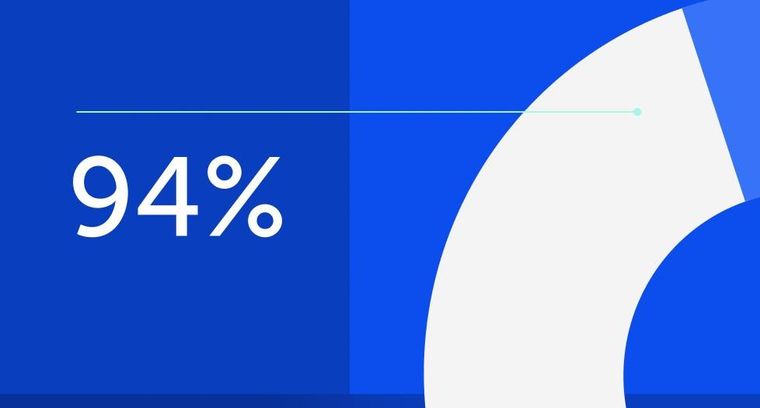
94% of researchers rate our articles as excellent or good
Learn more about the work of our research integrity team to safeguard the quality of each article we publish.
Find out more
REVIEW article
Front. Drug Deliv., 14 June 2023
Sec. Respiratory Drug Delivery
Volume 3 - 2023 | https://doi.org/10.3389/fddev.2023.1209534
Antibiotic resistance is exponentially increasing, and the number of deaths caused by bacterial infections is expected to surge. When dealing with the respiratory system, inefficient antibiotics heighten the chance of death from bacterial infection. However, the alternatives to antibiotics are limited. Bacteriophages are a valid option since they can target a specific type of bacterium. Bacteriophages are highly specific and can avoid any side effects when delivered. However, their poor stability makes their use inefficient. Encapsulation is commonly used to protect any bioactive compound for different types of delivery. In the case of respiratory delivery, particle engineering is used to generate stable dry powders to target the nasal or lung areas. This review article provides a guideline for engineering a process of nasal dry powders of encapsulated bacteriophages.
Antibiotic resistance is a sword of Damocles over many lives; the research community is rightly addressing it seriously. In the United States alone, more than 2.8 million antimicrobial-resistant infections are recorded each year, from which more than 35,000 people die (Stewart and Costerton, 2001). A bacterium that learns how to defeat the attack of antibiotics is called a superbug (Figure 1A)). There are five currently known superbugs: Methicillin-resistant Staphylococcus aureus, extended spectrum beta-lactamase (ESBL)-producing Enterobacteriaceae, Vancomycin-resistant Enterococcus, and multidrug-resistant Pseudomonas aeruginosa. However, this number is expected to grow (Perez et al., 2014).
FIGURE 1. Several bacteria are growing into superbugs, mutating to resist the most common antibiotics (A). Therefore, bacteriophages are increasing their effectiveness in defeating such superbugs by specifically targeting them, injecting their DNA, and reproducing in it (B). The delivery of bacteriophages via the respiratory tract has shown some disadvantages, such as disrupting the bacteriophage head during delivery (C). Thus, encapsulation can protect the bacteriophage once delivered, allowing it to reach the target: the bacteria or biofilms (D).
Bacteriophages—viruses that can infect and kill bacteria—are considered a promising strategy for overcoming antibiotic resistance (Eiserling, 2023). They attach to a specific type of bacterium, release their DNA, and replicate in the bacterial machinery. Later, due to several factors that differ across the type of bacterium and bacteriophage, the bacterium bursts to release virions that are ready to infect and kill other bacteria (Figure 1B) (Loc-Carrillo and Abedon, 2011). The specificity of the bacteria attacked is one of the main advantages of bacteriophages over antibiotics. Bacteriophages are considered safer since they only infect the target bacterium and have no effect on mammalian cells. Moreover, the exponential growth of bacteriophages may mean less frequent and lower doses than required for antibiotics (Sulakvelidze et al., 2001). Furthermore, the development of bacteriophages is relatively more rapid and inexpensive than antibiotic drugs (Parasion et al., 2014).
The most important concept for the success of phages in reaching bacterial targets is ensuring that a minimum inhibitory concentration is surpassed. This can be achieved by directly applying phages to the bacterium or by systemic delivery (Malik et al., 2017). Whenever delivering any bioactive compounds to the human body, large losses of compounds can occur when delivered to the respiratory tract due to degradation or aggregation. Encapsulation is the common method for enhancing compound stability and efficacy for drug delivery (Baldelli et al., 2022a). For phage delivery, the process of encapsulation involves the creation of a shell surrounding one or more bacteriophages. This shell protects the bacteriophages from external conditions and can increase adhesion to specific bacteria (Choińska-Pulit et al., 2015; Baldelli et al., 2022b). This can be extremely beneficial when bacteriophages are to reach the human lungs. The respiratory tract has evolved a mechanism to exclude anything from entering the lung. As shown in Figures 1C and D, the morphology of the airways can lead to bacteriophages being deposited in undesired locations, thus missing the target bacterium (Dasaraju and Liu, 1996). Moreover, the delivery of a free bacteriophage can trigger phage inactivation by the immune system (Żaczek et al., 2016).
Delivering bacteriophages to the respiratory system can be challenging since it can provoke different types of stress on the phages. First, a phage’s stability depends on its ionic strength; thus, any change to this parameter in the respiratory tract can generate severe damage. Second, the process of respiratory delivery can influence the osmotic pressure between the phages’ external and internal parts . Lastly, the mechanical stress involved in preparations, such as high-speed mixing, centrifugation, and spraying, can jeopardize the stability of phages by specifically deteriorating their dentures (Froman et al., 1954). Furthermore, phages, like several other treatments, can trigger the immune system of the nasal environment (Dąbrowska, 2019). Encapsulation can overcome these challenges and increase the delivery efficiency of bacteriophages to the respiratory tract.
Encapsulating a bioactive compound as dry powder can have other benefits, such as ease of use and low costs (Maa et al., 1999). Respiratory diseases such as asthma, cystic fibrosis, and chronic obstructive pulmonary diseases are currently treated using dry powders (Huang et al., 2004). Through particle engineering, scientists can engineer respirable microparticles composed of a wall material—generally a polymer of large molecular weight—and a bulk material—generally sugar or salt (Baldelli et al., 2015). The selection of the wall material influences the dimension and morphology of the microparticles, while the selection of the bulk material can impact the stability and aerosol efficiency of microparticles (Baldelli and Vehring, 2016a; Baldelli et al., 2016). Moreover, the wall material influences the location of particle deposition in the respiratory tract: a highly sticky and cohesive polymer could create aggregates that lead to an undesirable deposition of the drug delivered (Baldelli and Vehring, 2016b). In addition, the bulk material selection needs to be tailored for a specific bioactive compound. For instance, bulk materials that tend to crystallize when drying might damage the chemical structure of the bioactive compound in the inner core of the evaporating droplet (Baldelli et al., 2016; Gomez et al., 2021).
Consequently, selecting the materials and their ratios to properly encapsulate a bacteriophage is important for the efficacy and efficiency of bacteriophage delivery to the respiratory tract. In fact, if not properly planned, encapsulation can have the detrimental effect of greater deterioration of the phages. This review discusses the methods and materials for formulating respirable sprayed microparticles to encapsulate different types of bacteriophages.
Every year, more than 13 million people are affected by respiratory infections, 11.6% of which are provoked by bacteria (Dasaraju and Liu, 1996). These numbers are expected to increase with the antibiotic resistance of bacteria. The most common bacteria that affect the respiratory tract are Streptococcus pneumoniae, Haemophilus influenzae and Moraxella catarrhalis (Bosch et al., 2013). In addition to these, Staphylococcus aureus, Mycobacterium tuberculosis, Pseudomonas aeruginosa, Fusobacterium, Neiserria, and Corynebacterium are other types of bacteria that impact different areas of the respiratory tract (Figure 2) (Prat and Lacoma, 2016). Each bacterium is acquiring resistance to the most common types of antibiotics, such as penicillin, cefaclor, cefuroxime, azithromycin, clarithromycin, amoxicillin, levofloxacin, and cefditoren (Enne et al., 2002; Akcali et al., 2005; Ceyssens and Lavigne, 2010; Hoa et al., 2010; Nie et al., 2015; Foster, 2017; Cillóniz et al., 2018; van Rijn et al., 2019; Hennart et al., 2020).
FIGURE 2. Most common types of bacteria are shown in (A). The resistance of these common bacteria to the most common antibiotics is shown in (B) as a percentage of strain resistance. While in (C,D), an image of bacteriophages of Pseudomonas and different shapes of bacteriophages are provided with the request from COPYRIGHT 2010 Future Medicine Ltd (Ceyssens and Lavigne, 2010) and Springer Link (Rohwer and Barott, 2013).
Pneumococcus resistance occurs especially in asymptomatic children, who can hold the bacterium for a longer time. This bacterium shows resistance against the most common antibiotics. For example, β-lactam antibiotics act against S. aureus by inhibiting the final steps of peptidoglycan synthesis (cell wall) by attaching to high molecular-weight penicillin-binding proteins (Feng et al., 2000). However, the bacterium can alternate its cell walls, making the binding less efficient. Macrolides antibiotics restrict protein synthesis by binding 23S ribosomal target sites in bacteria. The response of the bacterium is a ribosomal alteration by an enzyme that methylates 23S rRNA subunits and is encoded by the ermB (erythromycin resistance methylase) gene (Foster, 2017). Fluoroquinolones are probably the strongest against Staphylococcus by inhibiting DNA synthesis by interacting with intracellular drug targets, DNA gyrase, and topoisomerase. However, even in this case, the bacterium can modify the quinolone resistance-determining region (QRDR) (Hoe et al., 2013). Many other bacteria follow the path of S. aureus (Enne et al., 2002; Akcali et al., 2005; Ceyssens and Lavigne, 2010; Perez et al., 2014; Ezewudo et al., 2018; Li et al., 2018; Kabwe et al., 2019; Rubin et al., 2020). Another example is M. tuberculosis (Mtb), which, despite its low mutation rate, generates drug-resistant tuberculosis (TB) that has significant health and economic costs (Sudre et al., 1992). Mtb can generate pulmonary cavities unseen by immune factors and poorly accessible to antimicrobial drugs, hitherto sheltering large populations of bacteria and providing the perfect circumstances for inducing resistance (Nguyen, 2016). Therefore, highly transmissible multiresistant Mtb strains are beginning to emerge (Nguyen, 2016).
Resistance to antibiotics highlights the importance of seeking alternative cures for bacterial infection, such as the use of bacteriophages.
Bacteriophages, also called ‘phages’, can be described as bacteria eaters. They have a basic structure consisting of a polyhedral head, a short collar, and a helical tail (Yap and Rossmann, 2014). Figures 2D and 2C show an example of a bacteriophage connected to Pseudomonas. There are three basic structural forms of bacteriophage: 1) an icosahedral (20-sided) head with a tail, 2) an icosahedral head without a tail, 3) and a filamentous form (Eiserling, 2023). Even though highly simplistic in structure, bacteriophages evolve to target a specific bacterium without impacting others. Such targeting specificity is ideal for delivery into the respiratory tract, where a large variety of bacteria reside (Stewart and Costerton, 2001). Another classification type mostly focuses on the bacteriophages’ tail components and is based on their biological cycle (Principi et al., 2019). According to this classification, there are two main groups: lytic or virulent, and lysogenic or temperate (Principi et al., 2019). Once either type enters the bacterial cell, the phage produces a viral genome and proteins instead of bacterial materials. Subsequently, the assembly and packing of the bacteriophages start, and the bacterial cells are lysed with the production of new virions that are ready to attack and infect other bacterial cells (Malik et al., 2017; Zhang et al., 2022). However, the process of cell bursting is implicated in the case of lytic bacteriophages. This process can vary according to the bacteriophage’s type, the pathogens against which the bacteriophage is focused, and the environments in which the bacteriophage–pathogen relationship arises (Chan et al., 2013).
Out of the estimated 1,030 phage particles, only a small number have been discovered and utilized to fight common bacterial infections in the lung.
Two types of bacteriophages, Dp-1 and Cpl-1, have been proven to reduce the number of S. pneumoniae bacteria along with other types of Streptococci (Mariano et al., 2016). Phage Dp-1 was discovered earlier (Loeffler et al., 2001; López et al., 2004), but Cpl-1 seems to be more effective (Loeffler et al., 2003). For example, Cpl-1 is also delivered nasally, the nasopharynx being the area most infected by S. pneumoniae (Doehn et al., 2013). Severely ill female mice (C57Bl/6) received 25 mL of aerosolized Cpl-1; after 48 h, the endolysin decreased pulmonary bacterial counts and prevented bacteremia. Although concentrations of inflammatory cytokines were augmented just after Cpl-1 inhalation, the mice improved speedily, as shown by their surging body weight and inflammatory infiltrates fixed in the lungs, leading to a mortality reduction of 80% (Doehn et al., 2013). Moreover, the dosage of 15 mg/kg, of either Cpl-1 or Pal, does not show any increase in pro-inflammatory cytokine levels or significant changes in the fecal microbiome (Harhala et al., 2018). However, another bacteriophage appears to be more effective than Cpl-1. By intravenously injecting about 5 log10 CFU/mL of Lyta and Col-1, the former seems to kill about 80% of β-lactam-resistant (penicillin MIC, 2 μg/mL) meningeal pneumococcal isolate (strain MJD3693) (Rodríguez-Cerrato et al., 2007). Moreover, at 5 h post-challenge, the bacterial titers in the peritoneal fluid and the blood of the control animals were 7.4 ± 0.6 and 6.5 ± 0.7 log10 CFU/mL, respectively. Research on creating or discovering bacteriophages for S. pneumonia is ongoing, and, in recent years, a few new samples have been created. Examples are oral microbiome, 23 TH and SA01 (van der Kamp et al., 2020) and choline-binding proteins (CBPs) (Maestro and Sanz, 2016) and polypeptides found in pneumococcus, and include cell wall hydrolases, adhesins, and other virulence factors, which still need both in vitro and in vivo testing . For example, endolysin MSlys, a type of CBP, is shown within 2-h and 4mM to decrease planktonic cultures by 3.5 log10 CFU/mL, and 24-and 48-h-old biofilms by 1.5 and 1.8 log10 CFU/mL, respectively (Silva et al., 2020).
Another detailed study relates to ClyJ, a novel chimeric lysin. ClyJ is a putative lysin (gp20) that was encoded by the Streptococcus phage SPSL1 utilizing the LytA autolysin as a model. Molecular dissection of gp20 exposed a binding domain (GPB) comprising choline-binding repeats (CBRs) that are explicit for S. pneumoniae. ClyJwas then created by fusing GPB to the CHAP (cysteine, histidine-dependent amidohydrolase/peptidase) catalytic domain of the PlyC lysin. In vivo tests showed that there was no resistance in S. pneumoniae strains after exposure to incrementally doubling concentrations of ClyJ for 8 days (Yang et al., 2019).
Six types of bacteriophages have been discovered for this bacterium, such as HP1, S2A, B, C, N3, and φflu. Of these, only HP1 and three types of S2 have been investigated. HP1 is described as moderate and is thus unable to kill H. influenzae bacteria (Adamczyk-Popławska et al., 2020). Recently, another bacteriophage has been discovered—the N3 phage is only in NTHI strains and has a pattern distinct from HP1. However, poor detailed information is available on the bacteriophages for H. influenzae (Samuels and Clarke, 1969; Williams et al., 2002).
This is one of the strongest bacteria against the effect of several antibiotics. However, the search for a strong bacteriophage is still ongoing and very recent in the literature (Göller et al., 2021). The known bacteriophages of S. aureus are Caudovirales (tailed phages). If the tail is very short, the bacteriophages are called Podoviridae; if the tail is long and non-contractile, Siphoviridae; if the tail is long, contractile, and double-sheathed, Myoviridae (Xia and Wolz, 2014). Despite efforts in discovering and characterizing bacteriophages, most are inactive against S. aureus. A recent study developed JD219 that shows a broad host range able to contaminate 61 of 138 clinical strains of S. aureus tested, including the more virulent MRSA strains. The phage JD419 shows a peculiar morphology, with an elongated capsid and a flexible tail. The activity was maintained at pH values of 6.0–8.0 and below 50°C (Feng et al., 2021). Another bacteriophage pSa-3 shows some activity. Furthermore, its production can be boosted by increasing the bacterial inoculum and dropping the seeding phage MOI; this combination strategy could cut the phage production time (Kim et al., 2021). Alternatively, when inoculated into mice with S. aureus A170 (108 CFU/mouse), phage (109 PFU) rescued 97% of the mice; when applied to nonlethal (5 × 106 CFU/mouse) 10-day infections, the phage (MSa) cleared the bacteria (Capparelli et al., 2007). The indication is that MSa can lyse only 7 of the 19 S. aureus. Moreover, this bacteriophage can act on bacteria formed for over 10 days (Capparelli et al., 2007). A newly discovered bacteriophage seems to kill S. aureus; however, it does not replicate, which is a major drawback for its replacement of antibiotics (Berryhill et al., 2021).
This bacterium is highly worrisome due to its apparently strong resistance to the principal antibiotics. However, there are problems in the manufacturing of its phages (Palomino et al., 2008). Therefore, there is very little research on identifying and developing a bacteriophage that would act as an antibiotic. Even the limited references do not determine a phage but rather verify the resistance of M. tuberculosis to antibiotics (Froman et al., 1954). The few references focus on determining a bacteriophage usable for detecting M. tuberculosis in dairy or skin (Marei et al., 2003; Kalantri et al., 2005; Pai et al., 2005).
The effect of bacteriophages on the virulence of this bacterium was proven several decades ago (Parsons and Frobisher, 1951); examples are βtox+, γtox−, and Ltox+ (Holmes and Barksdale, 1969). However, due to the small number of bacteriophages that were isolated and low interest in bacteriophages, research ceased (Carne, 1968).
Several Ngoϕfil bacteriophages have been discovered to be active on Neisseria bacteria. There are four genetic islands on the surface of these bacteria, encrypting four filamentous phages: Ngoϕ6, Ngoϕ7, Ngoϕ8, and Ngoϕ9 (Kłyż and Piekarowicz, 2018). Only the first two have been shown to be aggressive to Neisseria. Filamentous Phage Ngoϕ6 can infect the cell with a mechanism regardless of the phage receptor and could infect different Neisseria bacteria. This page hosts PivNM/Irg recombinase, which is the entry for Neisseria. However, further studies are needed to test the in vivo and in vitro success of this phage.
An icosahedral head and a segmented tail bacteriophage (Fnpϕ02) specifically infect this bacterium. This phage demonstrates a burst size of 100 phages per infected cell, determined along the 10-h rise period at 37°C (Machuca et al., 2010). FNU1 is another bacteriophage that acts against Fusobacterium. According to Kabwe et al. (2019), the median [Inter-Quartile Range (IQR)] absorbance at OD600 spectrophotometer of the biofilm of Fusobacterium was 2.17 (1.81–2.21), and with 24 h FNU1 treatment was 0.76 (0.71–0.89). Even for this bacterium, much work is still needed, and several investigations are required to determine a suitable bacteriophage.
About 32 prophages have been derived from this bacterium; however, none have demonstrated an aggressive effect on it (de Vries et al., 2009; Ariff et al., 2015).
Due to the aggressiveness of this bacterium, there is so much research that a review article summarizing them has recently been published (Chegini et al., 2020). Here, we report a brief list of all-mentioned bacteriophages acting against P. aeruginosa. One of the earliest discoveries was bacteriophage M-1, isolated from wastewater, that could eliminate biofilm caused by MDR isolates of P. aeruginosa in less than 6 h (Adnan et al., 2020). PB1-like, phiKZ-like, and LUZ24-like phages work against MDR P. aeruginosa under variable growth conditions, even against biofilms of P. aeruginosa (Latz et al., 2017). Pa193, Pa204, Pa222, and Pa223 eliminate the biofilm of P. aeruginosa isolated from patients with chronic rhinosinusitis with a single dose decrease the biofilm reproduction in 24 h (Fong et al., 2017). In addition, phage AZ1 can work against P. aeruginosa in planktonic and biofilm cells (Jamal et al., 2017). LysPA26, another bacteriophage, shows a higher antimicrobial activity at temperatures lower than 100°C (Guo et al., 2017). MAG1 and MAG4 can destroy 50% of the exposed bacteria—MAG4 has shown more lasting effects (Kwiatek et al., 2012). ФKMV, ФPA2, ФPaer4, and ФE2005 phages can also be effective against this bacteria, but in vitro and after 24 h (Mapes et al., 2016). The T7-like lytic phage (BVPaP-3) could constrain the biofilm formation (three logs) of hospital isolates of P. aeruginosa (Ahiwale et al., 2017). Lastly, phage PA1Ø is shown to be active against several Gram-negative and -positive bacteria, including P. aeruginosa (Kim et al., 2012).
Biofilms have often been expected to confer resistance on bacteriophages due to the impermeability of the biofilm matrix. However, even though they are far bigger than chemical antibiotics, bacteriophages are far smaller than their bacterial hosts, and many bacteriophages infect bacteria within biofilms. Therefore, there is potential for using bacteriophages against biofilm, but more investigations are necessary to materialize this potential (Stewart and Costerton, 2001; Harper et al., 2014).
Bacteriophages can be stored in two different forms: liquid suspension or solid/dry powder formulation (Chang et al., 2018a). Either option has advantages and disadvantages and can impact the selection of the route of administration. Some considerations for selecting between them are target-specific delivery, phage stability, and clearance by the reticuloendothelial system of the recipient (Loc-Carrillo and Abedon, 2011). Developing optimal formulations for therapeutic phages is thus important for the efficacy and efficiency of phage therapy (Loc-Carrillo and Abedon, 2011). In fact, protein misfolding, denaturalization, and aggregation can affect bacteriophages, leading to a loss of functionality. These detrimental effects are commonly observed when the bacteriophages are exposed to adverse conditions, such as changes in pH, temperature, or moisture content (Parasion et al., 2014).
The conventional preparation of bacteriophages is as a liquid formulation, as it is for many other bioactive compounds. Liquid formulations are easy to prepare and have an average shelf half-life of about a year, depending on the type of phage. However, this method lacks accuracy; moreover, bacterial contaminants, such as exotoxins, endotoxins, or lipopolysaccharide (LPS) from the lysed cells, could damage the purity of bacteriophage formulations (Luong et al., 2020; João et al., 2021).
Despite the fact that the method for extracting bacteriophage in liquid form has been improved, refining this liquid extraction increases the costs and times (Hashemi et al., 2013; Hietala et al., 2013; Bonilla et al., 2016).
Regardless of the procedure followed to create the liquid formulation, a dramatic reduction in bacteriophage titer can occur at any time, depending on the individual stability of the phage (Malik et al., 2017).
Dry powders, through lyophilization or other similar dehydration techniques, show a longer preservation of stability of bioactivity (Baldelli et al., 2022a). Such procedure is introduced in Figure 3. However, some dehydration processes need some additives, such as sugars, amino acids, proteins, and other components, to prevent osmotic damage or phage aggregation. Spray drying could also be used to prepare bacteriophage powders to be sold on the market. Details of spray drying will be shown in the following sections when discussing spray drying in encapsulation.
FIGURE 3. Procedure for preparing a solution of bacteriophages. Bacteriophage image represents the negative stain transmission electron micrograph of T4 phage processed through the PoT protocol (Bonilla et al., 2016), Copyright © 2016.
Several drying techniques would allow the formation of dry powders. For example, thin film freeze drying (TFFD) (AboulFotouh et al., 2022) and lyophilization (Kawasaki et al., 2019) are some of the most straightforward techniques for generating dry powders for respiratory delivery. These, however, show some significant disadvantages for the purpose of proper encapsulation. First, a chemical procedure would be required to encapsulate bioactive compounds. This procedure can produce chemical waste, and be lengthy and costly (Peng et al., 2019). The main drawback of TFFD is its extremely long processing time, which would not allow it to be transferred into large-scale production (Zhang et al., 2020; Ergin, 2022). A bacteriophage has been successfully encapsulated via lyophilization: Golshahi et al. (2011) encapsulated the phages S4-M and ϕKZ for Burkholderia cepacia and Pseudomonas aeruginosa using a mixture of lactose and lactoferrin at 60: 40 w⁄w. However, powders produced by lyophilization tend to have a broad size distribution, resulting in poor target delivery (Shekunov et al., 2007). About 15%–30% of the particles show an average size of 5–0.5 µm; this results in only 45% of the powder reaching areas beyond the throat in the respiratory tract (Golshahi et al., 2011).
Particle engineering theory can assist spray drying and the prevention of damage (Vehring et al., 2007; Vehring, 2008; Baldelli et al., 2022c; Baldelli et al., 2023). The theory of particle engineering explains the formation process of solid micro- and nanoparticles from liquid droplets (Baldelli and Vehring, 2016b). An extensive review article describes the parameters influencing particle formation; for example, temperature, airflow, atomizer diameter, and percentages of the chemical compounds can all influence dry powders’ chemical and morphological properties (Shoyele and Cawthorne, 2006; Vehring et al., 2007; Baldelli et al., 2016; Ferraz-Albani et al., 2017; Baldelli et al., 2022b). Particle engineering is thus the leading theory of some of the most common drying technologies, such as spray drying (SD) (Vehring et al., 2007) and spray freeze drying (SFD) (Baldelli et al., 2022d), as shown in Figure 4. The main difference between the former and latter is the operating temperature: SFD allows colder temperatures (Baldelli et al., 2022d). These drying techniques have been used for several decades to produce dry powders to deliver bioactive compounds to the respiratory system (Baldelli et al., 2022a). SD is the most common technique used for producing dry powders containing encapsulated bioactive compounds for respiratory delivery. Due to the average size of SD microparticles, the lung is the most common deposition location of SD powders. The smaller the diameter of SD microparticles, the deeper in the respiratory system they can be deposited (Baldelli et al., 2015).
FIGURE 4. Comparison between spray and spray freeze drying for the encapsulation of bacteriophages. The presence of both wall and bulk materials supports this encapsulation. The images have been adjusted from a previous publication (Baldelli et al., 2022a). Copyrights obtained from © 2022 Elsevier Ltd.
Spray drying procedures tend to apply thermal energy to the evaporating solvent for a few milliseconds. This thermal stress is commonly intense, though limited, but can last throughout the spraying procedure; even at lower temperatures than the inlet ones, a process taking a few seconds can jeopardize the stability of bioactive compounds. Another possible source of damage can be shear stress, which occurs when spraying the liquid feed over the nozzle and atomizing it into small droplets (Ma et al., 2015). To bypass these types of stress, and thus the possibility of damaging the bacteriophage during the delivery process, encapsulation can be used using SD or SFD. This can be achieved by adding additional materials to spray the formulation. These materials have been called “wall” and “bulk” (Baldelli et al., 2022d; Ahad et al., 2023; Baldelli et al., 2023; Nimbkar et al., 2023). Wall material is the outer layer of micro- or nanoparticles containing an encapsulated compound. The purpose of this material is obvious: it creates a shell that covers and protects a bioactive compound. This shell is useful for reasons other than the increase of bioavailability of the encapsulated bioactive compound. The wall material is usually a polymer with a higher molecular weight than the bioactive compound to be encapsulated. This characteristic allows the formation of the shell at an early stage in the particle formation process (Baldelli et al., 2015). Forming a shell for each sprayed droplet ensures a high bioavailability of the bioactive compound and, thus, a long half-shell life (Risch, 1995; Baldelli et al., 2022a). The properties of the dry powder are highly dependent on the properties of the shell (Shamaei et al., 2017). For instance, if the polymer is known to show mucoadhesive properties, then the dry powders are expected to have a high residence time on the mucosa in the respiratory tract (Baldelli et al., 2022b). Adhesion forces can also be influenced by the type of material used to form the spray-dried microparticle wall (Fahs et al., 2010). However, the roughness of the microparticles can impact the adhesion forces. This property can be tuned by both the wall material, the spraying conditions, and the presence of a bulk material (Baldelli and Vehring, 2016b). The bulk material is considered a cushion for the bioactive compound for protection greater than that provided by a shell alone. Depending on the quantity, the presence of a bulk material has a great influence on the morphology of spray-dried microparticles (Baldelli et al., 2022a). In some cases, wall and bulk materials are not the only components of a spray-dried formation to encapsulate bioactive compounds—amino acids, such as leucine, are widely used to encapsulate a bioactive compound via spraying techniques (Lechuga-Ballesteros et al., 2008; Ordoubadi et al., 2021; Wang et al., 2021; Baldelli et al., 2022a). The use of amino acids is justified by the promotion of shell formation. Previous research has found that the presence of leucine, at weight percentages between 6 and 22, facilitates shell formation in a variety of spray-dried formulations (Chow et al., 2017). Leucine recrystallizes on the surface of the particles, generating a shell to decrease inter-particle interaction and boost the dispersibility of the powder (Leung et al., 2016). Other amino acids have been used, but there is poor available knowledge on the comparison of these other amino acids with leucine (Carrigy et al., 2020). This review article on designing encapsulated bacteriophage thus focuses on the selection of bulk and wall materials.
While the use of bacteriophages in treating bacterial infections is well-established, the use of spraying techniques as a method for encapsulating bacteriophages for nose or lung delivery is fairly recent. Therefore, the encapsulation of bacteriophages using spraying techniques has been demonstrated in only a limited quantity of research (Table 1). Most of the references cited in Table 1 use a common laboratory-scale spray dryer, Buchi 290, with an atomizer nozzle of 0.6–0.7 mm in diameter. In addition to Buchi 290, other similar products, such as Buchi 191 and 90, were used in previous studies. The reason for this common use is the popularity of this spray dryer and its ability to scale up results (Vehring et al., 2007). In addition, spray drying produces microparticles of about 0.5–20 μm, which is suitable for delivery to the respiratory tract (Baldelli et al., 2015). Furthermore, twin-fluid atomizers have been shown to create lower stress than other types, such as vibrational ones, on the encapsulation of bacteriophages (Carrigy et al., 2020). Another connection between most previous research on the encapsulation of bacteriophages for respiratory delivery is the target: about 90% of previous research focuses on P. aeruginosa. The phages of this bacterium seem to be more stable than others; for instance, they appear stable for temperatures between 25°C and 50°C and pH between 3 and 11 (Akremi et al., 2022). In addition, the antibiotic resistance of P. aeruginosa is one of the highest, attracting the attention of the research community (Golshahi et al., 2011).
TABLE 1. List of research that creates encapsulated dry powder for delivery to the respiratory system of bacteriophages. Type of phage, target, method, and materials used are shown. The number of phages used in the following references varies between 109 and 1010 PFU/m. FR an
Regardless of the target, the number of bacteriophages used needs to be above 1010 pfu/mL since, assuming possible losses during the encapsulation procedure, the required quantity for an effective treatment against most respirator bacteria is 106 pfu/mL (Su et al., 1998; Danelishvili et al., 2006; Chhibber et al., 2008; Chegini et al., 2020). The optimization of the encapsulation procedure could potentially reduce the losses of the bacteriophages. Such optimization can be achieved by tuning the atomization conditions and the materials selected. Having the greatest effect on the stability of sprayed bioactive compounds are the atomization liquid and airflow speed rates and the spraying temperatures (Baldelli et al., 2022e). The gas flow levels of the feed are between 1.8 and 3 mL/min and of the gas are between 11 and 13 L/min. Within these values, bacteriophages appear not to undergo the lowest stress. Lower levels of feed flow rate do not allow the liquid to form droplets and evaporate into dry microparticles (Sheu and Rosenberg, 1995). Higher levels of gas flow rate can reduce the spraying yields and increase the stress applied to bacteriophages (Carrasquillo et al., 2001). An elevated atomizing airflow (12 compared 5 L/min) showed a higher reduction of phage titer (Vandenheuvel et al., 2013).
The lower the temperature, the less damage occurs to a bioactive compound through a spraying procedure (Baldelli et al., 2022d). Bacteriophages have been encapsulated using spray drying at temperatures of the following ranges: inlet at 60°C and outlet at 40°C. For example, one of the lowest titer reductions (0.11) of P. aeruginosa phages has been achieved using these inlet and outlet temperature conditions (Li et al., 2021).
Atomization conditions can have an impact on the stability of bioactive compounds, but the efficiency of the encapsulation procedure is mostly dependent on material selection. When no materials are added to the formulation of bacteriophages, the lowest titer reduction is achieved. Therefore, adding another component to the matrix ensures the survival of most of the bacteriophages. Sugars have been the most common additive used (Shoyele and Cawthorne, 2006). Without these, spray-dried phages were unable to retain their bioactivity during the spray drying process, resulting in log10 6.7 loss or complete titer reduction [118]. In addition, sugars and carbohydrates are able to replace the bioactive compound water hydrogen bonds due to their relatively high molecular weight and structure (Chang et al., 2017).
The most popular sugars are trehalose, mannitol, and lactose, which are also the most commonly used in respiratory delivery (Vehring, 2008). For instance, lactose and trehalose are commonly used to shield biomaterials against desiccation in spray drying. Both saccharides show a high glass transition temperature when in anhydrous amorphous form (108°C and 115°C for lactose and trehalose, respectively). For instance, the majority of PEV1, PEV20, and PEV61 phages spray dried with 12 mg/mL trehalose or lactose resulted in less than log 10 1.5 titer loss (Chang et al., 2017). However, amorphous lactose is hygroscopic; Tg decreases when exposed to succeeding uptake of moisture. The hygroscopic nature of amorphous lactose could generate a lack of phage protection. Amorphous powders are thermodynamically unstable; they can, thus, recrystallize when exposed to moisture (Chang et al., 2017). Thus, trehalose seems more efficient as a stabilizer since it is not influenced by high residual moisture contents (Vandenheuvel et al., 2013). When trehalose is used as an excipient when drying a bioactive compound, the compound's immobilization in a glassy matrix hinders the denaturalization of the bioactive compound. Furthermore, trehalose is highly attracted to water and can act as a lyoprotectant (Ly et al., 2019). For instance, after spray drying, P. aeruginosa’s phage shows a total 1.3 log titer reduction in formulations containing 40%, 60%, and 80% trehalose, and 2.4 and 5.1 log reductions in formulations containing 20% and no trehalose, respectively (Leung et al., 2017).
Although the use of lactose alone seems to be not as functional as trehalose in encapsulating bioactive compounds, the addition of leucine makes the lactose–leucine combination efficient. Leucine is a hydrophobic amino acid that is often used in spray-dried and respirable formulations to enhance powder flowability and dispersibility (Wang et al., 2022). Moreover, leucine can also influence stability against moisture for spray-dried inhalable powders stored at elevated humidities. In the evaporation process, the high hydrophobicity of leucine allows its distribution on the surface, forming a crystalline shell and enhancing the protection of the bacteriophage. For example, spray-dried PEV20 powder containing lactose and L-leucine remains stable for 1 year at 20°C when stored inside an aluminum pouch (Lin et al., 2018). Furthermore, the highest lung dose is obtained with solutions involving 17 mg/mL of lactose and 8 mg/mL of leucine for PEV1 and PEV61, and 20 mg/mL of lactose and 5 mg/mL of leucine for PEV20 (mostly because of the morphology) (Chang et al., 2017). The combination of lactose, trehalose, and leucine can further enhance the protection of the bioactive compound. Formulations containing 40% trehalose, 40% mannitol, and 20% leucine had better storage stability, under storage conditions of −4°C and 30% relative humidity, of P. aeruginosa phage with no further phage loss after 1 month and <1 log storage loss (Yan et al., 2021). The presence of leucine also supports the storage and of phages. Spray-dried powder containing 80% lactose, 20% leucine, and podovirus phage can be stored and handled below 60% RH to prevent crystallization; they stay stable for 1 year at 15% humidity and humidity 20° (Yan et al., 2021).
Other materials used in previous research focusing on encapsulation via spray drying of bacteriophages are mannitol, dextran, and pullulan. Of these, dextran has the highest molecular weight, allowing its distribution across the surface of an evaporating droplet, thus forming a shell. Moreover, dextran is used for spray drying biological materials, preventing P. aeruginosa from connecting to lung epithelial cells and for improving CF sputum clearance (Chang et al., 2021).
Spray drying is the leading technique in encapsulating a bacteriophage for respiratory delivery. The relevant conditions are the inlet and outlet temperatures. For any bacteriophage, these should be below 80°C and 40°C, respectively. When using water as a solvent, it is a challenge to ensure the formation of a shell during the particle formation process. Therefore, as explained in the previous section, leucine, and similar amino acids are valid alternatives since they have been shown to promote shell formation. Together with an amino acid, a common selection is to use a sugar, such as lactose, sucrose, trehalose, or mannitol. Lactose and sucrose have low glass transition temperatures and tend to be more susceptible to crystallization upon room temperature storage (Thiyagarajan et al., 2021). Avoiding crystallization could be beneficial to reducing the risk of damaging the phages. Therefore, as demonstrated by previous research (Ma et al., 2015), trehalose could be a valid option. However, mannitol could be another option when both the procedure and storage temperatures are kept above water’s freezing point. At warm temperatures, mannitol tends not to crystallize (Littringer et al., 2012). The combination of mannitol and trehalose appears to be favorable for phage encapsulation. As a crystalline bulking agent, mannitol can avert the failure of a powder cake, which facilitates drying at higher temperatures and acts as an excipient in inhibiting trehalose from crystallizing (Su et al., 2017). Mannitol is projected to crystallize during the drying stage and form a supporting skeleton for the particles to avoid further mobility of the trehalose particle structure when drying (Chang et al., 2021).
The amount of sugar needed may vary according to the shape and length of the phages. The larger the phage, the more material might be needed to preserve the phage’s bioavailability. For instance, lytic bacteriophages Dp-1 and Cp-1 are roughly 6 µm long. Spray-dried microparticles larger than this would be expected in order to enclose the bacteriophage without major damage. However, a wall material could be used to enhance the protection of the bacteriophages. This could complicate the formulation matrix, but it could ensure shell formation, control the adhesion forces between multiple sprayed microparticles, and improve adhesion to the mucus layer in the respiratory tract (Baldelli and Vehring, 2016b). Moreover, the presence of a hydrophobic polymer on the surface of sprayed microparticles could reduce the impact of humidity on the half-shelf life of powders. In particular, a thicker layer on the surface could increase half-shelf life (Vandenheuvel et al., 2013; Baldelli et al., 2023). Humidity causes the crystallization of any amorphous matrix, ruining the embedded phages. Therefore, reducing its effect on the phages could greatly enhance the half-shelf life of powders. Another process absent in the literature is spray freeze drying. Even though this can be more challenging to design and scale up than spray drying, it can maintain the procedure’s temperatures below 10°C. These low temperatures commonly show a lower chance of jeopardizing the bioavailability of bioactive compounds (Baldelli et al., 2022d).
Resistance to antibiotics affecting the respiratory tract has drastically increased. For instance, Streptococcus pneumoniae shows almost 100% strain resistance to the popular antibiotics penicillin and cefactor. Bacteriophages can bypass such resistance and kill bacteria and biofilms in the respiratory tract. In the case of Staphylococcus aureus, the most common bacteriophage type is Caudovirales. For instance, a type of Caudovirales (phage (109 PFU) has been shown to fully eradicate the infection in mice (5 × 106 CFU/mouse). Although bacteriophages are high efficient at targeting and killing specific types of bacteria, they show low efficacy when delivered to the respiratory system. The encapsulation of bacteriophages can support improved deposition location in the respiratory tract and, thus, the drug’s efficacy. Spray drying is the leading technique for creating encapsulated bioactive compounds. However, due to the high sensitivity of bacteriophages, spray freeze drying might be more appropriate due to the lower stress applied to the bioactive compound. However, the size of the particle formed by spray freeze drying can be applied mostly to nasal delivery. For most types of bacteriophage, leucine or an alternative amino acid is suggested for promoting particle formation. Furthermore, a bulk material is recommended for further protection of the encapsulated compound and for the design of the microparticles. A combination of trehalose and mannitol seems the optimal solution since it avoids any crystallization effect which might jeopardize the stability of the encapsulated bacteriophage. Due to the limited research literature available on the encapsulation of bacteriophages for delivery into the respiratory tract, these conclusions can be considered solely as assumptions.
All authors listed have made a substantial, direct, and intellectual contribution to the work and approved it for publication.
The authors declare that the research was conducted in the absence of any commercial or financial relationships that could be construed as a potential conflict of interest.
All claims expressed in this article are solely those of the authors and do not necessarily represent those of their affiliated organizations, or those of the publisher, the editors, and the reviewers. Any product that may be evaluated in this article, or claim that may be made by its manufacturer, is not guaranteed or endorsed by the publisher.
AboulFotouh, K., Uno, N., Xu, H., Moon, C., Sahakijpijarn, S., Christensen, D. J., et al. (2022). Formulation of dry powders of vaccines containing MF59 or AddaVax by Thin-Film Freeze-Drying: Towards a dry powder universal flu vaccine. Int. J. Pharm. 624, 122021. doi:10.1016/j.ijpharm.2022.122021
Adamczyk-Popławska, M., Tracz-Gaszewska, Z., Lasota, P., Kwiatek, A., and Piekarowicz, A. (2020). Haemophilus influenzae HP1 Bacteriophage encodes a Lytic cassette with a pinholin and a signal-arrest-release endolysin. Int. J. Mol. Sci. 21, 4013. doi:10.3390/ijms21114013
Adnan, M., Shah, M. R. A., Jamal, M., Jalil, F., Andleeb, S., Nawaz, M. A., et al. (2020). Isolation and characterization of bacteriophage to control multidrug-resistant Pseudomonas aeruginosa planktonic cells and biofilm. Biologicals 63, 89–96. doi:10.1016/j.biologicals.2019.10.003
Ahad, T., Gull, A., Masoodi, F. A., Nissar, J., Masoodi, L., and Sajad Wani, M. (2023). Effect of excipient wall materials on the development of ginger oleoresin microcapsules: Assessing the physicochemical, antioxidant and structural properties. J. Sci. Food Agric. 103, 73–82. doi:10.1002/jsfa.12113
Ahiwale, S., Bankar, A., Tagunde, S., and Kapadnis, B. (2017). A bacteriophage mediated gold nanoparticles synthesis and their anti-biofilm activity. Indian J. Microbiol. 57, 188–194. doi:10.1007/s12088-017-0640-x
Akcali, S., Surucuoglu, S., Cicek, C., and Ozbakkaloglu, B. (2005). In vitro activity of ciprofloxacin, ofloxacin and levofloxacin against Mycobacterium tuberculosis. Ann. Saudi Med. 25, 409–412. doi:10.5144/0256-4947.2005.409
Akremi, I., Merabishvili, M., Jlidi, M., Haj Brahim, A., Ben Ali, M., Karoui, A., et al. (2022). Isolation and characterization of lytic Pseudomonas aeruginosa bacteriophages isolated from sewage samples from Tunisia. Viruses 14, 2339. doi:10.3390/v14112339
Ariff, A., Wise, M. J., Kahler, C. M., Tay, C. Y., Peters, F., Perkins, T. T., et al. (2015). Novel Moraxella catarrhalis prophages display hyperconserved non-structural genes despite their genomic diversity. BMC Genomics 16, 860–919. doi:10.1186/s12864-015-2104-1
Baldelli, A., Boraey, M. A., Nobes, D. S., and Vehring, R. (2015). Analysis of the particle formation process of structured microparticles. Mol. Pharm. 12, 2562–2573. doi:10.1021/mp500758s
Baldelli, A., Boraey, M. A., Oguzlu, H., Cidem, A., Rodriguez, A. P., Ong, H. X., et al. (2022). Engineered nasal dry powder for the encapsulation of bioactive compounds. Drug Discov. Today 27 (8), 2300–2308. doi:10.1016/j.drudis.2022.04.012
Baldelli, A., Cidem, A., Guo, Y., Ong, H. X., Singh, A., Traini, D., et al. (2022). Spray freeze drying for protein encapsulation: Impact of the formulation to morphology and stability. Dry. Technol. 41, 137–150. doi:10.1080/07373937.2022.2089162
Baldelli, A., Etayash, H., Oguzlu, H., Mandal, R., Jiang, F., Hancock, R. E., et al. (2022). Antimicrobial properties of spray-dried cellulose nanocrystals and metal oxide-based nanoparticles-in-microspheres. Chem. Eng. J. Adv. 10, 100273. doi:10.1016/j.ceja.2022.100273
Baldelli, A., Liang, D. Y., Guo, Y., and Pratap-Singh, A. (2022). Effect of the formulation on mucoadhesive spray-dried microparticles containing iron for food fortification. Food Hydrocoll. 134, 107906. doi:10.1016/j.foodhyd.2022.107906
Baldelli, A., Oguzlu, H., Liang, D. Y., Subiantoro, A., Woo, M. W., and Pratap-Singh, A. (2022). Spray freeze drying of dairy products: Effect of formulation on dispersibility. J. Food Eng. 335, 111191. doi:10.1016/j.jfoodeng.2022.111191
Baldelli, A., Power, R. M., Miles, R. E., Reid, J. P., and Vehring, R. (2016). Effect of crystallization kinetics on the properties of spray dried microparticles. Aerosol Sci. Technol. 50, 693–704. doi:10.1080/02786826.2016.1177163
Baldelli, A., Ren, M., Liang, D. Y., Lai, S., Hartono, B., Sum, K., et al. (2023). Sprayed microcapsules of minerals for fortified food. J. Funct. Foods 101, 105401. doi:10.1016/j.jff.2023.105401
Baldelli, A., and Vehring, R. (2016). Analysis of cohesion forces between monodisperse microparticles with rough surfaces. Colloids Surfaces A Physicochem. Eng. Aspects 506, 179–189. doi:10.1016/j.colsurfa.2016.06.009
Baldelli, A., and Vehring, R. (2016). Control of the radial distribution of chemical components in spray-dried crystalline microparticles. Aerosol Sci. Technol. 50, 1130–1142. doi:10.1080/02786826.2016.1216941
Berryhill, B. A., Huseby, D. L., McCall, I. C., Hughes, D., and Levin, B. R. (2021). Evaluating the potential efficacy and limitations of a phage for joint antibiotic and phage therapy of Staphylococcus aureus infections. Proc. Natl. Acad. Sci. 118, e2008007118. doi:10.1073/pnas.2008007118
Bonilla, N., Rojas, M. I., Cruz, G. N. F., Hung, S.-H., Rohwer, F., and Barr, J. J. (2016). Phage on tap–a quick and efficient protocol for the preparation of bacteriophage laboratory stocks. PeerJ 4, e2261. doi:10.7717/peerj.2261
Bosch, A. A., Biesbroek, G., Trzcinski, K., Sanders, E. A., and Bogaert, D. (2013). Viral and bacterial interactions in the upper respiratory tract. PLoS Pathog. 9, e1003057. doi:10.1371/journal.ppat.1003057
Capparelli, R., Parlato, M., Borriello, G., Salvatore, P., and Iannelli, D. (2007). Experimental phage therapy against Staphylococcus aureus in mice. Antimicrob. Agents Chemother. 51, 2765–2773. doi:10.1128/AAC.01513-06
Carne, H. (1968). Action of bacteriophages obtained from Corynebacterium diphtheriae on C. ulcerans and C. ovis. Nature 217, 1066–1067. doi:10.1038/2171066b0
Carrasquillo, K. G., Stanley, A. M., Aponte-Carro, J. C., De Jésus, P., Costantino, H. R., Bosques, C. J., et al. (2001). Non-aqueous encapsulation of excipient-stabilized spray-freeze dried BSA into poly (lactide-co-glycolide) microspheres results in release of native protein. J. Control. Release 76, 199–208. doi:10.1016/s0168-3659(01)00430-8
Carrigy, N. B., Liang, L., Wang, H., Kariuki, S., Nagel, T. E., Connerton, I. F., et al. (2020). Trileucine and pullulan improve anti-campylobacter bacteriophage stability in engineered spray-dried microparticles. Ann. Biomed. Eng. 48, 1169–1180. doi:10.1007/s10439-019-02435-6
Ceyssens, P.-J., and Lavigne, R. (2010). Bacteriophages of Pseudomonas. Future Microbiol. 5, 1041–1055. doi:10.2217/fmb.10.66
Chan, B. K., Abedon, S. T., and Loc-Carrillo, C. (2013). Phage cocktails and the future of phage therapy. Future Microbiol. 8, 769–783. doi:10.2217/fmb.13.47
Chang, R. Y. K., Chen, K., Wang, J., Wallin, M., Britton, W., Morales, S., et al. (2018). Proof-of-principle study in a murine lung infection model of antipseudomonal activity of phage PEV20 in a dry-powder formulation. Antimicrob. Agents Chemother. 62, e01714–e01717. doi:10.1128/AAC.01714-17
Chang, R. Y. K., Chow, M. Y., Khanal, D., Chen, D., and Chan, H.-K. (2021). Dry powder pharmaceutical biologics for inhalation therapy. Adv. Drug Deliv. Rev. 172, 64–79. doi:10.1016/j.addr.2021.02.017
Chang, R. Y. K., Wallin, M., Lin, Y., Leung, S. S. Y., Wang, H., Morales, S., et al. (2018). Phage therapy for respiratory infections. Adv. Drug Del. Rev. 133, 76–86. doi:10.1016/j.addr.2018.08.001
Chang, R. Y., Wong, J., Mathai, A., Morales, S., Kutter, E., Britton, W., et al. (2017). Production of highly stable spray dried phage formulations for treatment of Pseudomonas aeruginosa lung infection. Eur. J. Pharm. Biopharm. 121, 1–13. doi:10.1016/j.ejpb.2017.09.002
Chegini, Z., Khoshbayan, A., Taati Moghadam, M., Farahani, I., Jazireian, P., and Shariati, A. (2020). Bacteriophage therapy against Pseudomonas aeruginosa biofilms: A review. Ann. Clin. Microbiol. Antimicrob. 19, 45–17. doi:10.1186/s12941-020-00389-5
Chhibber, S., Kaur, S., and Kumari, S. (2008). Therapeutic potential of bacteriophage in treating Klebsiella pneumoniae B5055-mediated lobar pneumonia in mice. J. Med. Microbiol. 57, 1508–1513. doi:10.1099/jmm.0.2008/002873-0
Choińska-Pulit, A., Mituła, P., Śliwka, P., Łaba, W., and Skaradzińska, A. (2015). Bacteriophage encapsulation: Trends and potential applications. Trends Food Sci. Technol. 45, 212–221. doi:10.1016/j.tifs.2015.07.001
Chow, M. Y., Qiu, Y., Lo, F. F., Lin, H. H., Chan, H.-K., Kwok, P. C., et al. (2017). Inhaled powder formulation of naked siRNA using spray drying technology with l-leucine as dispersion enhancer. Int. J. Pharm. 530, 40–52. doi:10.1016/j.ijpharm.2017.07.013
Cillóniz, C., Garcia-Vidal, C., Ceccato, A., and Torres, A. (2018). Antimicrobial resistance among Streptococcus pneumoniae. SpringerNature 2018, 13–38. doi:10.1007/978-3-319-78538-7_2
Dąbrowska, K. (2019). Phage therapy: What factors shape phage pharmacokinetics and bioavailability? Systematic and critical review. Med. Res. Rev. 39, 2000–2025. doi:10.1002/med.21572
Danelishvili, L., Young, L. S., and Bermudez, L. E. (2006). In vivo efficacy of phage therapy for Mycobacterium avium infection as delivered by a nonvirulent mycobacterium. Microb. Drug Resist. 12, 1–6. doi:10.1089/mdr.2006.12.1
Dasaraju, P. V., and Liu, C. (1996). Infections of the respiratory system, medical microbiology. 4th edition. The University of Texas.
de Vries, S. P., Bootsma, H. J., Hays, J. P., and Hermans, P. W. (2009). Molecular aspects of Moraxella catarrhalis pathogenesis. Microbiol. Mol. Biol. Rev. 73, 389–406. doi:10.1128/MMBR.00007-09
Doehn, J. M., Fischer, K., Reppe, K., Gutbier, B., Tschernig, T., Hocke, A. C., et al. (2013). Delivery of the endolysin Cpl-1 by inhalation rescues mice with fatal pneumococcal pneumonia. J. Antimicrob. Chemother. 68, 2111–2117. doi:10.1093/jac/dkt131
Enne, V. I., King, A., Livermore, D. M., and Hall, L. M. (2002). Sulfonamide resistance in Haemophilus influenzae mediated by acquisition of sul2 or a short insertion in chromosomal folP. Antimicrob. Agents Chemother. 46, 1934–1939. doi:10.1128/aac.46.6.1934-1939.2002
Ergin, F. (2022). Effect of freeze drying, spray drying and electrospraying on the morphological, thermal, and structural properties of powders containing phage Felix O1 and activity of phage Felix O1 during storage. Powder Technol. 404, 117516. doi:10.1016/j.powtec.2022.117516
Ezewudo, M., Borens, A., Chiner-Oms, Á., Miotto, P., Chindelevitch, L., Starks, A. M., et al. (2018). Integrating standardized whole genome sequence analysis with a global Mycobacterium tuberculosis antibiotic resistance knowledgebase. Sci. Rep. 8, 15382–15410. doi:10.1038/s41598-018-33731-1
Fahs, A., Brogly, M., Bistac, S., and Schmitt, M. (2010). Hydroxypropyl methylcellulose (HPMC) formulated films: Relevance to adhesion and friction surface properties. Carbohydr. Polym. 80, 105–114. doi:10.1016/j.carbpol.2009.10.071
Feng, Q. L., Wu, J., Chen, G. Q., Cui, F., Kim, T., and Kim, J. (2000). A mechanistic study of the antibacterial effect of silver ions on Escherichia coli and Staphylococcus aureus. J. Biomed. Mater. Res. 52, 662–668. doi:10.1002/1097-4636(20001215)52:4<662::aid-jbm10>3.0.co;2-3
Feng, T., Leptihn, S., Dong, K., Loh, B., Zhang, Y., Stefan, M. I., et al. (2021). JD419, a Staphylococcus aureus phage with a unique morphology and broad host range. Front. Microbiol. 12, 602902. doi:10.3389/fmicb.2021.602902
Ferraz-Albani, L. A., Baldelli, A., Knapp, C. J., Jäger, W., Vehring, R., Nobes, D. S., et al. (2017). Enhanced evaporation of microscale droplets with an infrared laser. J. Heat Transf. 139. doi:10.1115/1.4034486
Fong, S. A., Drilling, A., Morales, S., Cornet, M. E., Woodworth, B. A., Fokkens, W. J., et al. (2017). Activity of bacteriophages in removing biofilms of Pseudomonas aeruginosa isolates from chronic rhinosinusitis patients. Front. Cell. Infect. Microbiol. 7, 418. doi:10.3389/fcimb.2017.00418
Foster, T. J. (2017). Antibiotic resistance in Staphylococcus aureus. Current status and future prospects. FEMS Microbiol. Rev. 41, 430–449. doi:10.1093/femsre/fux007
Froman, S., Will, D. W., and Bogen, E. (1954). Bacteriophage active against virulent Mycobacterium tuberculosis—I. Isolation and activity. Am. J. Public Health Nations Health 44, 1326–1333. doi:10.2105/ajph.44.10.1326
Göller, P. C., Elsener, T., Lorgé, D., Radulovic, N., Bernardi, V., Naumann, A., et al. (2021). Multi-species host range of staphylococcal phages isolated from wastewater. Nat. Commun. 12, 6965–7017. doi:10.1038/s41467-021-27037-6
Golshahi, L., Lynch, K., Dennis, J., and Finlay, W. (2011). In vitro lung delivery of bacteriophages KS4-M and ΦKZ using dry powder inhalers for treatment of Burkholderia cepacia complex and Pseudomonas aeruginosa infections in cystic fibrosis. J. Appl. Microbiol. 110, 106–117. doi:10.1111/j.1365-2672.2010.04863.x
Gomez, M., Archer, M., Barona, D., Wang, H., Ordoubadi, M., Karim, S. B., et al. (2021). Microparticle encapsulation of a tuberculosis subunit vaccine candidate containing a nanoemulsion adjuvant via spray drying. Eur. J. Pharm. Biopharm. 163, 23–37. doi:10.1016/j.ejpb.2021.03.007
Guo, M., Feng, C., Ren, J., Zhuang, X., Zhang, Y., Zhu, Y., et al. (2017). A novel antimicrobial endolysin, LysPA26, against Pseudomonas aeruginosa. Front. Microbiol. 8, 293. doi:10.3389/fmicb.2017.00293
Harhala, M., Nelson, D. C., Miernikiewicz, P., Heselpoth, R. D., Brzezicka, B., Majewska, J., et al. (2018). Safety studies of pneumococcal endolysins Cpl-1 and Pal. Viruses 10, 638. doi:10.3390/v10110638
Harper, D. R., Parracho, H. M., Walker, J., Sharp, R., Hughes, G., Werthén, M., et al. (2014). Bacteriophages and biofilms. Antibiotics 3, 270–284. doi:10.3390/antibiotics3030270
Hashemi, H., Pouyanfard, S., Bandehpour, M., Mahmoudi, M., Bernasconi, M., Kazemi, B., et al. (2013). Efficient endotoxin removal from T7 phage preparations by a mild detergent treatment followed by ultrafiltration. Acta Virol. 57, 373–374.
Hennart, M., Panunzi, L. G., Rodrigues, C., Gaday, Q., Baines, S. L., Barros-Pinkelnig, M., et al. (2020). Population genomics and antimicrobial resistance in Corynebacterium diphtheriae. Genome Med. 12, 107–118. doi:10.1186/s13073-020-00805-7
Hietala, M., Mathew, A. P., and Oksman, K. (2013). Bionanocomposites of thermoplastic starch and cellulose nanofibers manufactured using twin-screw extrusion. Eur. Polym. J. 49, 950–956. doi:10.1016/j.eurpolymj.2012.10.016
Hoa, N. Q., Trung, N. V., Larsson, M., Eriksson, B., Phuc, H. D., Chuc, N. T., et al. (2010). Decreased Streptococcus pneumoniae susceptibility to oral antibiotics among children in rural vietnam: A community study. BMC Infect. Dis. 10, 85–11. doi:10.1186/1471-2334-10-85
Hoe, S., Semler, D. D., Goudie, A. D., Lynch, K. H., Matinkhoo, S., Finlay, W. H., et al. (2013). Respirable bacteriophages for the treatment of bacterial lung infections. J. Aerosol Med. Pulm. Drug Deliv. 26, 317–335. doi:10.1089/jamp.2012.1001
Holmes, R. K., and Barksdale, L. (1969). Genetic analysis of tox+ and tox− bacteriophages of Corynebacterium diphtheriae. J. Virology 3, 586–598. doi:10.1128/JVI.3.6.586-598.1969
Huang, J., Garmise, R. J., Crowder, T. M., Mar, K., Hwang, C. R., Hickey, A. J., et al. (2004). A novel dry powder influenza vaccine and intranasal delivery technology: Induction of systemic and mucosal immune responses in rats. Vaccine 23, 794–801. doi:10.1016/j.vaccine.2004.06.049
Jamal, M., Andleeb, S., Jalil, F., Imran, M., Nawaz, M. A., Hussain, T., et al. (2017). Isolation and characterization of a bacteriophage and its utilization against multi-drug resistant Pseudomonas aeruginosa-2995. Life Sci. 190, 21–28. doi:10.1016/j.lfs.2017.09.034
João, J., Lampreia, J., Prazeres, D. M. F., and Azevedo, A. M. (2021). Manufacturing of bacteriophages for therapeutic applications. Biotechnol. Adv. 49, 107758. doi:10.1016/j.biotechadv.2021.107758
Kabwe, M., Brown, T. L., Dashper, S., Speirs, L., Ku, H., Petrovski, S., et al. (2019). Genomic, morphological and functional characterisation of novel bacteriophage FNU1 capable of disrupting Fusobacterium nucleatum biofilms. Sci. Rep. 9, 9107–9112. doi:10.1038/s41598-019-45549-6
Kalantri, S., Pai, M., Pascopella, L., Riley, L., and Reingold, A. (2005). Bacteriophage-based tests for the detection of Mycobacterium tuberculosis in clinical specimens: A systematic review and meta-analysis. BMC Infect. Dis. 5, 59–13. doi:10.1186/1471-2334-5-59
Kawasaki, H., Shimanouchi, T., and Kimura, Y. (2019). Recent development of optimization of lyophilization process. J. Chem. 2019, 1–14. doi:10.1155/2019/9502856
Kim, S. G., Kwon, J., Giri, S. S., Yun, S., Kim, H. J., Kim, S. W., et al. (2021). Strategy for mass production of lytic Staphylococcus aureus bacteriophage pSa-3: Contribution of multiplicity of infection and response surface methodology. Microb. Cell Factories 20, 56–12. doi:10.1186/s12934-021-01549-8
Kim, S., Rahman, M., Seol, S. Y., Yoon, S. S., and Kim, J. (2012). Pseudomonas aeruginosa bacteriophage PA1Ø requires type IV pili for infection and shows broad bactericidal and biofilm removal activities. Appl. Environ. Microbiol. 78, 6380–6385. doi:10.1128/AEM.00648-12
Kłyż, A., and Piekarowicz, A. (2018). Phage proteins are expressed on the surface of Neisseria gonorrhoeae and are potential vaccine candidates. PLoS One 13, e0202437. doi:10.1371/journal.pone.0202437
Kwiatek, M., Parasion, S., Mizak, L., Gryko, R., Bartoszcze, M., and Kocik, J. (2012). Characterization of a bacteriophage, isolated from a cow with mastitis, that is lytic against Staphylococcus aureus strains. Archives virology 157, 225–234. doi:10.1007/s00705-011-1160-3
Latz, S., Krüttgen, A., Häfner, H., Buhl, E. M., Ritter, K., and Horz, H.-P. (2017). Differential effect of newly isolated phages belonging to PB1-like, phiKZ-like and LUZ24-like viruses against multi-drug resistant Pseudomonas aeruginosa under varying growth conditions. Viruses 9, 315. doi:10.3390/v9110315
Lechuga-Ballesteros, D., Charan, C., Stults, C. L., Stevenson, C. L., Miller, D. P., Vehring, R., et al. (2008). Trileucine improves aerosol performance and stability of spray-dried powders for inhalation. J. Pharm. Sci. 97, 287–302. doi:10.1002/jps.21078
Leung, S. S., Parumasivam, T., Gao, F. G., Carrigy, N. B., Vehring, R., Finlay, W. H., et al. (2016). Production of inhalation phage powders using spray freeze drying and spray drying techniques for treatment of respiratory infections. Pharm. Res. 33, 1486–1496. doi:10.1007/s11095-016-1892-6
Leung, S. S., Parumasivam, T., Gao, F. G., Carter, E. A., Carrigy, N. B., Vehring, R., et al. (2017). Effects of storage conditions on the stability of spray dried, inhalable bacteriophage powders. Int. J. Pharm. 521, 141–149. doi:10.1016/j.ijpharm.2017.01.060
Li, H., Yang, H., Zhou, Z., Li, X., Yi, W., Xu, Y., et al. (2018). Isolation, antibiotic resistance, virulence traits and phylogenetic analysis of Corynebacterium pseudotuberculosis from goats in southwestern China. Small Ruminant Res. 168, 69–75. doi:10.1016/j.smallrumres.2018.09.015
Li, M., Chang, R. Y. K., Lin, Y., Morales, S., Kutter, E., and Chan, H.-K. (2021). Phage cocktail powder for Pseudomonas aeruginosa respiratory infections. Int. J. Pharm. 596, 120200. doi:10.1016/j.ijpharm.2021.120200
Lin, B., Gu, H., Ni, H., Guan, B., Li, Z., Han, D., et al. (2018). Effect of mixing methane, ethane, propane and ethylene on the soot particle size distribution in a premixed propene flame. Combust. Flame 193, 54–60. doi:10.1016/j.combustflame.2018.03.002
Lin, Y., Chang, R. Y. K., Britton, W. J., Morales, S., Kutter, E., Li, J., et al. (2019). Inhalable combination powder formulations of phage and ciprofloxacin for P. aeruginosa respiratory infections. Eur. J. Pharm. Biopharm. 142, 543–552. doi:10.1016/j.ejpb.2019.08.004
Littringer, E. M., Mescher, A., Eckhard, S., Schröttner, H., Langes, C., Fries, M., et al. (2012). Spray drying of mannitol as a drug carrier—The impact of process parameters on product properties. Dry. Technol. 30, 114–124. doi:10.1080/07373937.2011.620726
Loc-Carrillo, C., and Abedon, S. T. (2011). Pros and cons of phage therapy. Bacteriophage 1, 111–114. doi:10.4161/bact.1.2.14590
Loeffler, J. M., Djurkovic, S., and Fischetti, V. A. (2003). Phage lytic enzyme Cpl-1 as a novel antimicrobial for pneumococcal bacteremia. Infect. Immun. 71, 6199–6204. doi:10.1128/iai.71.11.6199-6204.2003
Loeffler, J. M., Nelson, D., and Fischetti, V. A. (2001). Rapid killing of Streptococcus pneumoniae with a bacteriophage cell wall hydrolase. Science 294, 2170–2172. doi:10.1126/science.1066869
López, R., García, E., and García, P. (2004). Enzymes for anti-infective therapy: Phage lysins. Drug Discov. Today Ther. Strateg. 1, 469–474. doi:10.1016/j.ddstr.2004.09.002
Luong, T., Salabarria, A.-C., Edwards, R. A., and Roach, D. R. (2020). Standardized bacteriophage purification for personalized phage therapy. Nat. Protoc. 15, 2867–2890. doi:10.1038/s41596-020-0346-0
Ly, A., Carrigy, N. B., Wang, H., Harrison, M., Sauvageau, D., Martin, A. R., et al. (2019). Atmospheric spray freeze drying of sugar solution with phage D29. Front. Microbiol. 10, 488. doi:10.3389/fmicb.2019.00488
Ma, J., Zhang, X., Bao, Y., and Liu, J. (2015). A facile spraying method for fabricating superhydrophobic leather coating. Colloids Surfaces A Physicochem. Eng. Aspects 472, 21–25. doi:10.1016/j.colsurfa.2015.02.019
Maa, Y.-F., Nguyen, P.-A., Sweeney, T., Shire, S. J., and Hsu, C. C. (1999). Protein inhalation powders: Spray drying vs spray freeze drying. Pharm. Res. 16, 249–254. doi:10.1023/a:1018828425184
Machuca, P., Daille, L., Vinés, E., Berrocal, L., and Bittner, M. (2010). Isolation of a novel bacteriophage specific for the periodontal pathogen Fusobacterium nucleatum. Appl. Environ. Microbiol. 76, 7243–7250. doi:10.1128/AEM.01135-10
Maestro, B., and Sanz, J. M. (2016). Choline binding proteins from Streptococcus pneumoniae: A dual role as enzybiotics and targets for the design of new antimicrobials. Antibiotics 5, 21. doi:10.3390/antibiotics5020021
Malik, D. J., Sokolov, I. J., Vinner, G. K., Mancuso, F., Cinquerrui, S., Vladisavljevic, G. T., et al. (2017). Formulation, stabilisation and encapsulation of bacteriophage for phage therapy. Adv. Colloid Interface Sci. 249, 100–133. doi:10.1016/j.cis.2017.05.014
Mapes, A. C., Trautner, B. W., Liao, K. S., and Ramig, R. F. (2016). Development of expanded host range phage active on biofilms of multi-drug resistant Pseudomonas aeruginosa. Bacteriophage 6, e1096995. doi:10.1080/21597081.2015.1096995
Marei, A. M., El-Behedy, E. M., Mohtady, H. A., and Afify, A. F. (2003). Evaluation of a rapid bacteriophage-based method for the detection of Mycobacterium tuberculosis in clinical samples. J. Med. Microbiol. 52, 331–335. doi:10.1099/jmm.0.05091-0
Mariano, R., Wuchty, S., Vizoso-Pinto, M. G., Häuser, R., and Uetz, P. (2016). The interactome of Streptococcus pneumoniae and its bacteriophages show highly specific patterns of interactions among bacteria and their phages. Sci. Rep. 6, 24597–24610. doi:10.1038/srep24597
Matinkhoo, S., Lynch, K. H., Dennis, J. J., Finlay, W. H., and Vehring, R. (2011). Spray-dried respirable powders containing bacteriophages for the treatment of pulmonary infections. J. Pharm. Sci. 100, 5197–5205. doi:10.1002/jps.22715
Nguyen, L. (2016). Antibiotic resistance mechanisms in M. tuberculosis: An update. Archives Toxicol. 90, 1585–1604. doi:10.1007/s00204-016-1727-6
Nie, W., Duan, H., Huang, H., Lu, Y., and Chu, N. (2015). Species identification and clarithromycin susceptibility testing of 278 clinical nontuberculosis mycobacteria isolates. BioMed Res. Int. 2015, 506598. doi:10.1155/2015/506598
Nimbkar, S., Leena, M. M., Moses, J., and Anandharamakrishnan, C. (2023). Development of iron-vitamin multilayer encapsulates using 3 fluid nozzle spray drying. Food Chem. 406, 135035. doi:10.1016/j.foodchem.2022.135035
Ordoubadi, M., Gregson, F. K., Wang, H., Nicholas, M., Gracin, S., Lechuga-Ballesteros, D., et al. (2021). On the particle formation of leucine in spray drying of inhalable microparticles. Int. J. Pharm. 592, 120102. doi:10.1016/j.ijpharm.2020.120102
Pai, M., Kalantri, S., Pascopella, L., Riley, L. W., and Reingold, A. L. (2005). Bacteriophage-based assays for the rapid detection of rifampicin resistance in Mycobacterium tuberculosis: A meta-analysis. J. Infect. 51, 175–187. doi:10.1016/j.jinf.2005.05.017
Palomino, J. C., Martin, A., Von Groll, A., and Portaels, F. (2008). Rapid culture-based methods for drug-resistance detection in Mycobacterium tuberculosis. J. Microbiol. Methods 75, 161–166. doi:10.1016/j.mimet.2008.06.015
Parasion, S., Kwiatek, M., Gryko, R., Mizak, L., and Malm, A. (2014). Bacteriophages as an alternative strategy for fighting biofilm development. Pol. J. Microbiol. 63, 137–145. doi:10.33073/pjm-2014-019
Parsons, E., and Frobisher, M. (1951). Effect of bacteriophage on virulence of Corynebacterium diphtheriae. Proc. Soc. Exp. Biol. Med. 78, 746–747. doi:10.3181/00379727-78-19204
Peabody, J., Muttil, P., Chackerian, B., and Tumban, E. (2017). Characterization of a spray-dried candidate HPV L2-VLP vaccine stored for multiple years at room temperature. Papillomavirus Res. 3, 116–120. doi:10.1016/j.pvr.2017.03.004
Peng, S., Zou, L., Zhou, W., Liu, W., Liu, C., and McClements, D. J. (2019). Encapsulation of lipophilic polyphenols into nanoliposomes using pH-driven method: Advantages and disadvantages. J. Agric. food Chem. 67, 7506–7511. doi:10.1021/acs.jafc.9b01602
Perez, A. C., Pang, B., King, L. B., Tan, L., Murrah, K. A., Reimche, J. L., et al. (2014). Residence of Streptococcus pneumoniae and Moraxella catarrhalis within polymicrobial biofilm promotes antibiotic resistance and bacterial persistence in vivo. Pathogens Dis. 70, 280–288. doi:10.1111/2049-632X.12129
Prat, C., and Lacoma, A. (2016). Bacteria in the respiratory tract—how to treat? Or do not treat? Int. J. Infect. Dis. 51, 113–122. doi:10.1016/j.ijid.2016.09.005
Principi, N., Silvestri, E., and Esposito, S. (2019). Advantages and limitations of bacteriophages for the treatment of bacterial infections. Front. Pharmacol. 10, 513. doi:10.3389/fphar.2019.00513
Rodríguez-Cerrato, V., García, P., Huelves, L., García, E., Del Prado, G., Gracia, M., et al. (2007). Pneumococcal LytA autolysin, a potent therapeutic agent in experimental peritonitis-sepsis caused by highly β-lactam-resistant Streptococcus pneumoniae. Antimicrob. Agents Chemother. 51, 3371–3373. doi:10.1128/AAC.00137-07
Rohwer, F., and Barott, K. (2013). Viral information. Biol. Philosophy 28, 283–297. doi:10.1007/s10539-012-9344-0
Rubin, D. H., Ross, J. D., and Grad, Y. H. (2020). The frontiers of addressing antibiotic resistance in Neisseria gonorrhoeae. Transl. Res. 220, 122–137. doi:10.1016/j.trsl.2020.02.002
Saboo, S., Tumban, E., Peabody, J., Wafula, D., Peabody, D. S., Chackerian, B., et al. (2016). Optimized formulation of a thermostable spray-dried virus-like particle vaccine against human papillomavirus. Mol. Pharm. 13, 1646–1655. doi:10.1021/acs.molpharmaceut.6b00072
Samuels, J., and Clarke, J. (1969). New bacteriophage of Haemophilus influenzae. J. Virology 4, 797–798. doi:10.1128/JVI.4.5.797-798.1969
Shamaei, S., Seiiedlou, S. S., Aghbashlo, M., Tsotsas, E., and Kharaghani, A. (2017). Microencapsulation of walnut oil by spray drying: Effects of wall material and drying conditions on physicochemical properties of microcapsules. Innovative food Sci. Emerg. Technol. 39, 101–112. doi:10.1016/j.ifset.2016.11.011
Shekunov, B. Y., Chattopadhyay, P., Tong, H. H., and Chow, A. H. (2007). Particle size analysis in pharmaceutics: Principles, methods and applications. Pharm. Res. 24, 203–227. doi:10.1007/s11095-006-9146-7
Sheu, T. Y., and Rosenberg, M. (1995). Microencapsulation by spray drying ethyl caprylate in whey protein and carbohydrate wall systems. J. food Sci. 60, 98–103. doi:10.1111/j.1365-2621.1995.tb05615.x
Shoyele, S. A., and Cawthorne, S. (2006). Particle engineering techniques for inhaled biopharmaceuticals. Adv. drug Deliv. Rev. 58, 1009–1029. doi:10.1016/j.addr.2006.07.010
Silva, M. D., Oliveira, H., Faustino, A., and Sillankorva, S. (2020). Characterization of MSlys, the endolysin of Streptococcus pneumoniae phage MS1. Biotechnol. Rep. 28, e00547. doi:10.1016/j.btre.2020.e00547
Stewart, P. S., and Costerton, J. W. (2001). Antibiotic resistance of bacteria in biofilms. Lancet 358, 135–138. doi:10.1016/s0140-6736(01)05321-1
Su, M.-T., Venkatesh, T. V., and Bodmer, R. (1998). Large-and small-scale preparation of bacteriophage λ lysate and DNA. BioTechniques 25, 44–46. doi:10.2144/98251bm08
Su, W., Jia, N., Li, H., Hao, H., and Li, C. (2017). Polymorphism of D-mannitol: Crystal structure and the crystal growth mechanism. Chin. J. Chem. Eng. 25, 358–362. doi:10.1016/j.cjche.2016.09.002
Sudre, P., Ten Dam, G., and Kochi, A. (1992). Tuberculosis: A global overview of the situation today. Bull. World Health Organ. 70, 149–159.
Sulakvelidze, A., Alavidze, Z., and Morris, J. G. (2001). Bacteriophage therapy. Bacteriophage Ther. Antimicrob. agents Chemother. 45, 649–659. doi:10.1128/AAC.45.3.649-659.2001
Tabare, E., Glonti, T., Cochez, C., Ngassam, C., Pirnay, J.-P., Amighi, K., et al. (2021). A design of experiment approach to optimize spray-dried powders containing Pseudomonas aeruginosa Podoviridae and Myoviridae bacteriophages. Viruses 13, 1926. doi:10.3390/v13101926
Thiyagarajan, D., Huck, B., Nothdurft, B., Koch, M., Rudolph, D., Rutschmann, M., et al. (2021). Spray-dried lactose-leucine microparticles for pulmonary delivery of antimycobacterial nanopharmaceuticals. Drug Deliv. Transl. Res. 11, 1766–1778. doi:10.1007/s13346-021-01011-7
van der Kamp, I., Draper, L. A., Smith, M. K., Buttimer, C., Ross, R. P., and Hill, C. (2020). A new phage lysin isolated from the oral microbiome targeting streptococcus pneumoniae. Pharmaceuticals 13, 478. doi:10.3390/ph13120478
van Rijn, S. P., Zuur, M. A., Anthony, R., Wilffert, B., van Altena, R., Akkerman, O. W., et al. (2019). Evaluation of carbapenems for treatment of multi-and extensively drug-resistant. Mycobacterium Tuberc. Antimicrob. Agents Chemother. 63, e01489–e01418. doi:10.1128/AAC.01489-18
Vandenheuvel, D., Singh, A., Vandersteegen, K., Klumpp, J., Lavigne, R., and Van den Mooter, G. (2013). Feasibility of spray drying bacteriophages into respirable powders to combat pulmonary bacterial infections. Eur. J. Pharm. Biopharm. 84, 578–582. doi:10.1016/j.ejpb.2012.12.022
Vehring, R., Foss, W. R., and Lechuga-Ballesteros, D. (2007). Particle formation in spray drying. J. Aerosol Sci. 38, 728–746. doi:10.1016/j.jaerosci.2007.04.005
Vehring, R. (2008). Pharmaceutical particle engineering via spray drying. Pharm. Res. 25, 999–1022. doi:10.1007/s11095-007-9475-1
Wang, H., Ordoubadi, M., Connaughton, P., Lachacz, K., Carrigy, N., Tavernini, S., et al. (2022). Spray dried rugose lipid particle platform for respiratory drug delivery. Pharm. Res. 39, 805–823. doi:10.1007/s11095-022-03242-w
Wang, Z., Wang, H., and Vehring, R. (2021). Leucine enhances the dispersibility of trehalose-containing spray-dried powders on exposure to a high-humidity environment. Int. J. Pharm. 601, 120561. doi:10.1016/j.ijpharm.2021.120561
Williams, B. J., Golomb, M., Phillips, T., Brownlee, J., Olson, M. V., and Smith, A. L. (2002). Bacteriophage HP2 of Haemophilus influenzae. J. Bacteriol. 184, 6893–6905. doi:10.1128/jb.184.24.6893-6905.2002
Xia, G., and Wolz, C. (2014). Phages of Staphylococcus aureus and their impact on host evolution. Infect. Genet. Evol. 21, 593–601. doi:10.1016/j.meegid.2013.04.022
Yan, W., He, R., Tang, X., Tian, B., Liu, Y., Tong, Y., et al. (2021). The influence of formulation components and environmental humidity on spray-dried phage powders for treatment of respiratory infections caused by Acinetobacter baumannii. Pharmaceutics 13, 1162. doi:10.3390/pharmaceutics13081162
Yang, H., Gong, Y., Zhang, H., Etobayeva, I., Miernikiewicz, P., Luo, D., et al. (2019). ClyJ is a novel pneumococcal chimeric lysin with a cysteine-and histidine-dependent amidohydrolase/peptidase catalytic domain. Antimicrob. Agents Chemother. 63, e02043–e02018. doi:10.1128/AAC.02043-18
Yap, M. L., and Rossmann, M. G. (2014). Structure and function of bacteriophage T4. Future Microbiol. 9, 1319–1327. doi:10.2217/fmb.14.91
Zhang, Y., Soto, M., Ghosh, D., and Williams, R. O. (2020). Manufacturing stable bacteriophage powders using thin film freeze-drying technology, bioRxiv.
Żaczek, M., Łusiak-Szelachowska, M., Jończyk-Matysiak, E., Weber-Dąbrowska, B., Międzybrodzki, R., Owczarek, B., et al. (2016). Antibody production in response to staphylococcal MS-1 phage cocktail in patients undergoing phage therapy. Front. Microbiol. 7, 1681. doi:10.3389/fmicb.2016.01681
Keywords: particle engineering, respiratory delivery, spraying techniques, phage therapy, bacteriophage
Citation: Baldelli A and Liang M (2023) Design of respirable sprayed microparticles of encapsulated bacteriophages. Front. Drug Deliv. 3:1209534. doi: 10.3389/fddev.2023.1209534
Received: 20 April 2023; Accepted: 17 May 2023;
Published: 14 June 2023.
Edited by:
Philip Chi Lip Kwok, The University of Sydney, AustraliaReviewed by:
Hideyuki Sato, University of Shizuoka, JapanCopyright © 2023 Baldelli and Liang. This is an open-access article distributed under the terms of the Creative Commons Attribution License (CC BY). The use, distribution or reproduction in other forums is permitted, provided the original author(s) and the copyright owner(s) are credited and that the original publication in this journal is cited, in accordance with accepted academic practice. No use, distribution or reproduction is permitted which does not comply with these terms.
*Correspondence: Alberto Baldelli, YmFsZGVsbGlAbWFpbC51YmMuY2E=; Mingtao Liang, cm9nZXIubGlhbmdAbmV3Y2FzbHRlLmVkdS5hdQ==
Disclaimer: All claims expressed in this article are solely those of the authors and do not necessarily represent those of their affiliated organizations, or those of the publisher, the editors and the reviewers. Any product that may be evaluated in this article or claim that may be made by its manufacturer is not guaranteed or endorsed by the publisher.
Research integrity at Frontiers
Learn more about the work of our research integrity team to safeguard the quality of each article we publish.