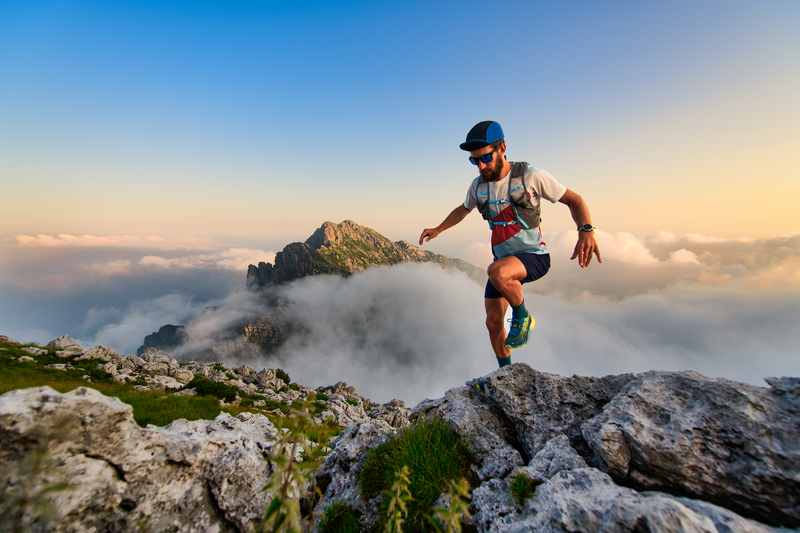
94% of researchers rate our articles as excellent or good
Learn more about the work of our research integrity team to safeguard the quality of each article we publish.
Find out more
MINI REVIEW article
Front. Drug Deliv. , 12 July 2022
Sec. CNS Drug Delivery
Volume 2 - 2022 | https://doi.org/10.3389/fddev.2022.946045
This article is part of the Research Topic Endo- and Transcytotic Pathways at the Brain Barriers View all 5 articles
Biologics are at the frontier of CNS disease treatment. This applies both to therapeutic molecules such as peptides, antibodies and RNA interference agents, and to delivery vehicles of biological origin such as viral vectors and extracellular vesicles. Unlike small molecules, biologics are not likely to diffuse across cell membranes. To get into and across brain capillary endothelial cells (BCEC) forming the blood–brain barrier, they normally employ active, energy-dependent processes. They can initiate these processes non-specifically or trigger them by interaction with various receptor or transporter molecules at the luminal surface of BCEC. Designing biologics to use this specific engagement is more common in smaller formats, especially peptides and antibodies, but can also apply to targeted vehicles. This targeted design has employed a number of molecules expressed on BCEC – the transferrin receptor being the most common example, although there has been progress in identifying molecules that are even more specific to BCEC. In addition, the format of biologics and a multitude of their biophysical properties affect the way they interact with BCEC, and this diversity is even more salient between different classes of biologics. It affects the entire span of interaction with BCEC, from the initial engagement at the luminal surface to intracellular sorting, and eventually, entrapment or routing toward exocytosis into the brain parenchyma. In this article, I reviewed the progress in identifying novel targets that make the interactions between biologics and BCEC more specific, and in our understanding of the interplay between the properties of biologics and these interactions.
Brain targeting is needed for intravenously (IV) administered biologics because otherwise their brain accumulation after an IV injection is low. The statement above is an oversimplification on several counts. First, ‘low’ is relative: for instance, while ∼0.1% of the injected dose is indicated for monoclonal antibodies (mAbs) (Bard et al., 2000; Boado et al., 2010; Atwal et al., 2011), the relevant question is how much of the therapeutic needs to reach brain parenchyma to achieve a sufficient effect, and, consequently, whether the safety profile allows reaching that level. Second, the site of entry may well be crucial: a fraction of a biologic reaching the brain across the blood–brain barrier (BBB) may have a different distribution and, consequently, effect than the same fraction entering the brain from the choroid plexus, owing to the high density of the capillary bed with small (∼25 µm) distance to the nearest cells such as neurons (Schlageter et al., 1999; Mabuchi et al., 2005). Third, unmodified large biologics serving as delivery vehicles—e.g., adeno-associated viruses (AAVs) (Foust et al., 2009) or extracellular vesicles (EVs) (Wiklander et al., 2015; Banks et al., 2020)—have been shown to reach the brain; although the extent of this accumulation varies considerably, the percentage of the injected dose also has a different meaning in this scenario, at least for EVs because of their large loading capacity.
Nevertheless, targeting has been shown to increase brain transport, and therefore is an attractive option at least for that reason alone, and, possibly, for getting access to a greater brain volume. Here, I focus on several aspects: 1) interactions of non-targeted large biologics, 2) strategies for choosing targets and optimizing interactions with them, 3) considerations relevant for different formats of biologics, and 4) transport mechanisms helping them cross the BBB.
If a vehicle is not intentionally targeted to certain molecules on brain capillary endothelial cells (BCEC), this does not necessarily mean that its interaction with BCEC is not specific. An early example comes from nanomedicine, where the interaction of nanoparticles (NPs) with BCEC is thought to be at least partially mediated by apolipoprotein E (ApoE) recruited in the bloodstream as part of the protein corona and subsequently interacting with the low-density lipoprotein receptor (LDLR) on the surface of BCEC (Kreuter et al., 2002). The point here is not so much ApoE per se but the fact that the recruitment of proteins from the bloodstream can mediate specific interactions with cellular receptors in hepatic or extra-hepatic delivery (Akinc et al., 2010; Dilliard et al., 2021). It seems reasonable to expect similar mechanisms applied to EVs, where not only biophysical properties, but also the protein signature on the EV surface can affect corona formation—or, indeed, itself mediate specific interactions with BCEC (Haqqani et al., 2013; Qu et al., 2018). Another example comes from AAV studies where the transport of PHP.eB capsid across the BBB was found to be mediated by lymphocyte antigen 6 complex, locus A (Ly6a)—notably, explaining the lack of PHP.eB transport in other species that do not express Ly6a, and, partially, in other mouse strains which have a reduced amount or an altered genetic variant of it (Hordeaux et al., 2018; Huang et al., 2019; Mathiesen et al., 2020). These observations point that non-targeted biologics, especially in large formats, may turn out to engage specific targets—or their combination—on the surface of BCEC, owing to their intrinsic properties or the properties they acquire in the bloodstream. Furthermore, the complexity of vehicles can make them likely to engage several extracellular and cellular actors spanning the entire range from the glycocalyx to the plasma membrane and intracellular sorting, to exocytosis on the abluminal side. AAV field again offers an example, with cellular interactions of AAV9 mediated by at least three different receptors, terminal β-galactose on glycocalyx, laminin receptor, and adeno-associated virus receptor (Akache et al., 2006; Shen et al., 2011; Pillay et al., 2017). This essentially redefines targeting, shifting it from the luminal membrane of BCEC to all cellular interactions, where individual steps of internalization, sorting, and exocytosis can be more or less specific to BCEC. It is unclear whether this level of specificity can be designed rationally. However, large-scale screening, common for AAVs and, more recently, lipid NPs (whose lessons can, to some extent, apply to EVs) (Akinc et al., 2008; Whitehead et al., 2014; Dahlman et al., 2017) can provide insights by generating BBB-penetrant vehicles (e.g., AAV capsids PHP.B, PHP.eB, AAV.F, CAP-B10, CAP-B22, CAP-Mac, 9P801, etc.) (Deverman et al., 2016; Hanlon et al., 2019; Nonnenmacher et al., 2021; Chuapoco et al., 2022; Goertsen et al., 2022) but also by identifying patterns promoting accumulation in different organs, including the brain, at least within a given class. This data, especially if available for different species, could then inform a computational design (Ogden et al., 2019; Bryant et al., 2021).
I will focus on optimizing biologics for targeted delivery, the choice and characterization of targets, and the possible benefits of combinatorial targeting, using the transferrin receptor (TfR) and low-density lipoprotein receptor-1 (LRP-1) to illustrate the first two aspects. A detailed account of other commonly used BCEC targets and their use in delivery across BBB can be found in comprehensive reviews elsewhere (Lajoie and Shusta 2015; Terstappen et al., 2021).
Since the discovery of the TfR expression on BCEC (Jefferies et al., 1984), and the initial attempts to use it as a molecular Trojan horse (Friden et al., 1991; Friden et al., 1993; Huwyler et al., 1996), TfR has remained among the most studied shuttle targets. It has been used to enhance the transport of a broad range of molecules and vehicles, including peptides, mAbs, EVs, or even AAVs (Friden et al., 1993; Wu and Pardridge 1998; Yu et al., 2011; Zhang et al., 2018; Crook et al., 2020; Kim et al., 2020). The rationale for using TfR would be its high expression on BCEC and specificity to BCEC compared to other endothelial cells (Jefferies et al., 1984), although this specificity is limited as TfR is also highly expressed on certain cells of non-endothelial origin, including, notably, reticulocytes (Jandl and Katz 1963; Couch et al., 2013; Sun et al., 2019). A TfR-targeted biologic is also the only currently approved large molecule exploiting targeted delivery across the BBB—pabinafusp alfa, approved in Japan for the treatment of mucopolysaccharidosis type II, comprises iduronate-2-sulfatase fused to a high-affinity, bivalent TfR mAb (Giugliani et al., 2021). TfR-mediated delivery optimization has taken much effort, focusing primarily on antibodies or their fragments enabling the transport of payloads or large vehicles across the BBB. Insights derived from this optimization are likely relevant for other targets, although, ultimately, every biologic-target complex may have its unique signature affecting BBB transport.
Early studies using TfR mAbs employed them in a bivalent format, with the mAb fused to the therapeutic payload or decorating larger vehicles which could themselves contain the payload. While there is little doubt that this allowed TfR-targeted constructs to internalize in BCEC, one key factor in the interpretation of these studies is whether the constructs would leave BCEC to enter brain parenchyma. Capillary depletion studies have shown that bivalent TfR constructs remained largely restricted to brain endothelium (Moos and Morgan 2001). Subsequently, (Yu et al., 2011) improved brain transport by reducing the affinity to TfR in a bispecific mAb with one arm targeting TfR and the other β-secretase (BACE1), while (Niewoehner et al., 2014) refined the optimization from a different angle, improving the transport of a bivalent anti-amyloid β mAb by fusing a Fab fragment of an anti-TfR mAb to its heavy chain, thus enforcing monovalent binding to TfR. Clearly, as far as TfR engagement is concerned, the approaches taken in (Yu et al., 2011) and (Niewoehner et al., 2014) are not entirely dissimilar since a bispecific antibody with one arm targeting TfR is essentially a monovalent shuttle. An interesting attempt to combine the bivalent format with the monovalent binding mode was made in (Hultqvist et al., 2017), where a bivalent TfR mAb was forced to engage TfR as a monovalent binder by a linker sterically preventing bivalent binding. Further optimization included the use of single-domain antibodies with low (Wouters et al., 2020) or high (Stocki et al., 2021) affinities, the use of a pH-dependent binder (Sade et al., 2014), and a variation of the monovalent, low-affinity binding approach with the binder engineered into the Fc domain of therapeutic mAbs (Kariolis et al., 2020; Arguello et al., 2022). This optimization also informed the design of NPs decorated with TfR mAbs for brain penetration—by simply using optimized TfR mAb fragments on the surface of NPs (Johnsen et al., 2018) but also, in a manner distinct to large particles, fine-tuning the density of those fragments on the particle surface, thus modifying the particle’s avidity (Wiley et al., 2013; Johnsen et al., 2019).
LRP-1 is another well-explored BCEC target. A family of Kunitz domain-derived peptides named Angiopeps, particularly Angiopep-2, has been shown to cross BCEC (Demeule et al., 2008a) through an LRP-1–mediated mechanism (Demeule et al., 2008b; Bertrand et al., 2010). In (Sakamoto et al., 2017), an Angiopeps family-unrelated peptide identified in phage display and named L57 was shown to bind LRP-1 and accumulate in the brain after an IV injection. LRP-1 has been used to enhance NP-mediated transport of biologics across the BBB (Ke et al., 2009; Tian et al., 2015), with one study optimizing the LRP1-mediated transport of NPs by tuning the avidity to the receptor (Tian et al., 2020), which parallels the approach taken by Wiley et al. (2013) for TfR-mediated NP transport. Additionally, ANG1005, comprising three paclitaxel molecules covalently linked to Angiopep-2, has been used in a clinical trial for the treatment of recurrent brain metastases from breast cancer (Kumthekar et al., 2020).
The key question with LRP-1-mediated transport is probably whether LRP-1 exists in in vivo BCEC in the first place. Single-cell transcriptomics data suggest that it may not (Yang et al., 2022; Chen et al., 2020). Proteomics data may seem more encouraging, with several reports indicating LRP-1 presence (Uchida et al., 2011; Al Feteisi et al., 2018; Al-Majdoub et al., 2019; Campeau et al., 2020), although, notably Zuchero et al. (2016) did not find LRP-1 in a proteomic characterization of BCEC after CD31+/CD45− fluorescence-activated cell sorting. One caveat with proteomics studies is the contamination with cells of the brain parenchyma (Al Feteisi et al., 2018; Al-Majdoub et al., 2019). The presence of e.g., aquaporin-4 or glial fibrillary acidic protein could be a clear sign of this contamination, and these markers are in fact present in untargeted proteomic studies (Al Feteisi et al., 2018; Campeau et al., 2020). The fact that LRP-1 is highly expressed in parenchymal cells (Zhang et al., 2014; Munji et al., 2019) further complicates things—one would expect contamination to be less of an issue for proteins with negligible gene expression on those cells, but in the case of LRP-1, their contribution may well be substantial. In addition to specific cell sorting, laser-capture microdissection (LCM) with sufficiently small sections could shed light here since sections with no trace of exclusive parenchymal cell markers and with a strong presence of e.g., claudin-5 could be reasonably expected to include only whole BCEC or their lumen-facing fractions, and in those sections, the presence of LRP-1 or lack thereof would be definitive. Spatially-resolved proteome of the brain cortex was studied using LCM with small sections in Zhu et al. (2018). However, while the proteome of 100 µm and especially 200 µm sections in Zhu et al. (2018) appears sufficiently rich within the coverage limit of the study, it also shows a strong presence of parenchymal markers, while the proteome of the smallest sections (50 µm) does not seem to provide a conclusive answer, especially given the small sample size. Ultimately, the question of a target’s presence and level on BCEC, whether for LRP-1 or in other cases where a target’s expression on BCEC is uncertain, will likely be addressed by single-cell proteomics, assuming sufficient coverage.
Combinatorial targeting can, in principle, increase the probability of circulating biologics’ interaction with BCEC. This approach is likely easier to implement for larger vehicles such as NPs and EVs, than for mAbs and especially for peptides. Dual and even triple targeting to BCEC has been shown to increase the transport of liposomes and niosomes across the BBB (Markoutsa et al., 2014; Mészáros et al., 2018; Veszelka et al., 2021). One relevant aspect here could be the purpose of the second—or subsequent—moieties. If all shuttle moieties are present simply to increase the specificity of interaction with BCEC or, alternatively, if all are optimized for transporting the vehicle across BCEC, this may be moot. However, if all but one of them are present to increase the retention on the surface of BCEC, increasing the probability that the primary, optimized moiety will interact with its intended target, then, conceivably, this approach could benefit from targeting molecules in close proximity to each other. As an example, solute carrier family 7 member 5 (SLC7A5) forms a heterodimer with the CD98 heavy chain (CD98hc) (Lee et al., 2019); this and similar combinations of spatially close targets could potentially be exploited for a greater efficiency of interaction with BCEC.
Overall, the progress in single-cell studies and the availability of proteomics data have fostered the discovery of new potential targets at BCEC (Shusta 2005; Mäger et al., 2017). In a recent study, Cegarra et al. (2022) explored integral membrane protein 2A (ITM2A) as a potential shuttle target at the BBB identified by the Collaboration on the Optimization of Macromolecular Pharmaceutical Access to Cellular Targets consortium; while the study results are not encouraging for the use of ITM2A, it is likely that large-scale omics-based characterization efforts such as those undertaken by the Human Cell Atlas (Regev et al., 2017; Eraslan et al., 2022) will be used to explore new targets allowing improved transport across the BBB.
There are several considerations affecting biologics, some more relevant to certain classes. The transport of naked oligonucleotides across the BBB is negligible, disfavored not only by a poor half-life but also by electrostatic repulsion by the negatively charged BCEC glycocalyx abundant in sialic acid residues (Vorbrodt 1989). mAbs benefit from neonatal Fc receptor-mediated recycling, increasing their circulation time in comparison to other types of biologics (Ghetie et al., 1996). While pharmacokinetic considerations are out of the paper’s scope, one key factor linking them to cellular interactions is whether the reduced affinity to BCEC targets, which potentially increases dissociation from the target and transport into brain parenchyma, is of greatest benefit to mAbs precisely because of the longer half-life giving them more time to interact with BCEC specifically, thus compensating for the reduced binding to BCEC targets. This would clearly affect e.g., peptides and nanocarriers decorated with targeted ligands. However, a study of gold NPs decorated with low-affinity or monovalent TfR-targeted mAbs indicates that this benefit extends to NPs as well, despite their shorter half-life (Johnsen et al., 2018). Furthermore, the larger size of nanocarriers may adversely affect the extent to which they get across the BBB, penetrate the narrow-spaced barrier formed by astrocytic endfeet beyond the BBB (Kucharz et al., 2021), and distribute in the brain parenchyma with the extracellular space pore size estimated at ∼40–65 nm (Thorne and Nicholson 2006). This can be offset by the substantial loading capacity of nanocarriers, allowing the release of many payload molecules for a single transcytosis event. The density of the targeting ligands on the surface of nanocarriers further allows transport optimization (Wiley et al., 2013; Johnsen et al., 2019). Protein corona formation is another factor specific to nanocarriers: in addition to modifying the intrinsic properties of nanocarriers in the bloodstream, it can also mask targeting ligands, thus precluding their specific interaction with BCEC (Salvati et al., 2013; Xiao et al., 2021). A way to address this limitation by employing a defined, pre-formed corona has been proposed in Kaleta et al. (2020).
With TfR targeting, one attractive rationale has been that targeted biologics would simply follow the presumed route taken by the native ligand (Bien-Ly et al., 2014; Niewoehner et al., 2014). In this scenario, a biologic-target complex would proceed from the luminal to the abluminal side of BEC to release the payload—as, presumably, would Tf with TfR. This reasoning could work for TfR and several other receptors such as LDLR, known or thought to carry native ligands across the BBB. It is less clear for other targets. For instance, Zuchero et al. (2016) identified and explored basigin, glucose transporter 1 (GLUT1), and CD98hc as promising targets for mAb transport, demonstrating brain accumulation and the therapeutic effect of a CD98hc/BACE1 bispecific. Leaving basigin with its receptor and chaperone functions aside (for its further exploration as a BCEC target see (Christensen et al., 2021)), one cannot but wonder what would be the mechanism of trans-BBB transport in the case of mAbs or other biologics targeting CD98hc and GLUT1 (SLC3A2 and SLC2A1, respectively). The native transport mechanism invoked by solute carriers (SLCs) is facilitated diffusion, i.e., they engage no endocytic machinery behind the luminal surface of BCEC as far as their postulated function is concerned. Additionally, the size of most, if not all biologics is beyond that of molecules natively transported by SLCs. As a result, it may not seem likely that biologics engage the native transport mechanism of SLCs. However, if one excludes this native mechanism, it is conceivable that biologics’ engagement with CD98hc and GLUT1 itself induces endocytosis after the binding event or simply exploits the constitutive recycling process of SLCs, in which case protein turnover becomes highly relevant. Then, the question would be how the biologic would get to the abluminal side. One possible explanation is that the complex, or only the biologic that is part of it, is artificially redirected toward exocytosis, owing to the biologic’s properties that would enable such redirection.
Notably, in the case of TfR targeting, it has been postulated that a suboptimal format can fully or partially re-route a biologic toward lysosomal degradation in contrast to Tf and TfR that presumably reach the abluminal side natively (Pardridge et al., 1991; Bien-Ly et al., 2014; Niewoehner et al., 2014). One then has to wonder: why, conversely, would an optimal format not be able to re-route a biologic, with or without the target, to the abluminal side, even if the native ligand and/or target molecule do not normally reach it? Taking that reasoning further and applying it to the TfR-mediated transport, the fundamental question would be: does TfR get across the BCEC at all, in any scenario? This discussion would have two distinctly separate components: the fate of the native TfR–Tf complex, and the fate of TfR in a complex with a biologic that crosses the BBB. Both components are out of scope for this paper; for an overview of the former, focusing on the insights from the iron metabolism field, see (Skjørringe T et al., 2015; Duck and Connor 2016).
All these considerations may be more fundamental than practical in nature; after all, targets thought to be expressed on and, better yet, specific to BCEC have been exploited for brain delivery regardless of the tentative underlying mechanism. However, they may inform the strategy behind choosing targets and optimizing interactions with them. In this scenario, the early endosome likely becomes the nexus defining the fate of a biologic inside BCEC, and the desired outcome, in most cases, would be to route the biologic from that nexus toward the abluminal side. Ultimately, one key factor governing this (re)direction is likely the entirety of the properties characterizing the target–biologic complex or the biologic alone, depending on what is destined for exocytosis. Intuitively, key properties can include conformation and size, the latter being far easier to optimize than the former. In addition to size, optimization could also be focused on the probability of the biologic’s dissociation from the target. For instance, one could argue that the efforts to optimize TfR-mediated transport, described above, converge on two themes: the size of the biologic–TfR complex and the probability of the biologic’s dissociation from TfR along the endocytic pathway or on the abluminal side of BCEC. The monovalent binding mode presumably improves the transport by eliminating crosslinking of TfR by two arms of the mAb (Niewoehner et al., 2014; Hultqvist et al., 2017). The actual observation is that the cross-linked complex is prone to lysosomal routing and eventual degradation. The question, however, is why; and one possible explanation is that the cross-linked complex is too large for any other intracellular fate, whether recycling to the luminal membrane or routing to the abluminal side. The same considerations are clearly applicable to bispecific mAbs. In a different vein, changing antibody affinity and making its interaction with TfR sensitive to the local environment (such as low pH in the endosomal compartment) would increase the chances of the biologic’s dissociation from TfR—thus, in another way, reducing the size of the entity routed for exocytosis—although it can also decrease the probability of bivalent binding by reducing the chance that both arms of the mAb would bind TfR at the same time. All these considerations could be amended for other formats of biologics, but also for targets. For instance, would reduced affinity be relevant for brain-targeted peptides whose binding mode is intrinsically monovalent? Could crosslinking avoidance be less relevant for proteins with lower densities on the plasma membrane? While modeling can probably help with these or other similar questions, in the end, they can only be answered experimentally.
I confirm being the sole contributor of this work and have approved it for publication.
HB is employed by Roche Global IT Solution Centre.
All claims expressed in this article are solely those of the authors and do not necessarily represent those of their affiliated organizations, or those of the publisher, the editors and the reviewers. Any product that may be evaluated in this article, or claim that may be made by its manufacturer, is not guaranteed or endorsed by the publisher.
I am grateful to all researchers whose studies inspired me to reflect on the BBB transport of biologics.
Akache, B., Grimm, D., Pandey, K., Yant, S. R., Xu, H., and Kay, M. A. (2006). The 37/67-kilodalton Laminin Receptor Is a Receptor for Adeno-Associated Virus Serotypes 8, 2, 3, and 9. J. Virol. 80 (19), 9831–9836. doi:10.1128/jvi.00878-06
Akinc, A., Querbes, W., De, S., Qin, J., Frank-Kamenetsky, M., Jayaprakash, K. N., et al. (2010). Targeted Delivery of RNAi Therapeutics with Endogenous and Exogenous Ligand-Based Mechanisms. Mol. Ther. 18 (7), 1357–1364. doi:10.1038/mt.2010.85
Akinc, A., Zumbuehl, A., Goldberg, M., Leshchiner, E. S., Busini, V., Hossain, N., et al. (2008). A Combinatorial Library of Lipid-like Materials for Delivery of RNAi Therapeutics. Nat. Biotechnol. 26 (5), 561–569. doi:10.1038/nbt1402
Al Feteisi, H., Al-Majdoub, Z. M., Achour, B., Couto, N., Rostami-Hodjegan, A., and Barber, J. (2018). Identification and Quantification of Blood-Brain Barrier Transporters in Isolated Rat Brain Microvessels. J. Neurochem. 146 (6), 670–685. doi:10.1111/jnc.14446
Al-Majdoub, Z. M., Al Feteisi, H., Achour, B., Warwood, S., Neuhoff, S., Rostami-Hodjegan, A., et al. (2019). Proteomic Quantification of Human Blood-Brain Barrier SLC and ABC Transporters in Healthy Individuals and Dementia Patients. Mol. Pharm. 16 (3), 1220–1233. doi:10.1021/acs.molpharmaceut.8b01189
Arguello, A., Mahon, C. S., Calvert, M. E. K., Chan, D., Dugas, J. C., Pizzo, M. E., et al. (2022). Molecular Architecture Determines Brain Delivery of a Transferrin Receptor-Targeted Lysosomal Enzyme. J. Exp. Med. 219 (3), e20211057. doi:10.1084/jem.20211057
Atwal, J. K., Chen, Y., Chiu, C., Mortensen, D. L., Meilandt, W. J., Liu, Y., et al. (2011). A Therapeutic Antibody Targeting BACE1 Inhibits Amyloid-β Production In Vivo. Sci. Transl. Med. 3 (84), 84ra43. doi:10.1126/scitranslmed.3002254
Banks, W. A., Sharma, P., Bullock, K. M., Hansen, K. M., Ludwig, N., and Whiteside, T. L. (2020). Transport of Extracellular Vesicles across the Blood-Brain Barrier: Brain Pharmacokinetics and Effects of Inflammation. Ijms 21 (12), 4407. doi:10.3390/ijms21124407
Bard, F., Cannon, C., Barbour, R., Burke, R.-L., Games, D., Grajeda, H., et al. (2000). Peripherally Administered Antibodies against Amyloid β-peptide Enter the Central Nervous System and Reduce Pathology in a Mouse Model of Alzheimer Disease. Nat. Med. 6 (8), 916–919. doi:10.1038/78682
Bertrand, Y., Currie, J.-C., Demeule, M., Régina, A., Ché, C., Abulrob, A., et al. (2010). Transport Characteristics of a Novel Peptide Platform for CNS Therapeutics. J. Cell. Mol. Med. 14 (12), 2827–2839. doi:10.1111/j.1582-4934.2009.00930.x
Bien-Ly, N., Yu, Y. J., Bumbaca, D., Elstrott, J., Boswell, C. A., Zhang, Y., et al. (2014). Transferrin Receptor (TfR) Trafficking Determines Brain Uptake of TfR Antibody Affinity Variants. J. Exp. Med. 211 (2), 233–244. doi:10.1084/jem.20131660
Boado, R. J., Zhou, Q.-H., Lu, J. Z., Hui, E. K.-W., and Pardridge, W. M. (2010). Pharmacokinetics and Brain Uptake of a Genetically Engineered Bifunctional Fusion Antibody Targeting the Mouse Transferrin Receptor. Mol. Pharm. 7 (1), 237–244. doi:10.1021/mp900235k
Bryant, D. H., Bashir, A., Sinai, S., Jain, N. K., Ogden, P. J., Riley, P. F., et al. (2021). Deep Diversification of an AAV Capsid Protein by Machine Learning. Nat. Biotechnol. 39 (6), 691–696. doi:10.1038/s41587-020-00793-4
Campeau, A., Mills, R. H., Blanchette, M., Bajc, K., Malfavon, M., Munji, R. N., et al. (2020). Multidimensional Proteome Profiling of Blood-Brain Barrier Perturbation by Group B Streptococcus. mSystems 5 (4), e00368–00320. doi:10.1128/mSystems.00368-20
Cegarra, C., Chaves, C., Déon, C., Do, T. M., Dumas, B., Frenzel, A., et al. (2022). Exploring ITM2A as a New Potential Target for Brain Delivery. Fluids Barriers CNS 19 (1), 25. doi:10.1186/s12987-022-00321-3
Chen, M. B., Yang, A. C., Yousef, H., Lee, D., Chen, W., Schaum, N., et al. (2020). Brain Endothelial Cells Are Exquisite Sensors of Age-Related Circulatory Cues. Cell. Rep. 30 (13), 4418–4432. e4414. doi:10.1016/j.celrep.2020.03.012
Christensen, S. C., Hudecz, D., Jensen, A., Christensen, S., and Nielsen, M. S. (2021). Basigin Antibodies with Capacity for Drug Delivery across Brain Endothelial Cells. Mol. Neurobiol. 58 (9), 4392–4403. doi:10.1007/s12035-021-02421-x
Chuapoco, M. R., Flytzanis, N. C., Goeden, N., Octeau, J. C., Roxas, K. M., Chan, K. Y., et al. (2022). Intravenous Gene Transfer throughout the Brain of Infant Old World Primates Using AAV. bioRxiv. doi:10.1101/2022.01.08.475342
Couch, J. A., Yu, Y. J., Zhang, Y., Tarrant, J. M., Fuji, R. N., Meilandt, W. J., et al. (2013). Addressing Safety Liabilities of TfR Bispecific Antibodies that Cross the Blood-Brain Barrier. Sci. Transl. Med. 5 (183), 183ra57–12. doi:10.1126/scitranslmed.3005338
Crook, Z. R., Girard, E., Sevilla, G. P., Merrill, M., Friend, D., Rupert, P. B., et al. (2020). A TfR-Binding Cystine-Dense Peptide Promotes Blood-Brain Barrier Penetration of Bioactive Molecules. J. Mol. Biol. 432 (14), 3989–4009. doi:10.1016/j.jmb.2020.04.002
Dahlman, J. E., Kauffman, K. J., Xing, Y., Shaw, T. E., Mir, F. F., Dlott, C. C., et al. (2017). Barcoded Nanoparticles for High Throughput In Vivo Discovery of Targeted Therapeutics. Proc. Natl. Acad. Sci. U.S.A. 114 (8), 2060–2065. doi:10.1073/pnas.1620874114
Demeule, M., Currie, J.-C., Bertrand, Y., Ché, C., Nguyen, T., Régina, A., et al. (2008a). Involvement of the Low-Density Lipoprotein Receptor-Related Protein in the Transcytosis of the Brain Delivery Vector Angiopep-2. J. Neurochem. 106 (4), 1534–1544. doi:10.1111/j.1471-4159.2008.05492.x
Demeule, M., Régina, A., Ché, C., Poirier, J., Nguyen, T., Gabathuler, R., et al. (2008b). Identification and Design of Peptides as a New Drug Delivery System for the Brain. J. Pharmacol. Exp. Ther. 324 (3), 1064–1072. doi:10.1124/jpet.107.131318
Deverman, B. E., Pravdo, P. L., Simpson, B. P., Kumar, S. R., Chan, K. Y., Banerjee, A., et al. (2016). Cre-dependent Selection Yields AAV Variants for Widespread Gene Transfer to the Adult Brain. Nat. Biotechnol. 34 (2), 204–209. doi:10.1038/nbt.3440
Dilliard, S. A., Cheng, Q., and Siegwart, D. J. (2021). On the Mechanism of Tissue-specific mRNA Delivery by Selective Organ Targeting Nanoparticles. Proc. Natl. Acad. Sci. U. S. A. 118 (52), e2109256118. doi:10.1073/pnas.2109256118
Duck, K. A., and Connor, J. R. (2016). Iron Uptake and Transport across Physiological Barriers. Biometals 29 (4), 573–591. doi:10.1007/s10534-016-9952-2
Eraslan, G., Drokhlyansky, E., Anand, S., Fiskin, E., Subramanian, A., Slyper, M., et al. (2022). Single-nucleus Cross-Tissue Molecular Reference Maps toward Understanding Disease Gene Function. Science 376 (6594), eabl4290. doi:10.1126/science.abl4290
Foust, K. D., Nurre, E., Montgomery, C. L., Hernandez, A., Chan, C. M., and Kaspar, B. K. (2009). Intravascular AAV9 Preferentially Targets Neonatal Neurons and Adult Astrocytes. Nat. Biotechnol. 27 (1), 59–65. doi:10.1038/nbt.1515
Friden, P. M., Walus, L. R., Musso, G. F., Taylor, M. A., Malfroy, B., and Starzyk, R. M. (1991). Anti-transferrin Receptor Antibody and Antibody-Drug Conjugates Cross the Blood-Brain Barrier. Proc. Natl. Acad. Sci. U.S.A. 88 (11), 4771–4775. doi:10.1073/pnas.88.11.4771
Friden, P. M., Walus, L. R., Watson, P., Doctrow, S. R., Kozarich, J. W., Bäckman, C., et al. (1993). Blood-brain Barrier Penetration and In Vivo Activity of an NGF Conjugate. Science 259 (5093), 373–377. doi:10.1126/science.8420006
Ghetie, V., Hubbard, J. G., Kim, J.-K., Tsen, M.-F., Lee, Y., and Ward, E. S. (1996). Abnormally Short Serum Half-Lives of IgG in β2-microglobulin-deficient Mice. Eur. J. Immunol. 26 (3), 690–696. doi:10.1002/eji.1830260327
Giugliani, R., Martins, A. M., Okuyama, T., Eto, Y., Sakai, N., Nakamura, K., et al. (2021). Enzyme Replacement Therapy with Pabinafusp Alfa for Neuronopathic Mucopolysaccharidosis II: An Integrated Analysis of Preclinical and Clinical Data. Int. J. Mol. Sci. 22 (20), 10938. doi:10.3390/ijms222010938
Goertsen, D., Flytzanis, N. C., Goeden, N., Chuapoco, M. R., Cummins, A., Chen, Y., et al. (2022). AAV Capsid Variants with Brain-wide Transgene Expression and Decreased Liver Targeting after Intravenous Delivery in Mouse and Marmoset. Nat. Neurosci. 25 (1), 106–115. doi:10.1038/s41593-021-00969-4
Hanlon, K. S., Meltzer, J. C., Buzhdygan, T., Cheng, M. J., Sena-Esteves, M., Bennett, R. E., et al. (2019). Selection of an Efficient AAV Vector for Robust CNS Transgene Expression. Mol. Ther. - Methods & Clin. Dev. 15, 320–332. doi:10.1016/j.omtm.2019.10.007
Haqqani, A. S., Delaney, C. E., Tremblay, T.-L., Sodja, C., Sandhu, J. K., and Stanimirovic, D. B. (2013). Method for Isolation and Molecular Characterization of Extracellular Microvesicles Released from Brain Endothelial Cells. Fluids Barriers CNS 10 (1), 4. doi:10.1186/2045-8118-10-4
Hordeaux, J., Wang, Q., Katz, N., Buza, E. L., Bell, P., and Wilson, J. M. (2018). The Neurotropic Properties of AAV-PHP.B Are Limited to C57BL/6J Mice. Mol. Ther. 26 (3), 664–668. doi:10.1016/j.ymthe.2018.01.018
Huang, Q., Chan, K. Y., Tobey, I. G., Chan, Y. A., Poterba, T., Boutros, C. L., et al. (2019). Delivering Genes across the Blood-Brain Barrier: LY6A, a Novel Cellular Receptor for AAV-PHP.B Capsids. PLoS One 14 (11), e0225206. doi:10.1371/journal.pone.0225206
Hultqvist, G., Syvänen, S., Fang, X. T., Lannfelt, L., and Sehlin, D. (2017). Bivalent Brain Shuttle Increases Antibody Uptake by Monovalent Binding to the Transferrin Receptor. Theranostics 7 (2), 308–318. doi:10.7150/thno.17155
Huwyler, J., Wu, D., and Pardridge, W. M. (1996). Brain Drug Delivery of Small Molecules Using Immunoliposomes. Proc. Natl. Acad. Sci. U.S.A. 93 (24), 14164–14169. doi:10.1073/pnas.93.24.14164
Jandl, J. H., and Katz, J. H. (1963). The Plasma-To-Cell Cycle of Transferrin*. J. Clin. Invest. 42 (3), 314–326. doi:10.1172/jci104718
Jefferies, W. A., Brandon, M. R., Hunt, S. V., Williams, A. F., Gatter, K. C., and Mason, D. Y. (1984). Transferrin Receptor on Endothelium of Brain Capillaries. Nature 312 (5990), 162–163. doi:10.1038/312162a0
Johnsen, K. B., Bak, M., Kempen, P. J., Melander, F., Burkhart, A., Thomsen, M. S., et al. (2018). Antibody Affinity and Valency Impact Brain Uptake of Transferrin Receptor-Targeted Gold Nanoparticles. Theranostics 8 (12), 3416–3436. doi:10.7150/thno.25228
Johnsen, K. B., Bak, M., Melander, F., Thomsen, M. S., Burkhart, A., Kempen, P. J., et al. (2019). Modulating the Antibody Density Changes the Uptake and Transport at the Blood-Brain Barrier of Both Transferrin Receptor-Targeted Gold Nanoparticles and Liposomal Cargo. J. Control. Release 295, 237–249. doi:10.1016/j.jconrel.2019.01.005
Kaleta, L., Meyer, A., Ried, C., Michael, R., Schäker-theobald, K., Talmon, S., et al. (2020). Albumin-modified Nanoparticles Carrying a Targeting Ligand. Google Patents.
Kariolis, M. S., Wells, R. C., Getz, J. A., Kwan, W., Mahon, C. S., Tong, R., et al. (2020). Brain Delivery of Therapeutic Proteins Using an Fc Fragment Blood-Brain Barrier Transport Vehicle in Mice and Monkeys. Sci. Transl. Med. 12 (545), eaay1359. doi:10.1126/scitranslmed.aay1359
Ke, W., Shao, K., Huang, R., Han, L., Liu, Y., Li, J., et al. (2009). Gene Delivery Targeted to the Brain Using an Angiopep-Conjugated Polyethyleneglycol-Modified Polyamidoamine Dendrimer. Biomaterials 30 (36), 6976–6985. doi:10.1016/j.biomaterials.2009.08.049
Kim, G., Kim, M., Lee, Y., Byun, J. W., Hwang, D. W., and Lee, M. (2020). Systemic Delivery of microRNA-21 Antisense Oligonucleotides to the Brain Using T7-Peptide Decorated Exosomes. J. Control. Release 317, 273–281. doi:10.1016/j.jconrel.2019.11.009
Kreuter, J., Shamenkov, D., Petrov, V., Ramge, P., Cychutek, K., Koch-Brandt, C., et al. (2002). Apolipoprotein-mediated Transport of Nanoparticle-Bound Drugs across the Blood-Brain Barrier. J. Drug Target. 10 (4), 317–325. doi:10.1080/10611860290031877
Kucharz, K., Kristensen, K., Johnsen, K. B., Lund, M. A., Lønstrup, M., Moos, T., et al. (2021). Post-capillary Venules Are the Key Locus for Transcytosis-Mediated Brain Delivery of Therapeutic Nanoparticles. Nat. Commun. 12 (1), 4121. doi:10.1038/s41467-021-24323-1
Kumthekar, P., Tang, S.-C., Brenner, A. J., Kesari, S., Piccioni, D. E., Anders, C., et al. (2020). ANG1005, a Brain-Penetrating Peptide-Drug Conjugate, Shows Activity in Patients with Breast Cancer with Leptomeningeal Carcinomatosis and Recurrent Brain Metastases. Clin. Cancer Res. 26 (12), 2789–2799. doi:10.1158/1078-0432.ccr-19-3258
Lajoie, J. M., and Shusta, E. V. (2015). Targeting Receptor-Mediated Transport for Delivery of Biologics across the Blood-Brain Barrier. Annu. Rev. Pharmacol. Toxicol. 55 (1), 613–631. doi:10.1146/annurev-pharmtox-010814-124852
Lee, Y., Wiriyasermkul, P., Jin, C., Quan, L., Ohgaki, R., Okuda, S., et al. (2019). Cryo-EM Structure of the Human L-type Amino Acid Transporter 1 in Complex with Glycoprotein CD98hc. Nat. Struct. Mol. Biol. 26 (6), 510–517. doi:10.1038/s41594-019-0237-7
Mabuchi, T., Lucero, J., Feng, A., Koziol, J. A., and del Zoppo, G. J. (2005). Focal Cerebral Ischemia Preferentially Affects Neurons Distant from Their Neighboring Microvessels. J. Cereb. Blood Flow. Metab. 25 (2), 257–266. doi:10.1038/sj.jcbfm.9600027
Mäger, I., Meyer, A. H., Li, J., Lenter, M., Hildebrandt, T., Leparc, G., et al. (2017). Targeting Blood-Brain-Barrier Transcytosis – Perspectives for Drug Delivery. Neuropharmacology 120, 4–7. doi:10.1016/j.neuropharm.2016.08.025
Markoutsa, E., Papadia, K., Giannou, A. D., Spella, M., Cagnotto, A., Salmona, M., et al. (2014). Mono and Dually Decorated Nanoliposomes for Brain Targeting, In Vitro and In Vivo Studies. Pharm. Res. 31 (5), 1275–1289. doi:10.1007/s11095-013-1249-3
Mathiesen, S. N., Lock, J. L., Schoderboeck, L., Abraham, W. C., and Hughes, S. M. (2020). CNS Transduction Benefits of AAV-PHP.eB over AAV9 Are Dependent on Administration Route and Mouse Strain. Mol. Ther. - Methods & Clin. Dev. 19, 447–458. doi:10.1016/j.omtm.2020.10.011
Mészáros, M., Porkoláb, G., Kiss, L., Pilbat, A. M., Kóta, Z., Kupihár, Z., et al. (2018). Niosomes Decorated with Dual Ligands Targeting Brain Endothelial Transporters Increase Cargo Penetration across the Blood-Brain Barrier. Eur. J. Pharm. Sci. 123, 228–240. doi:10.1016/j.ejps.2018.07.042
Moos, T., and Morgan, E. H. (2001). Restricted Transport of Anti-transferrin Receptor Antibody (OX26) through the Blood-Brain Barrier in the Rat. J. Neurochem. 79 (1), 119–129. doi:10.1046/j.1471-4159.2001.00541.x
Munji, R. N., Soung, A. L., Weiner, G. A., Sohet, F., Semple, B. D., Trivedi, A., et al. (2019). Profiling the Mouse Brain Endothelial Transcriptome in Health and Disease Models Reveals a Core Blood-Brain Barrier Dysfunction Module. Nat. Neurosci. 22 (11), 1892–1902. doi:10.1038/s41593-019-0497-x
Niewoehner, J., Bohrmann, B., Collin, L., Urich, E., Sade, H., Maier, P., et al. (2014). Increased Brain Penetration and Potency of a Therapeutic Antibody Using a Monovalent Molecular Shuttle. Neuron 81 (1), 49–60. doi:10.1016/j.neuron.2013.10.061
Nonnenmacher, M., Wang, W., Child, M. A., Ren, X.-Q., Huang, C., Ren, A. Z., et al. (2021). Rapid Evolution of Blood-Brain-Barrier-Penetrating AAV Capsids by RNA-Driven Biopanning. Mol. Ther. - Methods & Clin. Dev. 20, 366–378. doi:10.1016/j.omtm.2020.12.006
Ogden, P. J., Kelsic, E. D., Sinai, S., and Church, G. M. (2019). Comprehensive AAV Capsid Fitness Landscape Reveals a Viral Gene and Enables Machine-Guided Design. Science 366 (6469), 1139–1143. doi:10.1126/science.aaw2900
Pardridge, W. M., Buciak, J. L., and Friden, P. M. (1991). Selective Transport of an Anti-Transferrin Receptor Antibody Through the Blood-Brain Barrier In Vivo. Journal of Pharmacology and Experimental Therapeutics 259 (1), 66–70.
Pillay, S., Zou, W., Cheng, F., Puschnik, A. S., Meyer, N. L., Ganaie, S. S., et al. (2017). Adeno-associated Virus (AAV) Serotypes Have Distinctive Interactions with Domains of the Cellular AAV Receptor. J. Virol. 91 (18), e00391–17. doi:10.1128/JVI.00391-17
Qu, M., Lin, Q., Huang, L., Fu, Y., Wang, L., He, S., et al. (2018). Dopamine-loaded Blood Exosomes Targeted to Brain for Better Treatment of Parkinson's Disease. J. Control. Release 287, 156–166. doi:10.1016/j.jconrel.2018.08.035
Regev, A., Teichmann, S. A., Lander, E. S., Amit, I., Benoist, C., Birney, E., et al. (2017). The Human Cell Atlas. Elife 6, e27041. doi:10.7554/elife.27041
Sade, H., Baumgartner, C., Hugenmatter, A., Moessner, E., Freskgård, P.-O., and Niewoehner, J. (2014). A Human Blood-Brain Barrier Transcytosis Assay Reveals Antibody Transcytosis Influenced by pH-dependent Receptor Binding. PLoS One 9 (4), e96340. doi:10.1371/journal.pone.0096340
Sakamoto, K., Shinohara, T., Adachi, Y., Asami, T., and Ohtaki, T. (2017). A Novel LRP1-Binding Peptide L57 that Crosses the Blood Brain Barrier. Biochem. Biophysics Rep. 12, 135–139. doi:10.1016/j.bbrep.2017.07.003
Salvati, A., Pitek, A. S., Monopoli, M. P., Prapainop, K., Bombelli, F. B., Hristov, D. R., et al. (2013). Transferrin-functionalized Nanoparticles Lose Their Targeting Capabilities when a Biomolecule Corona Adsorbs on the Surface. Nat. Nanotech 8 (2), 137–143. doi:10.1038/nnano.2012.237
Schlageter, K. E., Molnar, P., Lapin, G. D., and Groothuis, D. R. (1999). Microvessel Organization and Structure in Experimental Brain Tumors: Microvessel Populations with Distinctive Structural and Functional Properties. Microvasc. Res. 58 (3), 312–328. doi:10.1006/mvre.1999.2188
Shen, S., Bryant, K. D., Brown, S. M., Randell, S. H., and Asokan, A. (2011). Terminal N-Linked Galactose Is the Primary Receptor for Adeno-Associated Virus 9. J. Biol. Chem. 286 (15), 13532–13540. doi:10.1074/jbc.m110.210922
Shusta, E. V. (2005). Blood-brain Barrier Genomics, Proteomics, and New Transporter Discovery. Neurotherapeutics 2 (1), 151–161. doi:10.1602/neurorx.2.1.151
Skjørringe, T., Burkhart, A., Johnsen, K. B., and Moos, T. (2015). Divalent Metal Transporter 1 (DMT1) in the Brain: Implications for a Role in Iron Transport at the blood-Brain Barrier, and Neuronal and Glial Pathology. Front. Mol. Neurosci. 8, 19. doi:10.3389/fnmol.2015.00019
Stocki, P., Szary, J., Rasmussen, C. L. M., Demydchuk, M., Northall, L., Logan, D. B., et al. (2021). Blood-brain Barrier Transport Using a High Affinity, Brain-Selective VNAR Antibody Targeting Transferrin Receptor 1. Faseb J. 35 (2), e21172. doi:10.1096/fj.202001787r
Sun, J., Boado, R. J., Pardridge, W. M., and Sumbria, R. K. (2019). Plasma Pharmacokinetics of High-Affinity Transferrin Receptor Antibody-Erythropoietin Fusion Protein Is a Function of Effector Attenuation in Mice. Mol. Pharm. 16 (8), 3534–3543. doi:10.1021/acs.molpharmaceut.9b00369
Terstappen, G. C., Meyer, A. H., Bell, R. D., and Zhang, W. (2021). Strategies for Delivering Therapeutics across the Blood-Brain Barrier. Nat. Rev. Drug Discov. 20 (5), 362–383. doi:10.1038/s41573-021-00139-y
Thorne, R. G., and Nicholson, C. (2006). In Vivo diffusion Analysis with Quantum Dots and Dextrans Predicts the Width of Brain Extracellular Space. Proc. Natl. Acad. Sci. U.S.A. 103 (14), 5567–5572. doi:10.1073/pnas.0509425103
Tian, X., Leite, D. M., Scarpa, E., Nyberg, S., Fullstone, G., Forth, J., et al. (2020). On the Shuttling across the Blood-Brain Barrier via Tubule Formation: Mechanism and Cargo Avidity Bias. Sci. Adv. 6 (48), eabc4397. doi:10.1126/sciadv.abc4397
Tian, X., Nyberg, S., Sharp, P. S., Daneshpour, N., Armes, S. P., Berwick, J., et al. (2015). LRP-1-mediated Intracellular Antibody Delivery to the Central Nervous System. Sci. Rep. 5, 11990. doi:10.1038/srep11990
Uchida, Y., Ohtsuki, S., Katsukura, Y., Ikeda, C., Suzuki, T., Kamiie, J., et al. (2011). Quantitative Targeted Absolute Proteomics of Human Blood-Brain Barrier Transporters and Receptors. J. Neurochem. 117 (2), 333–345. doi:10.1111/j.1471-4159.2011.07208.x
Veszelka, S., Meszaros, M., Porkolab, G., Szecsko, A., Kondor, N., Ferenc, G., et al. (2021). A Triple Combination of Targeting Ligands Increases the Penetration of Nanoparticles across a Blood-Brain Barrier Culture Model. Pharmaceutics 14 (1), 86. doi:10.3390/pharmaceutics14010086
Vorbrodt, A. W. (1989). Ultracytochemical Characterization of Anionic Sites in the Wall of Brain Capillaries. J. Neurocytol. 18 (3), 359–368. doi:10.1007/bf01190839
Whitehead, K. A., Dorkin, J. R., Vegas, A. J., Chang, P. H., Veiseh, O., Matthews, J., et al. (2014). Degradable Lipid Nanoparticles with Predictable In Vivo siRNA Delivery Activity. Nat. Commun. 5 (1), 4277. doi:10.1038/ncomms5277
Wiklander, O. P. B., Nordin, J. Z., O'Loughlin, A., Gustafsson, Y., Corso, G., Mäger, I., et al. (2015). Extracellular Vesicle In Vivo Biodistribution Is Determined by Cell Source, Route of Administration and Targeting. J. Extracell. Vesicles 4, 26316. doi:10.3402/jev.v4.26316
Wiley, D. T., Webster, P., Gale, A., and Davis, M. E. (2013). Transcytosis and Brain Uptake of Transferrin-Containing Nanoparticles by Tuning Avidity to Transferrin Receptor. Proc. Natl. Acad. Sci. U.S.A. 110 (21), 8662–8667. doi:10.1073/pnas.1307152110
Wouters, Y., Jaspers, T., De Strooper, B., and Dewilde, M. (2020). Identification and In Vivo Characterization of a Brain-Penetrating Nanobody. Fluids Barriers CNS 17 (1), 62. doi:10.1186/s12987-020-00226-z
Wu, D., and Pardridge, W. M. (1998). Pharmacokinetics and Blood-Brain Barrier Transport of an Anti-transferrin Receptor Monoclonal Antibody (OX26) in Rats after Chronic Treatment with the Antibody. Drug Metab. Dispos. 26 (9), 937–939.
Xiao, W., Wang, Y., Zhang, H., Liu, Y., Xie, R., He, X., et al. (2021). The Protein Corona Hampers the Transcytosis of Transferrin-Modified Nanoparticles through Blood-Brain Barrier and Attenuates Their Targeting Ability to Brain Tumor. Biomaterials 274, 120888. doi:10.1016/j.biomaterials.2021.120888
Yang, A. C., Vest, R. T., Kern, F., Lee, D. P., Agam, M., Maat, C. A., et al. (2022). A Human Brain Vascular Atlas Reveals Diverse Mediators of Alzheimer’s Risk. Nature 603 (7903), 885–892. doi:10.1038/s41586-021-04369-3
Yu, Y. J., Zhang, Y., Kenrick, M., Hoyte, K., Luk, W., Lu, Y., et al. (2011). Boosting Brain Uptake of a Therapeutic Antibody by Reducing its Affinity for a Transcytosis Target. Sci. Transl. Med. 3 (84), 84ra44. doi:10.1126/scitranslmed.3002230
Zhang, X., He, T., Chai, Z., Samulski, R. J., and Li, C. (2018). Blood-brain Barrier Shuttle Peptides Enhance AAV Transduction in the Brain after Systemic Administration. Biomaterials 176, 71–83. doi:10.1016/j.biomaterials.2018.05.041
Zhang, Y., Chen, K., Sloan, S. A., Bennett, M. L., Scholze, A. R., O'Keeffe, S., et al. (2014). An RNA-Sequencing Transcriptome and Splicing Database of Glia, Neurons, and Vascular Cells of the Cerebral Cortex. J. Neurosci. 34 (36), 11929–11947. doi:10.1523/jneurosci.1860-14.2014
Zhu, Y., Dou, M., Piehowski, P. D., Liang, Y., Wang, F., Chu, R. K., et al. (2018). Spatially Resolved Proteome Mapping of Laser Capture Microdissected Tissue with Automated Sample Transfer to Nanodroplets. Mol. Cell. Proteomics 17 (9), 1864–1874. doi:10.1074/mcp.tir118.000686
Keywords: blood–brain barrier, biologics, endocytosis, receptor-mediated transcytosis, intracellular transport, targeted drug delivery
Citation: Baghirov H (2022) The Roads We Take: Cellular Targets and Pathways Leading Biologics Across the Blood–Brain Barrier. Front. Drug. Deliv. 2:946045. doi: 10.3389/fddev.2022.946045
Received: 17 May 2022; Accepted: 06 June 2022;
Published: 12 July 2022.
Edited by:
Michelle Ann Erickson, University of Washington, United StatesReviewed by:
Ibolya András, University of Miami, United StatesCopyright © 2022 Baghirov. This is an open-access article distributed under the terms of the Creative Commons Attribution License (CC BY). The use, distribution or reproduction in other forums is permitted, provided the original author(s) and the copyright owner(s) are credited and that the original publication in this journal is cited, in accordance with accepted academic practice. No use, distribution or reproduction is permitted which does not comply with these terms.
*Correspondence: Habib Baghirov, aGFiaWIuYmFnaGlyb3ZAcm9jaGUuY29t
Disclaimer: All claims expressed in this article are solely those of the authors and do not necessarily represent those of their affiliated organizations, or those of the publisher, the editors and the reviewers. Any product that may be evaluated in this article or claim that may be made by its manufacturer is not guaranteed or endorsed by the publisher.
Research integrity at Frontiers
Learn more about the work of our research integrity team to safeguard the quality of each article we publish.