- 1Laboratory of Transgenic Models of Diseases, Institute of Molecular Genetics of the CAS, v.v.i, Vestec, Czechia
- 2Czech Centre for Phenogenomics, Institute of Molecular Genetics of the CAS, v.v.i, Vestec, Czechia
Severe acute respiratory syndrome coronavirus 2 (SARS-CoV2) is a positive-sense-single stranded RNA virus and the cause of the coronavirus disease 2019 (COVID-19). The World Health Organisation has confirmed over 250 million cases with over 5.1 million deaths as a result of this pandemic since December 2019. A global outbreak of such intensity and perseverance is due to the novelty of SARS-CoV2 virus, meaning humans lack any pre-existing immunity to the virus. Humanised animal models, from rodents to primates, simulating SARS-CoV2 transmission, cell entry and immune defence in humans have already been crucial to boost understanding of its molecular mechanisms of infection, reveal at-risk populations, and study the pathophysiology in vivo. Focus is now turning towards using this knowledge to create effective vaccines and therapeutic agents, as well as optimise their safety for translatable use in humans. SARS-CoV2 possesses remarkable adaptability and rapid mutagenic capabilities thus exploiting innovative animal models will be pivotal to outmanoeuvre it during this pandemic. In this review, we summarise all generated SARS-CoV2-related animal models to date, evaluate their suitability for COVID-19 research, and address the current and future state of the importance of animal models in this field.
1 COVID-19 Origin and SARS-CoV2 Transmission
A pandemic is defined as a disease that is prevalent in an entire country or the world, and thus is undoubtedly the correct term for the coronavirus disease 2019 (COVID-19) outbreak. COVID-19 is caused by the severe acute respiratory syndrome coronavirus 2 (SARS-CoV2) which has been pinpointed to have originated from Wuhan, China in December 2019 and has since then spread over all continents including Antarctica (Triggle et al., 2021). Before the COVID-19 outburst there were already two identified and relatively well-known human coronaviruses causing severe respiratory pneumonia namely, SARS-CoV and MERS-CoV. They both originate from bats but spilled over to intermediate hosts namely, civets and dromedary camels, respectively. The origin of SARS-CoV2 is also suggested, based on its sequence similarity to the SARS-CoV, to have originated from bats and later spilled over to an animal reservoir, however it is not yet confirmed (Forni et al., 2017). Bats are and continue to be a copious source for novel viral sequences (Jiang et al., 2022). The bat species are among one of the oldest mammals and they exhibit great diversity and are widely spread across the globe (X. M. Zhang et al., 1992). Cross-species mixing between different kinds of bats has facilitated a maintenance of less discriminatory viruses capable of infecting a broader variety of hosts. Bats are thus a carrier of a pool of viruses able to perform inter-species transmission, which has been a reason for concern long before the COVID-19 outbreak (Calisher et al., 2006). SARS-CoV2 is a pneumotropic virus that mainly spreads through respiratory secretions like coughing and sneezing. The transmission may also occur via contaminated surfaces where the virus can survive up to 6 days, making preventive measures such as surface disinfection, hand hygiene and masks important in combating transmission (Leclerc et al., 2020).
Once infected, COVID-19 manifests in a large variety of symptoms however, the most common ones include fever, sore throat, fatigue, cough, dyspnoea and immune system dysregulation, often ending with cytokine storm (Ragab et al., 2020). SARS-CoV2 is not only capable of affecting the respiratory system by pulmonary infiltration and inflammation but can spread to multiple organ systems. For the majority of people the disease symptoms are mild and the infection runs its course without any medical intervention but for approximately 5–10%, it severely affects the fitness of the individual and for another 2% it has a mortal outcome (Gavriatopoulou et al., 2021).
The SARS-CoV2 consists of approximately 29.9kB of single-stranded, non-segmented, positive-sense RNA.(Triggle et al., 2021). The genome is composed by 13–15 open reading frames largely resembling the make-up of MERS-CoV and SARS-CoV. The genome contains 11 protein coding genes with ultimate expression of 12 expressed proteins (Lu et al., 2020).
Structurally, it consists of four proteins namely, spike (S), envelope (E), membrane (M) and nucleocapsid (N) (Figure 1A). These proteins play important parts in entry, fusion and replication in the host cells. The non-structural have roles imperative to viral pathogenesis by regulating early transcription, helicase activity, gene transactivation and countering antiviral response (J Alsaadi and Jones, 2019; Tang et al., 2020).
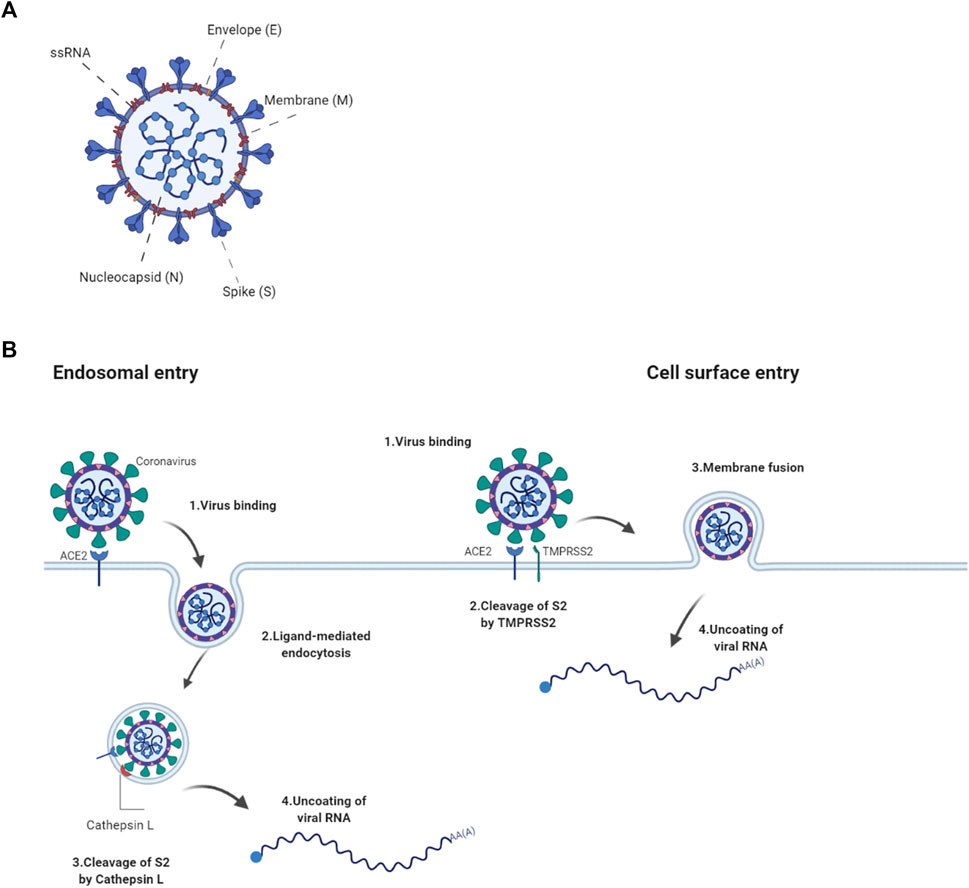
FIGURE 1. ACE2-mediated entry of SARS-COV2 into a cell (A) The SARS-CoV2 virion consists of structural proteins, namely spike (S), envelope (E), membrane (M), nucleocapsid (N) The positive-sense, single-stranded RNA genome (+ssRNA) is bound by N in a beads-on-a-string formation. (B) SARS-CoV2 binds to the cell via S interaction with the host’s ACE2 receptor. Entry to the host cell either goes through receptor-mediated endocytosis where fusion is potentiated by the cleavage of S2 by Cathepsin L or by cell surface fusion via the TMPRSS2 serine protease. Following fusion, the virion is uncoated and the viral genome released. Created with Biorender.com.
The spike glycoprotein plays a pivotal role in the pathogenesis of SARS-CoV2 as it pivotal for the entry into the host cell. It is assembled as a homotrimer and inserted in multiple copies into the virus membrane, giving the virus a crown-like appearance, thus its name coronavirus (Jackson et al., 2022). It consists of two functional subunits, S1 and S2, that both part take in the entry of the virus. The S1 subunit has a receptor-binding domain (RBD) and is responsible for anchoring the host cell upon binding between the RBD and the human angiotensin-converting enzyme 2 (hACE2), thus stabilizing the virus (Hoffmann et al., 2020). Once the RBD region of the S1 subunit binds to the hACE2, the virus enters the host’s endosomes via ligand-mediated endocytosis or membrane-fusion. Once bound to the ACE2, the S protein undergoes conformational changes, which are important to therapeutically limit its infection cycle (Wrapp et al., 2020). Although several mutations have been found in the RBD of the S1 subunit, its affinity to and interaction with the hACE2 is preserved in most species however not in mouse (Chan et al., 2020; Wrapp et al., 2020). The S2 subunit functions as a fusion protein between the virus and the host cell membrane. The S2 exhibit three different conformational changes during the process namely, i) native state before fusion, ii) intermediate state and iii) post fusion hairpin state (Qing and Gallagher, 2020; Walls et al., 2020). Finally the S protein is cleaved either by the host cell surface serine protease TMPRSS or by host’s Cathepsin L in the endosomal compartment at the S2′ cleavage site (Figure 1B; Simmons et al., 2005). The cleavage releases a fusion peptide, which initiates the fusion pore formation. Once the pore expands and the cell membranes of both the virion and the host are combined, the viral genome can be released in to the cytoplasm. The cell membrane or endosomal fusion, represent the two different modes of entry for the viral genome to be released.
The N protein, composed by two separate domains, is present in the nucleocapsid complex that tightly binds the RNA genome of the virus. Both the N-terminal and C-terminal domain can bind to RNA but is more efficient when both bind simultaneously (Chang et al., 2006). The N protein bind the viral RNA genome in a beads-on-a-string conformation. The ribonucleotide protein (RNP) complex is subsequently packaged in to viral particles enveloped by a fatty lipid bi-layer (Fehr and Perlman, 2015).
The envelope protein is a relatively small protein that plays a substantial role in viral assembly. The protein assemble in to the host membrane forming protein-lipid pores referred to as viroporins. The envelope protein is highly conserved between SARS-CoV and SARS-CoV2 (Fehr and Perlman, 2015).
SARS-CoV2’s membrane protein is the most abundant structural protein and is a transmembrane with a short NH2 terminal on the outside and a long cytoplasmic COOH terminus. Completion of viral assembly is potentiated partly by the binding between M proteins and N proteins leading to a stabilization of the N-Protein and RNA complex internally (Thomas, 2020).
2 Infection Route and ACE2 Function
The primary route of entry for the SARS-CoV2 is the upper respiratory tract. The virus gains access to the host cells by binding to the ACE2 receptors and subsequently introduced in to the cytoplasm via receptor-mediated endocytosis. The virus particles then goes through uncoating. The RNA and proteins needed for translation are released followed by transcription and assembly, finally the viral loads are shed thus completing the viral replication cycle (Jiang et al., 2020). As the virus sheds, the newly replicated and released particles bind upon another host cell and the cycle starts again. The ACE2 receptor is a carboxypeptidase consisting of 805 amino acids that removes a single amino acid from the C terminus of its substrates (Turner and Hooper, 2002). The ACE2 receptors are expressed in alveolar epithelial cells and capillary endothelial cells that are abundant in organs such as the lungs, kidneys, brain and gut hence explaining the multisystem infection found in a substantial amount of patients (Samavati and Uhal, 2020). The physiological role of ACE2 in humans is to convert angiotensin I and II to angiotensin 1–9 and angiotensin 1–7, respectively. This is one of the steps making up the Renin Angiotensin Aldosterone System (RAAS) a system, which functions to elevate blood volume and arterial tone via sodium and water reabsorption and vascular tone (Nehme et al., 2019). Infection results in a decrease of physiologically available ACE2 receptors thus disrupts the RAAS system, leading to potential downstream complications such as inflammation and circulatory dysfunction (Guo et al., 2020).
3 Translational studies: Importance of Mouse Models
Since the start of the COVID-19 pandemic, we have gained substantial knowledge about the SARS-CoV2 virus in terms of its genetic make-up, transmission, infection and pathogenesis. This allows us to develop therapeutic agents to combat it. However, to perform scientifically sound and reliable research it is of the utmost importance to work with an appropriate model organism for in vivo study. The laboratory mouse is the most used animal in medical research as they are inexpensive, easy to handle, are genetically very similar to humans and can be genetically modified relatively easy (Sellers, 2017). They are often present as inbred strains, making it a highly controlled system, which is desirable in medical research. The mouse as an organism for translational research in COVID-19 medical research is however not well suited for COVID-19 as the ACE2 receptor of the mouse is not efficiently bound by the SARS-CoV2 virus, thus rendering the mouse immune to severe infection. This seemingly huge barrier has been surpassed by the generation of various modified mouse models capable of infection (Jia et al., 2020), as exemplified in the text below.
The COVID-19 outbreak pointed out a desperate need for relevant animal models for SARS-CoV2 research. As mentioned above wild type mouse cells and tissues are not very susceptible to SARS-CoV2 due to lack of human ACE2 specifically. Basically, mouse Ace2 does not bind the virus efficiently enough to mediate cell entry. To overcome this obstacle and study COVID-19 in mouse models, researchers have developed several approaches such as “murinisation” of SARS-CoV2 (Dinnon et al., 2020; Gu et al., 2020) or humanisation of mouse models (McCray et al., 2007; Tseng et al., 2007; Menachery et al., 2016). Alternatively they used different animal models which are sensitive to known SARS-CoV2 variants, such as hamsters, ferrets or non-human primates (Enkirch and von Messling, 2015; Finch et al., 2020; Munster et al., 2020; Rockx et al., 2020; Gruber et al., 2021).
Several transgenic mouse models have been developed and used in COVID-19 research to overcome limits of mouse Ace2 and generate inexpensive models with high-throughput study potential. The models are based on ubiquitous or cell/tissue specific expression of human ACE2, a protein well-known for its importance in SARS-CoV2 entry in the cell.
3.1 K18-hACE2 Model
This mouse model expresses hACE2 under control of the epithelial cell cytokeratin-18 promoter, expressed in subset of epithelial cells (Table 1). Even though the model has been generated to study SARS-CoV during 2003 epidemics, it remains relevant for SARS-CoV2 research too (McCray et al., 2007). K18-hACE2 model is susceptible to both strains of SARS-CoV, one and 2. In the context of COVID-19 research, the model responds to the infection by progressive weight loss, high viral titres in the lung at the beginning of infection, and with progressing infection increasing viral titres in the brain and gut. Other less severely affected organs are heart, kidney and spleen (Figure 2). (McCray et al., 2007; Rathnasinghe et al., 2020).
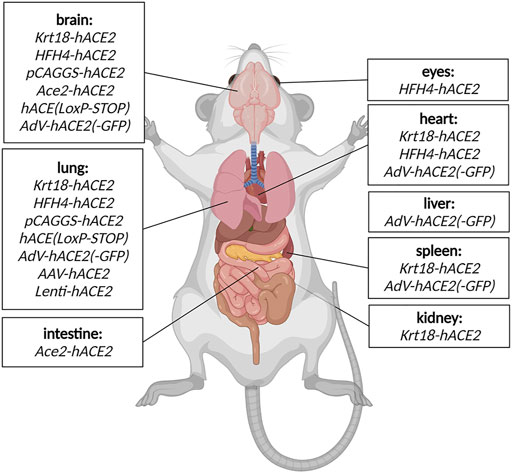
FIGURE 2. Detected SARS-CoV2 replication in COVID-19 mouse models. The organs where viral replication has been detected in specific COVID mouse models are depicted schematically. Created with Biorender.com.
3.2 HFH4-hACE2 Model
The model expresses hACE2 under lung ciliated epithelial cell-specific promoter (HFH4/FOXJ1), which was supposed to drive lung-specific hACE2 expression. However, detailed characterization of the model revealed a moderate hACE2 expression in other tissues such as the brain, eye, heart, liver, kidney and gut (Figure 2). The model is highly responsive to SARS-CoV2 infection with main replication of the virus in lungs, eyes, heart and brain accompanied with severe symptoms such as interstitial pneumonia sometimes succumbed to lethal encephalitis (Table 1) (Menachery et al., 2016; Jiang et al., 2020).
3.3 pCAGG-hACE2 Model
A model generated with multiple random integrations of pCAG-hACE2 cassette throughout the genome. Cytomegalovirus enhancer with chicken β-actin promoter (CAG) allows ubiquitous and constant expression of hACE2 in all tissues but mainly in lung, brain, heart and kidney (Table 1). This model is highly susceptible to SARS-CoV1 and 2 after intranasal application. The infection starts with initial exponential growth of viral titre in lungs and continues with gradual transmission to the brain. Slight presence of the virus was also observed in heart, kidney, spleen and small intestine (Figure 2). Of note, the lethal titre of the virus for the pCAGG-hACE2 model (2 × 102 to 2 × 104 TCID50) is lower than in case of K18-hACE2 model (104 to 105 TCID50) This fact might be connected to multiple insertion of pCAGG-hACE2 cassette in the genome in combination with ubiquitous and strong expression of hACE2 (Tseng et al., 2007; Asaka et al., 2021).
3.4 Ace2-hACE2 Model
Two major Ace2-hACE2 models have been generated to more closely mimic expression pattern of Ace2. The first model by was generated by random integration of a hACE2 cDNA under control of Ace2 promoter (Yang X. H. et al., 2007). The model indeed recapitulates endogenous expression of Ace2 in tissues such as lung, kidney, heart and intestines; the model is responsive to SARS-CoV2 infection with the major impact on lung tissue (Bao et al., 2020b).
The second Ace2-hACE2 model, generated by Sun S.-H. et al. (2020), is based on replacement of Ace2 coding sequence with hACE2 and tdTomato cDNA (Table 1). Therefore, the expression of transgenic cassette hACE2-IRES-tdTomato is under control of endogenous Ace2 promoter and present only in one or two copies depending on zygosity. Despite the lack of clinical symptoms or elevated mortality, this model responds to SARS-CoV2 infection by interstitial pneumonia of distinct scale depending on age. Sun’s group also points out different abundance of hACE2 throughout-tissues in human (kidney, heart, oesophagus, bladder, ileum) and hACE2 in their model (liver, spleen, small intestine, ovary, and brain), however without further explanation. Furthermore, the group identified brain, lung and trachea as the main tissues of SARS-CoV2 replication (Figure 2; Sun S.-H. et al., 2020).
3.5 hACE2(LoxP-STOP) Model
hACE2 (LoxP-STOP) also termed TgCAGLoxPStopACE2GFP is a model generated by random integration of a loxP-CRE dependent cassette under the CAG promoter (Table 1). In the presence of Cre recombinase, the STOP cassette is removed and expression of hACE2 cDNA and eGFP is turned on. This model allows for conditioned, tissue-specific and traceable expression of hACE2-IRES-GFP transgene (Bruter et al., 2021). Dolskiy and collective have tested two inducible and ubiquitously expressed Cre-ERT2 drivers (UBC-ACE2 and Rosa26-ACE2) to promote conditioned hACE2 expression. In this case, the most severely affected organs were lung and brain (Figure 2). Their results further suggested that severity and infection progress is dependent on the particular Cre driver, more specifically on its expression potency. Furthermore, relatively recent changes in renin-angiotensin system due to hACE2 overexpression can be another factor influencing response to the infection (Dolskiy et al., 2022).
3.6 Rosa26-chACE2
A model similar to the previous one, but a CAG-LoxP-STOP-LoxP-hACE2 cassette is inserted in Rosa26 locus in a site-specific manner (Table 1). Therefore, transgene copy number depends on zygosity. The model has not been validated yet through SARS-CoV2 infection. It is available at Czech Centre for Phenogenomics and will be soon available via European Mouse Mutant Archive (EMMA) (Czech Centre for Phenogenomics, 2021).
Of note, transgenic models have an important role in SARS-CoV2 research. However, their ectopic expression of ACE2 protein, specifically in case of K18-hACE2, HFH4-hACE2, and pCAGG-hACE2 models may lead to different response, development and impact of the infection. This fact to some extend limits translatability of gathered data to clinical practise (Shou et al., 2021). Therefore, ACE2 under control of endogenous Ace2 promoter or conditional expression might provide more precise understanding of systemic or tissue-specific importance of ACE2 in the context of COVID-19.
3.7 Sensitised Mouse Models
In order to circumvent the desperate need for COVID-19 mouse models in the peak of pandemics, researchers focused on development of alternative SARS-CoV2- sensitive mouse models. Paradoxically, a rapid generation of such models was mediated by viruses. Inhalation or intranasal application of a viral vector carrying hACE2 gene under strong promoter may lead to humanisation of upper and lower respiratory tracts. This approach allows fast, affordable and versatile generation of a sensitive model in various mouse strains and genetic backgrounds. It has been shown that the most suitable viral vectors for rapid humanisation happen to be adeno-associated virus, adenovirus, and lentivirus.
3.8 AAV-hACE2
Two independent groups have used Adeno-Associated vector of serotype 9 to deliver a cassette with hACE2 under control of CMV to the lung (Table 1; Israelow et al. (2020) have used commercially available AAV-CMV-hACE2 plasmid for AAV production and applied the vector virus via injection into the trachea. The De Gasparo’s group assembled the AAV-CMV-hACE2 plasmid by subcloning hACE2 cDNA isolated form HEK293 cells and the vector was administered with forced inhalation into the lung and upper respiratory system. Both groups confirmed functionality of AAV-mediated humanisation where treated mice became susceptible to SARS-CoV2 infection accompanied with progressive inflammatory immune response in lung (Figure 1; Israelow et al., 2020; De Gasparo et al., 2021). In addition to establishing a new sensitized model, Israelow and collective focused on deciphering the role of type I interferon during SARS-CoV2 infection. Whereas, De Gasparo and collective tested bispecific antibodies that reduced SARS-CoV2 infection and weight loss associated with ongoing virus infection. Humanisation with AAV offers rapid, adaptable mouse model with long-term transgene expression and low immunogenicity which is crucial for immunological studies (De Gasparo et al., 2021; Kovacech et al., 2022).
3.9 AdV-hACE2
Replication defective Adenovirus encoding hACE2 (AdV-hACE2) was used to humanise several mouse strains in order to overcome unavailability of transgenic models (Table 1). The vector is delivered intranasally and it is capable to sensitise lung tissue for SARS-CoV2 entry and replication. In other organs, low levels of SARS-CoV2 replication was also identified, such as heart, spleen, brain and liver (Figure 2). Sensitised models suffer from weight loss, develop lung pathologies and respond positively to treatment with neutralising antibodies. However, the model has limitations in the form of bronchial inflammation associated with AdV delivery (Hassan et al., 2020).
3.10 Lenti-hACE2
Lentiviral vectors can be also used for sensitising a mouse to SARS-CoV2. Two independent publications describe utility of a lentiviral vector encoding hACE2 and its ability to avoid significant immune response in lung tissue before SARS-CoV2 exposure (Table 1). The advantage of lentiviral systems is their integrative character, with possibly stable long-term expression allowing re-infection studies in the sensitised mice. Both publications emphasize the role of IFNAR1 depletion and its impact on SARS-CoV2 progression in sensitised models. However, the collectives also point out the presence of mild COVID-19 symptoms in the models, probably due to relatively low expression of hACE2 by lentivirus (Rawle et al., 2021; Katzman et al., 2022).
Transgenic mouse models, expressing hACE2, represent convenient systems for large-scale, rapid (compared to other animal models), and relatively inexpensive SARS-CoV2 research. However, their availability during pandemics has been limited and their expansion in larger cohorts is time-consuming and expensive. Furthermore, distinct transgenic models differ in their response to infection, some suffer from lethal neuroinvasion, some show only mild symptoms. In general, variability of these models is significant, and no universal transgenic model has been established yet (Yang XH. et al., 2007; Yang XH. et al., 2007; McCray et al., 2007; Tseng et al., 2007; Menachery et al., 2016; Jiang et al., 2020; Sun J. et al. (2020) Bruter et al., 2021; Dolskiy et al., 2022).
In contrast with transgenic models stand virus-sensitised models, which can be generated on wide variety of genetic backgrounds and genotypes in relatively large scale and short-time. Sensitised models often do not develop severe disease mainly due to absence of neuroinvasion, but their symptoms and impact on lung tissue resembles pathology in COVID-19 patients. Moreover, the distribution and scale of hACE2 expression varies with tropism of a used viral vector or promoter. Importantly, use of viral vectors may be associated with a risk of potential inflammation leading to interference with subsequent SARS-CoV2 infection (Hassan et al., 2020; Israelow et al., 2020; De Gasparo et al., 2021; Rawle et al., 2021; Katzman et al., 2022).
3.11 Other Animal Models
Alternatives to mouse models are other animals that are naturally susceptible to SARS-CoV2, such as hamsters (Mesocricetus auratus, Phodopus roborovskii, Cricetulus griseus), ferrets (Mustela putorius furo) minks (Neovison vison) (Shuai et al., 2021)and non-human primates (Macaca mulatta, Macaca fascicularis, Chlorocebus aethiops) (Enkirch and von Messling, 2015; Finch et al., 2020; Gruber et al., 2021; Munster et al., 2020; Rockx et al., 2020). In these models there is no need for genetic modifications in order to study COVID-19 progression. Nevertheless, the models are less frequently used either due to lack of research tools, limited availability, high costs, complex husbandry or associated ethical concerns.
3.12 Murinised SARS-CoV2
While most efforts have been made in generating mouse models humanising the ACE2 to potentiate study of entry and infection in vivo, efforts have also been made in murinising the SARS-CoV2 virus itself. In a study by Muruato et al. (2021), they used a reverse genetic system and in vivo adaptation to successfully generate SARS-CoV2 strains capable of infecting mice (Muruato et al., 2021). Following infection of the murinised SARS-CoV2 strain the mouse lung exhibited substantial damage manifested with inflammation, immune infiltration, and pneumonia. The infection with the adapted virus was however only exhibited in the upper respiratory tract, thus is inappropriate for studies focusing on multisystem infection. It is worth mentioning that the novel adaptation of the virus was shown to keep its ability to infect human airway cells (Muruato et al., 2021). This system, with a murinised SARS-CoV and a standard wild type laboratory mouse, overcomes tropism leading to encephalitis seen in infected transgenic mouse models whilst offering a system applicable for both in vivo mouse studies and in vitro studies on human primary cells (McCray et al., 2007; Winkler et al., 2020). In a study from 2020 the investigators had also produced a murinised SARS-CoV2 via reverse genetics to remodel the interaction between the mouse ACE2 and the virus which resulted in a recombinant virus able to infect the BALB/c mice. It was able replicate in both young and old mice however leading to more severe disease in older mice and exhibiting more clinically relevant phenotypes as compared to the disease presentation between non-modified SARS-CoV2 and transgenic mouse models (Dinnon et al., 2020). This gives the murinised ACE2 system better face validity, however the construct validity is decreased.
4 Recent Translational Applications of Rodent Models Susceptible to SARS-CoV2
The transgenic and transiently sensitised, humanised mouse models of SARS-CoV2 infection have gifted scientists the opportunity to study the potential destruction this, so far, relentless virus can cause to its host in vivo. Towards the beginning of the pandemic, initial studies using these models focused on the mechanisms in which viral entry can occur as well as their points of entry, the tissues primarily affected and the pathology of those tissues. These ongoing attempts to recreate infection have assisted our understanding of the infection timeline and has provided a guide to possible symptoms to be aware of in COVID-19 patients. Discussed here are animal studies performed in order to obtain risk assessments of new variants and evaluate the efficacy and safety of candidate anti-viral drugs for treatment in COVID-19 patients.
4.1 Risk Assessments of Variants of Concern
Particular mutations in the RBD have been key to identifying variants of concern. N501Y is one substitution that is characteristic of the Alpha (B.1.1.7) variant but is also found in the Beta (B.1.351), Gamma (B.1.1.28) and Omicron (B.1.1.529) variants (European Centre for Disease Prevention and Control, 2021; He et al., 2021). This means that N501Y is present in all but one variant of concern. In silico models predicted that this substitution occurs at a key residue for the RBD that is directly responsible for its strengthened affinity for ACE2 (Shahhosseini et al., 2021). The influence of N501Y was proved using a hamster model, where both donors and recipients inoculated with virus carrying N501Y showed significantly increased viral load in nasal washes and lung and trachea homogenates at 1–4 dpi compared to virus carrying a predecessor ‘wild-type’ spike protein. Substitutions S982A and D1118H were also shown to decrease viral fitness (Liu et al., 2022). Interestingly, N501Y increases the infectivity of hosts expressing both either hACE2 or mACE2 (Pan et al., 2021), as it has been revealed that variants with this substitution possess an 8-fold higher affinity for the receptor (Bayarri-Olmos et al., 2021). While this demonstrates a key application of using animals in order to evaluate the potential potency of infection with rapidly evolving variants, we need more studies that apply these principles in the established transgenic and sensitised animal models expressing hACE2. This is because, ultimately, we will require data on how mutations in SARS-CoV2 will affect transmission between, and the health of, humans in the future.
Studies of this nature have been carried out. K18-hACE2 mice infected with B.1.1.7 show increased weight loss and hyperthermia earlier compared to mice exposed to B.1.351 or the initial WA-1 variant of concern. However, B.1.1.7 and B.1.351 infected mice display more severe clinical manifestations overall compared to WA-1 in a viral dose-dependent manner, with WA-1 infected mice displaying a 50% lower mortality rate at a dose of 103 pfu (Horspool et al., 2021). Again in K18-hACE2 mice, whilst both WA-1 and B.1.1.7 inoculated intranasally caused COVID-19-like disease in the mice, a lower dose of B.1.1.7 was required to cause a severe disease state (Bayarri-Olmos et al., 2021). In contrast, C57Bl/6J hACE2 knock-in mice display reduced viral load in lung and nasal turbinate, and a more minor lung pathology and inflammatory response on exposure to WA-1, B.1.1.7 or B.1.351 variants compared to the K18-hACE2 model, where viral RNA is concentrated at the epithelia of larger airways (Winkler et al., 2022). This is most likely attributed to the difference in approach of hACE2 expression in these two mouse lines and highlights the benefits of multiple rodent models of infection, chosen depending on the study focus, but also shows how different models could be affected when exposed to differing strains. Here, studies using models that more accurately recreate human infection to novel strains will possess increased extrapolative power.
The appearance of the B.1.1.529 (Omicron) variant in late 2021 came with heightened suspicions whether the current vaccines and therapies in progress would still provide suitable protection against a variant with >30 mutations in the RBD compared to variants described so far (Hodcroft, 2021). Halfmann et al. found that K18-hACE2 mice inoculated with B.1.351 showed significantly increased viral load in nasal turbinate and lung tissue homogenates at 3 dpi, and greater weight loss at 6 dpi compared to B.1.1.529 infected mice (Halfmann et al., 2022), suggesting reduced severity in viral manifestation on infection with the Omicron lineage in comparison to Beta lineage. These studies show the potential of exploiting the current animal models available in order to screen variants with specific mutations to assess their risk to humans, and for practitioners and governments to make appropriate decisions regarding patient care and infection control strategies.
4.2 Screening the Efficacy of Anti-Viral Therapies
The current pandemic has called for the development of anti-viral drugs in order to reduce or eliminate viral infection in, especially, hospitalised COVID-19 patients. Due to the haste in which these drugs are required to ease the pressure of the pandemic on the world, drug development processes for SARS-CoV2 may be accelerated straight to clinical trials in humans, bypassing preclinical animal safety and efficacy studies.
Anti-viral molecules have been tested in a mouse setting though. PF-07304814 is a phosphate prodrug that on administration is processed into its active form PF-00835231, a potent cysteine protease inhibitor of coronavirus 3CLpro, that was originally considered as a treatment for the 2002 SARS-CoV epidemic in 2003 (Hoffman et al., 2020). PF-00835231 is effective against alpha, beta, and gamma coronaviruses by preventing viral replication through inhibition of essential proteolysis by 3CLpro. BALB/C mice infected with SARS-CoV2 MA10 display no weight loss and complete viral elimination when PF-00835231 is administered subcutaneously twice per day at a dose of 300 mg/kg. Initial weight loss is observed in mice receiving 30–100 mg/kg doses, which recovered to the starting weight at 4 dpi with viral load decreasing in dose-dependent manner. Significant decreases in viral load were also measured in SARS-COV2 exposed mice expressing hACE2 on treatment with PF-00835231. Additionally, this trend is also obtained even when treatment was delayed by 1dpi (Boras et al., 2021), highlighting the importance of identifying infection early, especially in high-risk patients. Despite hACE2 being expressed under a CMV promoter, which may not accurately follow the expected human expression of ACE2, this work shows the power of this inhibitor to prevent viral replication and poses a good option for further development into human clinics.
PF-07321332 (Nirmatrelvir) is another 3CLpro inhibitor, which is the active component of the Pfizer-produced PAXLOVID™ (Pfizer, 2021), that gained approval in the UK (Medicines & Healthcare products Regulatory Agency, 2021a) and the United States (U.S. Food and Drug Administration, 2021a) at the end of 2021, and in the EU in January 2022 for treatment of COVID-19 (European Medicines Agency, 2022). An efficacy study in mice investigated the anti-viral activity of PF-07321332 in BALB/C mice infected with mouse-adapted SARS-CoV2 MA10. Mice treated via oral administration were protected from weight loss, had significantly reduced lung viral titre at 4 dpi and showed markedly decreased nucleocaspid presence in lung sections (Owen et al., 2021). Syrian hamsters have been shown to be protected from severe B.1.351 infection when treated with PF-07321332. Significant dose-dependent reductions in viral lung titre and improved weight retention at 4 dpi, as well as lung anatomy closely resembling uninfected hamsters was observed in those treated with PF-07321332. Hamsters were also completely protected from infection when co-housed for 2 days with a B.1.617.2 (Delta) variant positive cage mate when treated with PF-07321332 compared to those not (Abdelnabi et al., 2022). These rodent models support the continuing development and protective ability of PAXLOVIDTM use in COVID-19 patients against multiple variants of concern, including the benefits of easy oral administration. Yet, further validation in humanised ACE2 rodent models, such as the hACE2 model used in Bao et al. (2020a) may be required for increased value in vivo, as the mentioned studies comprised of mouse adapted SARS-CoV2 infection and wild-type Syrian hamsters as part of their models. These studies could also be extended to examine potential side effects or long term ramifications for patients prescribed this anti-viral treatment. Synthesis and study of additional 3CLpro inhibitors with favourable oral, intraperitoneal, and intravenous bioavailability have been reported and trialled in Sprague-Dawley rats and a CRISPR/Cas9 generated hACE2 expressing mice model (Qiao et al., 2021). However more work is required in this area, and PF-07321332 seems to have won the race for clinical trial approval.
Molnupiravir is another anti-viral drug that instead enforces a high mutagenesis rate via integration of its active form, β-D-N4-hydroxycytidine triphosphate (NHC), into viral RNA in the place of cytidine or uridine (Sheahan et al., 2020; Kabinger et al., 2021). It has so far gained approval for at-risk and hospitalised patients in the United States (U.S. Food and Drug Administration, 2021b) and the UK (Medicines & Healthcare products Regulatory Agency, 2021b). NHC shows potent viral inhibition and significantly reduces viral load in cell culture (Zhou et al., 2021) and diminishes weight loss, indicators of lung haemorrhage and lung viral titre at 500 mg/kg dosage in C57Bl/6 mice infected with either mouse adapted SARS-MA15 or MERS-CoV. Importantly, initiating NHC treatment before 24 h post infection showed to be crucial to maintaining reductions in weight loss, lung haemorrhaging, viral lung titre and lung and alveolar injury scores (Sheahan et al., 2020). However, a warning of mutagenic toxicity to host DNA during NHC treatment has been given, where mutations in a reporter gene increased in a dose-dependent manner with NHC. It has been suggested that the possible conversion of NHC to dNHC (2’-deoxyribose form of NHC) could be the cause of this increased mutational rate in the host genome (Zhou et al., 2021), and should be investigated further in an in vivo model focusing on tissues with natural proliferative tendency.
One study utilised immunodeficient mice with hACE2-and hTMPRSS2- expressing human lung tissue implanted in the animals’ backs. This in vivo tissue model was susceptible to SARS-CoV, MERS-CoV and SARS-CoV2 infection, showed histopathological symptoms echoing viral damage and a 1000-fold increase in proinflammatory cytokines. Beginning NHC treatment at 12- and 24-h post-infection was extremely effective at reducing viral load, however if treatment started 12 h prior to infection, viral titre in the implanted human lung tissue was measured at >100,000-fold lower than the vehicle control, bestowing the protective potential of Molnupiravir in high-risk patients (Wahl et al., 2021). Molunipiravir-derived inhibitors have also shown to be effective at impeding SARS-CoV2 transmission in ferrets (Cox et al., 2021), and reducing viral replication and its associated lung pathologies in SARS-CoV2-susceptible Syrian hamsters, with amplified viral RNA mutations detected in hamsters that started treatment 12 h pre-infection compared to 12 h post-infection or vehicle control (Rosenke et al., 2021). When used in combination with Favipiravir, another anti-viral drug that acts through lethal mutagenesis but requires higher doses for optimal SARS-CoV2 suppression (Kaptein et al., 2020), hamsters treated with sub-optimal doses of a Molnupiravir/Favipiravir cocktail displayed lower viral loads than hamsters treated with only one alone, with an implied additional transmission protection from cage-mates (Abdelnabi et al., 2021b).
These examples show that animal models can serve effectively in the screening of anti-virals in the current and future pandemics, and could assist in the recommendation of single or combinational therapies to complement human clinical trials. Finally, Molnupiravir has also shown its high protective ability against the B.1.1.7 and B.1.351 variants in Syrian hamsters (Abdelnabi et al., 2021a) and emphasises NHC’s potent anti-viral mechanism is not dependent on specific sequences in the viral genome which may be mutated in future variants of concern, such is the case with e.g. monoclonal antibody treatment.
5 Future Research Directions
5.1 Risk Factors Suitable for Rodent Research
This pandemic has revealed that certain individuals are risk of developing severe COVID-19 illness or death. Factors such as age, male sex, and ethnicity have been attributed to a tendency to suffer from severe symptoms (Ebinger et al., 2020; Mughal et al., 2020; Williamson et al., 2020), as well as patients with comorbidities such as diabetes, hypertension and obesity (Alguwaihes et al., 2020; Ebinger et al., 2020; Huang et al., 2020; Li X. et al., 2020; Mughal et al., 2020; Williamson et al., 2020; Goyal et al., 2022). Whereas asthma may actually be protective (Avdeev et al., 2020; Skevaki et al., 2020; Zhu et al., 2020). Genetic or induced mouse models of these disease states are already well established, and the opportunity to combine transgenic and sensitised SARS-CoV2 models with models of human conditions potentially vulnerable to COVID-19 is waiting to be seized. This will allow us to further study comorbidities that may aggravate SARS-CoV2 transmission and pathophysiology, or contribute to any long-term damaging effects in humans. The human population is genetically and culturally diverse, but isolating comorbidities or genetic traits for study in a controlled environment will be vital.
5.1.1 Age
A report from early in the pandemic described that every additional 10 years of age associates with a 1.5-fold increased chance of requiring a higher level of hospitalised care during COVID-19 infection (Ebinger et al., 2020). SARS-COV2-related deaths peak in those aged 80+, who possess more than a 20-fold higher chance of death than those aged 50–59 (Williamson et al., 2020). This most likely attributed to an increase in comorbidities with age, even if yet to be detected. Rodents experience a much shorter life span than humans, making them an excellent model for studying age-related changes in COVID-19 research. ACE2 receptor expression has been described to both increase (Baker et al., 2021; Wark et al., 2021) and decrease (Chen et al., 2020; J.; Gu et al., 2021; Xudong et al., 2006; Yoon et al., 2016) with age in humans and rodents. However, Berni Canani et al. (2021) observed no significant differences in ACE2 expression between children <10 years old and adults 20–80 years old, and Li M.-Y. et al. (2020) detected this same trend when comparing expression across multiple tissues in adults above or below 49 years of age. These contradicting reports suggest that ACE2 expression alone may not be a robust marker for identifying severe risk of SARS-CoV2 infection, and other factors in combination with ACE2 receptor expression must possess a decisive role. Comprehensive studies encompassing widespread tissue analysis of ACE2 expression in multiple age groups could well be accomplished to solve this, surely context-dependent, matter in rodent models of infection.
5.1.2 Diabetes
Diabetic patients are at increased risk of hospitalisation and mortality on infection with SARS-CoV2 (Ebinger et al., 2020), and are significantly more likely to require oxygen, intubation, antibiotics or dexamethasone on admission to hospital than non-diabetic patients (Alguwaihes et al., 2020). This is not entirely surprising considering increased cellular glucose levels assists in supporting viral replication (Codo et al., 2020). Overexpression of hACE2 boosts glucose tolerance and pancreatic β-cell function in diabetic mice (Bindom et al., 2010), whilst ACE2−/y knockout mice display impaired glucose tolerance alongside hepatic steatosis (Cao et al., 2016). Infection-induced downregulation of ACE2, and the resulting angiotensin II excess, therefore intensifies an already unbalanced glucose homeostasis. For these reasons, a bi-directional relationship between diabetes and COVID-19 infection has been proposed (Muniangi-Muhitu et al., 2020).
HFD-induced diabetic DPP4H/M male C57Bl/6 mice have been shown to be more vulnerable to severe signs of disease on infection with MERS-CoV when compared to lean controls, displaying prolonged weight loss and lung inflammation up to 21dpi (Kulcsar et al., 2019). Ma et al. (2021) however is the only study we found to date that has addressed the effect of the current SARS-CoV2 in a hACE2 expressing mouse model of diabetes. Ob/ob mice showed greater weight loss and increased lung immune infiltration when compared to non-diabetic mice at 5 dpi. Interestingly, this study also observed higher fasting blood glucose levels in both wild type and ob/ob mice infected with SARS-CoV2, compared to non-infected. Insulin tolerance was also non-significantly reduced in infected ob/ob mice (Ma et al., 2021). This is an alarming observation, and shows the potency of COVID-19 infection to disturb glucose homeostasis, not only in diabetic patients. Given that genetically-, chemically- or diet-induced rodent models of type 1 and type 2 diabetes are well established (King, 2012) in scientific literature, more research utilising hACE2-expressing rodents combined with these diabetic models will be extremely beneficial to understanding the risk posed on diabetic and non-diabetic people, both during and after contracting COVID-19.
5.1.3 Obesity
Obesity is another major risk factor for severe COVID-19 symptoms (Alguwaihes et al., 2020; Goyal et al., 2022) and COVID-19-related hospitalisation and mortality (Popkin et al., 2020). Its involvement in instigating this is likely intertwined with other comorbidities such as diabetes and hypertension. ACE2 is expressed in subcutaneous and visceral adipose tissue (Al-Benna, 2020), and SARS-CoV2 nucleocapsids were detected in up to 5% of adipocytes in a small cohort of deceased COVID-19 patients (Basolo et al., 2022). Consequently, more adipose tissue will lead to surges in viral penetration and illness.
C57Bl/6 male mice fed a high-fat diet (HFD) display higher ACE2 expression in the lungs and trachea but reduced Tmprss2 expression in the oesophagus, whereas obese females display reduced ACE2 expression in the oesophagus and trachea with no differences in lung tissue (Sarver and Wong, 2021). This, at least in rodents, shows how obesity affects the expression of key SARS-CoV2 entry proteins differently in the two sexes, and should be investigated further in order to understand whether human patients should be treated according to sex. Further, HFD-fed rats see a 3.8- and 6-fold increase in lung Ace2 expression, and a 5.1- and 3.4-fold increase in Tmprss2 expression compared to standard and ketogenic diet fed rats, respectively. AT1R and AT2R levels were also significantly increased in HFD fed rats. Interestingly though, mice fed a ketogenic diet saw reduced AT1R expression in pulmonary tissue compared to rats fed standard chow (da Eira et al., 2021), and this type of diet may help to safeguard diabetic or hypertensive humans. It would be meaningful to see further studies into the potential protective effects of certain diets on SARS-CoV2 infected rodent models.
The obesity-prone C57Bl/6N strain can provide valuable information in support of increased weight and diet on disease advancement and severity in SARS-CoV2 infected rodent models. Zhang et al., utilising leptin receptor dysfunctional C57BL/KsJ-db/db mice, observed a maintained 10% weight loss and more severe pulmonary pathology and inflammation in the obese model compared to db/+ controls inoculated with a mouse-adapted SARS-CoV2. Viral load was also significantly higher in obese lungs, nasal turbinates and trachea (Zhang et al., 2021). HFD-fed C57Bl/6N mice transduced with AdV-hACE2 also display more severe lung pathology than lean mice at 10 days post SARS-CoV2 infection, however a more comprehensive inflammatory profile should be included when studying models such as these (Rai et al., 2021).
This presented evidence further supports the role of obesity in severe COVID-19 patients, and in a way embodies the fusion of two pandemics. Researchers may now also look towards rodent models, preferably expressing hACE2 under its namesake promoter, to develop treatments to ease symptoms and reduce mortality in these patients in the short term. Patients may then turn to improve their diet and lifestyle habits post-recovery.
5.1.4 Hypertension
As the ACE2 receptor is responsible for initial SARS-CoV2 cell entry, it is logical that hypertension was among the top clinical presentations in patients suffering from severe COVID-19 (Huang et al., 2020; Li X. et al., 2020), through viral disruption of RAAS. ACE2 usually acts a negative regulator of RAAS, lowering blood pressure with anti-inflammatory effects. A number of RAAS modulators have been tested on rat primary in vitro cultures that principally act to increase or decrease ACE2 mRNA or protein levels (Hu et al., 2021), which in regards to a COVID-19 patient may either encourage additional viral penetration or further increase blood pressure, respectively. ACE inhibitors or ARB drugs however, seem to display a protective effect against SARS-CoV2 infection and in-hospital mortality (Hippisley-Cox et al., 2020). Nevertheless, these types of studies mainly take into account hospital admissions and must account for a large number of comorbidities and variables.
Diet-induced obese C57Bl/6J mice display weight loss, improved glucose tolerance and reduced expression of inflammatory cytokines when treated with ACE inhibitors (Premaratna et al., 2012). ACE−/- mice show a similar trend (Jayasooriya et al., 2008), displaying the potential for this treatment to ease multiple COVID-19-related risk factors at once. There are a high number of inbred, outbred and transgenic rodent models used for hypertension research (Lerman et al., 2019). Jiang et al. (2022) recently published that SARS-CoV2 viral load in the lungs is higher in transgenic hACE2-expressing mice that have been induced into hypertension compared to normotensive hACE2 mice. Further, AT1R blocker treatment improved lung pathology, reduced blood pressure and downregulated IL-6 and TNF-α expression in hypertensive hACE2 mice. This signifies that treatment provided protection to the organs on SARS-CoV2 infection overall, despite increased viral penetration in the heart and kidneys initially at early infection (Jiang et al., 2022).
A recent preprint article reported that the ACE inhibitor Lisinopril can raise the ACE2 expression landscape in the lungs, small intestine, kidney and brain of healthy mice, an effect that persists to at least 21 days post-termination of treatment (Brooks et al., 2022). Captopril, which also acts as an ACE inhibitor, appears to improve lung pathology and reduce inflammation during SARS-CoV2 infection in an angiotensin II-induced hypertensive and hACE2-expressing mouse model, without any detectable effect on viral load (Gao et al., 2021). These reports reinforce the potential but need for further clarification on RAAS modulators in COVID-19 research, but studies focusing specifically on hypertension in rodents on infection with the SARS-CoV2 virus are lacking. Nonetheless, with blood pressure measurements by techniques such as tail-cuff plethysmography and radiotelemetry readily available for use in rodents (Burger et al., 2014) and with a number of hypertension remedies on the market, future COVID-19 animal research focused on hypertension risk or the efficacy of RAAS modulators would benefit from integrating these methodologies into their study design for a greater in vivo view of hypertension in the current pandemic.
5.2 Insights Into Long-COVID
Post-acute COVID-19 sequelae or ‘long-COVID’ is a condition in which patients continue to suffer multiple COVID-19-related symptoms weeks or even months after testing negative for the virus, and can come in continuous or relapsing forms. The mechanisms behind symptom persistence are still unclear as presentation varies from patient to patient. Large scale studies from around the globe have witnessed exhaustive lists of symptoms (Davis et al., 2021; Hossain et al., 2021; C. Huang et al., 2021; Pérez-González et al., 2022), with those who were hospitalised or required intensive care during primary infection especially at risk (Xie et al., 2022).
Rodent models of post-acute COVID-19 syndrome have been close to non-existent so far. This is likely due to subsided infections, or death, of animals in the models currently available, and the incorporation of early terminal analysis into experiment design. To more accurately study the long-term effects of SARS-CoV2 infection we require models that are even more ‘comprehensively human’ than those presented in Table 1, which more closely mimic aspects such as our own immune system. Researching viral infections by utilising non-human primates is an attractive option, due to marked similarities in physiology and immune responses to antigens with humans with the possibility for longitudinal studies in controlled environments (Estes et al., 2018). The rhesus macaque, African green monkey and pigtail macaque are susceptible to SARS-CoV2 infection and show mild-moderate COVID-19-associated lung pathologies (Clancy et al., 2021). Further, Böszörményi et al. (2021) observed that infected macaques show worsening lung lesions in CT scans, increases in specific cytokines in plasma, mild to moderate histopathological signs of pneumonia and the presence of viral RNA levels in a myriad of tissues up to 38 dpi, despite all subjects testing negative for SARS-CoV2 after 14 dpi. This suggests that these non-human primates are also susceptible to post-acute COVID-19 in a similar way to humans (Böszörményi et al., 2021).
For many researchers however, rodents are a preferred model based on their lower maintenance costs, shorter gestation period and the wealth of tools for transgenic manipulation. A promising example of a mouse model with a humanised immune system is MISTRG6. These immunodeficient mice express seven human cytokine genes knocked into their respective locus in the mouse’s genome, and tolerate human hematopoietic stem cell engraftment (Rongvaux et al., 2014). MISTRG6 mice that transiently express hACE2 sustain prolonged viral titres and RNA, more severe lung pathology, and immune cell signatures to at least 35dpi of SARS-CoV2 compared to controls, emulating severe COVID-19 disease in humans. Convalescent plasma therapy showed a protective effect in these mice in regards to weight loss and viral clearance, however only prophylactic monoclonal antibody treatment improved prevention of T cell lung infiltration (Sefik et al., 2021). This again highlights the importance of early diagnosis in high risk patients, and it will be interesting to see more therapies tested on this model over longer time periods.
Finally, a recent preprint article has described their tracking of 10-weeks- and 1-year-old BALB/C mice for 120 days post infection with mouse adapted SARS-CoV2 MA10. Younger mice cleared infection twice as fast as older mice, with cytokine responses enduring in the latter until 30 dpi. Interestingly though, mice in the younger age group displayed a greater capability for tissue repair, and Molnupiravir was also effective at reducing disease prevalence in the older age group (Dinnon et al., 2022). Although this study is yet to be peer-reviewed, long-term mouse studies such as this will prove valuable in the fight against post-acute COVID-19 syndrome.
6 Concluding Remarks
The COVID-19 pandemic prompted a global scientific effort to produce a number of diverse animal models that mimic SARS-CoV2 infection. Mice have been and continue to be the preferred model organism used in scientific research due to their easy manipulation, short breeding time and genetic similarity to humans. However, the SARS-CoV2 virus binds inefficiently to the ACE2 receptor in mice thus preventing severe infection. This seemingly large barrier has been surmounted by the generation of transgenic and humanised mouse models. Additionally, efforts have been placed in reverse engineering the virus itself to increase its affinity to the mouse ACE2 receptor and causing COVID-19 symptoms. The aim of this review was to highlight individual COVID-19 mouse models and the tissue-specific replication of the virus and pathophysiology upon infection. We substantiate the review with examples of how these models have been used in regards to risk assessment of novel strains, developing therapeutics and elucidating the mechanisms of risk factors such as old age, diabetes, obesity and hypertension. We believe that this review can be used as a comprised guide for investigators researching which mouse model or which strategy to employ in regards to future COVID-19 research.
Author Contributions
PN, MR, and LS wrote the article with equal contributions. RS supervised.
Conflict of Interest
The authors declare that the research was conducted in the absence of any commercial or financial relationships that could be construed as a potential conflict of interest.
Publisher’s Note
All claims expressed in this article are solely those of the authors and do not necessarily represent those of their affiliated organizations, or those of the publisher, the editors and the reviewers. Any product that may be evaluated in this article, or claim that may be made by its manufacturer, is not guaranteed or endorsed by the publisher.
References
Abdelnabi, R., Foo, C. S., de Jonghe, S., Maes, P., Weynand, B., and Neyts, J. (2021a). Molnupiravir Inhibits Replication of the Emerging SARS-CoV-2 Variants of Concern in a Hamster Infection Model. J. Infect. Dis. 224 (5), 749–753. doi:10.1093/infdis/jiab361
Abdelnabi, R., Foo, C. S., Jochmans, D., Vangeel, L., de Jonghe, S., Augustijns, P., et al. (2022). The Oral Protease Inhibitor (PF-07321332) Protects Syrian Hamsters against Infection with SARS-CoV-2 Variants of Concern. Nat. Commun. 13 (1). doi:10.1038/s41467-022-28354-0
Abdelnabi, R., Foo, C. S., Kaptein, S. J. F., Zhang, X., Do, T. N. D., Langendries, L., et al. (2021b). The Combined Treatment of Molnupiravir and Favipiravir Results in a Potentiation of Antiviral Efficacy in a SARS-CoV-2 Hamster Infection Model. EBioMedicine 72, 103595. doi:10.1016/j.ebiom.2021.103595
Al-Benna, S. (2020). Association of High Level Gene Expression of ACE2 in Adipose Tissue with Mortality of COVID-19 Infection in Obese Patients. Obes. Med. 19, 100283. doi:10.1016/j.obmed.2020.100283
Alguwaihes, A. M., Al-Sofiani, M. E., Megdad, M., Albader, S. S., Alsari, M. H., Alelayan, A., et al. (2020). Diabetes and Covid-19 Among Hospitalized Patients in Saudi Arabia: a Single-centre Retrospective Study. Cardiovasc. Diabetol. 19 (1), 205. doi:10.1186/s12933-020-01184-4
Alsaadi, E. J. A., and Jones, I. M. (2019). Membrane Binding Proteins of Coronaviruses. Future Virol. 14 (4), 275–286. doi:10.2217/fvl-2018-0144
Asaka, M. N., Utsumi, D., Kamada, H., Nagata, S., Nakachi, Y., Yamaguchi, T., et al. (2021). Highly Susceptible SARS-CoV-2 Model in CAG Promoter-Driven hACE2-Transgenic Mice. JCI Insight 6 (19), e152529. doi:10.1172/JCI.INSIGHT.152529
Avdeev, S., Moiseev, S., Brovko, M., Yavorovskiy, A., Umbetova, K., Akulkina, L., et al. (2020). Low Prevalence of Bronchial Asthma and Chronic Obstructive Lung Disease Among Intensive Care Unit Patients with COVID‐19. Allergy 75 (10), 2703–2704. doi:10.1111/all.14420
Baker, S. A., Kwok, S., Berry, G. J., and Montine, T. J. (2021). Angiotensin-converting Enzyme 2 (ACE2) Expression Increases with Age in Patients Requiring Mechanical Ventilation. PLoS ONE 16 (2 February), e0247060. doi:10.1371/journal.pone.0247060
Bao, L., Deng, W., Huang, B., Gao, H., Liu, J., Ren, L., et al. (2020a). The Pathogenicity of SARS-CoV-2 in hACE2 Transgenic Mice. Nature 583 (7818), 830–833. doi:10.1038/s41586-020-2312-y
Bao, L., Gao, H., Deng, W., Lv, Q., Yu, H., Liu, M., et al. (2020b). Transmission of Severe Acute Respiratory Syndrome Coronavirus 2 via Close Contact and Respiratory Droplets Among Human Angiotensin-Converting Enzyme 2 Mice. J. Infect. Dis. 222 (4), 551–555. doi:10.1093/INFDIS/JIAA281
Basolo, A., Poma, A. M., Bonuccelli, D., Proietti, A., Macerola, E., Ugolini, C., et al. (2022). Adipose Tissue in COVID-19: Detection of SARS-CoV-2 in Adipocytes and Activation of the Interferon-Alpha Response. J. Endocrinol. Invest. 45, 1021–1029. doi:10.1007/s40618-022-01742-5
Bayarri-Olmos, R., Johnsen, L. B., Idorn, M., Reinert, L. S., Rosbjerg, A., Vang, S., et al. (2021). The alpha/B.1.1.7 SARS-CoV-2 Variant Exhibits Significantly Higher Affinity for ACE-2 and Requires Lower Inoculation Doses to Cause Disease in K18-hACE2 Mice. ELife 10, e70002. doi:10.7554/eLife.70002
Berni Canani, R., Comegna, M., Paparo, L., Cernera, G., Bruno, C., Strisciuglio, C., et al. (2021). Age-Related Differences in the Expression of Most Relevant Mediators of SARS-CoV-2 Infection in Human Respiratory and Gastrointestinal Tract. Front. Pediatr. 9. doi:10.3389/fped.2021.697390
Bindom, S. M., Hans, C. P., Xia, H., Boulares, A. H., and Lazartigues, E. (2010). Angiotensin I-Converting Enzyme Type 2 (ACE2) Gene Therapy Improves Glycemic Control in Diabetic Mice. Diabetes 59 (10), 2540–2548. doi:10.2337/db09-0782
Boras, B., Jones, R. M., Anson, B. J., Arenson, D., Aschenbrenner, L., Bakowski, M. A., et al. (2021). Preclinical Characterization of an Intravenous Coronavirus 3CL Protease Inhibitor for the Potential Treatment of COVID19. Nat. Commun. 12 (1), 6055. doi:10.1038/s41467-021-26239-2
Böszörményi, K. P., Stammes, M. A., Fagrouch, Z. C., Kiemenyi-Kayere, G., Niphuis, H., Mortier, D., et al. (2021). The post-acute Phase of Sars-Cov-2 Infection in Two Macaque Species Is Associated with Signs of Ongoing Virus Replication and Pathology in Pulmonary and Extrapulmonary Tissues. Viruses 13 (8), 1673. doi:10.3390/v13081673
Brooks, S. D., Smith, R. L., Moreira, A. S., and Ackerman, H. C. (2022). Oral Lisinopril Raises Tissue Levels of ACE2, The SARS-CoV-2 Receptor, in Healthy Male and Female Mice. Front. Pharmacol. 13, 798349. doi:10.3389/fphar.2022.798349
Bruter, A. V., Korshunova, D. S., Kubekina, M. V., Sergiev, P. V., Kalinina, A. A., Ilchuk, L. A., et al. (2021). Novel Transgenic Mice with Cre-dependent Co-expression of GFP and Human ACE2: a Safe Tool for Study of COVID-19 Pathogenesis. Transgenic Res. 30 (3), 289–301. doi:10.1007/S11248-021-00249-8/TABLES/1
Burger, D., Reudelhuber, T. L., Mahajan, A., Chibale, K., Sturrock, E. D., and Touyz, R. M. (2014). Effects of a Domain-Selective ACE Inhibitor in a Mouse Model of Chronic Angiotensin II-dependent Hypertension. Clin. Sci. 127 (1), 57–63. doi:10.1042/CS20130808
Calisher, C. H., Childs, J. E., Field, H. E., Holmes, K. V., and Schountz, T. (2006). Bats: Important Reservoir Hosts of Emerging Viruses. Clin. Microbiol. Rev. 19 (3), 531–545. doi:10.1128/CMR.00017-06
Cao, X., Yang, F., Shi, T., Yuan, M., Xin, Z., Xie, R., et al. (2016). Angiotensin-converting Enzyme 2/angiotensin-(1-7)/Mas axis Activates Akt Signaling to Ameliorate Hepatic Steatosis. Sci. Rep. 6, 21592. doi:10.1038/srep21592
Chan, J. F.-W., Yip, C. C.-Y., To, K. K.-W., Tang, T. H.-C., Wong, S. C.-Y., Leung, K.-H., et al. (2020). Improved Molecular Diagnosis of COVID-19 by the Novel, Highly Sensitive and Specific COVID-19-RdRp/Hel Real-Time Reverse Transcription-PCR Assay Validated In Vitro and with Clinical Specimens. J. Clin. Microbiol. 58 (5), e00310–20. doi:10.1128/JCM.00310-20
Chang, C.-k., Sue, S.-C., Yu, T.-h., Hsieh, C.-M., Tsai, C.-K., Chiang, Y.-C., et al. (2006). Modular Organization of SARS Coronavirus Nucleocapsid Protein. J. Biomed. Sci. 13 (1), 59–72. doi:10.1007/s11373-005-9035-9
Chen, J., Jiang, Q., Xia, X., Liu, K., Yu, Z., Tao, W., et al. (2020). Individual Variation of the SARS‐CoV‐2 Receptor ACE2 Gene Expression and Regulation. Aging Cell 19 (7), e13168. doi:10.1111/acel.13168
Clancy, C. S., Shaia, C., Munster, V., de Wit, E., Hawman, D., Okumura, A., et al. (2021). Histologic Pulmonary Lesions of SARS-CoV-2 in 4 Nonhuman Primate Species: An Institutional Comparative Review. Vet. Pathol., 3009858211067468. doi:10.1177/03009858211067468
Codo, A. C., Davanzo, G. G., Monteiro, L. d. B., de Souza, G. F., Muraro, S. P., Virgilio-da-Silva, J. V., et al. (2020). Elevated Glucose Levels Favor SARS-CoV-2 Infection and Monocyte Response through a HIF-1α/Glycolysis-dependent Axis. Cel Metab. 32 (3), 437–446. e5. doi:10.1016/j.cmet.2020.07.007
Cox, R. M., Wolf, J. D., and Plemper, R. K. (2021). Therapeutically Administered Ribonucleoside Analogue MK-4482/EIDD-2801 Blocks SARS-CoV-2 Transmission in Ferrets. Nat. Microbiol. 6 (1), 11–18. doi:10.1038/s41564-020-00835-2
Czech Centre for Phenogenomics (2021). Covid-19 Mouse Models Available at CCP - Czech Centre for Phenogenomics. Vestec, Czechia. https://www.phenogenomics.cz/2021/09/covid-19-mouse-models-available-at-ccp/.
da Eira, D., Jani, S., and Ceddia, R. B. (2021). Obesogenic and Ketogenic Diets Distinctly Regulate the SARS-CoV-2 Entry Proteins ACE2 and TMPRSS2 and the Renin-Angiotensin System in Rat Lung and Heart Tissues. Nutrients 13 (10), 3357. doi:10.3390/nu13103357
Davis, H. E., Assaf, G. S., McCorkell, L., Wei, H., Low, R. J., Re'em, Y., et al. (2021). Characterizing Long COVID in an International Cohort: 7 Months of Symptoms and Their Impact. EClinicalMedicine 38, 101019. doi:10.1016/j.eclinm.2021.101019
De Gasparo, R., Pedotti, M., Simonelli, L., Nickl, P., Muecksch, F., Cassaniti, I., et al. (2021). Bispecific IgG Neutralizes SARS-CoV-2 Variants and Prevents Escape in Mice. Nature 593 (7859), 424–428. doi:10.1038/s41586-021-03461-y
Dinnon, K. H., Leist, S. R., Okuda, K., Dang, H., Fritch, E. J., Gully, K. L., et al. (2022). A Model of Persistent post SARS-CoV-2 Induced Lung Disease for Target Identification and Testing of Therapeutic Strategies. BioRxiv. doi:10.1101/2022.02.15.480515
Dinnon, K. H., Leist, S. R., Schäfer, A., Edwards, C. E., Martinez, D. R., Montgomery, S. A., et al. (2020). A Mouse-Adapted Model of SARS-CoV-2 to Test COVID-19 Countermeasures. Nature 586 (7830), 560–566. doi:10.1038/s41586-020-2708-8
Dolskiy, A. A., Gudymo, A. S., Taranov, O. S., Grishchenko, I. V., Shitik, E. M., Prokopov, D. Y., et al. (2022). The Tissue Distribution of SARS-CoV-2 in Transgenic Mice with Inducible Ubiquitous Expression of hACE2. Front. Mol. Biosciences 8, 1339. doi:10.3389/FMOLB.2021.821506/BIBTEX
Ebinger, J. E., Achamallah, N., Ji, H., Claggett, B. L., Sun, N., Botting, P., et al. (2020). Pre-existing Traits Associated with Covid-19 Illness Severity. PLoS ONE 15 (7 July), e0236240. doi:10.1371/journal.pone.0236240
Enkirch, T., and von Messling, V. (2015). Ferret Models of Viral Pathogenesis. Virology 479-480, 259–270. doi:10.1016/J.VIROL.2015.03.017
Estes, J. D., Wong, S. W., and Brenchley, J. M. (2018). Nonhuman Primate Models of Human Viral Infections. Nat. Rev. Immunol. 18 (6), 390–404. doi:10.1038/s41577-018-0005-7
European Centre for Disease Prevention and Control (2021). Assessment of the Further Emergence of the SARS-CoV-2 Omicron VOC in the Context of the Ongoing Delta VOC Transmission in the EU/EEA, 18th Update. Stockholm, Sweden: ECDC. Available at: https://www.ecdc.europa.eu/en/publications-data/covid-19-assessment-further-emergence-omicron-18th-risk-assessment (Accessed February 25, 2022).
European Medicines Agency (2021). EMA Issues Advice on Use of Lagevrio (Molnupiravir) for the Treatment of COVID-19. Amsterdam, Netherlands: European Medicines Agency. Available at: https://www.ema.europa.eu/en/news/ema-issues-advice-use-lagevrio-molnupiravir-treatment-covid-19 (Accessed February 10, 2022).
European Medicines Agency (2022). Paxlovid. European Medicines Agency. Available at: https://www.ema.europa.eu/en/medicines/human/EPAR/paxlovid (Accessed February 11, 2022).
Fehr, A. R., and Perlman, S. (2015). Coronaviruses: an Overview of Their Replication and Pathogenesis. Methods Mol. Biol. 1282, 1–23. doi:10.1007/978-1-4939-2438-7_1
Finch, C. L., Crozier, I., Lee, J. H., Byrum, R., Cooper, T. K., Liang, J., et al. (2020). Characteristic and Quantifiable COVID-19-like Abnormalities in CT- and PET/CT-imaged Lungs of SARS-CoV-2-Infected Crab-Eating Macaques (Macaca fascicularis). BioRxiv. doi:10.1101/2020.05.14.096727
Forni, D., Cagliani, R., Clerici, M., and Sironi, M. (2017). Molecular Evolution of Human Coronavirus Genomes. Trends Microbiol. 25 (1), 35–48. doi:10.1016/j.tim.2016.09.001
Gao, W.-C., Ma, X., Wang, P., Ma, X., Wang, P., He, X.-Y., et al. (2021). Captopril Alleviates Lung Inflammation in SARS-CoV-2-Infected Hypertensive Mice. Zoolog. Res. 42 (5), 633–636. doi:10.24272/J.ISSN.2095-8137.2021.206
Gavriatopoulou, M., Ntanasis-Stathopoulos, I., Korompoki, E., Fotiou, D., Migkou, M., Tzanninis, I.-G., et al. (2021). Emerging Treatment Strategies for COVID-19 Infection. Clin. Exp. Med. 21 (2), 167–179. doi:10.1007/s10238-020-00671-y
Goyal, A., Gupta, Y., Kalaivani, M., Praveen, P. A., Ambekar, S., and Tandon, N. (2022). SARS-CoV-2 Seroprevalence in Individuals with Type 1 and Type 2 Diabetes Compared with Controls. Endocr. Pract. 28 (2), 191–198. doi:10.1016/j.eprac.2021.12.009
Gruber, A. D., Firsching, T. C., Trimpert, J., and Dietert, K. (2021). Hamster Models of COVID-19 Pneumonia Reviewed: How Human Can They Be? Vet. Pathol., 030098582110571. doi:10.1177/03009858211057197
Gu, H., Chen, Q., Yang, G., He, L., Fan, H., Deng, Y.-Q., et al. (2020). Rapid Adaptation of SARS-CoV-2 in BALB/c Mice: Novel Mouse Model for Vaccine Efficacy. BioRxiv. doi:10.1101/2020.05.02.073411
Gu, J., Yin, J., Zhang, M., Li, J., Wu, Y., Chen, J., et al. (2021). Study on the Clinical Significance of Ace2 and its Age-Related Expression. Jir Vol. 14, 2873–2882. doi:10.2147/JIR.S315981
Guo, T., Fan, Y., Chen, M., Wu, X., Zhang, L., He, T., et al. (2020). Cardiovascular Implications of Fatal Outcomes of Patients with Coronavirus Disease 2019 (COVID-19). JAMA Cardiol. 5 (7), 811–818. doi:10.1001/jamacardio.2020.1017
Halfmann, P. J., Iida, S., Iwatsuki-Horimoto, K., Maemura, T., Kiso, M., Scheaffer, S. M., et al. (2022). SARS-CoV-2 Omicron Virus Causes Attenuated Disease in Mice and Hamsters. Nature 603, 687–692. doi:10.1038/s41586-022-04441-6
Hassan, A. O., Case, J. B., Winkler, E. S., Thackray, L. B., Kafai, N. M., Bailey, A. L., et al. (2020). A SARS-CoV-2 Infection Model in Mice Demonstrates Protection by Neutralizing Antibodies. Cell 182 (3), 744–753. doi:10.1016/J.CELL.2020.06.011
He, X., Hong, W., Pan, X., Lu, G., and Wei, X. (2021). SARS‐CoV‐2 Omicron Variant: Characteristics and Prevention. MedComm 2 (4), 838–845. doi:10.1002/mco2.110
Hippisley-Cox, J., Young, D., Coupland, C., Channon, K. M., Tan, P. S., Harrison, D. A., et al. (2020). Risk of Severe COVID-19 Disease with ACE Inhibitors and Angiotensin Receptor Blockers: Cohort Study Including 8.3 Million People. Heart 106 (19), 1503–1511. doi:10.1136/heartjnl-2020-317393
Hodcroft, E. B. (2021). CoVariants: SARS-CoV-2 Mutations and Variants of Interest. Available at https://covariants.org/ (Accessed February 10, 2022).
Hoffman, R. L., Kania, R. S., Brothers, M. A., Davies, J. F., Ferre, R. A., Gajiwala, K. S., et al. (2020). Discovery of Ketone-Based Covalent Inhibitors of Coronavirus 3CL Proteases for the Potential Therapeutic Treatment of COVID-19. J. Med. Chem. 63 (21), 12725–12747. doi:10.1021/acs.jmedchem.0c01063
Hoffmann, M., Kleine-Weber, H., Schroeder, S., Krüger, N., Herrler, T., Erichsen, S., et al. (2020). SARS-CoV-2 Cell Entry Depends on ACE2 and TMPRSS2 and Is Blocked by a Clinically Proven Protease Inhibitor. Cell 181 (2), 271–280. e8. doi:10.1016/j.cell.2020.02.052
Horspool, A. M., Ye, C., Wong, T. Y., Russ, B. P., Lee, K. S., Winters, M. T., et al. (2021). SARS-CoV-2 B.1.1.7 and B.1.351 Variants of Concern Induce Lethal Disease in K18-hACE2 Transgenic Mice Despite Convalescent Plasma Therapy. BioRxiv. doi:10.1101/2021.05.05.442784
Hossain, M. A., Hossain, K. M. A., Saunders, K., Uddin, Z., Walton, L. M., Raigangar, V., et al. (2021). Prevalence of Long COVID Symptoms in Bangladesh: A Prospective Inception Cohort Study of COVID-19 Survivors. BMJ Glob. Health 6 (12), e006838. doi:10.1136/bmjgh-2021-006838
Hu, Y., Liu, L., and Lu, X. (2021). Regulation of Angiotensin-Converting Enzyme 2: A Potential Target to Prevent COVID-19? Front. Endocrinol. 12. doi:10.3389/fendo.2021.725967
Huang, C., Huang, L., Wang, Y., Li, X., Ren, L., Gu, X., et al. (2021). 6-month Consequences of COVID-19 in Patients Discharged from Hospital: a Cohort Study. The Lancet 397 (10270), 220–232. doi:10.1016/S0140-6736(20)32656-8
Huang, S., Wang, J., Liu, F., Liu, J., Cao, G., Yang, C., et al. (2020). COVID-19 Patients with Hypertension Have More Severe Disease: a Multicenter Retrospective Observational Study. Hypertens. Res. 43 (8), 824–831. doi:10.1038/s41440-020-0485-2
Israelow, B., Song, E., Mao, T., Lu, P., Meir, A., Liu, F., et al. (2020). Mouse Model of SARS-CoV-2 Reveals Inflammatory Role of Type I Interferon Signaling. J. Exp. Med. 217 (12). doi:10.1084/JEM.20201241
Jackson, C. B., Farzan, M., Chen, B., and Choe, H. (2022). Mechanisms of SARS-CoV-2 Entry into Cells. Nat. Rev. Mol. Cel Biol 23 (1), 3–20. doi:10.1038/s41580-021-00418-x
Jayasooriya, A. P., Mathai, M. L., Walker, L. L., Begg, D. P., Denton, D. A., Cameron-Smith, D., et al. (2008). Mice Lacking Angiotensin-Converting Enzyme Have Increased Energy Expenditure, with Reduced Fat Mass and Improved Glucose Clearance. Proc. Natl. Acad. Sci. U.S.A. 105 (18), 6531–6536. www.pnas.orgcgi. doi:10.1073/pnas.0802690105
Jia, H., Yue, X., and Lazartigues, E. (2020). ACE2 Mouse Models: a Toolbox for Cardiovascular and Pulmonary Research. Nat. Commun. 11 (1), 5165. doi:10.1038/s41467-020-18880-0
Jiang, R.-D., Di, Liu, M.-Q., Liu, Y., Shan, C., Zhou, Y.-W., Shen, X.-R., et al. (2020). Pathogenesis of SARS-CoV-2 in Transgenic Mice Expressing Human Angiotensin-Converting Enzyme 2. Cell 182 (1), 50–58. e8. doi:10.1016/J.CELL.2020.05.027
Jiang, X., Li, H., Liu, Y., Bao, L., Zhan, L., Gao, H., et al. (2022). The Effects of ATIR Blocker on the Severity of COVID-19 in Hypertensive Inpatients and Virulence of SARS-CoV-2 in Hypertensive hACE2 Transgenic Mice. J. Cardiovasc. Trans. Res. 15, 38–48. doi:10.1007/s12265-021-10147-3
Kabinger, F., Stiller, C., Schmitzová, J., Dienemann, C., Kokic, G., Hillen, H. S., et al. (2021). Mechanism of Molnupiravir-Induced SARS-CoV-2 Mutagenesis. Nat. Struct. Mol. Biol. 28 (9), 740–746. doi:10.1038/s41594-021-00651-0
Kaptein, S. J. F., Jacobs, S., Langendries, L., Seldeslachts, L., ter Horst, S., Liesenborghs, L., et al. (2020). Favipiravir at High Doses Has Potent Antiviral Activity in SARS-CoV-2−infected Hamsters, whereas Hydroxychloroquine Lacks Activity. Proc. Natl. Acad. Sci. U.S.A. 117 (43), 26955–26965. doi:10.1073/pnas.2014441117/-/DCSupplemental
Katzman, C., Israely, T., Melamed, S., Politi, B., Sittner, A., Yahalom-Ronen, Y., et al. (2022). Modeling Sars-Cov-2 Infection in Mice Using Lentiviral Hace2 Vectors Infers Two Modes of Immune Responses to Sars-Cov-2 Infection. Viruses 14 (1), 11. doi:10.3390/V14010011/S1
King, A. J. (2012). The Use of Animal Models in Diabetes Research. Br. J. Pharamacology 166, 877–894. doi:10.1111/j.1476-5381.2012.01911.x
Kovacech, B., Fialova, L., Filipcik, P., Skrabana, R., Zilkova, M., Paulenka-Ivanovova, N., et al. (2022). Monoclonal Antibodies Targeting Two Immunodominant Epitopes on the Spike Protein Neutralize Emerging SARS-CoV-2 Variants of Concern. EBioMedicine 76, 103818. doi:10.1016/j10.1016/j.ebiom.2022.103818
Kulcsar, K. A., Coleman, C. M., Beck, S. E., and Frieman, M. B. (2019). Comorbid Diabetes Results in Immune Dysregulation and Enhanced Disease Severity Following MERS-CoV Infection. JCI Insight 4 (20), e131774. doi:10.1172/jci.insight.131774
Leclerc, Q. J., Fuller, N. M., Knight, L. E., Funk, S., Knight, G. M., and Knight, G. M. (2020). What Settings Have Been Linked to SARS-CoV-2 Transmission Clusters? Wellcome Open Res. 5, 83. doi:10.12688/wellcomeopenres.15889.1
Lerman, L. O., Kurtz, T. W., Touyz, R. M., Ellison, D. H., Chade, A. R., Crowley, S. D., et al. (2019). Animal Models of Hypertension: A Scientific Statement from the American Heart Association. Hypertension 73 (6), e87–e120. doi:10.1161/HYP.0000000000000090
Li, M.-Y., Li, L., Zhang, Y., and Wang, X.-S. (2020). Expression of the SARS-CoV-2 Cell Receptor Gene ACE2 in a Wide Variety of Human Tissues. Infect. Dis. Poverty 9 (1). doi:10.1186/s40249-020-00662-x
Li, X., Xu, S., Yu, M., Wang, K., Tao, Y., Zhou, Y., et al. (2020). Risk Factors for Severity and Mortality in Adult COVID-19 Inpatients in Wuhan. J. Allergy Clin. Immunol. 146 (1), 110–118. doi:10.1016/j.jaci.2020.04.006
Liu, Y., Liu, J., Plante, K. S., Plante, J. A., Xie, X., Zhang, X., et al. (2022). The N501Y Spike Substitution Enhances SARS-CoV-2 Infection and Transmission. Nature 602 (7896), 294–299. doi:10.1038/s41586-021-04245-0
Lu, R., Zhao, X., Li, J., Niu, P., Yang, B., Wu, H., et al. (2020). Genomic Characterisation and Epidemiology of 2019 Novel Coronavirus: Implications for Virus Origins and Receptor Binding. The Lancet 395 (10224), 565–574. doi:10.1016/s0140-6736(20)30251-8
Ma, Y., Lu, D., Bao, L., Qu, Y., Liu, J., Qi, X., et al. (2021). SARS‐CoV‐2 Infection Aggravates Chronic Comorbidities of Cardiovascular Diseases and Diabetes in Mice. Anim. Models Exp. Med. 4 (1), 2–15. doi:10.1002/ame2.12155
McCray, P. B., Pewe, L., Wohlford-Lenane, C., Hickey, M., Manzel, L., Shi, L., et al. (2007). Lethal Infection of K18- hACE2 Mice Infected with Severe Acute Respiratory Syndrome Coronavirus. J. Virol. 81 (2), 813–821. doi:10.1128/JVI.02012-06
Medicines & Healthcare products Regulatory Agency (2021b). First Oral Antiviral for COVID-19, Lagevrio (Molnupiravir), Approved by MHRA. [Press release]London, United Kingdom. Available at: https://www.gov.uk/government/news/first-oral-antiviral-for-covid-19-lagevrio-molnupiravir-approved-by-mhra (Accessed February 24, 2022).
Medicines & Healthcare products Regulatory Agency (2021a). Oral COVID-19 Antiviral, Paxlovid, Approved by UK regulator. [Press release]. London, United Kingdom (Available at: https://www.gov.uk/government/news/oral-covid-19-antiviral-paxlovid-approved-by-uk-regulator (Accessed February 24, 2022).
Menachery, V. D., Yount, B. L., Sims, A. C., Debbink, K., Agnihothram, S. S., Gralinski, L. E., et al. (2016). SARS-like WIV1-CoV Poised for Human Emergence. Proc. Natl. Acad. Sci. U.S.A. 113 (11), 3048–3053. doi:10.1073/PNAS.1517719113
Mughal, M. S., Kaur, I. P., Jaffery, A. R., Dalmacion, D. L., Wang, C., Koyoda, S., et al. (2020). COVID-19 Patients in a Tertiary US Hospital: Assessment of Clinical Course and Predictors of the Disease Severity. Respir. Med. 172, 106130. doi:10.1016/j.rmed.2020.106130
Muniangi-Muhitu, H., Akalestou, E., Salem, V., Misra, S., Oliver, N. S., and Rutter, G. A. (2020). Covid-19 and Diabetes: A Complex Bidirectional Relationship. Front. Endocrinol. 11. doi:10.3389/fendo.2020.582936
Munster, V. J., Feldmann, F., Williamson, B. N., van Doremalen, N., Pérez-Pérez, L., Schulz, J., et al. (2020). Respiratory Disease in Rhesus Macaques Inoculated with SARS-CoV-2. Nature 585 (7824), 268–272. doi:10.1038/S41586-020-2324-7
Muruato, A., Vu, M. N., Johnson, B. A., Davis-Gardner, M. E., Vanderheiden, A., Lokugamage, K., et al. (2021). Mouse-adapted SARS-CoV-2 Protects Animals from Lethal SARS-CoV challenge. Plos Biol. 19 (11), e3001284. doi:10.1371/journal.pbio.3001284
Nehme, A., Zouein, F. A., Zayeri, Z. D., and Zibara, K. (2019). An Update on the Tissue Renin Angiotensin System and its Role in Physiology and Pathology. Jcdd 6 (2), 14. doi:10.3390/jcdd6020014
Owen, D. R., Allerton, C. M. N., Anderson, A. S., Aschenbrenner, L., Avery, M., Berritt, S., et al. (2021). An Oral SARS-CoV-2 M Pro Inhibitor Clinical Candidate for the Treatment of COVID-19. Science 374 (6575), 1586–1593. https://www.science.org. doi:10.1126/science.abl4784
Pan, T., Chen, R., He, X., Yuan, Y., Deng, X., Li, R., et al. (2021). Infection of Wild-type Mice by SARS-CoV-2 B.1.351 Variant Indicates a Possible Novel Cross-Species Transmission Route. Sig Transduct Target. Ther. 6 (1), 420. doi:10.1038/s41392-021-00848-1
Pérez-González, A., Araújo-Ameijeiras, A., Fernández-Villar, A., Crespo, M., Poveda, E., Cabrera, J. J., et al. (2022). Long COVID in Hospitalized and Non-hospitalized Patients in a Large Cohort in Northwest Spain, a Prospective Cohort Study. Sci. Rep. 12 (1), 3369. doi:10.1038/s41598-022-07414-x
Popkin, B. M., Du, S., Green, W. D., Beck, M. A., Algaith, T., Herbst, C. H., et al. (2020). Individuals with Obesity and COVID‐19: A Global Perspective on the Epidemiology and Biological Relationships. Obes. Rev. 21 (11), e13128. doi:10.1111/obr.13128
Premaratna, S. D., Manickam, E., Begg, D. P., Rayment, D. J., Hafandi, A., Jois, M., et al. (2012). Angiotensin-converting Enzyme Inhibition Reverses Diet-Induced Obesity, Insulin Resistance and Inflammation in C57BL/6J Mice. Int. J. Obes. 36 (2), 233–243. doi:10.1038/ijo.2011.95
Qiao, J., Li, Y.-S., Zeng, R., Liu, F.-L., Luo, R.-H., Huang, C., et al. (2021). SARS-CoV-2 M Pro Inhibitors with Antiviral Activity in a Transgenic Mouse Model. Science 371 (6536), 1374–1378. https://pymol.org. doi:10.1126/science.abf1611
Qing, E., and Gallagher, T. (2020). SARS Coronavirus Redux. Trends Immunol. 41 (4), 271–273. doi:10.1016/j.it.2020.02.007
Ragab, D., Salah Eldin, H., Taeimah, M., Khattab, R., and Salem, R. (2020). The COVID-19 Cytokine Storm; what We Know So Far. Front. Immunol. 11. doi:10.3389/fimmu.2020.01446
Rai, P., Chuong, C., LeRoith, T., Smyth, J. W., Panov, J., Levi, M., et al. (2021). Adenovirus Transduction to Express Human ACE2 Causes Obesity-specific Morbidity in Mice, Impeding Studies on the Effect of Host Nutritional Status on SARS-CoV-2 Pathogenesis. Virology 563, 98–106. doi:10.1016/j.virol.2021.08.014
Rathnasinghe, R., Strohmeier, S., Amanat, F., Gillespie, V. L., Krammer, F., García-Sastre, A., et al. (2020). Comparison of Transgenic and Adenovirus hACE2 Mouse Models for SARS-CoV-2 Infection. BioRxiv. doi:10.1101/2020.07.06.190066
Rawle, D. J., Le, T. T., Dumenil, T., Yan, K., Tang, B., Nguyen, W., et al. (2021). ACE2-lentiviral Transduction Enables Mouse SARS-CoV-2 Infection and Mapping of Receptor Interactions. Plos Pathog. 17 (7), e1009723. doi:10.1371/JOURNAL.PPAT.1009723
Rockx, B., Kuiken, T., Herfst, S., Bestebroer, T., Lamers, M. M., Oude Munnink, B. B., et al. (2020). Comparative Pathogenesis of COVID-19, MERS, and SARS in a Nonhuman Primate Model. Science 368 (6494), 1012–1015. doi:10.1126/SCIENCE.ABB7314
Rongvaux, A., Willinger, T., Martinek, J., Strowig, T., Gearty, S. v., Teichmann, L. L., et al. (2014). Development and Function of Human Innate Immune Cells in a Humanized Mouse Model. Nat. Biotechnol. 32 (4), 364–372. doi:10.1038/nbt.2858
Rosenke, K., Hansen, F., Schwarz, B., Feldmann, F., Haddock, E., Rosenke, R., et al. (2021). Orally Delivered MK-4482 Inhibits SARS-CoV-2 Replication in the Syrian Hamster Model. Nat. Commun. 12 (1). doi:10.1038/s41467-021-22580-8
Samavati, L., and Uhal, B. D. (2020). ACE2, Much More Than Just a Receptor for SARS-COV-2. Front. Cell Infect. Microbiol. 10. doi:10.3389/fcimb.2020.00317
Sarver, D. C., and Wong, G. W. (2021). Obesity Alters Ace2 and Tmprss2 Expression in Lung, Trachea, and Esophagus in a Sex-dependent Manner: Implications for COVID-19. Biochem. Biophysical Res. Commun. 538, 92–96. doi:10.1016/j.bbrc.2020.10.066
Sefik, E., Israelow, B., Mirza, H., Zhao, J., Qu, R., Kaffe, E., et al. (2021). A Humanized Mouse Model of Chronic COVID-19. Nat. Biotechnol. doi:10.1038/s41587-021-01155-4
Sellers, R. S. (2017). Translating Mouse Models. Toxicol. Pathol. 45 (1), 134–145. doi:10.1177/0192623316675767
Shahhosseini, N., Babuadze, G., Wong, G., and Kobinger, G. P. (2021). Mutation Signatures and In Silico Docking of Novel Sars-Cov-2 Variants of Concern. Microorganisms 9 (5), 926. doi:10.3390/microorganisms9050926
Sheahan, T. P., Sims, A. C., Zhou, S., Graham, R. L., Pruijssers, A. J., Agostini, M. L., et al. (2020). An Orally Bioavailable Broad-Spectrum Antiviral Inhibits SARS-CoV-2 in Human Airway Epithelial Cell Cultures and Multiple Coronaviruses in Mice. Sci. Transl. Med. 12 (541). doi:10.1126/SCITRANSLMED.ABB5883
Shou, S., Liu, M., Yang, Y., Kang, N., Song, Y., Tan, D., et al. (2021). Animal Models for COVID-19: Hamsters, Mouse, Ferret, Mink, Tree Shrew, and Non-human Primates. Front. Microbiol. 12. doi:10.3389/fmicb.2021.626553
Shuai, L., Zhong, G., Yuan, Q., Wen, Z., Wang, C., He, X., et al. (2021). Replication, Pathogenicity, and Transmission of SARS-CoV-2 in Minks. Natl. Sci. Rev. 8 (3), 2021. doi:10.1093/NSR/NWAA291
Simmons, G., Gosalia, D. N., Rennekamp, A. J., Reeves, J. D., Diamond, S. L., and Bates, P. (2005). Inhibitors of Cathepsin L Prevent Severe Acute Respiratory Syndrome Coronavirus Entry. Proc. Natl. Acad. Sci. U.S.A. 102 (33), 11876–11881. doi:10.1073/pnas.0505577102
Skevaki, C., Karsonova, A., Karaulov, A., Xie, M., and Renz, H. (2020). Asthma-associated Risk for COVID-19 Development. J. Allergy Clin. Immunol. 146 (6), 1295–1301. doi:10.1016/j.jaci.2020.09.017
Su, Y., Yuan, D., Chen, D. G., Ng, R. H., Wang, K., Choi, J., et al. (2022). Multiple Early Factors Anticipate post-acute COVID-19 Sequelae. Cell 185, 881–895. doi:10.1016/j.cell.2022.01.014
Sun, J., Zhuang, Z., Zheng, J., Li, K., Wong, R. L.-Y., Liu, D., et al. (2020). Generation of a Broadly Useful Model for COVID-19 Pathogenesis, Vaccination, and Treatment. Cell 182 (3), 734–743. e5. doi:10.1016/j.cell.2020.06.010
Sun, S.-H., Chen, Q., Gu, H.-J., Yang, G., Wang, Y.-X., Huang, X.-Y., et al. (2020). A Mouse Model of SARS-CoV-2 Infection and Pathogenesis. Cell Host and Microbe 28 (1), 124–133. e4. doi:10.1016/J.CHOM.2020.05.020/ATTACHMENT/34A485A8-CAD9-409E-AA5B-0EF7B1B54781/MMC1.PDF
Tang, C., Deng, Z., Li, X., Yang, M., Tian, Z., Chen, Z., et al. (2020). Helicase of Type 2 Porcine Reproductive and Respiratory Syndrome Virus Strain HV Reveals a Unique Structure. Viruses 12 (2), 215. doi:10.3390/v12020215
Thomas, S. (2020). The Structure of the Membrane Protein of SARS-CoV-2 Resembles the Sugar Transporter SemiSWEET. Pai 5 (1), 342–363. doi:10.20411/pai.v5i1.377
Triggle, C. R., Bansal, D., Ding, H., Islam, M. M., Farag, E. A. B. A., Hadi, H. A., et al. (2021). A Comprehensive Review of Viral Characteristics, Transmission, Pathophysiology, Immune Response, and Management of SARS-CoV-2 and COVID-19 as a Basis for Controlling the Pandemic. Front. Immunol. 12, 631139. doi:10.3389/fimmu.2021.631139
Tseng, C.-T. K., Huang, C., Newman, P., Wang, N., Narayanan, K., Watts, D. M., et al. (2007). Severe Acute Respiratory Syndrome Coronavirus Infection of Mice Transgenic for the Human Angiotensin-Converting Enzyme 2 Virus Receptor. J. Virol. 81 (3), 1162–1173. doi:10.1128/JVI.01702-06/ASSET/DACA1C30-425C-4D1C-8B34-1CE2B664B6C9/ASSETS/GRAPHIC/ZJV0030787060008.JPEG
Turner, A. J., and Hooper, N. M. (2002). The Angiotensin-Converting Enzyme Gene Family: Genomics and Pharmacology. Trends Pharmacol. Sci. 23 (4), 177–183. doi:10.1016/s0165-6147(00)01994-5
U.S. Food and Drug Administration (2021b). Coronavirus (COVID-19) Update: FDA Authorizes Additional Oral Antiviral for Treatment of COVID-19 in Certain Adults. Silver Spring, MD: FDA. Available at: https://www.fda.gov/news-events/press-announcements/coronavirus-covid-19-update-fda-authorizes-additional-oral-antiviral-treatment-covid-19-certain (Accessed February 11, 2022).
U.S. Food and Drug Administration (2021a). Coronavirus (COVID-19) Update: FDA Authorizes First Oral Antiviral for Treatment of COVID-19. Silver Spring, MD: FDA. Available at: https://www.fda.gov/news-events/press-announcements/coronavirus-covid-19-update-fda-authorizes-first-oral-antiviral-treatment-covid-19 (Accessed February 10, 2022).
Wahl, A., Gralinski, L. E., Johnson, C. E., Yao, W., Kovarova, M., Dinnon, K. H., et al. (2021). SARS-CoV-2 Infection Is Effectively Treated and Prevented by EIDD-2801. Nature 591 (7850), 451–457. doi:10.1038/s41586-021-03312-w
Walls, A. C., Park, Y.-J., Tortorici, M. A., Wall, A., McGuire, A. T., and Veesler, D. (2020). Structure, Function, and Antigenicity of the SARS-CoV-2 Spike Glycoprotein. Cell 181 (2), 281–292. e6. doi:10.1016/j.cell.2020.02.058
Wark, P. A. B., Pathinayake, P. S., Kaiko, G., Nichol, K., Ali, A., Chen, L., et al. (2021). ACE2 Expression Is Elevated in Airway Epithelial Cells from Older and Male Healthy Individuals but Reduced in Asthma. Respirology 26 (5), 442–451. doi:10.1111/resp.14003
Williamson, E. J., Walker, A. J., Bhaskaran, K., Bacon, S., Bates, C., Morton, C. E., et al. (2020). Factors Associated with COVID-19-Related Death Using OpenSAFELY. Nature 584 (7821), 430–436. doi:10.1038/s41586-020-2521-4
Winkler, E. S., Chen, R. E., Alam, F., Yildiz, S., Case, J. B., Uccellini, M. B., et al. (2022). SARS-CoV-2 Causes Lung Infection without Severe Disease in Human ACE2 Knock-In Mice. J. Virol. 96 (1), e0151121. doi:10.1128/JVI10.1128/JVI.01511-21
Winkler, E. S., Bailey, A. L., Kafai, N. M., Nair, S., McCune, B. T., Yu, J., et al. (2020). SARS-CoV-2 Infection of Human ACE2-Transgenic Mice Causes Severe Lung Inflammation and Impaired Function. Nat. Immunol. 21 (11), 1327–1335. doi:10.1038/s41590-020-0778-2
Wrapp, D., Wang, N., Corbett, K. S., Goldsmith, J. A., Hsieh, C.-L., Abiona, O., et al. (2020). Cryo-EM Structure of the 2019-nCoV Spike in the Prefusion Conformation. Science 367 (6483), 1260–1263. doi:10.1126/science.abb2507
Xie, Y., Xu, E., Bowe, B., and Al-Aly, Z. (2022). Long-term Cardiovascular Outcomes of COVID-19. Nat. Med. 28, 583–590. doi:10.1038/s41591-022-01689-3
Xudong, X., Junzhu, C., Xingxiang, W., Furong, Z., and Yanrong, L. (2006). Age- and Gender-Related Difference of ACE2 Expression in Rat Lung. Life Sci. 78 (19), 2166–2171. doi:10.1016/j.lfs.2005.09.038
Yang, X. H., Deng, W., Tong, Z., Liu, Y. X., Zhang, L. F., Zhu, H., et al. (2007b). Mice Transgenic for Human Angiotensin-Converting Enzyme 2 Provide a Model for SARS Coronavirus Infection. Comp. Med. 57 (5), 450–459.
Yang, X. H., Deng, W., Tong, Z., Liu, Y.-x., Zhang, L., and Zhu, H. (2007a). Mice Transgenic for Human Angiotensin-Converting Enzyme 2 Provide a Model for SARS Coronavirus Infection. Comp. Med. 57, 450–459. Ingenta Connect. from https://www.ingentaconnect.com/content/aalas/cm/2007/00000057/00000005/art00003# (Retrieved March 6, 2022).
Yong, S. J. (2021). Long COVID or post-COVID-19 Syndrome: Putative Pathophysiology, Risk Factors, and Treatments. Infect. Dis. 53 (Issue 10), 737–754. doi:10.1080/23744235.2021.1924397
Yoon, H. E., Kim, E. N., Kim, M. Y., Lim, J. H., Jang, I.-A., Ban, T. H., et al. (2016). Age-Associated Changes in the Vascular Renin-Angiotensin System in Mice. Oxidative Med. Cell Longevity 2016, 1–14. doi:10.1155/2016/6731093
Zhang, X., Kousoulas, K. G., and Storz, J. (1992). The Hemagglutinin/esterase Gene of Human Coronavirus Strain OC43: Phylogenetic Relationships to Bovine and Murine Coronaviruses and Influenza C Virus. Virology 186 (1), 318–323. doi:10.1016/0042-6822(92)90089-8
Zhang, Y.-N., Zhang, Z.-R., Zhang, H.-Q., Li, X.-D., Li, J.-Q., Zhang, Q.-Y., et al. (2021). Increased Morbidity of Obese Mice Infected with Mouse-Adapted SARS-CoV-2. Cell Discov 7 (Issue 1), 74. doi:10.1038/s41421-021-00305-x
Zhou, S., Hill, C. S., Sarkar, S., Tse, L. v., Woodburn, B. M. D., Schinazi, R. F., et al. (2021). β-d-N4-hydroxycytidine Inhibits SARS-CoV-2 through Lethal Mutagenesis but Is Also Mutagenic to Mammalian Cells. J. Infect. Dis. 224 (3), 415–419. doi:10.1093/infdis/jiab247
Keywords: COVID-19, mouse, model, SARS-CoV2, sensitised, humanized, mice
Citation: Nickl P, Raishbrook MJ, Syding LA and Sedlacek R (2022) Advances in Modelling COVID-19 in Animals. Front. Drug. Discov. 2:899587. doi: 10.3389/fddsv.2022.899587
Received: 18 March 2022; Accepted: 14 April 2022;
Published: 02 May 2022.
Edited by:
Bruno Villoutreix, Institut National de la Santé et de la Recherche Médicale (INSERM), FranceReviewed by:
Venkata Ramireddy Narala, Yogi Vemana University, IndiaDhanasekaran Sakthivel, ZIP Diagnostics Pty Ltd., Australia
Copyright © 2022 Nickl, Raishbrook, Syding and Sedlacek. This is an open-access article distributed under the terms of the Creative Commons Attribution License (CC BY). The use, distribution or reproduction in other forums is permitted, provided the original author(s) and the copyright owner(s) are credited and that the original publication in this journal is cited, in accordance with accepted academic practice. No use, distribution or reproduction is permitted which does not comply with these terms.
*Correspondence: Radislav Sedlacek, cmFkaXNsYXYuc2VkbGFjZWtAaW1nLmNhcy5jeg==
†These authors have contributed equally to this work and share first authorship