- University of Tennessee College of Veterinary Medicine, Knoxville, TN, United States
In vitro drug elution experiments are commonly performed when evaluating the fitness of drug delivery devices for in vivo use. Evaluation of drug elution characteristics spans many drug delivery applications including local delivery of antimicrobials and chemotherapeutics, and is of particular interest for prevention and treatment of orthopedic infections. Despite widespread utility, there is little agreement on methodology to perform such studies, and there are recognized limitations in published works. We evaluated three of the most commonly reported in vitro drug elution methods. We utilized a commercially available collagen matrix (Fibro-Gide®, Geistlich) and an antibiotic that is widely used for local antimicrobial therapy (gentamicin). The protocols used are: 1. complete replacement of media and washing of device, 2. complete replacement of media without washing, or 3. partial replacement of media. The results show statistically significant differences in elution characteristics among the three methods utilizing this delivery vehicle and drug. These results may provide the framework for moving toward more consistent methodology for in vitro elution experiments and address certain acknowledged limitations in the literature.
1 Introduction
Local drug delivery has become a common tool in clinical practice as a way for physicians to deliver medications to patients while minimizing risks of systemic side effects and toxicity that can occur with systemic administration, circulation, and potential accumulation of drugs (Noel et al., 2008; Fleiter et al., 2014; Chapman et al., 2020). Local drug delivery encompasses many applications, including delivery of antimicrobials, growth factors, chemotherapeutic agents, or anesthetic compounds (Noel et al., 2008; Cummings and Chahar, 2012; Wang et al., 2017; Chapman et al., 2020; Worth et al., 2020). With a wide range of applications, the benefits of local drug delivery are considerable, ranging from improving patient’s post-operative comfort (Cummings and Chahar, 2012), increasing drug penetration to target sites to improve therapeutic success (Jackson et al., 2009; Arai et al., 2010), and delivering high local doses of chemotherapy to shrink tumors while avoiding the significant morbidity associated with systemic chemotherapy (Handal et al., 2011; Worth et al., 2020). One benefit in particular, is for the treatment of implant-associated bacterial infection (Caplin and García, 2019; Ter Boo et al., 2015).
Implantable medical devices are immensely important in today’s healthcare system. There is an extensive array of devices, from urinary catheters to prosthetic heart valves and artificial joints. These devices improve the quality of life of tens of millions of patients around the world (Jiang and Zhou, 2009; Van Epps and Younger, 2016; Caplin and García, 2019). Although the lifespans and capabilities of these devices have improved over the years, the risks of bacterial colonization, biofilm formation, and persistent or recurrent bacterial infection remain and constitute a leading cause of device failure (BryersMedical Biofilms, 2008; Stebbins et al., 2014; Caplin and García, 2019). The risk of infection is multifactorial and combines systemic host factors, local tissue environments, and device characteristics (Ter Boo et al., 2015). Treatment of device-associated bacterial infection traditionally involves systemic antimicrobial therapy, local debridement of affected tissues, and often requires device removal and replacement (Darouiche, 2004; Caplin and García, 2019). The use of local drug delivery devices can be used to strengthen the treatment protocol or can be placed initially, in cases where bacterial contamination is known or suspected (Caplin and García, 2019), with the goals of clearing bacterial contamination and preventing persistent bacterial colonization.
Local drug delivery devices serve a broad range of applications. To accommodate these functions, there are many different forms of these devices; including but not limited to: poly (methyl methacrylate) (PMMA) cement (Caplin and García, 2019; Ter Boo et al., 2015), calcium sulfates (Fleiter et al., 2014; Worth et al., 2020), natural and synthetic polymers such as collagen, chitosan, fibrin, polyurethanes, and poly (lactic-co-glycolic acid) (PLGA) (Inzana et al., 2016), and metal alloys (Liu et al., 2019). Within this broad range of materials and applications, there are multiple consistencies in the development of experimental devices for in vivo use. Prior to moving an experimental drug delivery device to an in vivo investigation, the device’s material characteristics should be thoroughly characterized (Mondal and Pal, 2019).The devices will typically be assessed for biocompatibility in order to reduce the risk of a material-induced foreign body response, which is non-conducive to healing (Fenton et al., 2018). The devices will be loaded with the drug they are intended to deliver, to assess in vitro drug elution characteristics. The goal of in vitro elution testing is to gain a sense of how the chosen drug, drug-loading strategy, and device characteristics will contribute to drug release kinetics (Stebbins et al., 2014; Li and Mooney, 2016). Drug elution experiments may aid in determining the expected bioactivity of the released drug throughout the experimental period (Bayston et al., 2009; McLaren et al., 2014; Hassani Besheli et al., 2017). These experiments do not perfectly simulate an in vivo environment, which possesses ever-changing fluid dynamics, but do provide substantial utility in elucidating effects of material formation (Frew et al., 2017; Meeker et al., 2019), porosity (Mau et al., 2004; Anagnostakos and Kelm, 2009; Nugent et al., 2010), and drug variation (Galvez-Lopez et al., 2014) on elution profile. Such experiments are useful as predictors of likely in vivo behavior of local drug delivery devices and can guide the decision to pursue in vivo investigations of experimental devices. This is particularly valuable as investigators strive to practice the “three R’s” of in vivo research, replace, reduce, and refine (Russell and Burch, 1959), and carry only strongly promising materials forward to an animal model.
Although in vitro drug elution experiments are frequently performed and possess utility in determining device fitness for in vivo drug delivery, currently there is no uniform approach to performing the experiments. Most experimental designs utilize one type of media, most commonly phosphate-buffered saline (PBS) (Worth et al., 2020), although some utilize serum (Varela-Moreira et al., 2022). After this point, experimental design parameters such as volume of incubating media, incubation temperature, sampling timepoints, and sampling procedure are all variable. Despite variable experimental parameters, data are presented similarly, via graphs depicting drug elution throughout time. This variability leads to limitations in interpreting results and in comparing devices and drugs. Our aim was to investigate the effects of methodology on drug elution characteristics utilizing one device and drug combination. We utilized a commercially available collagen matrix (Fibro-Gide®, Geistlich) and gentamicin, an antibiotic frequently used for local or systemic treatment of bacterial infections (Swieringa et al., 2008; Bennett-Guerrero et al., 2010; Barth et al., 2011; Fleiter et al., 2014; Ter Boo, 2016). We utilized three of the most commonly reported protocols which involved either 1. complete replacement of media and washing of devices (DiMaio et al., 1994; Kanellakopoulou et al., 1999; Cerretani et al., 2002), 2. complete replacement of media without washing (Jackson et al., 2009; Weiss et al., 2009; Meeker et al., 2019), or 3. partial replacement of media (Aiken et al., 2015; Bohrey et al., 2016; Worth et al., 2020). We hypothesized that the protocol utilized would have a significant effect on the elution characteristics, including the rate of release and amount of recovered gentamicin.
2 Materials and methods
2.1 Material and drug loading
A commercially available collagen matrix (Fibro-Gide®, Geistlich Pharma AG, Wolhusen, Switzerland) was utilized. Fibro-Gide® is provided as a sterilized block that varies in length and width but has a fixed height of 6 mm. For the purpose of these experiments, Fibro-Gide® (15 × 20 × 6 mm) was handled under sterile conditions and sectioned into 5 × 6 mm cylinders utilizing a sterile 5 mm biopsy punch. Experimental cylinders (5 × 6 mm) were created from the material and hydrophilicity was determined by calculating the equilibrium water content (EWC), as listed in Eq. 1.
Dry weights of cylinders (n = 3) were obtained, the cylinders were loaded with PBS and allowed to soak for 24 h, at which point the wet weight was obtained. This process determined the maximum loading volume of experimental cylinders to be 100 µL. This method’s determination step was performed solely to determine drug-loading capacity of the collagen matrix cylinders and therefore does not have a full dataset reported.
Cylinders were loaded by the direct application of the pre-determined volume of 100 µL gentamicin sulfate solution at a concentration of either 100 mg/ml or 50 mg/ml. The 50 mg/ml concentration was created by diluting gentamicin (100 mg/ml) 1:1 with sterile water (for injection). The total amount of gentamicin loaded into the cylinders was either 10 mg or 5 mg, based on the concentration of the solution. These doses were selected based on the maximum saturation of the collagen matrix and the desire to evaluate elution characteristics of both a high and low dose of the drug. The direct application of the antibiotic solution to the material, resulting in material impregnation, was selected as the most commonly utilized drug-loading strategy for collagen-based materials (Inzana et al., 2016). Once gentamicin was applied, the cylinders were allowed to incubate at room temperature for 12 h and were then moved to new wells to begin elution sampling protocols.
2.2 Sampling protocols
After loading, all samples were individually incubated in PBS (2 ml/well) at 37°C. At pre-determined timepoints (3, 24, and 48 h and days: 3, 4, 6, 8, 10, 12, and 14), the samples were removed from incubation for eluant collection. Sample collection occurred under sterile conditions with one of three protocols (n = 3/dose/protocol). The eluant samples were saved in cryovials (in duplicates) at -80°C for analysis.
1 Washing protocol: indwelling media were removed from wells and saved. The samples were washed five times with 2 ml of sterile water (total = 10 ml/well). Fresh PBS was replaced from each well (2 ml/well).
2 Complete turnover protocol: indwelling media were removed from wells and saved. Fresh PBS was replaced in each well (2 ml/well).
3 Partial turnover protocol: a portion of indwelling media (total = 200 μL/well) was removed from wells and saved. An equivalent volume of fresh PBS was replaced in each well (200 μL/well), resulting in a 10% volume dilution of remaining eluant media.
2.3 Drug concentration
The concentration of gentamicin in eluant samples from gentamicin impregnated Fibro-Gide® was determined using ultra high-pressure liquid chromatography (UHPLC) with mass spectrometry detection after dilution of the PBS samples with an internal standard solution (Analytical Chemistry, College of Veterinary Medicine, Service, Iowa State University, Ames, IA). The UHPLC consisted of an UltiMate 3000 Pump, Column Compartment and Autosampler (Thermo Scientific, San Jose, CA, United States) coupled with an Orbitrap mass spectrometer (Q Exactive Focus, Thermo Scientific, San Jose, CA, United States). The analysis was performed by hydrophilic interaction chromatography (HILIC) with a ZIC HILIC column, measuring 150 mm × 2.1 mm, 5 µm particles (Merck KGaA, Darmstadt, Germany through EMD Millipore, MA, United States). Gentamicin consists of a mixture of four components: gentamicin C1 (0.767 fractions of total; gentamicin C2/2a 0.175 fractions; gentamicin C1a 0.058 fractions). Calibration curves for gentamicin C1 and gentamicin C2/2a exhibited a correlation coefficient (r2) exceeding 0.995 across the concentration range. One of three calibration curves for gentamicin C1a had a correlation coefficient (r2) in the 0.985 range, while others exhibited r2 exceeding 0.991. The limit of quantitation (LOQ) was 0.04 μg/ml for gentamicin C1 and 0.01 μg/ml for the other two gentamicin components. The limit of detection (LOD) was 0.01 μg/ml for gentamicin C1 and 0.005 μg/ml for the other two gentamicin components.
3 Statistical analysis
The effects of method, dose, and time on response variable total gentamicin were examined using the mixed model analysis for repeated measures. The ranked transformation was applied when diagnostic analysis on residuals exhibited violation of normality and equal variance assumptions using the Shapiro–Wilk test and Levene’s test. Post hoc multiple comparisons were performed with Tukey’s adjustment. Statistical significance was identified at the level of 0.05. Analyses were conducted using SAS 9.4 TS1M4 (SAS institute Inc., Cary, NC).
4 Results
The samples loaded with 10 mg of gentamicin that underwent the washing (wash) protocol had an elution of 6.88 ± 0.49 mg (68.8 ± 0.49% total drug recovery) during the 14-day study period, with a peak reported elution of 6.84 ± 0.53 mg at 3 hours. This peak elution is equivalent to eluting approximately 68% of loaded gentamicin within the first 3 hours. The samples loaded with 10 mg of gentamicin that underwent the complete turnover (CT) protocol (n = 3), had an elution of 9.97 ± 1.5 mg (99.7 ± 1.5% total drug recovery) during the 14-day study period, with a peak reported elution of 9.14 ± 0.36 mg at 3 hours. This peak elution is equivalent to eluting 91.4 ± 0.36% of loaded gentamicin within the first 3 hours. The samples loaded with 10 mg of gentamicin that underwent the partial turnover (PT) protocol (n = 3), had a peak reported elution of 9.5 ± 2.8 mg at 3 hours. This peak elution is equivalent to eluting 95 ± 28% of the loaded gentamicin within the first 3 hours. The as-measured concentration of gentamicin in the eluant for the PT method represents an accumulation of gentamicin in the media over time and must be corrected for a change in the volume and new drug eluted per sampling interval (Table 1). The PT protocol sample analysis was adjusted to account for repeated sampling and dilution throughout the study period and additional drug eluted during each sampling period (partial turnover calculated and adjusted for volume: PT VA). When adjusted for repeated sampling of accumulated gentamicin, the reported elution (percentage of gentamicin recovered throughout study period) was 63.2 ± 10.9% for the PT VA (Table 1).
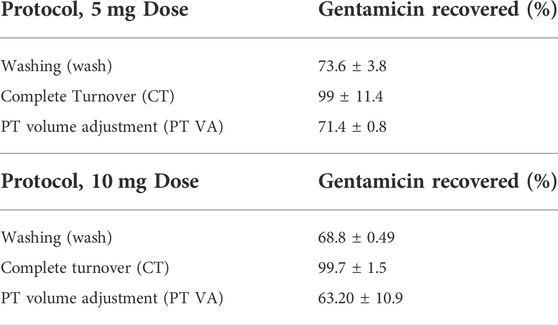
TABLE 1. Percent of gentamicin recovery. Complete turnover protocols recovered the most gentamicin regardless of dose (p < 0.0001). For 5 mg dose, CT protocol recovered statistically greater concentrations of gentamicin compared to wash and PT VA protocols (p = 0.0042, and p < 0.0001, respectively). For 10 mg dose, CT protocol recovered statistically greater concentrations of gentamicin compared to wash and PT VA protocols (p = 0.01 and p < 0.0001, respectively).
The samples loaded with 5 mg of gentamicin that underwent the wash protocol had an elution of 3.68 ± 0.19 mg (73.6 ± 3.8% total drug recovery) during the 14-day study period, with a peak reported elution of 3.62 ± 0.09 mg at 3 hours. This peak elution is equivalent to eluting 72.4 ± 1.8% of loaded gentamicin within the first 3 hours. The samples loaded with 5 mg of gentamicin that underwent the CT protocol (n = 3), had an elution of 4.95 ± 0.57 mg (99 ± 11.4% total drug recovery) during the 14-day study period, with a peak reported elution of 4.34 ± 0.31 mg at 3 hours. This peak elution is equivalent to eluting 86.8 ± 6.2% of loaded gentamicin within the first 3 hours. The samples loaded with 5 mg of gentamicin that underwent the PT protocol (n = 3), had a peak reported elution of 3.69 ± 0.09 mg at 3 hours. This peak elution is equivalent to eluting 73.8 ± 1.8% of loaded gentamicin within the first 3 hours. The PT protocol sample analysis was adjusted to account for repeated sampling and dilution throughout the study period and additional drugs eluted during each sampling period (partial turnover calculated and adjusted for volume: PT VA). When adjusted for repeated sampling of accumulated gentamicin, the reported elution (percentage of gentamicin recovered throughout the study period) was 71.4 ± 0.8% for the PT VA (Table 1).
There were significant differences found between doses of gentamicin loaded, between sampling protocols, and as a factor of time. The PT VA protocol resulted in elution of similar amounts of the drug compared with the wash protocol. The CT protocol eluted statistically significantly greater concentrations of gentamicin regardless of the loaded dose (p < 0.0001). For the 5 mg dose, the CT protocol recovered statistically greater concentrations of gentamicin compared to wash and PT VA protocols (p = 0.0042, and p < 0.0001, respectively). For 10 mg dose, the CT protocol recovered statistically greater concentrations of gentamicin compared to wash and PT VA protocols (p = 0.01 and p < 0.0001, respectively). The samples loaded with 10 mg of gentamicin eluted statistically more gentamicin than samples loaded with 5 mg (p < 0.0001). For the CT and wash protocols, there were significant differences found between gentamicin concentrations at consecutive timepoints; the CT protocol eluted statistically greater amounts of gentamicin at 3 hours compared to 24 h (p < 0.0001), as well as at day 4 compared to day 6 (p < 0.0001), and day 12 compared to day 14 (p = 0.01). Throughout the wash protocol, statistically greater amounts of gentamicin were found at 3 hours compared to 24 h (p < 0.0001) and remaining consecutive timepoints did not differ significantly. Within the PT VA protocol, there were no statistically significant differences in gentamicin concentrations between consecutive timepoints. Each protocol and dose with the exception of the PT protocol demonstrated similar elution profiles, characterized by an initial burst release of the majority of drugs within a 3-h period, followed by a sustained, low-level drug release. The PT protocol, without adjustments for drug accumulation and repeated sampling through time, demonstrated a gradual, sustained elution curve with little change over time (Figure 1). The PT protocol when adjusted to reflect removal of accumulated gentamicin (partial turnover calculated to reflect newly eluted drug: PT ND) demonstrated an initial burst release, followed by the sustained, low-level release (Figure 2).
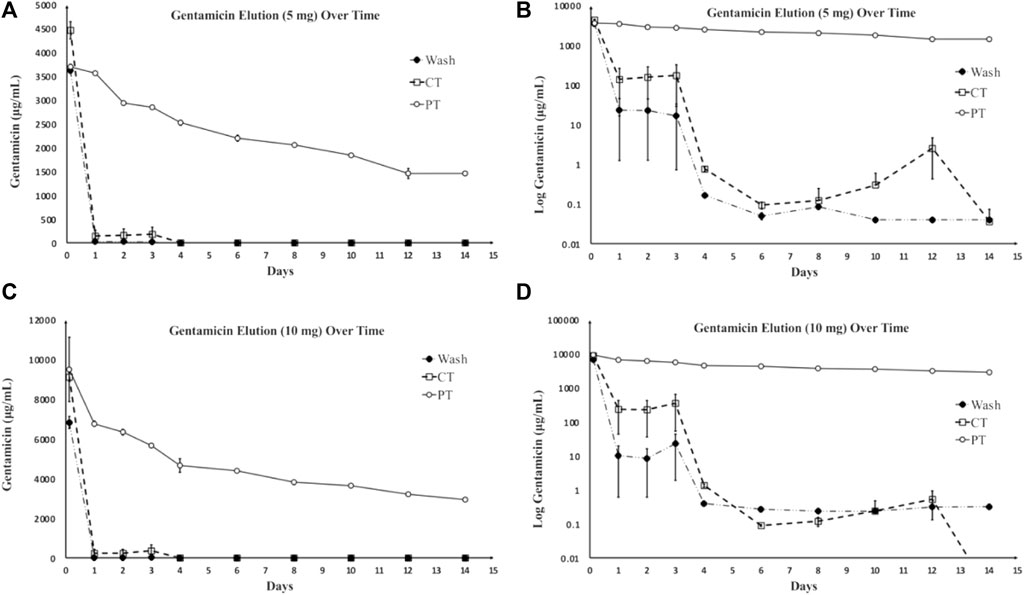
FIGURE 1. Gentamicin concentration as a result of elution over time. (A) Average eluted gentamicin generated by each method with a loaded dose of 5 mg gentamicin/device. (B) Log transformation applied to elution curves generated each method with a loaded dose of 5 mg gentamicin/device. (C) Average eluted gentamicin generated by each method with a loaded dose of 10 mg gentamicin/device. (D) Log transformation applied to elution curves generated each method with a loaded dose of 10 mg gentamicin/device. Due to the wide concentration range, log transformation facilitates most clear data visualization (Adams et al., 1992). Wash and CT elution curves demonstrate the anticipated initial burst release of drug followed by a sustained lower-level drug release. PT protocols demonstrate gradual, sustained elution curves, which is characteristic of a desirable elution profile (Mondal and Pal, 2019). Protocols represented as: PT = partial turnover, wash = washing, CT = complete turnover.
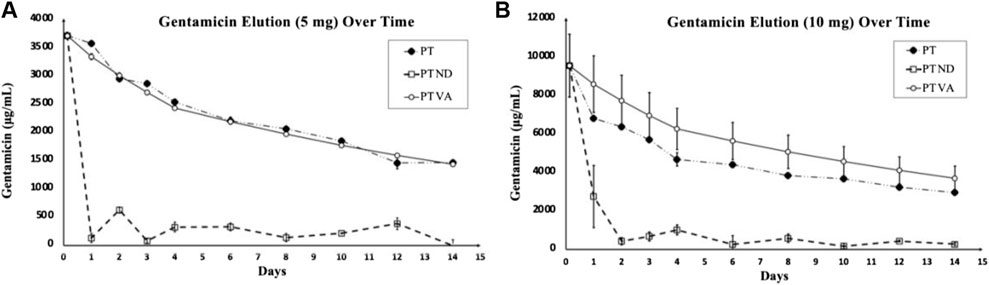
FIGURE 2. Gentamicin concentration as a result of elution over time generated by the PT protocol. (A) (B) Elution curves of average gentamicin eluted under the PT protocol with no adjustments (PT), adjusted for volume and accumulated gentamicin over time (PT VA) and adjusted to reflect removal of accumulated gentamicin “partial turnover calculated to reflect the newly eluted drug” (PT ND). (A) Devices loaded with 5 mg gentamicin. (B) Devices loaded with 10 mg gentamicin. PT and PT VA demonstrate gradual, sustained elution over time compared to PT ND that demonstrates the characteristic burst release followed by a sustained lower-level release.
5 Discussion
The results of this method comparison demonstrated significant differences between protocols and also demonstrated differences in the generated elution curves. These results were anticipated, and are relevant within the research area of local drug delivery devices. This study confirms that varying elution protocols within an otherwise controlled experimental design may result in significantly different elution profiles and amounts of the recovered drug. It can be extrapolated from these results that the selected sampling protocol has significant effects on the concentration of the drug in the eluant, amount of the drug recovered, as well as the elution curve generated from the data. Therefore, the sampling protocol should be selected with study goals in mind and results should be interpreted in light of the selected in vitro environment.
An important portion of the aforementioned results is the PT, PT VA, and PT ND protocol results. Without adjusting the PT protocol for the volume of media removed at each sampling point and calculating the amount of the additional drug eluted during the associated sampling period, the percentage of gentamicin recovery would erroneously appear to be much greater than the amount of the drug loaded. This can be attributed to the nature of the PT protocol. Although the PT protocol demonstrates the theoretical ideal drug elution profile, a gradual and sustained curve (Mondal and Pal, 2019), sampling removes so little of the media surrounding the drug delivery device that the results cannot be interpreted with respect to the amount of drug concentration measured at each time point. Without fully removing the surrounding media, the amount of the new drug eluted per sampling period can only be estimated based on changes in the concentration. This can lead to erroneous interpretation of results when there is a low-level drug release, as variations exist in the precision of drug concentration analysis. This elution protocol would likely only be relevant to model a device utilized to elute the drug in a confined space or severely compromised tissue where the eluted drug could not be eliminated.
These findings, while not unexpected, are important, as they suggest that when performing in vitro drug elution experiments, it would be valuable to carefully consider the protocol to be used in the context with the literature relative to the most commonly utilized protocols in the investigator’s specific area of interest, and then to also consider the goal of the elution experiment. Most often, investigators consider the goal of in vitro elution experiments to be the assessment of the material for in vivo use through the generation of a predictable, translational, in vitro to in vivo elution profile (Taylor et al., 2007; Weiss et al., 2009; Worth et al., 2020; Beenken et al., 2021). This goal is often unattainable, as the in vivo environment is difficult to simulate, and even a well-designed in vitro environment will differ in certain aspects. When the ever-changing fluid dynamics of the body are considered, it can be reasonably argued that a washing protocol or a continuous flow protocol (Perry et al., 2002) would most accurately simulate an in vivo environment. However, most recent reports of in vitro elution experiments for local drug delivery devices have focused on either a partial or complete turnover protocol (Ordikhani et al., 2016; Meeker et al., 2019; Worth et al., 2020). This may reflect the desire to model areas of tissue injury when lymphatics and vasculature are damaged and fluid dynamics are altered, leading to fluid accumulation, such as following trauma or radical debridement. Such experimental protocols may demonstrate more favorable elution curves and greater drug concentrations throughout the study period, as fewer drugs will be lost as waste during the washing process. If the same device, drug, and dose combinations were moved to an in vivo environment, it is likely that a far lower concentration of drug would be recovered.
It is important to acknowledge that this study focused on one device and drug combination and one loading mechanism; and it is important to note the loading mechanism. The collagen matrix cylinders in this experiment were loaded by impregnation, which facilitates drug incorporation by formation of weak bonds such as Van der Waals forces. This simulated an intra-operative technique for adding antimicrobial drugs to tissue scaffolds at the time of surgery. Under the conditions of this experiment, and using a collagen scaffold, there was no significant benefit to loading a greater amount of drug relative to the elution curve as the vast majority of drug was eluted within a short period of time. Local drug delivery devices that are loaded via impregnation typically exhibit an initial burst release of the drug, followed by a lower-level release, for variable amounts of time (Brigham et al., 2021). This is in contrast to materials that are loaded via specific molecular linkages or stimuli-responsive systems, which may exhibit more finely tunable controlled-release profiles (Inzana et al., 2016; Kaiser et al., 2021).
6 Conclusion and future directions
This study demonstrates that the sampling protocol may have significant effects on in vitro drug elution profiles. This is both an expected and relevant phenomenon within in vitro drug elution experiments. When investigating the in vitro drug elution characteristics of local drug delivery devices, experimental design is important, and sampling parameters should be selected with careful consideration of the experimental question. As the overall goal of determining the in vitro drug elution profile of local drug delivery devices is to determine suitability for in vivo usage and predict in vivo drug elution profiles, it would be interesting to compare the in vitro profiles generated by these devices, drugs, and dose combinations with in vivo profiles generated by the same device, drug, and dose combinations. This may elucidate an experimental design that is closest to simulating an in vivo environment and aid in the experimental set-up. It may also be of value to pursue a similar methodology comparison utilizing media within a range of pHs. PBS (pH 7.4) is the most commonly utilized media for in vitro elution experiments (Weiss et al., 2009; Worth et al., 2020; Beenken et al., 2021), but in vivo environments may have pH variation, particularly in areas of tissue damage or implant-associated infection, where the pH may be more acidic.
Data availability statement
The original contributions presented in the study are included in the article/Supplementary Materials; further inquiries can be directed to the corresponding author.
Author contributions
CB, DEA: conceptualization, writing, and editing.
Funding
Funding for open access to this research was provided by University of Tennessee’s Open Publishing Support Fund.
Acknowledgments
Kassandra Downing for technical support, Analytical Chemistry, College of Veterinary Medicine, Service, Iowa State University, Ames, IA for gentamicin concentration analysis, Xiaocun Sun for statistical support and chart generation.
Conflict of interest
The authors declare that the research was conducted in the absence of any commercial or financial relationships that could be construed as a potential conflict of interest.
Publisher’s note
All claims expressed in this article are solely those of the authors and do not necessarily represent those of their affiliated organizations, or those of the publisher, the editors, and the reviewers. Any product that may be evaluated in this article, or claim that may be made by its manufacturer, is not guaranteed or endorsed by the publisher.
References
Adams, K., Couch, L., Cierny, G., al, E., and Mader, J. T. (1992). In vitro and in vivo evaluation of antibiotic diffusion from antibiotic-impregnated polymethylmethacrylate beads. Clin. Orthop. Relat. Res. 278, 244–252. doi:10.1097/00003086-199205000-00037
Aiken, S. S., Cooper, J. J., Florance, H., Robinson, M. T., and Michell, S. (2015). Local release of antibiotics for surgical site infection management using high-purity calcium sulfate: An in vitro elution study. Surg. Infect. 16 (1), 54–61. Epub 2014/08/26. doi:10.1089/sur.2013.162
Anagnostakos, K., and Kelm, J. (2009). Enhancement of antibiotic elution from acrylic bone cement. J. Biomed. Mat. Res. B Appl. Biomater. 90 (1), 467–475. Epub 2009/01/16. doi:10.1002/jbm.b.31281
Arai, T., Benny, O., Joki, T., Menon, L. G., Machluf, M., Abe, T., et al. (2010). Novel local drug delivery system using thermoreversible gel in combination with polymeric microspheres or liposomes. Anticancer Res. 30, 1057–1064.
Barth, R. E., Vogely, H. C., Hoepelman, A. I., and Peters, E. J. (2011). 'To bead or not to bead?' treatment of osteomyelitis and prosthetic joint-associated infections with gentamicin bead chains. Int. J. Antimicrob. Agents 38 (5), 371–375. Epub 20110506. doi:10.1016/j.ijantimicag.2011.03.008
Bayston, R., Fisher, L. E., and Weber, K. (2009). An antimicrobial modified silicone peritoneal catheter with activity against both gram-positive and gram-negative bacteria. Biomaterials 30 (18), 3167–3173. Epub 20090316. doi:10.1016/j.biomaterials.2009.02.028
Beenken, K. E., Campbell, M. J., Ramirez, A. M., Alghazali, K., Walker, C. M., Jackson, B., et al. (2021). Evaluation of a bone filler scaffold for local antibiotic delivery to prevent Staphylococcus aureus infection in a contaminated bone defect. Sci. Rep. 11 (1), 10254. doi:10.1038/s41598-021-89830-z
Bennett-Guerrero, E., Ferguson, T. B., Lin, M., Garg, J., Mark, D. B., Scavo, V. A., et al. (2010). Effect of an implantable gentamicin-collagen sponge on sternal wound infections following cardiac surgery: A randomized trial. JAMA 304 (7), 755–762. doi:10.1001/jama.2010.1152
Bohrey, S., Chourasiya, V., and Pandey, A. (2016). Polymeric nanoparticles containing diazepam: Preparation, optimization, characterization, in-vitro drug release and release kinetic study. Nano Converg. 3 (1). doi:10.1186/s40580-016-0061-2
Brigham, N. C., Ji, R-R., and Becker, M. L. (2021). Degradable polymeric vehicles for postoperative pain management. Nat. Commun. 12 (1), 1367. doi:10.1038/s41467-021-21438-3
BryersMedical Biofilms, J. D. (2008). Medical biofilms. Biotechnol. Bioeng. 100 (1), 1–18. doi:10.1002/bit.21838
Caplin, J. D., and García, A. J. (2019). Implantable Antimicrobial biomaterials for local drug delivery in bone infection models. Acta Biomater. 93, 2–11. doi:10.1016/j.actbio.2019.01.015
Cerretani, D., Giorgi, G., Fornara, P., Bocchi, L., Neri, L., Ceffa, R., et al. (2002). The in vitro elution characteristics of vancomycin combined with imipenem-cilastatin in acrylic bone-cements: A pharmacokinetic study. J. Arthroplasty 17 (5), 619–626. doi:10.1054/arth.2002.32184
Chapman, C. A. R., Cuttaz, E. A., Goding, J. A., and Green, R. A. (2020). Actively controlled local drug delivery using conductive polymer-based devices. Appl. Phys. Lett. 116 (1), 010501. doi:10.1063/1.5138587
Cummings, I. K., and Chahar, P. (2012). Liposomal bupivacaine: A review of a new bupivacaine formulation. J. Pain Res. 5, 257–264. doi:10.2147/jpr.s27894
Darouiche, R. (2004). Treatment of infections associated with surgical implants. N. Engl. J. Med. 350 (14), 1422–1429. doi:10.1056/NEJMra035415
DiMaio, F. R., O’Halloran, J. J., and Quale, J. M. (1994). In vitro elution of ciprofloxacin from polymethylmethacrylate cement beads. J. Orthop. Res. 12, 79–82. doi:10.1002/jor.1100120110
Fenton, O. S., Olafson, K. N., Pillai, P. S., Mitchell, M. J., and Langer, R. (2018). Advances in biomaterials for drug delivery. Adv. Mat. 30, e1705328. Epub 2018/05/08. doi:10.1002/adma.201705328
Fleiter, N., Walter, G., Bosebeck, H., Vogt, S., Büchner, H., Hirschberger, W., et al. (2014). Clinical use and safety of a novel gentamicin-releasing resorbable bone graft substitute in the treatment of osteomyelitis/osteitis. Bone Jt. Res. 3 (7), 223–229. doi:10.1302/2046-3758.37.2000301
Frew, N. M., Cannon, T., Nichol, T., Smith, T. J., and Stockley, I. (2017). Comparison of the elution properties of commercially available gentamicin and bone cement containing vancomycin with 'home-made' preparations. Bone Jt. J. 99-B (1), 73–77. Epub 2017/01/06. doi:10.1302/0301-620X.99B1.BJJ-2016-0566.R1
Galvez-Lopez, R., Pena-Monje, A., Antelo-Lorenzo, R., Guardia-Olmedo, J., Moliz, J., Hernandez-Quero, J., et al. (2014). Elution kinetics, antimicrobial activity, and mechanical properties of 11 different antibiotic loaded acrylic bone cement. Diagn. Microbiol. Infect. Dis. 78 (1), 70–74. Epub 2013/11/16. doi:10.1016/j.diagmicrobio.2013.09.014
Handal, J. A., Schulz, J. F., Pahys, J. M., Williams, E. A., Kwok, S. C., and Samuel, S. P. (2011). Evaluation of elution and mechanical properties of two injectable chemotherapeutic bone cements. Chemotherapy 57 (3), 268–274. Epub 20110606. doi:10.1159/000327388
Hassani Besheli, N., Mottaghitalab, F., Eslami, M., Gholami, M., Kundu, S. C., Kaplan, D. L., et al. (2017). Sustainable release of vancomycin from silk fibroin nanoparticles for treating severe bone infection in rat tibia osteomyelitis model. ACS Appl. Mat. Interfaces 9 (6), 5128–5138. Epub 20170206. doi:10.1021/acsami.6b14912
Inzana, J. A., Schwarz, E. M., Kates, S. L., and Awad, H. A. (2016). Biomaterials approaches to treating implant-associated osteomyelitis. Biomaterials 81, 58–71. Epub 2016/01/03. doi:10.1016/j.biomaterials.2015.12.012
Jackson, S. R., Richelsoph, K. C., Courtney, H. S., Wenke, J. C., Branstetter, J. G., Bumgardner, J. D., et al. (2009). Preliminary in vitro evaluation of an adjunctive therapy for extremity wound infection reduction: Rapidly resorbing local antibiotic delivery. J. Orthop. Res. 27 (7), 903–908. Epub 2008/12/24. doi:10.1002/jor.20828
Jiang, G., and Zhou, D. D. (2009). “Technology Advances and challenges in hermetic packaging for implantable medical devices,” in Implantable neural prostheses 2 (Springer, NY: Biological and Medical Physics, Biomedical Engineering), 27–61.
Kaiser, P., Wächter, J., and Windbergs, M. (2021). Therapy of infected wounds: Overcoming clinical challenges by advanced drug delivery systems. Drug Deliv. Transl. Res. 11 (4), 1545–1567. doi:10.1007/s13346-021-00932-7
Kanellakopoulou, K., Kolia, M., Anastassiadis, A., Korakis, T., Giamarellos-Bourboulis, E. J., Andreopoulos, A., et al. (1999). Lactic acid polymers as biodegradable carriers of fluoroquinolones: An in vitro study. Antimicrob. Agents Chemother. 43 (3), 714–716. doi:10.1128/aac.43.3.714
Li, J., and Mooney, D. J. (2016). Designing hydrogels for controlled drug delivery. Nat. Rev. Mat. 1 (12), 16071. doi:10.1038/natrevmats.2016.71
Liu, Y., Lu, B., and Cai, Z. (2019). Recent progress on Mg- and Zn-based alloys for biodegradable vascular stent applications. J. Nanomater. 2019, 1–16. doi:10.1155/2019/1310792
Mau, H., Schelling, K., Heisel, C., Wang, J. S., and Breusch, S. J. (2004). Comparison of various vacuum mixing systems and bone cements as regards reliability, porosity and bending strength. Acta Orthop. Scand. 75 (2), 160–172. Epub 2004/06/08. doi:10.1080/00016470412331294415
McLaren, J. S., White, L. J., Cox, H. C., Ashraf, W., Rahman, C. V., Blunn, G. W., et al. (2014). A biodegradable antibiotic-impregnated scaffold to prevent osteomyelitis in a contaminated in vivo bone defect model. Eur. Cell. Mat. 27, 332–349. Epub 2014/06/09. doi:10.22203/ecm.v027a24
Meeker, D. G., Cooper, K. B., Renard, R. L., Mears, S. C., Smeltzer, M. S., and Barnes, C. L. (2019). Comparative study of antibiotic elution profiles from alternative formulations of polymethylmethacrylate bone cement. J. Arthroplasty 34 (7), 1458–1461. Epub 2019/04/03. doi:10.1016/j.arth.2019.03.008
Mondal, S., and Pal, U. (2019). 3d hydroxyapatite scaffold for bone regeneration and local drug delivery applications. J. Drug Deliv. Sci. Technol. 53, 101131. doi:10.1016/j.jddst.2019.101131
Noel, S. P., Courtney, H., Bumgardner, J. D., and Haggard, W. O. (2008). Chitosan films: A potential local drug delivery system for antibiotics. Clin. Orthop. Relat. Res. 466 (6), 1377–1382. doi:10.1007/s11999-008-0228-1
Nugent, M., McLaren, A., Vernon, B., and McLemore, R. (2010). Strength of antimicrobial bone cement decreases with increased poragen fraction. Clin. Orthop. Relat. Res. 468 (8), 2101–2106. Epub 2010/02/18. doi:10.1007/s11999-010-1264-1
Ordikhani, F., Zustiak, S. P., and Simchi, A. (2016). Surface modifications of titanium implants by multilayer bioactive coatings with drug delivery potential: Antimicrobial, biological, and drug release studies. JOM 68 (4), 1100–1108. doi:10.1007/s11837-016-1840-2
Perry, A. C., Rouse, M. S., Khaliq, Y., al, E., Hanssen, A. D., Osmon, D. R., et al. (2002). Antimicrobial release kinetics from polymethylmethacrylate in a novel continuous flow chamber. Clin. Orthop. Relat. Res. 403, 49–53. doi:10.1097/00003086-200210000-00009
Russell, W. M. S., and Burch, R. L. (1959). The principles of humane experimental technique. London: Methuen and Co. LTD.
Stebbins, N. D., Ouimet, M. A., and Uhrich, K. E. (2014). Antibiotic-containing polymers for localized, sustained drug delivery. Adv. Drug Deliv. Rev. 78, 77–87. Epub 2014/04/23. doi:10.1016/j.addr.2014.04.006
Swieringa, A. J., Goosen, J. H., Jansman, F. G., and Tulp, N. J. (2008). In vivo pharmacokinetics of a gentamicin-loaded collagen sponge in acute periprosthetic infection: Serum values in 19 patients. Acta Orthop. 79 (5), 637–642. doi:10.1080/17453670810016650
Taylor, R. R., Tang, Y., Gonzalez, M. V., Stratford, P. W., and Lewis, A. L. (2007). Irinotecan drug eluting beads for use in chemoembolization: In vitro and in vivo evaluation of drug release properties. Eur. J. Pharm. Sci. 30 (1), 7–14. Epub 20060915. doi:10.1016/j.ejps.2006.09.002
Ter Boo, G. J. (2016). Delivery of gentamicin from resorbable polymeric carriers as anti-infective strategy for implant-associated osteomyelitis. Enschede, Netherlands: University of Twente.
Ter Boo, G. J., Grijpma, D. W., Moriarty, T. F., Richards, R. G., and Eglin, D. (2015). Antimicrobial delivery systems for local infection prophylaxis in orthopedic- and trauma surgery. Biomaterials 52, 113–125. Epub 2015/03/31. doi:10.1016/j.biomaterials.2015.02.020
Van Epps, J. S., and Younger, J. G. (2016). Implantable device-related infection. Shock 46 (6), 597–608. doi:10.1097/SHK.0000000000000692
Varela-Moreira, A., van Leur, H., Krijgsman, D., Ecker, V., Braun, M., Buchner, M., et al. (2022). Utilizing in vitro drug release assays to predict in vivo drug retention in micelles. Int. J. Pharm. 618, 121638. Epub 20220305. doi:10.1016/j.ijpharm.2022.121638
Wang, Z., Wang, Z., Lu, W. W., Zhen, W., Yang, D., and Peng, S. (2017). Novel biomaterial strategies for controlled growth factor delivery for biomedical applications. NPG Asia Mat. 9 (10), e435–e. doi:10.1038/am.2017.171
Weiss, B. D., Weiss, E. C., Haggard, W. O., Evans, R. P., McLaren, S. G., and Smeltzer, M. S. (2009). Optimized elution of daptomycin from polymethylmethacrylate beads. Antimicrob. Agents Chemother. 53 (1), 264–266. Epub 2008/10/29. doi:10.1128/AAC.00258-08
Keywords: in vitro drug elution, local drug delivery, drug delivery device, collagen, gentamicin
Citation: Billings C and Anderson DE (2022) Comparison of three methods for assessment of drug elution: In vitro elution of gentamicin from a collagen-based scaffold. Front. Drug. Deliv. 2:958731. doi: 10.3389/fddev.2022.958731
Received: 31 May 2022; Accepted: 06 July 2022;
Published: 23 August 2022.
Edited by:
Mohammad-Ali Shahbazi, University Medical Center Groningen, NetherlandsReviewed by:
Fatemeh Ghorbani-Bidkorpeh, Shahid Beheshti University of Medical Sciences, IranElham Asadian, Shahid Beheshti University of Medical Sciences, Iran
Copyright © 2022 Billings and Anderson. This is an open-access article distributed under the terms of the Creative Commons Attribution License (CC BY). The use, distribution or reproduction in other forums is permitted, provided the original author(s) and the copyright owner(s) are credited and that the original publication in this journal is cited, in accordance with accepted academic practice. No use, distribution or reproduction is permitted which does not comply with these terms.
*Correspondence: Caroline Billings, Y2JpbGxpMTBAdm9scy51dGsuZWR1