- 1Sanofi, Industriepark Höchst, Frankfurt, Germany
- 2Sanofi, Orlando, FL, United States
Introduction: 9-PAHSA belongs to a class of endogenous mammalian bioactive lipids, fatty acid esters of hydroxy fatty acids (FAHFA), that are present in circulation at nanomolar concentrations in mice and humans. Published preclinical data suggest beneficial effects of 9-PAHSA treatment on glucose metabolism as well as modulation of immune function. However, receptor molecules with high affinity towards these lipids have not been identified so far.
Methods: In a broad screen of a panel of G protein-coupled receptors (GPCRs) we discovered that 9-PAHSA displays antagonist activity with an IC50 in the micromolar range on selected chemokine receptors, namely, CCR6, CCR7, CXCR4, and CXCR5. The potential immunomodulatory activities in a human cellular model of innate immunity were then investigated.
Results and discussion: In our in vitro experiments, a weak anti-inflammatory potential for high concentrations of 9-PAHSA (10–100 µM) could be detected, as treatment reduced the LPS-induced secretion of certain chemokines, such as CXCL10, MIP-1 beta and MCP. Regarding metabolic effects, we re-investigated 9-PAHSA on glucose metabolism and insulin sensitivity in vitro and in mice confirming conclusions from our earlier study that FAHFAs lack glucoregulatory activity following an acute treatment. In conclusion, the specific interactions with a subset of chemokine receptors may contribute to weak anti-inflammatory properties of 9-PAHSA, but further studies are needed to confirm its in anti-inflammatory potential in vivo.
Introduction
Branched fatty acid esters of hydroxy fatty acids (FAHFAs) were identified as a novel class of endogenous mammalian bioactive lipids present in circulation at nanomolar concentrations in mice and humans (Yore et al., 2014). Major FAHFAs are a combination of fatty acids like palmitic acid (PA), stearic acid (SA), oleic acid (OA) or palmitoleic acid (PO) and their corresponding hydroxylated fatty acid having been termed as PAHPA, OAHPA, PAHOA, OAHOA, PAHSA, and OAHSA (Balas et al., 2018).
In insulin resistant mice and humans, the circulating concentrations of specific PAHSA isomers are significantly reduced and therefore it has been suggested that their restoration may improve insulin resistance and could have value in the treatment of type 2 diabetes (Yore et al., 2014). A second study suggested that acute and chronic treatment with PAHSAs reduced ambient glycemia, improved glucose tolerance and improved insulin sensitivity in DIO mice, mainly by suppressing endogenous glucose production and by augmenting insulin-stimulated glucose uptake in muscle and heart (Zhou et al., 2019). Mechanistically, the resolution of insulin resistance has been attributed to activation of molecular GPCR targets such as GPR40 and GPR120 and reduction of the low-grade tissue inflammation observed under insulin resistant conditions (Yore et al., 2014; Syed et al., 2018a; Pflimlin et al., 2018). However, we could not confirm these beneficial effects related to glucose metabolism after exogenous PAHSA treatment in different cellular in vitro models as well as in obese and insulin resistant mice (Pflimlin et al., 2018). Methodological differences between the studies, in particular the vehicle used to administer PAHSAs, were considered as a potential underlying reason that may have masked the biological effects of PAHSA treatment (Syed et al., 2018b; Kuda, 2018). Further recent publications provide evidence that PAHSAs reduce cellular senescence of pancreatic beta cells and cardiovascular effects and promotes autophagy in cardiovascular complications in mice (Wang et al., 2021; Rubin de Celis et al., 2022)
In parallel to investigations on metabolic effects, it has been shown that PAHSAs exhibit anti-inflammatory properties. Yore et al. demonstrated that 9-PAHSA inhibited LPS-induced CD80, CD86, CD40, and MHC II expression in an in vitro system and reduced LPS-induced IL-12, IL-1β and TNFα secretion in DIO mice (Yore et al., 2014). Further, the percentage of adipose tissue (AT) macrophages expressing TNFα and IL-1β in high-fat diet (HFD)-fed mice was significantly reduced after 3 days of oral treatment with 9-PAHSA (Yore et al., 2014). Syed et al. reported evidence that treatment with 5- and 9-PAHSA reduced adipose tissue inflammation in obese mice fed a high fat diet (Syed et al., 2018a). In addition, Lee et al., 2016 have shown that 5- and 9-PAHSA treatment regulated both innate and adaptive immune responses in a murine colitis model by lowering colonic T-cell activation and reducing pro-inflammatory cytokine and chemokine expression
All published phenotypic results, however, do not provide strong evidence for high-affinity interactions of PAHSA derivatives towards known receptor molecules. We therefore broadly screened panels of G-protein coupled receptors on agonistic and antagonistic efficacies of 5- and 9-PAHSA based on that such derivatives have been described as agonists particularly of GPR40 and GPR120 at micromolar (μM) concentrations GPCRs (Yore et al., 2014; Cooper et al., 2017; Syed et al., 2018a; Pflimlin et al., 2018). Our broad screening included agonistic efficacy of 5- and 9-PAHSA on orphan GPCR’s, GPCRs without known ligands, to identify whether PAHSA’s may act as hitherto unidentified ligands. A second panel of GPCRs (GPCRmax) was comprised of cell lines expressing GPCRs with known ligands (de-orphaned GPCRs). These cell lines were used to evaluate the agonistic and antagonistic activity of both lipids and their activities were compared to established agonists and antagonists for these receptors. Surprisingly, we identified a group of chemokine receptors antagonized with higher potency by 9-PAHSA than 5-PAHSA.
To confirm an anti-inflammatory mode of action, we then tested 5- and 9-PAHSA in a unique, highly predictive human cellular model of innate immunity termed MIMIC® PTE (Higbee et al., 2009; Luna et al., 2015; Luna et al., 2018). This assay system has been developed and used to detect subtle differences in immune activation between biologics (monoclonal antibodies), vaccines (Toll-like receptor [TLR] agonists), branded and US-generic enoxaparins and insulins (Ma et al., 2010; Luna et al., 2015; Luna et al., 2018). This model of human innate immunity recapitulates the derivation of dendritic cells (DCs) under conditions designed to replicate the physiologic migration of monocytes through the vasculature into tissue sites, where they differentiate into DCs. In its currently established format, primary human umbilical vascular endothelial cells (HUVECs) promote the differentiation of blood monocytes into antigen presenting cells (APCs) in the absence of exogenous growth factors or cytokines.
Material and methods
5- And 9-PAHSA supply
For all experiments, racemic 5- and 9-PAHSA was used (chemical structure displayed in Supplementary Figure S1) and was synthesized at Sanofi with a chemical route that was described in detail elsewhere (Pflimlin et al., 2018). In selected GPCR experiments, additionally reference material supplied by Cayman Chemical Company (Ann Arbor, Michigan, USA) was used delivering identical results.
Screening 5- and 9-PAHSA against a panel of several human G protein-coupled receptors (GPCRs)
To determine the interaction of 5- and 9-PAHSA with a broad panel of GPCRs, existing cell lines with human orthologues and assay technologies established at Eurofins-DiscoverX (Fremont, CA, USA) were used. The DiscoverX technology utilized in the GPCR activity measurement is based on beta-arrestin recruitment. The ß-arrestin assay technology has been chosen to cover the broadest possible set of GPCRs with known or orphan ligands. The activation of all GPCRs in this panel was monitored in a homogenous, non-imaging assay format using enzyme fragment complementation with β-galactosidase (β-Gal) as functional reporter. The enzyme is split into two inactive complementary portions (EA for Enzyme Acceptor and ED for Enzyme Donor) expressed as fusion proteins in the cell. EA is fused to β-arrestin and ED is fused to the GPCR of interest. Activation of GPCRs results in recruitment of EA- β-arrestin to the ED-GPCR complex and subsequent formation of active β-galactosidase, which can be monitored by chemiluminescent substrates. To determine agonistic efficacy of PAHSAs at orphan and ligand-activated GPCRs, individual cell lines were expanded from freezer stocks according to standard procedures and thereafter seeded in a total volume of 20 μL into white walled, 384-well microplates and incubated at 37°C prior to testing. PAHSA stock solutions were prepared freshly in ethanol and intermediate diluted 5x in assay buffer. A small aliquot, 5 μL of these intermediate/stepwise dilutions was then added to cells and incubated further for 90–180 min, depending on the GPCR cell line. These different response times were selected based on pilot studies performed with each individual GPCR cell line. The final concentration of ethanol in the assay buffer did not exceed 1%. Complementation to form enzymatically active β-galactosidase was assessed by the addition of 12.5 μL proprietary enzyme substrate solution (PathHunter® detection reagent cocktail) followed by 1 h incubation at room temperature. For some GPCRs that exhibit low basal signal, activity was detected using a high sensitivity detection reagent (PathHunter® Flash Kit) to improve assay performance. For these assays, an equal volume (25 μL) of detection reagent was added to the wells and incubated for 1 h at room temperature. Chemiluminescence was detected with an Envision™ instrument (PerkinElmer). Percentage activity was calculated using the following formula:
% Activity = 100% × (mean RLU of test sample—mean RLU of vehicle control)/(mean RLU of vehicle control).
GPCRs with known ligands, were also tested to determine the antagonistic activities of PAHSAs. In pilot studies, each individual GPCR, was tested with its agonistic ligand at several concentrations to obtain a concentration-response curve. In the antagonistic mode, cells were pre-incubated with 5- or 9-PAHSA for 30 min at 37°C. Thereafter, the GPCR-specific agonist was added at a concentration to obtain a 80% of maximal activation (EC80); the cells were then further incubated for 90–180 min before β-galactosidase formation was detected by chemiluminescence. Antagonist-mediated percentage inhibition was calculated using the following formula:
% Inhibition = 100% × (1- (mean RLU of test sample - mean RLU of vehicle control)/(mean RLU of EC80 control - mean RLU of vehicle control)).
Measurement of GPR40 (FFAR1) activation
9-PAHSA was reported as an agonist of GPR40. Under the gene name FFAR1 this GPCR was included in the screened receptor panel (Syed et al., 2018a; Pflimlin et al., 2018). Independent of these screening efforts using a ß-arrestin endpoint, we sought to confirm agonism of GPR40 by 5- and 9-PAHSA using a second, G protein-dependent endpoint. A HEK293 cell line overexpressing GPR40 and chimeric Gα16 protein was cultivated and used in a calcium flux assay as described previously (Hansen and Brauner-Osborne, 2009; Pflimlin et al., 2018). Briefly, cells expressing GPR40 were seeded in poly-D-Lysine coated 96-well plates at a density of 40,000 cells per well and grown overnight at 37°C and 5% CO2 in DMEM medium containing Glutamax, 10% fetal calf serum, 1% non-essential amino acids, 1 μg/mL puromycin and 250 μg/mL Zeocin™. The following day, cells were incubated with the calcium sensitive dye Fluo-4, washed and incubated with serial dilutions of 5- or 9-PAHSA. In parallel, TAK-875 was used as a reference control (Kaku et al., 2015; Ito et al., 2016). For concentration-response curves, a TAK-875 stock solution (10 mM in DMSO) was further diluted with 100% DMSO prior to a final dilution step 1:100 in incubation medium. 5- and 9-PAHSA stock solutions in 100% ethanol were prepared and used for the dilutions keeping a constant final ethanol concentration of 1%. Each concentration was tested with overall n = 4 biological replicates. The difference between minimum and maximum calcium sensitive fluorescence was fitted using GraphPad Prism (log agonist versus response algorithm with four parameters and a variable slope).
Preparation of peripheral blood mononuclear cells
Apheresis blood products were collected from donors at the OneBlood blood bank (Orlando, FL, United States). The study protocol and donor program were reviewed and approved by Chesapeake Research Review Inc. (Columbia, MD, United States). At the time of the collection, peripheral blood mononuclear cells (PBMCs) from healthy donors were enriched by Ficoll density gradient separation and cryopreserved in DMSO-containing freezing media according to standard laboratory procedures. PBMCs were chosen at random from our pool for inclusion in each experiment.
Cell migration assay
Cell migration was performed in 24-well Boyden-Chamber trans-well setup with 5 μm pore size filters (EMD Millipore corp., Bellerica, MA). Endothelial cells EA.hy926 (Cat# CRL-2922, ATCC, Manassas, VA) were seeded in the apical compartment, incubated to confluency at 37°C 5% CO2. CD4+ T-cells were isolated from PBMCs from 6 donors using Easy Sip Human CD4 T-cell isolation kit (cat# 17952, Stemcell techologies, Tukwila, WA), pre-incubated with 5-PAHSA or 9-PAHSA in serum free media at concentrations of 10 uM or 1 uM, and finally added in the apical inserts of transwell plates at 5.105 cell per well. Recombinant human CCL19 (cat# 361-MI-025, R&D Systems, Mineapolis, MN) was added, in serum free media, to the basal compartments at a concentration of 300 ng/mL except for the mock conditions. Selection of this concentration was taken from previously published data (Picchio et al., 2008). Migration was stopped after 2 h, and cells migrated to the basolateral lower compartment counted using Celigo image cytometer (Nexcelom Bioscience LLC, Lawrence, MA). A migration index was established by the ratio of number of cells migrated in each condition over the number of cells migrated in CCL-19 condition, and therefore an index value below 1 will indicate an inhibition, while an index value greater than one will indicate an enhancement of cell migration. The statistical analysis was done using Mann-Whitney U test.
MIMIC® PTE assay
The MIMIC® PTE construct was assembled on a robotic line using a published methodology (Ma et al., 2010). Briefly, endothelial cells were grown to confluence on top of a collagen matrix (Advanced Biomatrix, San Diego, CA, United States). Thereafter, donor PBMCs prepared from frozen stocks were added to the assay wells. After 90 min incubation, non-migrated cells were washed away, and 5 or 9-PAHSA compounds were added at a concentration of 0.01, 0.1, 1, 10, and 100 µM. Both PAHSA compounds were tested by themselves or in combination with 10 ng/mL of lipopolysaccharide (LPS) in order to evaluate their immunosuppressive potential. LPS (10 ng/mL) was used as a positive control in these assays. The culture supernatant was harvested after 48 h and analyzed for cytokines/chemokines via a multiplex assay.
Cytokine/chemokine analysis
MIMIC® PTE culture supernatants were analysed using a Milliplex® human 12-plex multi-cytokine detection system (Millipore) as per the manufacturer’s protocol. The kit includes the following cytokines: IFN-α2, IFN-γ, IL-1β, IL-6, IL-8, IL-10, IL-12p40, CXCL10CXCL10, MCP-1, MIP1-β, RANTES and TNF-α. Analyte concentrations were calculated based on relevant standard curves using the Bio-Plex manager software.
Flow cytometry
MIMIC® PTE-derived cells were washed with PBS and stained with Live-Dead Aqua (InvitroGen, Carlsbad, CA, United States) for 20 min on ice. After washing and IgG-Fc blocking (mouse IgG1; Sigma-Aldrich), the cells were incubated with a cocktail of fluorochrome-labeled mAbs specific for non-myeloid lineage cells and the immune markers CD3, CD19, CD14, HLA-DR, CD86, CD83 and CD25 (BD Biosciences, San Jose, CA, United States). Thereafter, the cells were washed with buffered media and acquired on a BD Fortessa flow cytometer equipped with BD FACS Diva software (BD Biosciences). Data analysis was performed using FlowJo software (Tree Star, Ashland, OR, United States).
Metabolic in vitro and in vivo studies
2-deoxyglucose uptake was measured in in vitro experiments using differentiated human subcutaneous adipocytes as well as in differentiated 3T3-L1 cells. In vivo, oral glucose tolerance tests were performed in obese and insulin resistant diet-induced obese (DIO) mice. Before the oral glucose bolus, mice were either gavaged with vehicle, 9-PAHSA (45 mg/kg) or, as positive control groups, with metformin (LKT Laboratories Inc., St Paul, MN, United States; dose: 300 mg/kg) or with a SGLT-2 inhibitor (dapagliflozin, Forxiga®, AstraZeneca, Cambridge, United Kingdom; dose: 3 mg/kg; tablets were crushed and re-dissolved in vehicle). The experiment was conducted twice, with all compounds being dissolved in either olive oil (Sigma-Aldrich, Taufkirchen, Germany, catalog No. O1514) or in 50% PEG400, 0.5% Tween-80% and 49.5% water (“PEG/Tween vehicle”). The detailed description of the applied methods and procedures for these metabolic studies can be found in the Supplementary Datasheet S1.
Ingenuity pathway analysis
In order to raise a hypothesis related to the pathways and functions modulated by exogenous 9-PAHSA treatment, the results of our measurements on activation or antagonism of GPCRs and cytokines were combined (see Supplementary Table S2) and data were further interrogated through the use of Ingenuity Pathway Analysis (Ingenuity, Qiagen) as initially described in Krämer et al., 2014. Briefly, the set of antagonized GPCRs as well as the measured effects on CXCL10 were uploaded into the application. Each identifier was mapped to its corresponding object in Ingenuity’s Knowledge Base. Subsequently, a network was then algorithmically generated based on the connectivity of these entities. All six confirmed entities were then interrogated on interactions associated to functions and diseases. In a final step, a molecule activity prediction algorithm was applied to visualize a putative overall downstream effect of antagonism in this network of molecules and functions, to predict how GPCRs antagonized by 9-PAHSA modulate biological functions and selected diseases.
Statistical analysis
Statistical analyses were performed using GraphPad Prism (GraphPad Prism®, Version 8.02, La Jolla, CA, United States) or Microsoft® Excel. Statistical comparisons between three or more groups were performed using non-parametric ANOVA followed by Kruskal–Wallis multiple comparisons tests and non-parametric Mann Whitney t-test for two groups data. While most data are expressed as mean ± standard error of the mean (SEM), cytokine secretion, was represented using geometric mean ± 95% confidence interval (CI). p-values < 0.05 were considered as statistically significant. In the respective figures, asterisks indicate statistically significant differences compared to controls (*p < 0.05, **p < 0.01, ***p < 0.001).
Results
Agonistic and antagonistic activity of 5- and 9-PAHSA on a broad panel of G protein-coupled receptors
We screened a broad panel of G protein-coupled receptors (GPCRs) using ß-arrestin recruitment as the endpoint to identify potential novel binding partners of PAHSAs. Overall, 73 cell lines with recombinantly expressed orphan GPCRs were incubated with either 5-PAHSA or 9-PAHSA at a single concentration of 30 µM for 60–120 min and ß-arrestin recruitment tested by galactosidase complementation with subsequent chemiluminescence detection (Supplementary Tables S1A, B). 5- and 9-PAHSAs did not stimulate these GPCRs, as they exhibited agonistic efficacy <25% indicating very weak or no agonistic activity at these receptors (100% refers to the baseline signal which can be detected).
Next, the effects of PAHSAs were tested using cell lines expressing de-orphaned GPCRs in the absence or presence of their agonist ligands. (Figure 1; Supplementary Tables S2A, B, 3A, B). In this agonism mode, all 168-cell lines overexpressing GPCRs were incubated with 5- or 9-PAHSA at 30 µM for 60–120 min. Both 5- and 9-PAHSA produced agonist efficacy of <25% of the maximal effect produced by known ligands of the GPCRs. These results indicate that 5- and 9-PAHSA display very weak or no agonist activity at these selected receptors. In the case of GPR120, 9-PASHA increased the signal ∼23% above baseline, indicating very weak agonism at GPR120 in this assay format (Supplementary Tables 2A,B).
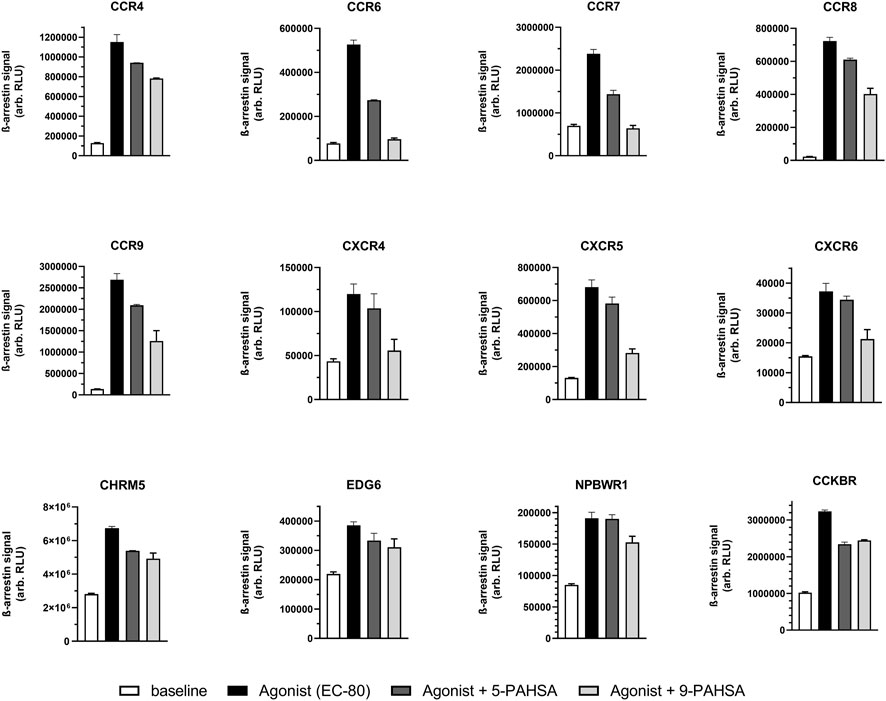
FIGURE 1. 5-PAHSA and 9-PAHSA displayed antagonistic efficacy only in the displayed subset of all GPCRs tested. Antagonistic efficacy was more pronounced with 9-PAHSA compared to 5-PAHSA. In three independent replicates, cells were pre-incubated with 5- or 9-PAHSA (30 µM) for 30 min at 37°C. The vehicle concentration was 1% ethanol. Thereafter, the GPCR-specific agonist was added at a concentration to obtain 80% of maximal activation (EC80); the cells were then further incubated for 90–180 min before ß-arrestin recruitment was detected by chemiluminescence. Antagonist-mediated percentage inhibition was calculated as described in the text.
Then, we tested the ability of both PAHSAs to block the response of known agonist ligands for the 168 de-orphaned GPCRs (Supplementary Tables 3A, B). In pilot studies, EC50 and EC80 concentrations were determined for the known ligands (Supplementary Table S4). Cells were pre-incubated with either 5- or 9- PAHSA before addition of known ligands at a concentration that elicited an EC80-response. We observed that 9-PAHSA antagonized the agonistic response at 12 of 168 GPCRs tested (Figure 1; Supplementary Table S3B). Overall, 9-PAHSA displayed stronger antagonism at these GPCRs than 5-PAHSA.
In a subsequent study, we determined concentration-dependent antagonistic efficacy of 9-PAHSA on those GPCRs which were blocked most strongly in the screening panel (see Figure 2A). Antagonism on selected CCRs (CCR6 & CCR7) and CXCR (CXCR4) receptors could be confirmed with IC50 values in the µM range. The derivative 9-PAHSA displayed IC50 values of 1.7 µM for CCR6, 3.2 µM for CCR7, 3.9 µM for CXCR4 and 19 µM for CXCR5, respectively.
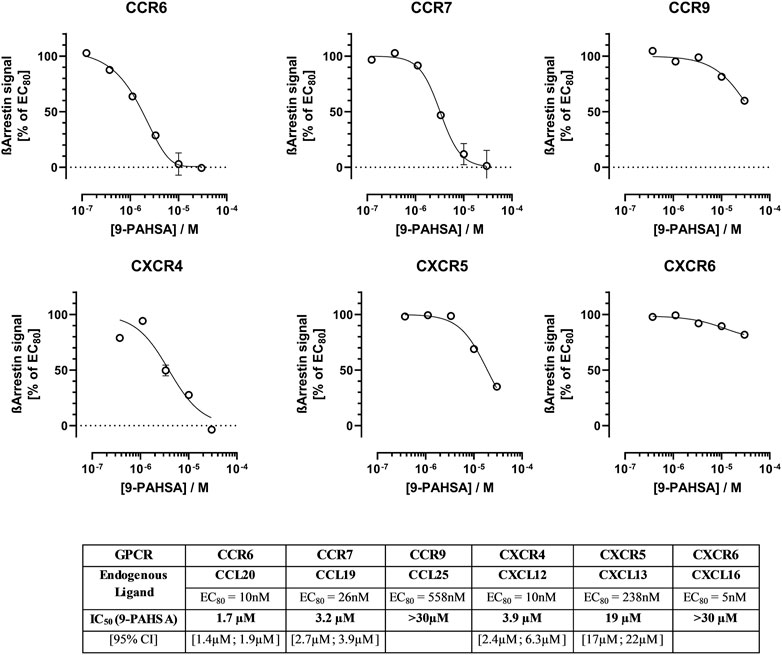
FIGURE 2. Concentration-dependent antagonistic effect of 9-PAHSA at selected immunomodulatory GPCRs. In three independent replications, cells were pre-incubated with different concentrations of 9-PAHSA for 30 min at 37°C. The vehicle concentration was kept constant at 1% ethanol. Thereafter, the GPCR-specific agonist was added at a concentration to obtain 80% of maximal activation (EC80); the cells were then further incubated for 90–180 min before ß-arrestin recruitment was detected by chemiluminescence. 9-PAHSA-mediated percentage inhibition was calculated as described in the text.
With respect to GPR40 (FFAR1) a GPCR with known metabolic functions, 5-PAHSA and 9-PAHSA failed to elicit agonistic or antagonistic responses in the ß-arrestin recruitment assay (Supplementary Tables S2A, B, 3A, B, see values for FFAR1). On the other hand, when 5- and 9-PAHSA were tested on a different endpoint, intracellular calcium, 9-PAHSA, but not 5-PAHSA, exhibited a low potency at raising intracellular calcium levels and displayed an EC50 of 6.2 µM [95% CI 4.9–7.6 µM]. In comparison, a known and potent GPR40 agonist with clinical efficacy, TAK-875, displayed more than 150-fold greater potency at stimulating GPR40 with an EC50 of 37.2 nM [95CI, 30–46 nM] (Figure 3). Overall, the agonist potency on GPR40 seems to be too low to elicit reasonable metabolic effects in experimental pharmacology models.
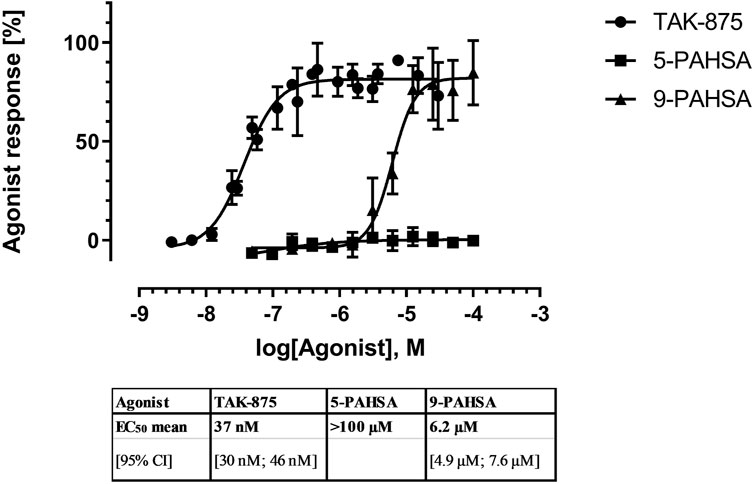
FIGURE 3. Concentration-dependent agonistic effect of 9-PAHSA on intracellular calcium in cells expressing recombinant human GPR40. In three independent replications, cells loaded with the calcium sensitive dye Fluo-4, washed, and then incubated with serial dilutions of 5- or 9-PAHSA. In parallel to PAHSA, TAK-875 was used as a reference agonist with proven clinical efficacy.
To provide a first confirmation on antagonistic activity of 9-PAHSA on their interaction with selected inflammatory GPCR under naïve, non-recombinant conditions, we focused first on a primary T-cell model, in which endogenously expressed CCR7 modulates cellular migration. Human CD4+ T-cells were isolated from six different human blood donors and tested on their ability to migrate through a confluent endothelial cell monolayer induced by the CCL19, the endogenous ligand for CCR7 (see Figure 4). T-cells were pre-incubated in serum free media. CCL19 was added to the basal compartment at a concentration of 300 ng/mL. In contrast 5- and 9-PAHSA were supplemented at concentrations of 1 or 10 µM to the apical compartments. After 2 h, CD4 T-cells that migrated through the endothelial cell layer to the basal compartment were counted. At a concentration of 10 µM, co-incubation with 9-PAHSA decreased significantly (p < 0.05), the number of cells migrating by −27%. In contrast, 5-PAHSA did not modulate the CCL19 induced CD4+ T-cells migration.
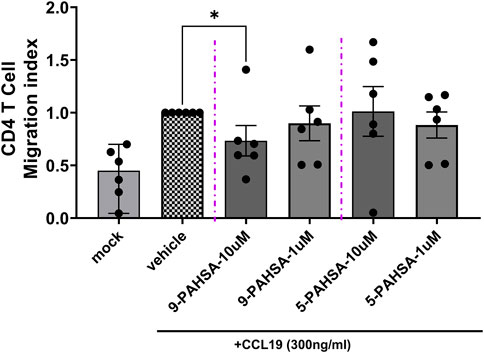
FIGURE 4. 9-PAHSA reduces CCL19 induced human CD4+ T-cell populations. Migration of human CD4+ T-cells was performed in 24-well Boyden-Chamber assay. Six donors of CD+4 T-cells were tested independently. Shown are the means from such independent replications normalized to the positive control (vehicle, CCL19 alone). All conditions contained CCL19 (300 ng/ml) in the lower compartment except the mock conditions.
Efficacy of PAHSA’s in a human cellular model of innate immunity (MIMIC® PTE)
Certain classes of lipids have been suggested to modulate inflammation and inhibit the production of proinflammatory cytokines (Oh et al., 2010; Wang et al., 2019). Hence, we evaluated the anti-inflammatory potential of 5- and 9- PAHSA by measuring their ability to inhibit a LPS pre-activated MIMIC® PTE construct like the LPS pre-activation reported here (Luna et al., 2015; Luna et al., 2018). The concentration for LPS was determined in a pilot experiment and according to those previous studies. 5- and 9-PAHSA were tested for their ability to alter cell viability in 48 h reverse transmigrated cells harvested from MIMIC® PTE assays. Both compounds were tested alone and in combination with LPS (10 ng/mL) as shown in Figures 5A,B. To facilitate the analyses, in this figure we normalized the mock control (no antigen) to 100% of viability to compare the impact of the compounds based on % metric. 5- and 9-PAHSA both displayed a non-significant slightly reduced reverse transmigrated cell viability (about 10%–15%), independent of treatment concentration (0.01 µM–100 µM) when compared to the no antigen control that was normalized to 100%. When tested along with LPS (10 ng/mL), a higher level of reduction in cell viability was observed for both compounds and in this case, this effect was observed in a concentration-dependent manner. Interestingly, LPS alone did not reduce cell viability, but only the combination of LPS and mainly, 9-PAHSA led to a stronger reduction in cell viability (about 35%–40% at the highest dose, p < 0.001, Figure 5B). With a low magnitude, 5-PAHSA also induced a significant reduction in cell viability at 100uM (p < 0.05, Figure 5A). While not included in this figure, the assay historical positive control (LPS + R848) usually reduced cell viability to 70%–80% in the MIMIC® PTE.
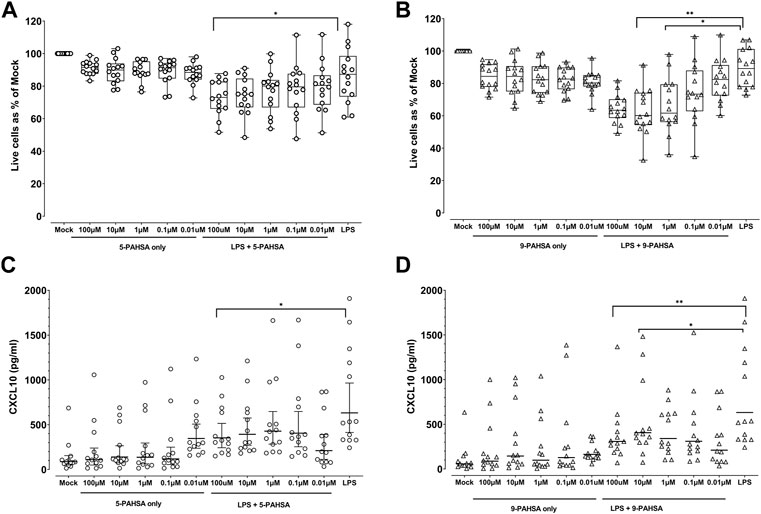
FIGURE 5. 9-PAHSA displayed a weak efficacy in the MIMIC® PTE model. (A) (5-PAHSA) and (B) (9-PAHSA): Cells were treated with controls, 5-PAHSA (A) or 9-PAHSA (B); (concentration in µM), in the absence or presence of LPS (10 ng/mL), at the indicated concentrations for 48 h. Thereafter, the cell viability was measured via flow cytometry. The mock condition for each donor was normalized to 100% and the treatment conditions were calculated against this value, n = 14 donors. LPS (10 ng/mL) was added 1 h before adding the compounds. LPS only condition (10 ng/mL) was also treated in a similar fashion. (C,D): MIMIC® PTE cultures were treated with different concentrations of 5- (C) or 9-PAHSA (D), (concentration in µM), in the absence or presence of LPS (10 ng/mL) for 48 h. Thereafter, the culture supernatants were collected and evaluated for the secretion of different cytokines by multiplex assay. The plotted values, CXCL10 were presented as geometric mean with 95% CI, n = 13, for CXCL10 secretion. LPS (10 ng/mL) was added 1 h before adding the compounds. LPS only condition (10 ng/mL) was also treated in a similar fashion. *p < 0.05, **p < 0.01, ***p < 0.001.
5- and 9-PAHSA fail to significantly affect antigen-presenting cell activation
Another important element of innate immune activation is to measure the activation status of APC. The APC activation status is measured by the cell surface expression of co-stimulatory markers, such as CD86, CD83, CD25, CD14, on top of HLA-DR + APC subpopulations (Collin et al., 2013). Both fatty acid derivatives, 5- and 9-PAHSA, were tested for their ability to alter the APC activation status of 14 donor PBMC samples in the 48 h MIMIC® PTE assays. While we have evaluated CD14, CD25, CD83 and CD86, we represented CD86 and CD83 as major co-stimulatory markers to be analysed (Supplementary Figure S2). As shown in this figure, 5-PAHSA and 9-PAHSA - when used alone or in combination with LPS - failed to induce statistically significant modifications on CD83 (top graphs) or CD86 (bottom graphs) expression for APC activation status. The derivative 9-PAHSA produced a non-significant 20% reduction in LPS-dependent upregulation of CD86. Other APC markers such as, CD14 and CD25 did not show any relevant changes to report (data not shown).
PAHSAs induce a slight reduction in the secretion of CXCL10 (IP-10)
In the next step of our evaluation, we assessed the ability of 5- and 9-PAHSA to modify cytokine/chemokine secretion individually, or in the presence of LPS. Of the 12 different cytokines and chemokines measured, the PAHSAs modulated secretion only of CXCL10. Incubation with 5- and 9-PAHSA resulted in a trend for minor reduction, when compared to the no antigen control (Figures 5C,D). When both PAHSAs were tested together with LPS (10 ng/mL), 9-PAHSA induced a higher reduction of CXCL10 secretion than 5-PAHSA. Moreover, the 2 highest concentrations of 9-PAHSA (10 μM and 100 µM) induced 2- and 3.7-fold reduction of LPS-induced CXCL10 secretion, respectively (p < 0.05, p < 0.001). In contrast, only the highest concentration of 5-PAHSA (100 µM) induced a statistically significant 1.8-fold reduction in LPS-induced secretion of this chemokine (p < 0.05, Figures 5C,D). This indicates differences in the potency of 9-PAHSA versus 5-PAHSA for LPS-induced CXCL10 secretion.
Antagonistic activity on CCR isoforms, CXCR isoform and CXCL10 predicts inactivation of 5- and 9-PAHSA at a variety of G protein-coupled receptors
An extended analysis of the interaction between the affected CCR and CXCR isoforms and CXCL10 was performed. Using the Ingenuity pathway knowledge those entities were then associated with functions and diseases (Supplementary Figure S3). Several cellular immune functions are known to be affected downstream of the selected receptors and CXCL10, including chemotaxis of leucocytes and homing of T-lymphocytes. A molecular activity prediction algorithm indicates that joint antagonism of CCR7, CCR9, CXCR4, and CXCR5 together with reduction of CXCL10 finally may inhibit several of these functions. Interestingly, by the application of known literature findings covered by the Ingenuity knowledge database and respective algorithms, blocking of the chemokine receptors and CXCL10 did not predict a modulation of diseases like rheumatoid arthritis or insulin-dependent diabetes mellitus.
5- or 9-PAHSA do not increase glucose uptake in human and mouse 3T3-L1 adipocytes
We supplemented our more inflammatory studies with in vitro and in vivo studies further investigating efficacies of PAHSA in metabolic control. First, the effect of 9-PAHSA on insulin sensitivity in adipocyte cell models was tested using in vitro differentiated human subcutaneous adipocytes or mouse 3T3-L1 cells. After 48 h pre-incubation with 9-PAHSA (20 µM) or vehicle, 14C-glucose uptake was stimulated with various concentrations of human insulin in the continued presence of 9-PAHSA or vehicle. In both adipocyte models, glucose uptake could be stimulated concentration-dependently by insulin (Supplementary Figures S4A, B). However, treatment with 9-PAHSA failed to affect the absolute magnitude of glucose uptake (maximal efficacy) elicited by insulin or the potency of insulin for stimulating glucose uptake (EC50 value: a measure of half-maximal stimulation by insulin) (Supplementary Figure S4C).
The type of vehicle utilized does not mask the lack of efficacy in DIO mice after acute treatment with 9-PAHSA
Acute treatment with 9-PAHSA did not elicit a beneficial effect on oral glucose tolerance in DIO mice irrespective of whether PEG/Tween or olive oil was used as a vehicle for administering PAHSA (Supplementary Figures S5A, B, D, E). In contrast, oral glucose tolerance in obese and insulin resistant DIO mice was significantly improved by the well-known anti-diabetic drugs metformin and dapagliflozin regardless of the vehicle used to administer these compounds (Supplementary Figures 5A, D). These findings are not in line with the hypothesis that an oral gavage with olive oil per se improves oral glucose tolerance to an extent that cannot be further improved by established agents as raised by Syed and colleagues (Syed et al., 2018b).
As expected, the obese DIO mice were insulin resistant as the vehicle-treated DIO mice displayed significantly higher baseline and glucose-stimulated plasma insulin concentrations compared to the lean control mice (Supplementary Figure S5C). The oral glucose bolus resulted in a significant increase in circulating plasma insulin in all treatment groups (Supplementary Figures S5C,F). Further, acute treatment with metformin in olive oil as a vehicle (Supplementary Figure S5C, p < 0.01) as well as metformin and dapagliflozin in PEG/Tween as a vehicle (Supplementary Figure S5F) reduced glucose-stimulated insulin secretion compared to vehicle-treated DIO mice, and the effect was statistically significant for dapagliflozin treatment (p < 0.001). In contrast, acute treatment with 9-PAHSA, regardless of the vehicle used, did not significantly affect glucose-stimulated insulin secretion during the oGTTs. Moreover, the glucose excursion during insulin tolerance tests after oral gavage with 9-PAHSA in DIO mice was not significantly different to controls, regardless of vehicle used.
Discussion
Branched fatty acid esters of hydroxy fatty acids (FAHFAs), including PAHSAs, have been recognized as novel bioactive lipids, which are present in the circulation and tissues of mammals as well as in different plants (Yore et al., 2014; Pflimlin et al., 2018; Kolar et al., 2019; Liberati-Cizmek et al., 2019; Rodriguez et al., 2019). In our studies, we discovered new molecular interactions between 9-PAHSA and a set of GPCRs that play an important role in inflammation, namely, CCR7, CCR9, CXCR4, and CXCR5. PAHSAs stimulated these GPCRs with potencies in the micromolar range that are at least comparable to previously described interactions between 9-PAHSA and metabolic GPCRs like GPR40 and GPR120. A wide panel of other GPCRs were neither agonized nor antagonized by 9-PASHA, hinting to a specific interference to CCR6, CCR7, CXCR4, and CXCR5. However, the intricacies of these new interactions need to be investigated further.
Several FAHFAs have been shown to possess interesting properties, including anti-inflammatory effects (Yore et al., 2014; Tortosa-Caparros et al., 2017; Li et al., 2018). In line with this proposal, data from a chemically induced colitis mouse model suggest that PAHSA treatment prevents mucosal damage and could protect against colitis through regulation of innate and adaptive immune responses (Lee et al., 2016). Furthermore, it has been shown recently that PAHSAs delayed the onset of T1D in NOD mice by attenuating immune responses through reduced T and B cell infiltration and CD4+ and CD8+ T-cell activation, while increasing Treg activation (Syed et al., 2019). Interestingly, Kuda et al. also reported anti-inflammatory properties for 9-PAHSA and another FAHFA derivative (docosahexaenoic acid-derived FAHFA) demonstrating a compound-dependent inhibition of the secretion of proinflammatory cytokines in a LPS-induced mouse macrophage cell line (Kuda et al., 2016). These data suggest that the anti-inflammatory properties are linked to the general chemical structure of FAHFAs and may not be unique to 9-PAHSA. In agreement with this hypothesis, Wang et al. revealed in an analysis of the structure-activity relationship for 9-PAHSA that modifications of the carbon chain lengths of the 9-hydroxystearic acid portion or palmitic acid hydrocarbon chain resulted in analogues that maintained or even increased the anti-inflammatory activity (Wang H. et al., 2019). Similarly, and confirmatory to our findings, a recent report confirms anti-inflammatory effects of 9-PAHSA. The authors have provided data detailing that 9-PAHSA along with other FAHFAs attenuated, e.g., LPS-induced TNF-α or IL-6 secretion in bone marrow–derived macrophages as well as gene expression of LPS-induced chemokines CCL-l2, CCL-3, and CCL-5. Interestingly, this study also shows that in principle, FAHFAs with lower branching of unsaturated acyl chains from the carboxylate head group are more likely to be anti-inflammatory (Aryal et al., 2021).
Despite this rather broad initial characterization of the anti-inflammatory potential of PAHSA, receptor interaction studies with those fatty acid derivatives on a molecular level are clearly under-represented leaving a gap in understanding the specific pharmacology. With respect to 9-PAHSA, the anti-inflammatory properties have been correlated to be–at least partially–dependent on its GPR120 agonist activity (Lee et al., 2016). We previously confirmed that 9-PAHSA exhibited agonism at GPR120 with an IC50 of 19 µM, albeit the potency for activating GPR120 and the maximal effect were significantly weaker compared to previously published data (Pflimlin et al., 2018) suggesting that mechanisms other than activation of GPR120 play a role in mediating anti-inflammatory effects. In our unbiased and broad screen of 5- and 9-PAHSA against more than 200 GPCRs with known ligands or orphan GPCRs, especially 9-PAHSA displayed antagonistic activity for a particular class of GPCRs, namely, CCR6, CCR7, CXCR4, and CXCR5. These GPCR’s all belong to a classes of conventional chemokine receptor (cCKRs) family, which are directly involved in the modulation of immune function (Charo and Ransohoff, 2006; Hughes and Nibbs, 2018). 9-PAHSA blocks these chemokine receptors at single digit micromolar concentrations indicating that high pharmacological levels of PAHSAs would be needed to exert anti-inflammatory effects in vivo. All chemokine receptors blocked by 9-PAHSA have been implicated in cellular immune-modulatory functions (see Supplementary Figure S3), but not directly in metabolic responses.
CCR6 is expressed on memory T-cells, dendritic cells and Th17 cells (Acosta-Rodriguez et al., 2007) (Wang Y. et al., 2019). In addition, CCR7 is a highly important receptor with a role in trafficking of B and T lymphocytes and dendritic cells to and across high endothelial venules and positioning those cells correctly in T-cell zones of secondary lymphoid organs (Yoshida et al., 1997). Recent data also suggest an involvement in the activation of bone marrow adaptive immunity in T2D (Santopaolo et al., 2021). Finally, CXCR4 has a wide cellular distribution, with expression on most immature and mature hematopoietic cell types (e.g., neutrophils, monocytes, T and B cells, dendritic cells, Langerhans cells and macrophages) and its activation has been associated with a variety of immune responses incl. diabetic wound healing (Fiorina et al., 2010).
The CCL19 chemokine was reported to play a prominent role in homing of lymphocytes, through interaction with CCR7 receptor, to secondary lymphoid tissues where T cell priming occurs (Comerford et al., 2013). To confirm now the significance of the observed antagonistic effect of 9-PAHSA on identified GPCRs, we sought to assess their effect on migration activity of CD4 T Cells across an endothelial cell monolayer and in presence of the CCL19 chemokine (Miyasaka and Tanaka, 2004; Picchio et al., 2008). The chemokine induced T-cell migration was reduced by co-incubation with 9-PAHSA but not by 5-PAHSA. The high concentrations of 10 µM are in accordance with the concentration response curves on the recombinant CCR7 receptor assessed in this work.
Finally, we sought to provide, a functional relevance of PAHSAs in the context of human inflammation, and therefore studied 5- and 9-PAHSA in a complex human cellular model of immunity (MIMIC® PTE system). These data suggest a minimal, yet non-significant anti-inflammatory effect of PAHSAs, mainly for the highest concentrations of 9-PAHSA tested. This weak anti-inflammatory effect was characterized by a small reduction of CD86 expression in APCs and the inhibition of a very specific chemokine secretion profile (mainly CXCL10) induced by LPS stimulation at high concentrations where a reduction in cell viability was also detected. These results, while showing a minimal reduction of innate immune activation induced by LPS as reported by Yore et al. (2014), were not completely aligned with this study result because they reported 9-PAHSA strongly blocked the LPS-induced effect on mouse BMDC activation (based on CD80, CD86, CD40, and MHC II expression), as well as the reduction in the secretion of pro-inflammatory cytokines, such as IL-12, IL-1β and TNFα (Yore et al., 2014). We observed a very modest reduction in secretion of certain chemokines most prominently CXCL10 and mainly induced by 9-PAHSA. The release of other major pro-inflammatory cytokines such as IL-6, IL-8, TNFα, MCP-1 and IL-1B was unaffected. In contrast to other studies, where PAHSA treatment reduced the secretion of IL-6, MCP-1 and TNFα in adipocyte and (Wang et al., 2018a) macrophage cell lines, we cannot confirm this result in the MIMIC® PTE model. Species differences may be accountable for this discrepancy.
A molecular activity calculating algorithm within the Ingenuity Knowledge Database predicted that orchestrated antagonism of the identified chemokine receptors together with a decrease in CXL10 finally may result in inhibition of chemotaxis and migration of immune cells. Thus, we would propose that 9-PAHSA at very high pharmacological levels may attenuate the movement of circulating leukocytes to the sites of inflammation and indirectly thereby reduces the local production and secretion of inflammatory mediators (Charo and Ransohoff, 2006). More direct evidence needs to be collected, e.g., by efficacy measurements of 9-PAHSA and related FAHSAs in models of chemotaxis, cell migration and inflammation.
From an analytical perspective, methods to detect FAHFAs in biological or organic samples have recently been fine-tuned (Zhu et al., 2017; Brezinova et al., 2018; Kolar et al., 2018). While there is no doubt about the existence of FAHSAs, the potential consequences of altered circulating PAHSA concentrations may be discussed controversially. Based on our data shown here, a weak anti-inflammatory efficacy of 9-PAHSA but not 5-PAHSA seems plausible in humans. However, it may be questioned if sufficiently high plasma concentrations could be achieved in humans by exogenous treatment with a naturally occuring PAHSA derivative.
Regarding pre-clinical metabolic consequences, we added some additional clarifying methods and data in our Supplementary Datasheet S1. It has been hypothesized that elevated FAHFAs in Bcl6AKO mice could be a marker rather than a mediator of systemic insulin sensitivity (Senagolage et al., 2018). Following the initial report of beneficial metabolic effects after exogenous 5- and 9-PAHSA treatment in obese mice (Yore et al., 2014), the same group also reported metabolic benefits in DIO mice (Syed et al., 2018a; Zhou et al., 2019). In addition, Bandak et al. found an improved islet function after chronic incubation with 5-PAHSA (Bandak et al., 2018) and Paluchova et al. recently described that 5-PAHSA beneficially primes adipocytes for glucose utilization (Paluchova et al., 2020). In contrast, in our previously reported experiments we did not find beneficial effects related to glucose metabolism after exogenous 5- or 9-PAHSA treatment (Pflimlin et al., 2018). Interestingly, Wang et al. demonstrated that 1-month of treatment with 9-PAHSA in ob/ob mice did not affect body weight, food intake, glycaemia, or insulin secretion (Wang et al., 2018a). Similarly, a lack of effects after 1-month treatment with 5-PAHSA on blood glucose has been reported in diabetic db/db mice, but instead treatment promoted hepato-steatosis (Wang et al., 2018b).
It was suggested that our previous findings reporting a lack of PAHSA treatment effect on metabolic parameters might be related to methodological differences (Syed et al., 2018b; Kuda, 2018) and those potential beneficial effects could have been masked in our in vitro and in vivo experiments. Such differences include the use of different vehicles to dissolve PAHSAs, the vendor of mice used in the studies, the housing conditions, the amount of glucose used in the glucose tolerance tests as well as general differences in the experimental procedures and conditions especially in experiments involving animals. In fact, it seems impossible that all variables potentially affecting a study can be fully controlled and kept identical between two studies as a remaining portion of biological and environmental variability is an inherent feature to life sciences. While striving for an exact reproduction of experimental conditions certainly has its justification, also differences in experimental procedures between two laboratories have distinct advantages, as the confirmation of key data in a real world setting clearly increases the robustness of the initial finding. In any case, we and others clearly appreciate the open scientific discussion, even on divergent conclusions, as they can provide valuable insights for the research community (von Herrath et al., 2019). In our current study, we have also addressed in the supplement key methodological difference cited as a source of discrepancy, namely, the vehicle used in the studies and have conducted a series of additional in vitro and in vivo experiments with adapted methods. In agreement with our previous findings, our current data do not support beneficial effects of 9-PAHSA on glucose metabolism regardless of the vehicle used. Thus, we cannot detect metabolic benefits of 9-PAHSA as previously described.
In conclusion, our studies indicate that 5- and 9-PAHSA neither agonize nor antagonize a broad range of orphan GPCRs or GPCRs with known ligands. If interactions between this fascinating class of fatty acid derivatives and GPCRs occur, those might be identified more on a combined antagonism of specific inflammatory receptors like CCR6, CCR7, CXCR4, and CXCR5. Caution is warranted when extrapolating the results from pre-clinical anti-inflammatory studies in vitro and in mice to humans (Fritsche, 2015). Despite that we could not confirm a strong efficacy of 9-PAHSA in a first in vitro model of innate human immunity, further studies with new PAHSA derivatives would be highly interesting to determine if the action of 9-PAHSA itself or novel, possibly more potent FAHFA analogue can be used as a viable treatment option for inflammatory conditions in humans.
Data availability statement
The original contributions presented in the study are included in the article/Supplementary Material, further inquiries can be directed to the corresponding author.
Ethics statement
The animal study was reviewed and approved by the Sanofi’s Institutional Animal Care and Use Committee (IACUC) termed AWB (Animal Welfare Body/ethics committee). Furthermore, all animal experimental procedures were approved by the responsible German government authorities (RP Darmstadt, Darmstadt, Germany).
Author contributions
PW and MK performed and supervised most in vitro experiments, analyzed the data and wrote the manuscript. MC, EL, and RM conducted the CD4+ T-cell migration and human MIMIC® PTE assay, analyzed respective data and wrote the manuscript. AK interpreted data, contributed to the design of the studies, and revised the manuscript. US analyzed mouse plasma samples. SP contributed to the synthesis of PAHSA, analysis of data and revised the manuscript. AK and NT designed experiments, interpreted data, and contributed to the manuscript writing. MB conducted in vivo studies, analyzed, and interpreted data and wrote the manuscript. PW, MK, AK, NT, and MB oversaw the project. All authors contributed to the article and approved the submitted version.
Funding
This study received funding from Sanofi. The funder had the following involvement with the study: All authors were employed during the study by Sanofi. The funder was not involved in the study design, collection, analysis, interpretation of data, the writing of this article or the decision to submit it for publication.
Acknowledgments
The authors would like to thank all involved members from the respective analytical, in vitro, and in vivo laboratories at Sanofi for excellent technical support; especially Elke Deckert, Jutta Rosenberger, Sandra Bumes, Silke Loy and Vanessa Tsovanyan. Furthermore, we deeply appreciate the continuous support by Edoardo Sarubbi (Sanofi) in testing PAHSAs on broad panels of G protein-coupled receptors.
Conflict of interest
All authors were during the time of project completion employees and shareholders of Sanofi. MB, AK and NT are now employees of CSL Behring Innovation GmbH, Germany, Eli Lilly and Company, USA or Bayer AG, Pharmaceuticals Division, respectively. CSL Behring Innovation GmbH, Eli Lilly and Company or Bayer AG, Pharmaceuticals Division were not involved in any aspect of this study. Authors PW, MK, AK, US, SP, NT, MB, MC, EL, and RM were employed by Sanofi.
The author PW declared that he was an editorial board member of Frontiers, at the time of submission. This had no impact on the peer review process and the final decision.
Publisher’s note
All claims expressed in this article are solely those of the authors and do not necessarily represent those of their affiliated organizations, or those of the publisher, the editors and the reviewers. Any product that may be evaluated in this article, or claim that may be made by its manufacturer, is not guaranteed or endorsed by the publisher.
Supplementary material
The Supplementary Material for this article can be found online at: https://www.frontiersin.org/articles/10.3389/fddsv.2023.1138461/full#supplementary-material
References
Acosta-Rodriguez, E. V., Rivino, L., Geginat, J., Jarrossay, D., Gattorno, M., Lanzavecchia, A., et al. (2007). Surface phenotype and antigenic specificity of human interleukin 17-producing T helper memory cells. Nat. Immunol. 8 (6), 639–646. doi:10.1038/ni1467
Aryal, P., Syed, I., Lee, J., Patel, R., Nelson, A. T., Siegel, D., et al. (2021). Distinct biological activities of isomers from several families of branched fatty acid esters of hydroxy fatty acids (FAHFAs). J. Lipid Res. 62, 100108. doi:10.1016/j.jlr.2021.100108
Balas, L., Feillet-Coudray, C., and Durand, T. (2018). Branched fatty acyl esters of hydroxyl fatty acids (FAHFAs), appealing beneficial endogenous fat against obesity and type-2 diabetes. Chemistry 24 (38), 9463–9476. doi:10.1002/chem.201800853
Bandak, B., Yi, L., and Roper, M. G. (2018). Microfluidic-enabled quantitative measurements of insulin release dynamics from single islets of Langerhans in response to 5-palmitic acid hydroxy stearic acid. Lab. Chip 18 (18), 2873–2882. doi:10.1039/c8lc00624e
Brezinova, M., Kuda, O., Hansikova, J., Rombaldova, M., Balas, L., Bardova, K., et al. (2018). Levels of palmitic acid ester of hydroxystearic acid (PAHSA) are reduced in the breast milk of obese mothers. Biochim. Biophys. Acta Mol. Cell. Biol. Lipids 1863 (2), 126–131. doi:10.1016/j.bbalip.2017.11.004
Charo, I. F., and Ransohoff, R. M. (2006). The many roles of chemokines and chemokine receptors in inflammation. N. Engl. J. Med. 354 (6), 610–621. doi:10.1056/NEJMra052723
Collin, M., McGovern, N., and Haniffa, M. (2013). Human dendritic cell subsets. Immunology 140 (1), 22–30. doi:10.1111/imm.12117
Comerford, I., Harata-Lee, Y., Bunting, M. D., Gregor, C., Kara, E. E., and McColl, S. R. (2013). A myriad of functions and complex regulation of the CCR7/CCL19/CCL21 chemokine axis in the adaptive immune system. Cytokine Growth Factor Rev. 24 (3), 269–283. doi:10.1016/j.cytogfr.2013.03.001
Cooper, A., Singh, S., Hook, S., Tyndall, J. D. A., and Vernall, A. J. (2017). Chemical tools for studying lipid-binding class A G protein-coupled receptors. Pharmacol. Rev. 69 (3), 316–353. doi:10.1124/pr.116.013243
Fiorina, P., Pietramaggiori, G., Scherer, S. S., Jurewicz, M., Mathews, J. C., Vergani, A., et al. (2010). The mobilization and effect of endogenous bone marrow progenitor cells in diabetic wound healing. Cell. Transpl. 19 (11), 1369–1381. doi:10.3727/096368910X514288
Fritsche, K. L. (2015). The science of fatty acids and inflammation. Adv. Nutr. 6 (3), 293S–301S. doi:10.3945/an.114.006940
Hansen, K. B., and Brauner-Osborne, H. (2009). FLIPR assays of intracellular calcium in GPCR drug discovery. Methods Mol. Biol. 552, 269–278. doi:10.1007/978-1-60327-317-6_19
Higbee, R. G., Byers, A. M., Dhir, V., Drake, D., Fahlenkamp, H. G., Gangur, J., et al. (2009). An immunologic model for rapid vaccine assessment - a clinical trial in a test tube. Altern. Lab. Anim. 37 (Suppl. 1), 19–27. doi:10.1177/026119290903701S05
Hughes, C. E., and Nibbs, R. J. B. (2018). A guide to chemokines and their receptors. FEBS J. 285 (16), 2944–2971. doi:10.1111/febs.14466
Ito, R., Tsujihata, Y., Suzuki, M., Miyawaki, K., Matsuda, K., and Takeuchi, K. (2016). Fasiglifam/TAK-875, a selective GPR40 agonist, improves hyperglycemia in rats unresponsive to sulfonylureas and acts additively with sulfonylureas. J. Pharmacol. Exp. Ther. 357 (1), 217–227. doi:10.1124/jpet.115.230730
Kaku, K., Enya, K., Nakaya, R., Ohira, T., and Matsuno, R. (2015). Efficacy and safety of fasiglifam (TAK-875), a G protein-coupled receptor 40 agonist, in Japanese patients with type 2 diabetes inadequately controlled by diet and exercise: A randomized, double-blind, placebo-controlled, phase III trial. Diabetes Obes. Metab. 17 (7), 675–681. doi:10.1111/dom.12467
Kolar, M. J., Nelson, A. T., Chang, T., Ertunc, M. E., Christy, M. P., Ohlsson, L., et al. (2018). Faster protocol for endogenous fatty acid esters of hydroxy fatty acid (FAHFA) measurements. Anal. Chem. 90 (8), 5358–5365. doi:10.1021/acs.analchem.8b00503
Kolar, M. J., Konduri, S., Chang, T., Wang, H., McNerlin, C., Ohlsson, L., et al. (2019). Linoleic acid esters of hydroxy linoleic acids are anti-inflammatory lipids found in plants and mammals. J. Biol. Chem. 294 (27), 10698–10707. doi:10.1074/jbc.RA118.006956
Krämer, A., Green, J., Pollard, J., and Tugendreich, S. (2014). Causal analysis approaches in ingenuity pathway analysis. Bioinforma. Oxf. Engl. 30 (4), 523–530. doi:10.1093/bioinformatics/btt703
Kuda, O., Brezinova, M., Rombaldova, M., Slavikova, B., Posta, M., Beier, P., et al. (2016). Docosahexaenoic acid-derived fatty acid esters of hydroxy fatty acids (FAHFAs) with anti-inflammatory properties. Diabetes 65 (9), 2580–2590. doi:10.2337/db16-0385
Kuda, O. (2018). On the complexity of PAHSA research. Cell. Metab. 28 (4), 541–542. doi:10.1016/j.cmet.2018.09.006
Lee, J., Moraes-Vieira, P. M., Castoldi, A., Aryal, P., Yee, E. U., Vickers, C., et al. (2016). Branched fatty acid esters of hydroxy fatty acids (FAHFAs) protect against colitis by regulating gut innate and adaptive immune responses. J. Biol. Chem. 291 (42), 22207–22217. doi:10.1074/jbc.M115.703835
Li, M., van Esch, B., Wagenaar, G. T. M., Garssen, J., Folkerts, G., and Henricks, P. A. J. (2018). Pro- and anti-inflammatory effects of short chain fatty acids on immune and endothelial cells. Eur. J. Pharmacol. 831, 52–59. doi:10.1016/j.ejphar.2018.05.003
Liberati-Cizmek, A. M., Bilus, M., Brkic, A. L., Baric, I. C., Bakula, M., Hozic, A., et al. (2019). Analysis of fatty acid esters of hydroxyl fatty acid in selected plant food. Plant Foods Hum. Nutr. 74 (2), 235–240. doi:10.1007/s11130-019-00728-8
Luna, E., Agrawal, P., Mehta, R., Boone, M. E., Vernhes, C., Denys, C., et al. (2018). Evaluation of the innate immunostimulatory potential of originator and non-originator copies of insulin glargine in an in vitro human immune model. PLoS One 13 (6), e0197478. doi:10.1371/journal.pone.0197478
Luna, E., Agrawal, P., Mehta, R., Vernhes, C., Viskov, C., Amiral, J., et al. (2015). Evaluation of immunostimulatory potential of branded and US-generic enoxaparins in an in vitro human immune system model. Clin. Appl. Thromb. Hemost. 21 (3), 211–222. doi:10.1177/1076029614562037
Ma, Y., Poisson, L., Sanchez-Schmitz, G., Pawar, S., Qu, C., Randolph, G. J., et al. (2010). Assessing the immunopotency of Toll-like receptor agonists in an in vitro tissue-engineered immunological model. Immunology 130 (3), 374–387. doi:10.1111/j.1365-2567.2009.03237.x
Miyasaka, M., and Tanaka, T. (2004). Lymphocyte trafficking across high endothelial venules: Dogmas and enigmas. Nat. Rev. Immunol. 4 (5), 360–370. doi:10.1038/nri1354
Oh, D. Y., Talukdar, S., Bae, E. J., Imamura, T., Morinaga, H., Fan, W., et al. (2010). GPR120 is an omega-3 fatty acid receptor mediating potent anti-inflammatory and insulin-sensitizing effects. Cell. 142 (5), 687–698. doi:10.1016/j.cell.2010.07.041
Paluchova, V., Oseeva, M., Brezinova, M., Cajka, T., Bardova, K., Adamcova, K., et al. (2020). Lipokine 5-PAHSA is regulated by adipose triglyceride lipase and primes adipocytes for de novo lipogenesis in mice. Diabetes 69 (3), 300–312. doi:10.2337/db19-0494
Pflimlin, E., Bielohuby, M., Korn, M., Breitschopf, K., Löhn, M., Wohlfart, P., et al. (2018). Acute and repeated treatment with 5-PAHSA or 9-PAHSA isomers does not improve glucose control in mice. Cell. Metab. 28 (2), 217–227.e13. doi:10.1016/j.cmet.2018.05.028
Picchio, M. C., Scala, E., Pomponi, D., Caprini, E., Frontani, M., Angelucci, I., et al. (2008). CXCL13 is highly produced by Sezary cells and enhances their migratory ability via a synergistic mechanism involving CCL19 and CCL21 chemokines. Cancer Res. 68 (17), 7137–7146. doi:10.1158/0008-5472.CAN-08-0602
Rodriguez, J. P., Guijas, C., Astudillo, A. M., Rubio, J. M., Balboa, M. A., and Balsinde, J. (2019). Sequestration of 9-hydroxystearic acid in FAHFA (fatty acid esters of hydroxy fatty acids) as a protective mechanism for colon carcinoma cells to avoid apoptotic cell death. Cancers (Basel). 11 (4), 524. doi:10.3390/cancers11040524
Rubin de Celis, M. F., Garcia-Martin, R., Syed, I., Lee, J., Aguayo-Mazzucato, C., Bonner-Weir, S., et al. (2022). PAHSAs reduce cellular senescence and protect pancreatic beta cells from metabolic stress through regulation of Mdm2/p53. Proc. Natl. Acad. Sci. U. S. A. 119 (47), e2206923119. doi:10.1073/pnas.2206923119
Santopaolo, M., Sullivan, N., Thomas, A. C., Alvino, V. V., Nicholson, L. B., Gu, Y., et al. (2021). Activation of bone marrow adaptive immunity in type 2 diabetes: Rescue by Co-stimulation modulator abatacept. Front. Immunol. 12, 609406. doi:10.3389/fimmu.2021.609406
Senagolage, M. D., Sommars, M. A., Ramachandran, K., Futtner, C. R., Omura, Y., Allred, A. L., et al. (2018). Loss of transcriptional repression by BCL6 confers insulin sensitivity in the setting of obesity. Cell. Rep. 25 (12), 3283–3298.e6. doi:10.1016/j.celrep.2018.11.074
Syed, I., Lee, J., Moraes-Vieira, P. M., Donaldson, C. J., Sontheimer, A., Aryal, P., et al. (2018a). Palmitic acid hydroxystearic acids activate GPR40, which is involved in their beneficial effects on glucose homeostasis. Cell. Metab. 27 (2), 419–427.e4. doi:10.1016/j.cmet.2018.01.001
Syed, I., Lee, J., Peroni, O. D., Yore, M. M., Moraes-Vieira, P. M., Santoro, A., et al. (2018b). Methodological issues in studying PAHSA biology: Masking PAHSA effects. Cell. Metab. 28 (4), 543–546. doi:10.1016/j.cmet.2018.09.007
Syed, I., Rubin de Celis, M. F., Mohan, J. F., Moraes-Vieira, P. M., Vijayakumar, A., Nelson, A. T., et al. (2019). PAHSAs attenuate immune responses and promote beta cell survival in autoimmune diabetic mice. J. Clin. Investig. 129 (9), 3717–3731. doi:10.1172/JCI122445
Tortosa-Caparros, E., Navas-Carrillo, D., Marin, F., and Orenes-Pinero, E. (2017). Anti-inflammatory effects of omega 3 and omega 6 polyunsaturated fatty acids in cardiovascular disease and metabolic syndrome. Crit. Rev. Food Sci. Nutr. 57 (16), 3421–3429. doi:10.1080/10408398.2015.1126549
von Herrath, M., Pagni, P. P., Grove, K., Christoffersson, G., Tang-Christensen, M., Karlsen, A. E., et al. (2019). Case reports of pre-clinical replication studies in metabolism and diabetes. Cell. Metab. 29 (4), 795–802. doi:10.1016/j.cmet.2019.02.004
Wang, H., Chang, T., Konduri, S., Huang, J., Saghatelian, A., Siegel, D., et al. (2019a). Identification and evaluation of reference genes for quantitative real-time PCR analysis in Polygonum cuspidatum based on transcriptome data. J. antibiotics 72 (6), 498–506. doi:10.1186/s12870-019-2108-0
Wang, Y., Li, W., Zhou, J., Wan, R., Hao, W., Gao, Y., et al. (2019b). Autoantibody-positivity in lean type II diabetes patients was associated with elevated Th17-like CD4(+)CXCR5(+) T cell responses. Mol. Immunol. 112, 305–311. doi:10.1016/j.molimm.2019.06.010
Wang, Y. M., Liu, H. X., and Fang, N. Y. (2018a). 9-PAHSA promotes browning of white fat via activating G-protein-coupled receptor 120 and inhibiting lipopolysaccharide/NF-kappa B pathway. Biochem. Biophys. Res. Commun. 506 (1), 153–160. doi:10.1016/j.bbrc.2018.09.050
Wang, Y. M., Liu, H. X., and Fang, N. Y. (2018b). High glucose concentration impairs 5-PAHSA activity by inhibiting AMP-activated protein kinase activation and promoting nuclear factor-kappa-B-mediated inflammation. Front. Pharmacol. 9, 1491. doi:10.3389/fphar.2018.01491
Wang, Y. M., Mi, S. L., Jin, H., Guo, Q. L., Yu, Z. Y., Wang, J. T., et al. (2021). 9-PAHSA improves cardiovascular complications by promoting autophagic flux and reducing myocardial hypertrophy in Db/Db mice. Front. Pharmacol. 12, 754387. doi:10.3389/fphar.2021.754387
Yore, M. M., Syed, I., Moraes-Vieira Pedro, M., Zhang, T., Herman, Mark A., Homan Edwin, A., et al. (2014). Discovery of a class of endogenous mammalian lipids with anti-diabetic and anti-inflammatory effects. Cell. 159, 318–332. doi:10.1016/j.cell.2014.09.035
Yoshida, R., Imai, T., Hieshima, K., Kusuda, J., Baba, M., Kitaura, M., et al. (1997). Molecular cloning of a novel human CC chemokine EBI1-ligand chemokine that is a specific functional ligand for EBI1, CCR7. J. Biol. Chem. 272 (21), 13803–13809. doi:10.1074/jbc.272.21.13803
Zhou, P., Santoro, A., Peroni, O. D., Nelson, A. T., Saghatelian, A., Siegel, D., et al. (2019). PAHSAs enhance hepatic and systemic insulin sensitivity through direct and indirect mechanisms. J. Clin. Investig. 129 (10), 4138–4150. doi:10.1172/JCI127092
Zhu, Q. F., Yan, J. W., Gao, Y., Zhang, J. W., Yuan, B. F., and Feng, Y. Q. (2017). Highly sensitive determination of fatty acid esters of hydroxyl fatty acids by liquid chromatography-mass spectrometry. J. Chromatogr. B Anal. Technol. Biomed. Life Sci. 1061-1062, 34–40. doi:10.1016/j.jchromb.2017.06.045
Keywords: receptors/ seven transmembrane domain, cell signaling, inflammation, obesity, cytokines, fatty acids, FAHFA, diabetes
Citation: Wohlfart P, Chehtane M, Luna E, Mehta R, Korn M, Konkar A, Schwahn U, Petry S, Tennagels N and Bielohuby M (2023) 9-PAHSA displays a weak anti-inflammatory potential mediated by specific antagonism of chemokine G protein-coupled receptors. Front. Drug Discov. 3:1138461. doi: 10.3389/fddsv.2023.1138461
Received: 05 January 2023; Accepted: 03 July 2023;
Published: 20 July 2023.
Edited by:
Rajeev K. Tyagi, Institute of Microbial Technology (CSIR), IndiaReviewed by:
James Edward Pease, Imperial College London, United KingdomGabriele Carullo, University of Siena, Italy
Copyright © 2023 Wohlfart, Chehtane, Luna, Mehta, Korn, Konkar, Schwahn, Petry, Tennagels and Bielohuby. This is an open-access article distributed under the terms of the Creative Commons Attribution License (CC BY). The use, distribution or reproduction in other forums is permitted, provided the original author(s) and the copyright owner(s) are credited and that the original publication in this journal is cited, in accordance with accepted academic practice. No use, distribution or reproduction is permitted which does not comply with these terms.
*Correspondence: Paulus Wohlfart, UGF1bHVzLldvaGxmYXJ0QHNhbm9maS5jb20=
†Present address: Anish Konkar, Diabetes and Complications, Lilly Research Laboratories, Eli Lilly and Company, Indianapolis, IN, United States Norbert Tennagels, Pharmaceuticals Division, Research and Development, DIU Exploratory Pathobiology, Bayer AG, Wuppertal, Germany Maximilian Bielohuby, CSL Behring Innovation GmbH, Marburg, Germany