grossi, V., Lari, B., Tomassetti, F., et al. (2021). The WHO international standard for COVID-19 serological tests: Towards harmonization of anti-spike assays. Int. Immunopharmacol. 100, 108095. doi:10.1016/j.intimp.2021.108095
Ivanova, L., Rausalu, K., Ošeka, M., Kananovich, D. G., Žusinaite, E., Tammiku-Taul, J., et al. (2021). Novel analogues of the chikungunya virus protease inhibitor: Molecular design, synthesis, and biological evaluation. ACS omega 6 (16), 10884–10896. doi:10.1021/acsomega.1c00625
Jacobs, L. G., Gourna Paleoudis, E., Lesky-Di Bari, D., Nyirenda, T., Friedman, T., Gupta, A., et al. (2020). Persistence of symptoms and quality of life at 35 days after hospitalization for COVID-19 infection. PloS one 15 (12), e0243882. doi:10.1371/journal.pone.0243882
Jacot, D., Pillonel, T., Greub, G., and Bertelli, C. (2021). Assessment of SARS-CoV-2 genome sequencing: Quality criteria and low-frequency variants. J. Clin. Microbiol. 59 (10), e0094421. doi:10.1128/JCM.00944-21
Jones, B. A., Grace, D., Kock, R., Alonso, S., Rushton, J., Said, M. Y., et al. (2013). Zoonosis emergence linked to agricultural intensification and environmental change. Proc. Natl. Acad. Sci. U. S. A. 110 (21), 8399–8404. doi:10.1073/pnas.1208059110
Jones, K. E., Patel, N. G., Levy, M. A., Storeygard, A., Balk, D., Gittleman, J. L., et al. (2008). Global trends in emerging infectious diseases. Nature 451 (7181), 990–993. doi:10.1038/nature06536
Kabinger, F., Stiller, C., Schmitzová, J., Dienemann, C., Kokic, G., Hillen, H. S., et al. (2021). Mechanism of molnupiravir-induced SARS-CoV-2 mutagenesis. Nat. Struct. Mol. Biol. 28 (9), 740–746. doi:10.1038/s41594-021-00651-0
Kamal, L., Ramadan, A., Farraj, S., Bahig, L., and Ezzat, S. (2022). The pill of recovery; Molnupiravir for treatment of COVID-19 patients; a systematic review. Saudi Pharm. J. SPJ official Publ. Saudi Pharm. Soc. 30 (5), 508–518. doi:10.1016/j.jsps.2022.03.002
Kara, G., Calin, G. A., and Ozpolat, B. (2022). RNAi-based therapeutics and tumor targeted delivery in cancer. Adv. drug Deliv. Rev. 182, 114113. doi:10.1016/j.addr.2022.114113
Karothia, D., Kumar Dash, P., Parida, M., Bhagyawant, S. S., and Kumar, J. S. (2020). Vector derived artificial miRNA mediated inhibition of West Nile virus replication and protein expression. Gene 729, 144300. doi:10.1016/j.gene.2019.144300
Keating, G. M. (2014). Sofosbuvir: A review of its use in patients with chronic hepatitis C. Drugs 74 (10), 1127–1146. doi:10.1007/s40265-014-0247-z
Kertes, J., et al. (2023). Association between AZD7442 (Tixagevimab-Cilgavimab) administration and severe acute respiratory syndrome coronavirus 2 (SARS-CoV-2) infection, hospitalization, and mortality. Clin. Infect. Dis. 76 (3), e126–e132.
Ketzinel-Gilad, M., Shaul, Y., and Galun, E. (2006). RNA interference for antiviral therapy. J. gene Med. 8 (8), 933–950. doi:10.1002/jgm.929
Khan, J., and Dunbar, N. M. (2021). Evolution of RNA viruses from SARS to SARS-CoV-2 and diagnostic techniques for COVID-19: A review. Beni-Suef Univ. J. Basic Appl. Sci. 10 (1), 1–4. doi:10.1111/trf.16228
Khandaker, G., Dierig, A., Rashid, H., King, C., Heron, L., and Booy, R. (2011). Systematic review of clinical and epidemiological features of the pandemic influenza A (H1N1) 2009. Influenza other Respir. viruses 5 (3), 148–156. doi:10.1111/j.1750-2659.2011.00199.x
Khare, S., Gurry, C., Freitas, L., Schultz, M. B., Bach, G., Diallo, A., et al. (2021). GISAID’s role in pandemic response. China CDC Wkly. 3 (49), 1049–1051. doi:10.46234/ccdcw2021.255
Khuroo, M. S., and Khuroo, M. S. (2016). Hepatitis E: An emerging global disease - from discovery towards control and cure. J. viral Hepat. 23 (2), 68–79. doi:10.1111/jvh.12445
Kirola, L. (2021). Genetic emergence of B.1.617.2 in COVID-19. New Microbes New Infect. 43, 100929. doi:10.1016/j.nmni.2021.100929
Klein, N. P. (2022). Effectiveness of COVID-19 pfizer-BioNTech BNT162b2 mRNA vaccination in preventing COVID-19–associated emergency department and urgent care encounters and hospitalizations among nonimmunocompromised children and adolescents aged 5–17 Years — VISION network, 10 States, april 2021–january 2022. MMWR. Morb. Mortal. Wkly. Rep. 71, 352–358. doi:10.15585/mmwr.mm7109e3
Kofman, A., Rahav, G., Yazzie, D., Shorty, H., Yaglom, H. D., Peterson, D., et al. (2018). Notes from the field: Exported case of Sin nombre hantavirus pulmonary syndrome - Israel, 2017. MMWR. Morb. Mortal. Wkly. Rep. 67 (40), 1129. doi:10.15585/mmwr.mm6740a5
Kokic, G., Hillen, H. S., Tegunov, D., Dienemann, C., Seitz, F., Schmitzova, J., et al. (2021). Mechanism of SARS-CoV-2 polymerase stalling by remdesivir. Nat. Commun. 12 (1), 279–287. doi:10.1038/s41467-020-20542-0
Konkolova, E., Dejmek, M., Hřebabecký, H., Šála, M., Böserle, J., Nencka, R., et al. (2020). Remdesivir triphosphate can efficiently inhibit the RNA-dependent RNA polymerase from various flaviviruses. Antivir. Res. 182, 104899. doi:10.1016/j.antiviral.2020.104899
Koonin, E. V., Krupovic, M., and Agol, V. I. (2021). The Baltimore classification of viruses 50 Years later: How does it stand in the light of virus evolution? Microbiol. Mol. Biol. Rev. MMBR 85 (3), e0005321. doi:10.1128/MMBR.00053-21
Koonin, E. V., Senkevich, T. G., and Dolja, V. V. (2006). The ancient Virus World and evolution of cells. Biol. direct 1 (1), 29–27. doi:10.1186/1745-6150-1-29
Kril, V., Aïqui-Reboul-Paviet, O., Briant, L., and Amara, A. (2021). New insights into chikungunya virus infection and pathogenesis. Annu. Rev. virology 8 (1), 327–347. doi:10.1146/annurev-virology-091919-102021
Kristen, A. V., Ajroud-Driss, S., Conceição, I., Gorevic, P., Kyriakides, T., and Obici, L. (2019). Patisiran, an RNAi therapeutic for the treatment of hereditary transthyretin-mediated amyloidosis. Neurodegener. Dis. Manag. 9 (1), 5–23. doi:10.2217/nmt-2018-0033
Ksiazek, T. G., Erdman, D., Goldsmith, C. S., Zaki, S. R., Peret, T., Emery, S., et al. (2003). A novel coronavirus associated with severe acute respiratory syndrome. N. Engl. J. Med. 348 (20), 1953–1966. doi:10.1056/NEJMoa030781
Kumar, N., Acharya, A., Gendelman, H. E., and Byrareddy, S. N. (2022). The 2022 outbreak and the pathobiology of the monkeypox virus. J. Autoimmun. 131, 102855. doi:10.1016/j.jaut.2022.102855
Kyriakidis, N. C., López-Cortés, A., González, E. V., Grimaldo, A. B., and Prado, E. O. (2021). SARS-CoV-2 vaccines strategies: A comprehensive review of phase 3 candidates. NPJ Vaccines 6, 28. doi:10.1038/s41541-021-00292-w
Lai, M. M., and Cavanagh, D. (1997). The molecular biology of coronaviruses. Adv. virus Res. 48, 1–100. doi:10.1016/S0065-3527(08)60286-9
Lam, S., Chen, K. C., Ng, M. M. L., and Chu, J. J. H. (2012). Expression of plasmid-based shRNA against the E1 and nsP1 genes effectively silenced Chikungunya virus replication. PloS one 7 (10), e46396. doi:10.1371/journal.pone.0046396
Larocca, R. A., Abbink, P., Peron, J. P. S., Zanotto, P. M. d. A., Iampietro, M. J., Badamchi-Zadeh, A., et al. (2016). Vaccine protection against Zika virus from Brazil. Nature 536 (7617), 474–478. doi:10.1038/nature18952
Lee, B. E., and Pang, X.-L. (2013). New strains of norovirus and the mystery of viral gastroenteritis epidemics. CMAJ Can. Med. Assoc. J. = J. de l’Association medicale Can. 185 (16), 1381–1382. doi:10.1503/cmaj.130426
Li, F. (2016). Structure, function, and evolution of coronavirus spike proteins. Annu. Rev. virology 3 (1), 237–261. doi:10.1146/annurev-virology-110615-042301
Lu, J., du Plessis, L., Liu, Z., Hill, V., Kang, M., Lin, H., et al. (2020). Genomic epidemiology of SARS-CoV-2 in Guangdong province, China. Cell 181 (5), 997–1003. doi:10.1016/j.cell.2020.04.023
Lv, Z., Chu, Y., and Wang, Y. (2015). HIV protease inhibitors: A review of molecular selectivity and toxicity. HIV/AIDS 7, 95–104. doi:10.2147/HIV.S79956
Mackenzie, J. (2005). Wrapping things up about virus RNA replication. Traffic 6 (11), 967–977. doi:10.1111/j.1600-0854.2005.00339.x
Malecela, M. N., and Ducker, C. (2021). A road map for neglected tropical diseases 2021–2030. Geneva, Switzerland: world health organization, 121–123. doi:10.1093/trstmh/trab002
Malik, S., Dhasmana, A., Bora, J., Uniyal, P., Slama, P., Preetam, S., et al. (2023). Ebola virus disease (EVD) outbreak re-emergence regulation in East Africa: Preparedness and vaccination perspective. Int. J. Surg. 109, 1029–1031. Preprint. doi:10.1097/JS9.0000000000000175
Malin, J. J., Suárez, I., Priesner, V., Fätkenheuer, G., and Rybniker, J. (2020). Remdesivir against COVID-19 and other viral diseases. Clin. Microbiol. Rev. 34, 001622–e220. doi:10.1128/cmr.00162-20
Marcondes, C. B., and Ximenes, M. F. F. M. (2016). Zika virus in Brazil and the danger of infestation by Aedes (Stegomyia) mosquitoes. Rev. Soc. Bras. Med. Trop. 49 (1), 4–10. doi:10.1590/0037-8682-0220-2015
Martin, D. P., Weaver, S., Tegally, H., San, J. E., Shank, S. D., Wilkinson, E., et al. (2021). The emergence and ongoing convergent evolution of the SARS-CoV-2 N501Y lineages. Cell 184 (20), 5189–5200. e7. doi:10.1016/j.cell.2021.09.003
Martinez, V. P., Bellomo, C., San Juan, J., Pinna, D., Forlenza, R., Elder, M., et al. (2005). Person-to-person transmission of Andes virus. Emerg. Infect. Dis. 11 (12), 1848–1853. doi:10.3201/eid1112.050501
Martins-Melo, F. R., Carneiro, M., Ramos, A. N., Heukelbach, J., Ribeiro, A. L. P., and Werneck, G. L. (2018). The burden of neglected tropical diseases in Brazil, 1990-2016: A subnational analysis from the global burden of disease study 2016. PLoS neglected Trop. Dis. 12 (6), e0006559. doi:10.1371/journal.pntd.0006559
Marzi, M., Vakil, M. K., Bahmanyar, M., and Zarenezhad, E. (2022). Paxlovid: Mechanism of action, synthesis, and in silico study. BioMed Res. Int. 2022, 7341493. doi:10.1155/2022/7341493
Maslow, J. N. (2019). Challenges and solutions in the development of vaccines against emerging and neglected infectious diseases. Hum. vaccines Immunother. 15 (10), 2230–2234. doi:10.1080/21645515.2019.1661209
McCarthy, K. R., Rennick, L. J., Nambulli, S., Robinson-McCarthy, L. R., Bain, W. G., Haidar, G., et al. (2021). Recurrent deletions in the SARS-CoV-2 spike glycoprotein drive antibody escape. Science 371 (6534), 1139–1142. doi:10.1126/science.abf6950
McIntosh, K., and Perlman, S. (2015). Coronaviruses, including severe acute respiratory syndrome (SARS) and Middle East respiratory syndrome (MERS). Mandell, Douglas, Bennett’s Princ. Pract. Infect. Dis. 2015, 1928–1936. e2. doi:10.1016/b978-1-4557-4801-3.00157-0
Mendes, É. A., Pilger, D. R. B. d., Santos Nastri, A. C. d. S., Malta, F. d. M., Pascoalino, B. D. S., Carneiro D'Albuquerque, L. A., et al. (2019). Sofosbuvir inhibits yellow fever virus in vitro and in patients with acute liver failure. Ann. hepatology 18 (6), 816–824. doi:10.1016/j.aohep.2019.09.001
Migueres, M., Lhomme, S., and Izopet, J. (2021). Hepatitis A: Epidemiology, high-risk groups, prevention and research on antiviral treatment. Viruses 13 (10), 1900. doi:10.3390/v13101900
Mitman, G. (2022). Hotspots, spillovers and the shifting geopolitics of zoonotic emerging infectious diseases: A commentary. Int. Rev. Environ. Hist. 8, 131–137. doi:10.22459/ireh.08.01.2022.09
Mohapatra, R. K., Sarangi, A. K., Kandi, V., Azam, M., Tiwari, R., and Dhama, K. (2022). Omicron (B.1.1.529 variant of SARS-CoV-2); an emerging threat: Current global scenario. J. Med. virology 94 (5), 1780–1783. doi:10.1002/jmv.27561
Morens, D. M., Folkers, G. K., and Fauci, A. S. (2004). The challenge of emerging and re-emerging infectious diseases. Nature 430 (6996), 242–249. doi:10.1038/nature02759
Mourãão, M. P. G., Bastos, M. S., Gimaqu, J. B. L., Mota, B. R., Souza, G. S., Grimmer, G. H. N., et al. (2009). Oropouche fever outbreak, Manaus, Brazil, 2007-2008. Emerg. Infect. Dis. 15 (12), 2063–2064. doi:10.3201/eid1512.090917
Moya, A., Holmes, E. C., and González-Candelas, F. (2004). The population genetics and evolutionary epidemiology of RNA viruses. Nat. Rev. Microbiol. 2 (4), 279–288. doi:10.1038/nrmicro863
Munivenkatappa, A., Sahay, R. R., Yadav, P. D., Viswanathan, R., and Mourya, D. T. (2018). Clinical and epidemiological significance of Kyasanur forest disease. Indian J. Med. Res. 148 (2), 145–150. doi:10.4103/ijmr.IJMR_688_17
Murakami, N., Hayden, R., Hills, T., Al-Samkari, H., Casey, J., Del Sorbo, L., et al. (2022). Therapeutic advances in COVID-19. Springer Nature, 38–52. Berlin, Germany.
Narayanan, A., Narwal, M., Majowicz, S. A., Varricchio, C., Toner, S. A., Ballatore, C., et al. (2022). Identification of SARS-CoV-2 inhibitors targeting Mpro and PLpro using in-cell-protease assay. Commun. Biol. 5 (1), 169–217. doi:10.1038/s42003-022-03090-9
Naveca, F. G., Nascimento, V., Souza, V., Corado, A. d. L., Nascimento, F., Silva, G., et al. (2022). Spread of gamma (P.1) sub-lineages carrying spike mutations close to the furin cleavage site and deletions in the N-terminal domain drives ongoing transmission of SARS-CoV-2 in amazonas, Brazil. Microbiol. Spectr. 10 (1), e0236621. doi:10.1128/spectrum.02366-21
Niknam, Z., Jafari, A., Golchin, A., Danesh Pouya, F., Nemati, M., Rezaei-Tavirani, M., et al. (2022). Potential therapeutic options for COVID-19: An update on current evidence. Eur. J. Med. Res. 27 (1), 6–15. doi:10.1186/s40001-021-00626-3
Nitsche, C. (2019). Proteases from dengue, West Nile and Zika viruses as drug targets. Biophys. Rev. 11 (2), 157–165. doi:10.1007/s12551-019-00508-3
Nunes, D. A. F., Santos, F. R. d. S., da Fonseca, S. T. D., de Lima, W. G., Nizer, W. S. d. C., Ferreira, J. M. S., et al. (2022a). NS2B-NS3 protease inhibitors as promising compounds in the development of antivirals against zika virus: A systematic review. J. Med. virology 94 (2), 442–453. doi:10.1002/jmv.27386
Nunes, D. R., Braconi, C. T., Ludwig-Begall, L. F., Arns, C. W., and Durães-Carvalho, R. (2022b). Deep phylogenetic-based clustering analysis uncovers new and shared mutations in SARS-CoV-2 variants as a result of directional and convergent evolution. PloS one 17 (5), e0268389. doi:10.1371/journal.pone.0268389
Nygren, T. M., Pilic, A., Böhmer, M. M., Wagner-Wiening, C., Wichmann, O., Harder, T., et al. (2022). Tick-borne encephalitis vaccine effectiveness and barriers to vaccination in Germany. Sci. Rep. 12 (1), 11706. doi:10.1038/s41598-022-15447-5
O’Brien, L. (2007). Inhibition of multiple strains of Venezuelan equine encephalitis virus by a pool of four short interfering RNAs. Antivir. Res. 75 (1), 20–29. doi:10.1016/j.antiviral.2006.11.007
Oka, T., Wang, Q., Katayama, K., and Saif, L. J. (2015). Comprehensive review of human sapoviruses. Clin. Microbiol. Rev. 28 (1), 32–53. doi:10.1128/CMR.00011-14
Okoli, G. N., Rabbani, R., Copstein, L., Al-Juboori, A., Askin, N., and Abou-Setta, A. M. (2021). Remdesivir for coronavirus disease 2019 (COVID-19): A systematic review with meta-analysis and trial sequential analysis of randomized controlled trials. Infect. Dis. 53, 691–699. doi:10.1080/23744235.2021.1923799
Ong, S. P., Choo, B. G. H., Chu, J. J. H., and Ng, M. L. (2006). Expression of vector-based small interfering RNA against West Nile virus effectively inhibits virus replication. Antivir. Res. 72 (3), 216–223. doi:10.1016/j.antiviral.2006.06.005
Ong, S. P., Chu, J. J. H., and Ng, M. L. (2008). Inhibition of West Nile virus replication in cells stably transfected with vector-based shRNA expression system. Virus Res. 135 (2), 292–297. doi:10.1016/j.virusres.2008.04.014
Ott, J. J., Stevens, G. A., Groeger, J., and Wiersma, S. T. (2012). Global epidemiology of Hepatitis B virus infection: New estimates of age-specific HBsAg seroprevalence and endemicity. Vaccine 30 (12), 2212–2219. doi:10.1016/j.vaccine.2011.12.116
Pacca, C. C., Severino, A. A., Mondini, A., Rahal, P., D'avila, S. G. P., Cordeiro, J. A., et al. (2009). RNA interference inhibits yellow fever virus replication in vitro and in vivo. Virus genes 38 (2), 224–231. doi:10.1007/s11262-009-0328-3
Papanikolaou, V., Chrysovergis, A., Ragos, V., Tsiambas, E., Katsinis, S., Manoli, A., et al. (2022). From delta to Omicron: S1-RBD/S2 mutation/deletion equilibrium in SARS-CoV-2 defined variants. Gene 814, 146134. doi:10.1016/j.gene.2021.146134
Parashar, D., Paingankar, M. S., Kumar, S., Gokhale, M. D., Sudeep, A. B., Shinde, S. B., et al. (2013). Administration of E2 and NS1 siRNAs inhibit chikungunya virus replication in vitro and protects mice infected with the virus. PLoS neglected Trop. Dis. 7 (9), e2405. doi:10.1371/journal.pntd.0002405
Paraskevis, D., Kostaki, E. G., Magiorkinis, G., Panayiotakopoulos, G., Sourvinos, G., and Tsiodras, S. (2020). Full-genome evolutionary analysis of the novel corona virus (2019-nCoV) rejects the hypothesis of emergence as a result of a recent recombination event. Infect. Genet. Evol. J. Mol. Epidemiol. Evol. Genet. Infect. Dis. 79, 104212. doi:10.1016/j.meegid.2020.104212
Pardi, N., Hogan, M. J., Pelc, R. S., Muramatsu, H., Andersen, H., DeMaso, C. R., et al. (2017). Zika virus protection by a single low dose nucleoside modified mRNA vaccination. Nature 543 (7644), 248–251. doi:10.1038/nature21428
Pardi, N., Hogan, M. J., Porter, F. W., and Weissman, D. (2018) ‘mRNA vaccines — A new era in vaccinology’, Nat. Rev. Drug Discov., 17(4), pp. 261–279.
Patterson, M., Grant, A., and Paessler, S. (2014b). Epidemiology and pathogenesis of Bolivian hemorrhagic fever. Curr. Opin. virology 5, 82–90. doi:10.1016/j.coviro.2014.02.007
Patterson, M., Koma, T., Seregin, A., Huang, C., Miller, M., Smith, J., et al. (2014a). A substitution in the transmembrane region of the glycoprotein leads to an unstable attenuation of Machupo virus. J. virology 88 (18), 10995–10999. doi:10.1128/JVI.01007-14
Paul, A. M., Shi, Y., Acharya, D., Douglas, J. R., Cooley, A., Anderson, J. F., et al. (2014). Delivery of antiviral small interfering RNA with gold nanoparticles inhibits dengue virus infection in vitro. J. general virology 95 1712–1722. doi:10.1099/vir.0.066084-0
Paules, C. I., Marston, H. D., and Fauci, A. S. (2020). Coronavirus infections—more than just the common cold. JAMA J. Am. Med. Assoc. 323 (8), 707–708. doi:10.1001/jama.2020.0757
Perez-Mendez, M. (2020). siRNA design to silence the 3’UTR region of zika virus. BioMed Res. Int. 2020, 6759346. doi:10.1155/2020/6759346
Pielnaa, P., Al-Saadawe, M., Saro, A., Dama, M. F., Zhou, M., Huang, Y., et al. (2020). Zika virus-spread, epidemiology, genome, transmission cycle, clinical manifestation, associated challenges, vaccine and antiviral drug development. Virology 543, 34–42. doi:10.1016/j.virol.2020.01.015
Plante, J. A., Liu, Y., Liu, J., Xia, H., Johnson, B. A., Lokugamage, K. G., et al. (2020). Spike mutation D614G alters SARS-CoV-2 fitness. Nature 8, 1–6. doi:10.1038/s41586-020-2895-3
Plowright, R. K., Parrish, C. R., McCallum, H., Hudson, P. J., Ko, A. I., Graham, A. L., et al. (2017). Pathways to zoonotic spillover. Nat. Rev. Microbiol. 15 (8), 502–510. doi:10.1038/nrmicro.2017.45
Plowright, R. K., Reaser, J. K., Locke, H., Woodley, S. J., Patz, J. A., Becker, D. J., et al. (2021). Land use-induced spillover: A call to action to safeguard environmental, animal, and human health. Lancet Planet. Health 5, e237–e245. doi:10.1016/s2542-5196(21)00031-0
Poland, G. A., Ovsyannikova, I. G., and Kennedy, R. B. (2019). Zika vaccine development: Current status. Mayo Clin. Proc. Mayo Clin. 94 (12), 2572–2586. doi:10.1016/j.mayocp.2019.05.016
Portillo, A., Palomar, A. M., Santibáñez, P., and Oteo, J. A. (2021). Epidemiological aspects of crimean-Congo hemorrhagic fever in western europe: What about the future? ’, Microorg. 9 (3), 649. doi:10.3390/microorganisms9030649
Prakash, O., Khan, D. N., Singh, T., Shukla, S., Prakash, S., and Amita, J. (2021). Effect of siRNA targeting dengue virus genes on replication of dengue virus: An in vitro experimental study. Virusdisease 32 (3), 518–525. doi:10.1007/s13337-021-00700-8
Raabe, V., Mehta, A. K., and Evans, J. D. (2022). Lassa virus infection: A summary for clinicians. Int. J. Infect. Dis. IJID 119, 187–200. doi:10.1016/j.ijid.2022.04.004
Raheel, U., Jamal, M., and Zaidi, N. U. S. S. (2015). A molecular approach designed to limit the replication of mature DENV2 in host cells. Viral Immunol. 28 (7), 378–384. doi:10.1089/vim.2015.0034
Rey, F. A., Stiasny, K., Vaney, M. C., Dellarole, M., and Heinz, F. X. (2018). The bright and the dark side of human antibody responses to flaviviruses: Lessons for vaccine design. EMBO Rep. 19 (2), 206–224. doi:10.15252/embr.201745302
Richner, J. M., Himansu, S., Dowd, K. A., Butler, S. L., Salazar, V., Fox, J. M., et al. (2017). Modified mRNA vaccines protect against Zika virus infection. Cell 168 (6), 1114–1125. doi:10.1016/j.cell.2017.02.017
Rijkers, G. T., Weterings, N., Obregon-Henao, A., Lepolder, M., Dutt, T. S., van Overveld, F. J., et al. (2021). Antigen presentation of mRNA-based and virus-vectored SARS-CoV-2 vaccines. Vaccines 9 (8), 848. doi:10.3390/vaccines9080848
Rocha, R. F., Del Sarto, J. L., Marques, R. E., Costa, V. V., and Teixeira, M. M. (2018). Host target-based approaches against arboviral diseases. Biol. Chem. 399 (3), 203–217. doi:10.1515/hsz-2017-0236
Rodon, J., Muñoz-Basagoiti, J., Perez-Zsolt, D., Noguera-Julian, M., Paredes, R., Mateu, L., et al. (2021). Identification of plitidepsin as potent inhibitor of SARS-CoV-2-induced cytopathic effect after a drug repurposing screen. Front. Pharmacol. 12, 646676. doi:10.3389/fphar.2021.646676
Rodrigues Faria, N., Lourenço, J., Marques de Cerqueira, E., Maia de Lima, M., Pybus, O., and Carlos Junior Alcantara, L. (2016). Epidemiology of chikungunya virus in bahia, Brazil, 2014-2015. PLoS Curr. 8. doi:10.1371/currents.outbreaks.c97507e3e48efb946401755d468c28b2
Rodríguez-Morales, A. J., Bonilla-Aldana, D. K., Risquez, A., Paniz-Mondolfi, A., and Suárez, J. A. (2021). Should we be concerned about Venezuelan hemorrhagic fever? - a reflection on its current situation in Venezuela and potential impact in Latin America amid the migration crisis. New microbes new Infect. 44, 100945. doi:10.1016/j.nmni.2021.100945
Sacchetto, L., Drumond, B. P., Han, B. A., Nogueira, M. L., and Vasilakis, N. (2020). Re-emergence of yellow fever in the neotropics - quo vadis? Emerg. Top. life Sci. 4 (4), 399–410. doi:10.1042/ETLS20200187
Saha, A., Bhagyawant, S. S., Parida, M., and Dash, P. K. (2016). Vector-delivered artificial miRNA effectively inhibited replication of Chikungunya virus. Antivir. Res. 134, 42–49. doi:10.1016/j.antiviral.2016.08.019
Sahin, U., Karikó, K., and Türeci, Ö. (2014). mRNA-based therapeutics — developing a new class of drugs. Nat. Rev. Drug Discov. 13 (10), 759–780. doi:10.1038/nrd4278
Sakai, T., and Morimoto, Y. (2022). The history of infectious diseases and medicine. Pathogens 11 (10), 1147. doi:10.3390/pathogens11101147
Sandbrink, J. B., and Shattock, R. J. (2020). RNA vaccines: A suitable platform for tackling emerging pandemics? Front. Immunol. 11, 608460. doi:10.3389/fimmu.2020.608460
Santacroce, L., Charitos, I. A., Carretta, D. M., De Nitto, E., and Lovero, R. (2021). The human coronaviruses (HCoVs) and the molecular mechanisms of SARS-CoV-2 infection. J. Mol. Med. 99 (1), 93–106. doi:10.1007/s00109-020-02012-8
Sederdahl, B. K., and Williams, J. V. (2020). Epidemiology and clinical characteristics of influenza C virus. Viruses 12 (1), 89. doi:10.3390/v12010089
Setten, R. L., Rossi, J. J., and Han, S.-P. (2019). The current state and future directions of RNAi-based therapeutics. Drug Discov. 18 (6), 421–446. doi:10.1038/s41573-019-0017-4
Shaikh, O. A., Shekha, M., Shaikh, G., Ullah, I., Khattak, Z. E., and Nashwan, A. J. (2022). Crimean-Congo hemorrhagic fever epidemic during COVID-19 in Iraq: A “double whammy”. Clin. case Rep. 10 (10), e6450. doi:10.1002/ccr3.6450
Sharma, H., Tripathi, A., Kumari, B., Vrati, S., and Banerjee, A. (2018). Artificial MicroRNA-mediated inhibition of Japanese encephalitis virus replication in neuronal cells. Nucleic acid. Ther. 28 (6), 357–365. doi:10.1089/nat.2018.0743
Shi, H., Han, X., and Zheng, C. (2020). Evolution of CT manifestations in a patient recovered from 2019 novel coronavirus (2019-nCoV) pneumonia in wuhan, China. Radiology 2020, 200269. doi:10.1148/radiol.2020200269
Shu, Y., and McCauley, J. (2017). Gisaid: Global initiative on sharing all influenza data - from vision to reality. Euro Surveill. 22 (13), 30494. doi:10.2807/1560-7917.ES.2017.22.13.30494
Sidahmed, A., Abdalla, S., Mahmud, S., and Wilkie, B. (2014). Antiviral innate immune response of RNA interference. J. Infect. Dev. Ctries. 8, 804–810. doi:10.3855/jidc.4187
Silva, J. V. J., Ludwig-Begall, L. F., Oliveira-Filho, E. F. d., Oliveira, R. A. S., Durães-Carvalho, R., Lopes, T. R. R., et al. (2018). A scoping review of chikungunya virus infection: Epidemiology, clinical characteristics, viral co-circulation complications, and control. Acta trop. 188, 213–224. doi:10.1016/j.actatropica.2018.09.003
Simmonds, P., Becher, P., Bukh, J., Gould, E. A., Meyers, G., Monath, T., et al. (2017). ICTV virus taxonomy profile: Flaviviridae. J. general virology 98 (1), 2–3. doi:10.1099/jgv.0.000672
Simon-Loriere, E., Faye, O., Prot, M., Casademont, I., Fall, G., Fernandez-Garcia, M. D., et al. (2017). Autochthonous Japanese encephalitis with yellow fever coinfection in Africa. N. Engl. J. Med. 376 (15), 1483–1485. doi:10.1056/NEJMc1701600
Simpson, D. I. (1972). Arbovirus diseases. Br. Med. Bull. 28 (1), 10–15. doi:10.1093/oxfordjournals.bmb.a070876
Siomi, H., and Siomi, M. C. (2009). On the road to reading the RNA-interference code. Nature 457 (7228), 396–404. doi:10.1038/nature07754
Siqueira-Batista, R., De Souza Bayão, T., Do Carmo Cupertino, M., Alfred Joseph Mayers, N., and Patrícia Gomes, A. (2019). Sofosbuvir use for yellow fever: A new perspective treatment. Pathogens Glob. health 113 (5), 207–208. doi:10.1080/20477724.2019.1679556
Sirisena, P. D. N., Mahilkar, S., Sharma, C., Jain, J., and Sunil, S. (2021). Concurrent dengue infections: Epidemiology and clinical implications. Indian J. Med. Res. 154 (5), 669–679. doi:10.4103/ijmr.IJMR_1219_18
Skowron, K., Bauza-Kaszewska, J., Grudlewska-Buda, K., Wiktorczyk-Kapischke, N., Zacharski, M., Bernaciak, Z., et al. (2021). Nipah virus-another threat from the world of zoonotic viruses. Front. Microbiol. 12, 811157. doi:10.3389/fmicb.2021.811157
Slon-Campos, J. L., Mongkolsapaya, J., and Screaton, G. R. (2018). The immune response against flaviviruses. Nat. Immunol. 19 (11), 1189–1198. doi:10.1038/s41590-018-0210-3
Sloots, T. P., Mackay, I. M., Bialasiewicz, S., Jacob, K. C., McQueen, E., Harnett, G. B., et al. (2006) ‘Human metapneumovirus, Australia, 2001-2004’, Emerg. Infect. Dis., 12(8), pp. 1263–1266. doi:10.3201/eid1708.051239
Soldan, S. S., and González-Scarano, F. (2014). The Bunyaviridae. Handb. Clin. neurology 123, 449–463. doi:10.1016/B978-0-444-53488-0.00021-3
Spernovasilis, N., Tsiodras, S., and Poulakou, G. (2022). Emerging and Re-emerging infectious diseases: Humankind’s companions and competitors. Microorganisms 10 (1), 98. doi:10.3390/microorganisms10010098
Stein, D. A., Perry, S. T., Buck, M. D., Oehmen, C. S., Fischer, M. A., Poore, E., et al. (2011). Inhibition of dengue virus infections in cell cultures and in AG129 mice by a small interfering RNA targeting a highly conserved sequence. J. virology 85 (19), 10154–10166. doi:10.1128/JVI.05298-11
Strauss, J. H., and Strauss, E. G. (1994). The alphaviruses: Gene expression, replication, and evolution. Microbiol. Rev. 58 (3), 491–562. doi:10.1128/mr.58.3.491-562.1994
Stuver, R., Shah, G. L., Korde, N. S., Roeker, L. E., Mato, A. R., Batlevi, C. L., et al. (2022). Activity of AZD7442 (tixagevimab-cilgavimab) against Omicron SARS-CoV-2 in patients with hematologic malignancies. Cancer cell 40 (6), 590–591. doi:10.1016/j.ccell.2022.05.007
Su, S., Wong, G., Shi, W., Liu, J., Lai, A. C. K., Zhou, J., et al. (2016). Epidemiology, genetic recombination, and pathogenesis of coronaviruses. Trends Microbiol. 24, 490–502. doi:10.1016/j.tim.2016.03.003
Suribhatla, R., Starkey, T., Ionescu, M. C., Pagliuca, A., Richter, A., and Lee, L. Y. W. (2023). Systematic review and meta-analysis of the clinical effectiveness of tixagevimab/cilgavimab for prophylaxis of COVID-19 in immunocompromised patients. Br. J. Haematol. Preprint. doi:10.1111/bjh.18782
Tajudeen, Y. A., Oladipo, H. J., Oladunjoye, I. O., Yusuf, R. O., Sodiq, H., Omotosho, A. O., et al. (2022). Emerging arboviruses of public health concern in Africa: Priorities for future research and control strategies. Challenges 55, 60. doi:10.3390/challe13020060
Takashita, E., Yamayoshi, S., Simon, V., van Bakel, H., Sordillo, E. M., Pekosz, A., et al. (2022). Efficacy of antibodies and antiviral drugs against Omicron BA.2.12.1, BA.4, and BA.5 subvariants. N. Engl. J. Med. 387 (5), 468–470. doi:10.1056/NEJMc2207519
Talukder, P., and Chanda, S. (2021). RNAi technology and investigation on possible vaccines to combat SARS-CoV-2 infection. Appl. Biochem. Biotechnol. 193 (6), 1744–1756. doi:10.1007/s12010-021-03548-2
Tang, X., Wu, C., Li, X., Song, Y., Yao, X., Wu, X., et al. (2020). On the origin and continuing evolution of SARS-CoV-2. Natl. Sci. Rev. 7 (6), 1012–1023. doi:10.1093/nsr/nwaa036
Tate, J. E. (2016) ‘Global, regional, and national estimates of Rotavirus mortality in children <5 Years of age, 2000-2013’, Clin. Infect. Dis., 62, pp. S96–S105. doi:10.1093/cid/civ1013
Tegally, H., Wilkinson, E., Giovanetti, M., Iranzadeh, A., Fonseca, V., Giandhari, J., et al. (2020) ‘Emergence and rapid spread of a new severe acute respiratory syndrome-related coronavirus 2 (SARS-CoV-2) lineage with multiple spike mutations in South Africa’, MedRxiv, p. 2020.
Tegally, H., Moir, M., Everatt, J., Giovanetti, M., Scheepers, C., Wilkinson, E., et al. (2022). Emergence of SARS-CoV-2 Omicron lineages BA.4 and BA.5 in South Africa. Nat. Med. 28 (9), 1785–1790. doi:10.1038/s41591-022-01911-2
Tobolowsky, F., Burakoff, A., House, J., Marzec, N., Neumeier, A., Sparks, P., et al. (2019). Notes from the field: Hantavirus pulmonary syndrome - denver, Colorado, 2018. MMWR. Morb. Mortal. Wkly. Rep. 68 (35), 771–772. doi:10.15585/mmwr.mm6835a5
Toledo, J., Torrez, A. P., Terrazas, A., Molina Gutiérrez, J. T., Ramírez, A. M., Romero, C., et al. (2021) ‘Public health implications of a new world arenavirus outbreak that occurred in Bolivia, 2019’, Travel Med. Infect. Dis., 43, p. 102124, doi:10.1016/j.tmaid.2021.102124
Tolksdorf, B., Nie, C., Niemeyer, D., Röhrs, V., Berg, J., Lauster, D., et al. (2021). Inhibition of SARS-CoV-2 replication by a small interfering RNA targeting the leader sequence. Viruses 13 (10), 2030. doi:10.3390/v13102030
Torres-Perez, F., Wilson, L., Collinge, S. K., Harmon, H., Ray, C., Medina, R. A., et al. (2010). Sin Nombre virus infection in field workers, Colorado, USA. Colo. USA’, Emerg. Infect. Dis. 16 (2), 308–310. doi:10.3201/eid1602.090735
Tripathi, P. K., Soni, A., Singh Yadav, S. P., Kumar, A., Gaurav, N., Raghavendhar, S., et al. (2020). Evaluation of novobiocin and telmisartan for anti-CHIKV activity. Virology 548, 250–260. doi:10.1016/j.virol.2020.05.010
Tully, D., and Griffiths, C. L. (2021) ‘Dengvaxia: The world's first vaccine for prevention of secondary dengue’, Ther. Adv. Vaccines Immunother., 9, 25151355211015839, 17(9), doi:10.1177/25151355211015839
Vangeel, L., Chiu, W, Jonghe, S, Maes, P, Slechten, B, Raymenants, J, et al. (2022). Remdesivir, Molnupiravir and Nirmatrelvir remain active against SARS-CoV-2 Omicron and other variants of concern. Antivir. Res. 198, 105252. doi:10.1016/j.antiviral.2022.105252
Verbeke, R., Lentacker, I., De Smedt, S. C., and Dewitte, H. (2019). Three decades of messenger RNA vaccine development. Nano Today 28, 100766. doi:10.1016/j.nantod.2019.100766
Vilibic-Cavlek, T., Savic, V., Ferenc, T., Mrzljak, A., Barbic, L., Bogdanic, M., et al. (2021). Lymphocytic choriomeningitis-emerging trends of a neglected virus: A narrative review. Trop. Med. Infect. Dis. 6 (2), 88. doi:10.3390/tropicalmed6020088
Wagner, E., Shin, A., Tukhanova, N., Turebekov, N., Nurmakhanov, T., Sutyagin, V., et al. (2022). First indications of Omsk haemorrhagic fever virus beyond Russia. Viruses 14 (4), 754. doi:10.3390/v14040754
Waller, C., Tiemensma, M., Currie, B. J., Williams, D. T., Baird, R. W., and Krause, V. L. (2022). Japanese encephalitis in Australia - a sentinel case. N. Engl. J. Med. 387 (7), 661–662. doi:10.1056/NEJMc2207004
Wang, D., Zhu, W., Yang, L., and Shu, Y. (2021). The epidemiology, virology, and pathogenicity of human infections with avian influenza viruses. Cold Spring Harb. Perspect. Med. 11 (4), a038620. doi:10.1101/cshperspect.a038620
Wang, F. (2021). Sending out alarms: A perspective on intercellular communications in insect antiviral immune response. Front. Immunol. 12, 613729. doi:10.3389/fimmu.2021.613729
Wang, M., Cao, R., Zhang, L., Yang, X., Liu, J., Xu, M., et al. (2020). Remdesivir and chloroquine effectively inhibit the recently emerged novel coronavirus (2019-nCoV) in vitro. Cell Res. 30 (3), 269–271. doi:10.1038/s41422-020-0282-0
Weaver, S. C., Charlier, C., Vasilakis, N., and Lecuit, M. (2018). Zika, chikungunya, and other emerging vector-borne viral diseases. Annu. Rev. Med. 69, 395–408. doi:10.1146/annurev-med-050715-105122
Wikel, S. K. (2018). Ticks and tick-borne infections: Complex ecology, agents, and host interactions. Veterinary Sci. 5 (2), 60. doi:10.3390/vetsci5020060
Wilcox, B. A., and Steele, J. A. (2021). “One health and emerging zoonotic diseases,” in Handbook of global health (Berlin, Germany: Springer), 2099–2147. doi:10.1007/978-3-030-45009-0_88
Wilder-Smith, A., Gubler, D. J., Weaver, S. C., Monath, T. P., Heymann, D. L., and Scott, T. W. (2017). Epidemic arboviral diseases: Priorities for research and public health. Lancet Infect. Dis. 17, e101–e106. doi:10.1016/s1473-3099(16)30518-7
Wolfe, N. D., Dunavan, C. P., and Diamond, J. (2007). Origins of major human infectious diseases. Nature 447 (7142), 279–283. doi:10.1038/nature05775
Wollner, C. J., Richner, M., Hassert, M. A., Pinto, A. K., Brien, J. D., and Richner, J. M. (2021). A dengue virus serotype 1 mRNA-LNP vaccine elicits protective immune responses. J. virology 95 (12), 024822–e2520. doi:10.1128/JVI.02482-20
Wong, M. C., Sara, J. J. C., Nadim, J. A., and Joseph, F. P. (2020). Evidence of recombination in coronaviruses implicating pangolin origins of nCoV-2019, bioRxiv : the preprint server for biology [Preprint]. doi:10.1101/2020.02.07.939207
Woolhouse, M. E. (2002). Population biology of emerging and re-emerging pathogens. Trends Microbiol. 10 (10), S3–S7. doi:10.1016/s0966-842x(02)02428-9
Wright, D., Kortekaas, J., Bowden, T. A., and Warimwe, G. M. (2019). Rift Valley fever: Biology and epidemiology. J. general virology 100 (8), 1187–1199. doi:10.1099/jgv.0.001296
Wu, A., Peng, Y., Huang, B., Ding, X., Wang, X., Niu, P., et al. (2020). Genome composition and divergence of the novel coronavirus (2019-nCoV) originating in China. Cell host microbe 27 (3), 325–328. doi:10.1016/j.chom.2020.02.001
Xie, P.-W., Xie, Y., Zhang, X. j., Huang, H., He, L. n., Wang, X. j., et al. (2013). Inhibition of Dengue virus 2 replication by artificial micrornas targeting the conserved regions. Nucleic acid. Ther. 23 (4), 244–252. doi:10.1089/nat.2012.0405
Xie, X., Zou, J., Fontes-Garfias, C. R., Xia, H., Swanson, K. A., Cutler, M., et al. (2021). Neutralization of N501Y mutant SARS-CoV-2 by BNT162b2 vaccine-elicited sera. New York: Cold Spring Harbor Laboratory. doi:10.1101/2021.01.07.425740
Yang, J., Lau, Y. C., Wu, P., Feng, L., Wang, X., Chen, T., et al. (2018). Variation in influenza B virus epidemiology by lineage, China. China’, Emerg. Infect. Dis. 24 (8), 1536–1540. doi:10.3201/eid2408.180063
Yang, J., Qi, J. L., Wang, X. X., Li, X. H., Jin, R., Liu, B. Y., et al. (2023). The burden of hepatitis C virus in the world, China, India, and the United States from 1990 to 2019. Front. public health 11, 1041201. doi:10.3389/fpubh.2023.1041201
Yang, Y., Wu, C., Wu, J., Nerurkar, V. R., Yanagihara, R., and Lu, Y. (2008). Inhibition of West Nile Virus replication by retrovirus-delivered small interfering RNA in human neuroblastoma cells. J. Med. virology 80 (5), 930–936. doi:10.1002/jmv.21164
Yap, T. L., Xu, T., Chen, Y. L., Malet, H., Egloff, M. P., Canard, B., et al. (2007). Crystal structure of the dengue virus RNA-dependent RNA polymerase catalytic domain at 1.85-angstrom resolution. J. virology 81 (9), 4753–4765. doi:10.1128/JVI.02283-06
Young, C. A. (2021). Remdesivir gains FDA approval as first treatment for COVID-19. Pharm. Today 27, 16–17. doi:10.1016/j.ptdy.2020.12.007
Young, P. R. (2018). Arboviruses: A family on the move. Adv. Exp. Med. Biol. 1062, 1–10. doi:10.1007/978-981-10-8727-1_1
Yuen, K. Y. (2021). “Hendra virus: Epidemiology dynamics in relation to climate change,” in Diagnostic tests and control measures (Amsterdam, Netherlands: One health), 12, 100207.
Zhang, Y., Corver, J., Chipman, P. R., Zhang, W., Pletnev, S. V., Sedlak, D., et al. (2003). Structures of immature flavivirus particles. EMBO J. 22, 2604–2613. doi:10.1093/emboj/cdg270
Zhou, P., Yang, X. L., Wang, X. G., Hu, B., Zhang, L., Zhang, W., et al. (2020). A pneumonia outbreak associated with a new coronavirus of probable bat origin. Nature 579 (7798), 270–273. doi:10.1038/s41586-020-2012-7
Keywords: zoonotic virus, arboviroses, emerging and reemerging viruses, antiviral drugs, neglected tropical diseases (NTD)
Citation: Rosa-Nunes D, Lucchi DBM, Andreata-Santos R, Janini LMR and Braconi CT (2023) Lessons that can be learned from the SARS-CoV-2 pandemic and their impact on the prophylaxis and treatment development for neglected tropical arboviruses. Front. Drug Discov. 3:1176768. doi: 10.3389/fddsv.2023.1176768
Received: 28 February 2023; Accepted: 28 April 2023;
Published: 01 June 2023.
Edited by:
Caio Haddad Franco, University of Coimbra, PortugalReviewed by:
Haibo Wu, Chongqing University, ChinaCopyright © 2023 Rosa-Nunes, Lucchi, Andreata-Santos, Janini and Braconi. This is an open-access article distributed under the terms of the Creative Commons Attribution License (CC BY). The use, distribution or reproduction in other forums is permitted, provided the original author(s) and the copyright owner(s) are credited and that the original publication in this journal is cited, in accordance with accepted academic practice. No use, distribution or reproduction is permitted which does not comply with these terms.
*Correspondence: Luiz Mario R. Janini, amFuaW5pQHVuaWZlc3AuYnI=; Carla Torres Braconi, Y3Ric2FudG9zQHVuaWZlc3AuYnI=
†These authors have contributed equally to this work and share senior authorship
Disclaimer: All claims expressed in this article are solely those of the authors and do not necessarily represent those of their affiliated organizations, or those of the publisher, the editors and the reviewers. Any product that may be evaluated in this article or claim that may be made by its manufacturer is not guaranteed or endorsed by the publisher.
Research integrity at Frontiers
Learn more about the work of our research integrity team to safeguard the quality of each article we publish.