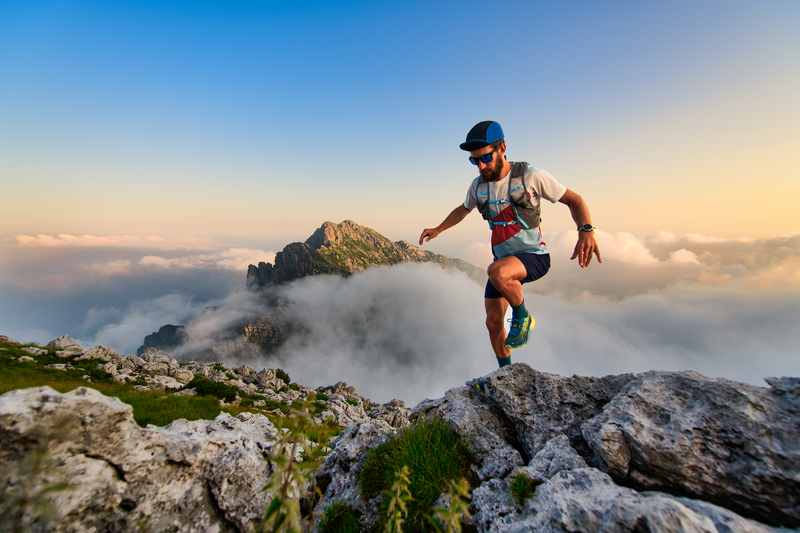
94% of researchers rate our articles as excellent or good
Learn more about the work of our research integrity team to safeguard the quality of each article we publish.
Find out more
MINI REVIEW article
Front. Drug Deliv. , 06 June 2022
Sec. Cardiovascular Drug Delivery
Volume 2 - 2022 | https://doi.org/10.3389/fddev.2022.913225
Cardiovascular diseases (CVD), including life-threatening atherosclerosis and arterial thrombosis, account for almost 50% of all deaths in Europe and around 30% of all deaths worldwide. Despite the ongoing improvement and accessibility of invasive cardiovascular interventions and pharmacological therapies, pathological processes often progress asymptomatically, before manifesting themselves as unstable angina pectoris, myocardial infarction, sudden cardiac death or stroke. Since atherosclerosis and thrombosis represent localized disease processes, insufficient response to systemically-administered drugs is a common problem. The available pharmacological therapies are often burdened by poor tolerability, limited efficacy and/or bioavailability. Although encapsulating drugs in a nanoscale shell increases their circulation time and availability, it does not guarantee disease-specific targeting, or the proper control of the drug release. In contrast, on-demand drug delivery has the advantage of localized treatment and allows reducing off-target effects by a stimuli-controlled drug release in the affected region. Such “smart” systems are expected to have a great therapeutic potential in CVD. This mini-review article highlights recent advances in stimuli-responsive drug delivery approaches to CVD, with specific drug release triggered either internally or externally.
Cardiovascular diseases (CVD) comprise a number of disorders of the heart and circulatory system, including atherosclerosis and thrombosis, the underlying causes of cardiovascular mortality. In particular, about 70–80% of acute atherothrombotic events result from the rupture of vulnerable atherosclerotic plaques, leading to cerebral ischemia, cardiac ischemia, or sudden cardiac death (Falk et al., 2013). Extensive pathologic and in vivo studies on atherosclerotic plaque development and destabilization, but also on the ischemic infarct regions, contributed to a deeper understanding of the disease processes and to the development of improved pharmacologic therapies (Pala et al., 2020). However, the efficacy of these therapies is still affected by poor tolerability and/or bioavailability of the drugs. Although pleiotropic anti-inflammatory activity of statins extends well beyond cholesterol lowering, adverse reactions including myopaty, as well as increased risk of diabetes and stroke are common (Collins et al., 2016). PCSK9 monoclonal antibodies are highly effective at lowering LDL-cholesterol (Stroes et al., 2022), but require frequent (once or twice a month) subcutaneous injection, have the drawbacks of high cost and may cause influenza-like symptoms, myalgia, hypersensitivity and allergic reactions. In an indication-seeking trial, anti-inflammatory therapy targeting the interleukin (IL)-1β with humanized monoclonal antibody canakinumab significantly reduced the rate of recurrent cardiovascular events independent of lipid-lowering therapy (Ridker et al., 2017), but the canakinumab injections may be associated with serious side effects, including allergic reactions, vertigo and increased risk of infections. These drawbacks of current therapies arise from their systemic administration, so that the plaque-specific effects are often limited despite an adequate control of systemic hyperlipidemia or inflammatory status. The long-recognized non-random distribution of atherosclerotic plaques within the arterial tree, which greatly facilitates site-specific invasive interventions (angioplasty, stenting, or endarterectomy), has not been taken into account when designing the new pharmaceutics and drug delivery systems. To improve drug bioavailability, encapsulation of drugs in a nanoscale shell was introduced. This approach increases the circulation time and availability of the pharmaceutics, but does not guarantee their disease-specific targeting, or the proper control of the drug release.
The site-specificity of atherothrombosis and resulting ischemia, as well as the growing understanding of pathologic mechanisms and biochemical factors involved in these processes call for the development of disease-targeted, personalized therapies. On demand, stimuli-responsive drug delivery systems offer multiple advantages in this context, including disease site-specific treatment and a controlled drug release in the affected region, which is expected to reduce adverse off-target effects. In the recent years, multiple stimuli-responsive drug delivery approaches to CVD have been reported including pH-, redox-, hypoxia-, enzyme-, shear- and light-responsive particles, polymers or hydrogels (Figure 1 and Table 1). The stimuli that control the release or the activity of drugs are commonly classified as physical, chemical, or biological. However, for the purpose of biomedical applications, a distinction is also made between intrinsic and external stimuli. In this paper, we follow the latter classification and discuss first the intrinsic stimuli, which are most commonly related to chemical and biological environment in the diseased tissues (tissue hypoxia, pH, oxidative stress, or enzymatic reactions), but also comprise biomechanical factors. Subsequently, we address recent reports on external stimuli, such as light, ultrasound, temperature or magnetic field.
FIGURE 1. Schematic overview of extrinsic and intrinsic stimuli used in “smart” cardiovascular drug delivery applications (created with BioRender.com).
TABLE 1. Overview of the highlighted stimuli-responsive drug delivery systems according to the type of stimulus.
This mini-review highlights some of the “smart” drug delivery systems that operate either at the micro- or nanoscale and have therapeutic potential in CVD.
Cardiovascular diseases are characterized by a specific pathologic microenvironment, involving chemical and biological factors that affect cellular functions and can be harnessed both to target and to activate the release of disease-specific drugs. The advantage of drug delivery systems that respond to intrinsic factors is that they are independent of external triggers and therefore capable of “intelligent” (i.e. responding to the presence and the strength of specific stimulus) release or activation of the drug. Thanks to their high sensitivity towards pathologic processes and environments, such carriers should not be activated in the healthy tissues with normal metabolic activity, thus limiting the potential off-target effects. Selected examples of their use for drug delivery are briefly outlined below.
In the process of atherosclerotic plaque formation, the deposition of lipids and inflammatory cells in the vessel wall, followed by arterial wall remodeling and fibrous cap formation increases the thickness of the wall beyond the physiological tissue oxygen diffusion limit of 200 µm. Reduced availability of oxygen leads to hypoxic cell injury (Liu et al., 2020) and contributes to the formation of the necrotic core in the atherosclerotic lesions. In later stages of the disease, during the rupture of atherosclerotic plaque, large amounts of thrombogenic material are exposed to the bloodstream, leading to an immediate start of coagulation process. The resulting thrombus may block the blood flow at the site of rupture, or embolize and occlude the smaller arteries at a downstream location. The loss of blood circulation results in rapid tissue hypoxia or even anoxia, which is a driving mechanism of cell death in the ischemic tissues, including infarcted myocardium and brain regions affected by ischemic stroke (Ferdinand and Roffe, 2016). However, although hypoxia-responsive drug delivery could be a promising therapeutic approach in CVD, relatively few studies focused on this factor until recently, when hypoxia-sensing nanocarriers inspired by mitochondria were described by (Lin et al., 2020). The authors developed double-shell poly (lactic-co-glycolic acid) (PLGA) nanoparticles contained melatonin to scavenge reactive oxygen species (ROS) and prevent apoptosis by activating mitochondrial melatonin receptor I to inhibit cytochrome c release. As a biological oxygen-sensing mechanism, circular DNA was incorporated on the surface of the particles by electrical adsorption. Oxygen-responsive expression of vascular endothelial growth factor (VEGF) was realized by binding hypoxia-inducible factor-1α (HIF-1α) with erythropoietin enhancers and was shown to respond to alternating hypoxia-normoxia conditions by up- and downregulating the reporter expression. In a mouse model of MI, these particles reduced cell death due to ischemia, improving structural and functional capacity of infarcted hearts (Lin et al., 2020).
Changes in pH of the tissues are commonly related to disease processes. Atherosclerotic lesions often exhibit local acidification of the extracellular fluids, resulting from hypoxia and the presence of activated macrophages, which synthesize ATP in the process of glycolysis contributing to enhanced local generation of lactate and protons. Low extracellular pH increases receptor-mediated phagocytosis and triggers the secretion of proinflammatory cytokines, but it also enhances oxidative modifications of LDL, thus contributing to atherogenesis (Oorni et al., 2015). In the ischemic myocardium lacking oxygen, oxidative phosphorylation and ATP production in the mitochondria are reduced and the preservation of heart muscle requires anaerobic glycolysis, which leads to development of acidosis (Oorni et al., 2015). In patients with MI, metabolic acidosis may occur and its severity correlates with mortality (Gandhi and Akholkar, 2015).
Consequently, tissue pH is a parameter that can be exploited for phase-transition in polymer-based drug delivery systems in specific microenvironments or tissues affected by pathological processes. This approach was developed in 2009 by (Namgung et al., 2009), who tested the possibility of pH-responsive plasmid DNA delivery to myocardium. For this, multiblock copolymer complexes (MBCP) with reversible sol-gel transition were synthesized from pluronic® and di-(ethylene glycol divinyl ether) and, upon gelation at 37°C, used as pH-sensitive carrier of pDNA. Under acidic conditions (pH 5.5) in vitro, the cleavage of acetal linkage caused degradation of the gel and a rapid release of pDNA (100% within 4 days). In mouse myocardium, the MBCP/pDNA polymeric gel showed a significant enhancement in gene transfection in comparison to that observed using naked pDNA, due to the protective effect of gel against enzymatic degradation and prolonged retention time of the matrix in the closed cavity of the pericardium.
In a more recent study, DNA-based nanotubes were utilized as a drug carrier system for the pH-dependent delivery of dexamethasone (Sellner et al., 2017). The nanotubes loaded with glucocorticoid-conjugated oligonucleotides were rapidly internalized by mouse macrophages in vitro and, thanks to the presence of pH-sensitive i-motif sequence, released dexamethasone in an acidic environment of endolysosomal compartment. Compared with free dexamethasone, DNA-dexamethasone nanotubes significantly reduced the tumor necrosis factor (TNF)-α expression in lipopolysaccharide-stimulated macrophages in vitro. In a mouse model of ischemia-reperfusion, the administration of DNA-dexamethasone nanotubes into postischemic muscle tissue led to reduced leukocyte transmigration and decreased the expression of the endothelial adhesion molecules.
Hypoxic conditions in atherosclerotic lesions, or myocardial anoxia that occurs during coronary vessel occlusion, lead to a loss of ATP production and an increase in ROS production in the mitochondria of affected cells (Zalba, 2021). Consequently, all acute cardiovascular events are associated with an excessive generation of ROS. Ischemic tissues are additionally challenged by reperfusion injury that occurs upon restoration of oxygen supply (Bugger and Pfeil, 2020). In the condition of antioxidant depletion by ischemia, secondary generation of ROS can damage cellular macromolecules leading to autophagy, apoptosis, and necrosis, but can also contribute to the local inflammatory responses, enhanced platelet aggregation, and loss of NO-mediated vasodilation (Bugger and Pfeil, 2020). Currently, no pharmacotherapy is available to attenuate ischemia-reperfusion (I/R) injury. Despite the fact that ROS have been implicated in the ischemic organ injury, the systemic administration of exogenous antioxidants has proven ineffective against oxidative stress-induced injury (Vivekananthan et al., 2003). Thus, better strategies that take into account the molecular mechanisms underlying I/R injury are urgently needed to improve patients’outcomes following MI.
In search for improved strategies of antioxidant delivery and ROS-sensitive targeting, several approaches have been reported. In a study by Li et al., ROS-responsive nanoparticles produced of poly (ethylene glycol) (PEG) and poly (propylene sulfide) (PPS) were used for encapsulation of a potent plant antioxidant, ginsenoside Rg3 (Li et al., 2020). Upon the exposure to ROS, Rg3-loaded PEG-PPS nanoparticles injected in the infarcted rat myocardium released Rg3, which improved the cardiac function by reducing oxidative stress, inflammation and fibrotic processes via FoxO3a-dependent mechanism. Similar approach was developed by Wu et al. to reduce oxidative stress in atherosclerotic lesions (Wu et al., 2018). ROS-responsive polymer micelles of PEG-PPS were loaded with andrographolide, a natural anti-atherosclerotic compound. Upon oxidation, the spherical micelles became soluble in water and formed random aggregates, releasing the drug. This reduced ROS levels in lipopolysaccharide-stimulated macrophages in vitro and inhibited the expression of IL-6 and monocyte chemoattractant protein (MCP)-1 in these cells and in atherosclerotic plaques. Further, their dual effect in alleviating inflammation and oxidative stress resulted in a very efficient prevention of atherosclerosis in ApoE−/− mice.
Another type of ROS-responsive theranostic nanoplatform was developed by Ma et al., where a fluorophore activated by two-photon aggregation-induced emission was linked to β-cyclodextrin using a ROS-responsive bond (Ma et al., 2020). The system was loaded with prednisolone via supramolecular interaction and packed into nanosized micelles based on a ROS-sensitive copolymer poly (2-methylthioethanol methacrylate)-poly (2-metha-cryloyloxyethyl phosphorylcholine). The resulting micelles, termed TPCDP@PMM have been shown to accumulate in atherosclerotic plaques of ApoE−/− mice and disrupt upon contact with ROS, leading to prednisolone release and atherosclerosis inhibition [Figure 2, (Ma et al., 2020)].
FIGURE 2. Anti-atherosclerotic effects of ROS-responsive lipid-scavenging micelles without drug loading (TPCD@PMM) and with prednisolone (TPCDP@PMM) in ApoE−/− mice. Shown are Oil Red O staining of (A) en face aortas (scale bars: 5 mm) and (B) sections of aortic arch (scale bars: 500 µm); (C) Sections of aortic arch stained with H&E, antibody to CD68, MMP-9, α-SMA, and Masson’s trichrome (scale bars: 500 µm). Adapted from (Ma et al., 2020) (Ma et al. Small, DOI: 10.1002/smll.202003253, Wiley 2020).
In a study by Mu et al., ROS-responsive polymeric micelles based on PEG-poly (tyrosine-ethyl oxalyl) (PEG-Ptyr-EO) that respond to the oxidative microenvironment of atherosclerotic plaques were also described (Mu et al., 2020). Hyaluronic acid (HA) coating was designed for targeting of CD44-positive inflammatory macrophages. In the presence of ROS, PEG-Ptyr-EO released simvastatin loaded into the particles to reduce the activation of plaque macrophages and additionally contributed to ROS consumption, thus diminishing the oxidative stress. Intravenous administration of ROS-responsive simvastatin-loaded micelles in a mouse model was shown to reduce the plaque cholesterol content and the burden of atherosclerosis. Similarly effective ROS-responsive system was recently introduced by Shen et al., who developed porous PLGA nanosystem loaded with ginsenoside and catalase, and coated with U937 cell membrane. Biomimetic membrane coating allowed the particles to escape phagocytosis and increased their uptake in endothelial cells. ROS-triggered release of ginsenoside resulted in a potent anti-angiogenic and anti-inflammatory response, significantly reduced plaque burden in ApoE−/−mice, and decreased the blood levels of atherogenic lipids as compared with free drug or particles without biomimetic membrane (Shen et al., 2022).
Two stimuli-responsive nanoplatforms loaded with an anti-proliferative drug rapamycin (RAP) were also reported (Dou et al., 2017), one based on acid-labile acetalated b-cyclodextrin (Ac-bCD) for degradation in mild acidic buffers, and the other composed of ROS-responsive Ox-bCD, obtained by chemical modification of b-CD with 1,1′-carbonyldiimidazole-activated 4-(hydroxymethyl) phenylboronic acid pinacol ester. Both types of nanoparticles showed anti-inflammatory potential in macrophages in vitro and significantly delayed progression of atherosclerosis in ApoE−/− mice upon intraperitoneal injection. Ox-bCD/RAP particles effectively prevented ROS generation in macrophages treated with phorbol 12-myristate 13-acetate, and the foam cell formation. Compared with PLGA/RAP particles, both stimuli-responsive nanosystems significantly decreased plaque size and lipid content in mice.
ROS are also responsible for the acute cerebral I/R injury (Carbone et al., 2015). The generation of ROS immediately after the onset of acute ischemic stroke results in a rapid depletion of antioxidants and scavenger enzymes (De Silva and Miller, 2016; Grochowski et al., 2018) thus driving cellular necrosis. Central nervous system cells are more vulnerable to ROS toxicity due to their inherently enhanced oxidative metabolism and less antioxidant enzymes, as well as a higher membrane fatty acid content (Olmez and Ozyurt, 2012). Upon restoration of blood flow, rapid tissue oxygenation leads to a second burst of ROS production, resulting in further injury. Several drugs or antioxidant therapeutics have been proposed for stroke therapy, including NOX (NADPH oxidase) inhibitors and xanthine oxidase inhibitors (Rodrigo et al., 2013), but their efficacy is limited by low specificity, low bioavailability, and side effects (De Silva and Miller, 2016).
Addressing this clinical need, ROS-sensing drug delivery systems have recently been developed for the treatment of stroke. To achieve the specific targeting of a neuroprotective agent NR2B9C to the ischemic site, and improve controllability of drug release, (Lv et al., 2018) produced a ROS-responsive nanocarrier. NR2B9C was loaded into dextran cores modified with ROS-sensitive boronic ester to enable release triggered by high intracellular ROS in ischemic neurons. Targeting of the nanosystem was realized by inserting a stroke homing peptide (CLEVSRKNC) into the shell composed of erythrocyte membrane. In vitro, the nanocarriers underwent a rapid hydrolysis in the presence of H2O2 and had strong protective effects against glutamate-induced cytotoxicity in rat adrenal pheochromocytoma cell line PC-12. In a rat model of middle cerebral artery occlusion, ROS-sensing nanoparticles improved the active targeting of NR2B9C to the ischemic area and reduced ischemic brain damage.
Apart from oxidative stress, the microenvironment of atherosclerotic lesions is characterized by an increased protease activity. Matrix metalloproteinases (MMPs), comprising over 20 structurally-related proteases that share a Zn2+ ion at the catalytic site, play a major role in arterial remodeling during atherogenesis and atherosclerotic plaque progression [reviewed in (Newby, 2006)]. In particular gelatinases (MMP-2 and 9) degrade nonfibrillar collagens, including type IV collagen, which is a major component of basement membranes of the vascular wall. Their upregulation in response to arterial injury thus enables migration of smooth muscle cells into the intima and subsequent fibrous cap formation. In advanced plaques, the activity of multiple proteases beyond MMPs, including mast cell chymase, elastase, as well as hyaluronidase, contributes to plaque inflammation and angiogenesis, apoptosis of SMCs and tissue proteolysis, which may cause thinning of a fibrous cap protecting atherosclerotic plaque. This characteristic feature of cardiovascular diseases can be utilized to specifically release drugs in the regions with increased enzymatic activity, if a cleavable, enzyme-responsive linker is introduced in the system. An interesting example of combining two distinct biological stimuli for control of the drug delivery to atherosclerotic lesions was reported by (Peters et al., 2019). The authors utilized peptide amphiphiles (PAs) to develop nanocarriers that sense the increased levels of MMP-2, MMP-9 and ROS. To this end, apolipoprotein A1-mimetic peptide was bound to PAs by peptide linkers cleaved by MMPs or ROS. The efficacy of the nanocarriers was tested in vitro on macrophages challenged with interferon gamma or lipopolysaccharide, showing the release of the ApoA1-Ac2–26 peptide and reduced macrophage activation.
A dual drug/siRNA delivery system responding to hyaluronidase type II (Hyal-2) was also reported (Zhao et al., 2018). The designed PLGA nanocarriers encapsulated atorvastatin to control lipid trafficking and reduce inflammation, and siRNA against lectin-like oxidized low-density lipoprotein receptor-1 (LOX-1). The particle cores were clad in three external layers: an innermost lipid bilayer, an intermediate apolipoprotein A1 layer for macrophage targeting, and an outer layer of high molecular weight HA (200 kDa). The outer layer allowed CD44-dependent targeting, and upon Hyal-2 cleavage led to exposure of the intermediate ApoA1 layer for enhanced entry into macrophages. The efficacy of this dual-therapy nanosystem was demonstrated in atherosclerotic mice upon 12-weeks biweekly administration, showing a significant decrease in plaque size, as well as reduced lipid and macrophage accumulation.
MMP-9 and MMP-2 are also important regulators of myocardial remodeling in the aftermath of the ischemia. MMP-2 levels have been shown to increase significantly during acute MI and can furthermore predict the severity of the disease (Li et al., 2021). In line with this, a siRNA delivery system that responds to local upregulation in proteolytic activity after MI was reported by (Wang et al., 2018). Cholesterol-modified siRNA against MMP-2 was sequestered in an injectable shear-thinning and self-healing hydrogel composed of HA modified with bCD and functionalized with cleavable peptide crosslinkers. The release of siRNA in the presence of proteases effectively silenced MMP-2 expression in cardiac fibroblasts in vitro. In a rat model of MI, protease-sensing siMMP-2 hydrogel led to significantly increased ejection fraction, stroke volume and cardiac output. Additionally, hydrogel erosion was gradually reduced upon silencing of MMP-2, providing mechanical support and improving myocardial thickness in the infarct at 4 weeks post-ischemic injury.
Another type of bio-responsive material, i.e. human elastin-like polypeptides fused with epidermal growth factor (EGF), were incorporated in 3D-printed alginate and chitosan scaffolds in a study by (Bandiera et al., 2021), enabling the release of EGF upon contact with elastases in vitro. The authors expect such scaffolds may in the future support tissue repair in patients with diabetes, vascular disease and inflammatory disorders, characterized by delayed healing.
The critical involvement of local hemodynamic forces in the initiation of atherosclerosis has been long recognized [3, 4], but the knowledge of precise role played by hemorheologic and hemodynamic factors in plaque destabilization and rupture has emerged more recently (Thrysoe et al., 2010; Cicha et al., 2011). In advanced stages of atherosclerosis, the presence of lesions protruding into the lumen dramatically alters the local hemodynamic conditions in affected arteries. As the lumen narrows due to increasing severity of stenosis, an increase in shear stress occurs (Sadeghi et al., 2011). Although elevated wall shear stress is unlikely to directly induce mechanical rupture of the plaque, the increasing shear stress upstream of the maximal narrowing activates inflammatory processes (Cicha et al., 2011). Furthermore, shear stress is known to activate platelets, as shown in patients with plaque erosion, in whom the prevalence of platelet-rich thrombus (84%) was the highest in the severe stenosis group (Kurihara et al., 2021). Thus, although hemodynamic forces directly affect many pathophysiological processes related to CVD, mechanosensing drug delivery systems that target local alterations in hemodynamic load associated with atherosclerosis or thrombosis have been very rarely utilized thus far (Korin, 2015). One of the conditions that should benefit from such approach is arterial thrombosis, underlying stroke or myocardial infarction (MI). The first mechanosensitive drug delivery system was described in 2012 by Korin et al., who designed micro-aggregates composed of PLGA nanoparticles coated with thrombolytic drug, tissue-plasminogen activator (tPA). Upon the exposure to abnormally high shear stress in the regions of vascular occlusion, the micro-aggregates were disrupted and released the drug locally. Shear-activated tPA-coated nanoparticles rapidly dissolved arterial clots in mouse mesenteric arteries, with complete clearance of occluding thrombi within 5 min of administration, at the effective drug dose 100 times lower than the required dose of free tPA (Korin et al., 2012).
More recently, Yao et al. developed mechanically-sensitive micelles for simvastatin release in stenotic region (Yao et al., 2020). To enable targeting of inflammatory macrophages via CD44 receptor, micelles based on high molecular weight HA hydrogel modified with glycidyl methacrylate were developed. The micelles effectively targeted activated macrophages in vitro and underwent deformation and resizing under stenotic conditions (75% stenosis) leading to a drug release enhanced by about 50% as compared to non-stenotic conditions. The precise targeting of fluorescently-labeled simvastatin micelles to macrophages was shown in ApoE−/− mice and the effective drug release in the plaque region was confirmed in atherosclerotic rabbits (Yao et al., 2020).
Such mechano-sensing strategy of drug delivery utilizing a universal hemodynamic phenomenon is expected to be applicable for all occlusive vascular conditions, including e.g. treatment of stenotic atherosclerotic plaques, pulmonary emboli, and ischemic stroke.
Drug delivery systems that respond to extrinsic stimuli can be activated by an externally-triggered process, which allows a good control of stimulus strength and timing. However, their efficacy depends to a large degree on a precise targeting to achieve local activation and/or drug release. Such drug delivery systems may in some cases demand more complexity, as specific targeting may be required to reduce off-target effects and their accumulation in healthy tissues.
The potential of photoangioplasty, light-activated therapy utilizing photosensitizers in combination with endovascular light delivery devices for selective reduction of atherosclerotic or re-stenotic lesions, has been long recognized (Rockson et al., 2000), (Waksman et al., 2006). To enhance the targeting precision, magnetofluorescent nanoparticles loaded with light-activated phototoxic moieties were subsequently developed (McCarthy et al., 2010). Upon irradiation of the plaques with 650 nm light, these agents, enabled an efficient focal ablation of inflammatory macrophages, without affecting endothelial cells or SMCs. In the recent years, many more reports described smart photoactivated nanocarriers (Karimi et al., 2017), examples of which are highlighted below.
An elegant approach to rescuing cardiomyoblasts from I/R injury has been reported by Woods et al. The authors developed cell-permeable ruthenium (II) complex releasing hydrogen sulfide (H2S) in response to red light irradiation. The resulting increase in intracellular H2S was shown to protect H9c2 cardiomyoblasts from the oxidative injury during I/R in vitro (Woods et al., 2018).
Another interesting example of light-responsive particles are mesoporous silica-coated gold nanorods functionalized with an arginine-rich peptide developed for imaging and phototermal therapy of thrombosis (Chang et al., 2021). A complex involving urokinase-type plasminogen activator (uPA), an algae-derived platelet-targeting moiety with anticoagulant function (fucoidan), and a contrast agent for near-infrared (NIR) fluorescence imaging (indocyanine green) was loaded onto the particle pores to enable precise targeting, thrombus imaging, as well as photothermal thrombolysis upon exposure to NIR irradiation (808 nm). In a mouse model of mesenteric thrombosis, NIR irradiation for 5 min (2.0 W/cm2) induced a fast release of uPA resulting in rapid thrombolysis and a slower release of fucoidan. This approach can thus contribute to extended prevention of thrombosis and improved endothelial regeneration, at the same time reducing the risk of hemorrhagic complications.
Catheter-directed thrombolysis for acute peripheral arterial occlusion requires percutaneous intervention and carries a considerable risk of hemorrhage, damage to blood vessel and infection. To reduce the risk associated with thrombolysis and improve its efficacy, temperature- and ultrasound-responsive carriers have been investigated. As an example of the former, thermosensitive PEGylated magnetoliposomes loaded with tPA were developed by Liu et al. and tested in a rat embolic model (Liu et al., 2019). The local temperature control at the right iliac artery was achieved by superfusion of the target site with saline at 37°C vs. 43°C, using a water bath and a peristaltic pump. After the clot lodging in the iliac artery, magnetic guidance followed by focal hyperthermia significantly restored iliac blood flow within 15 min post intraarterial administration, whereas tPA at the same dose did not elicit significant restoration. The effect observed at 43°C was significantly higher as compared to that at 37°C, demonstrating the benefits of thermoresponsive thrombolysis.
In the recent years, clinical interest has been drawn to so-called contrast-enhanced sonothrombolysis, based on adding ultrasound contrast agents, e.g. micro- or nano-bubbles with gaseous inner core, together with tPA to the standard catheter-directed thrombolysis and applying pulsed ultrasound (Nederhoed et al., 2021). The technique is considered a promising alternative to the standard catheter-directed thrombolysis, but has certain limitations, as the delivery of tPA through an end-hole microcatheter at the proximal face of an occlusive clot does not allow an effective reperfusion (Kleven et al., 2021). However, ultrasound contrast agents can also serve as carriers for thrombolytic drugs, that are released upon destruction of microbubbles or enhanced cavitation by ultrasound. For the purpose of sonothrombolysis, tPA-loaded echogenic liposomes were developed by Shakhar et al. The liposomes co-encapsulated tPA and octafluoropropane gas (OFP) to promote bubble activity in response to ultrasound exposure (Shekhar et al., 2017). In the pilot studies with human blood clots, the tPA-OFP liposomes showed improved thrombolytic activity upon application of ultrasound as compared with free tPA. While the thrombolytic efficacy of the liposomes and ultrasound was comparable to the combination of free tPA, Definity and pulsed ultrasound, the authors showed that the lipid shell effectively protected the drug against degradation by plasminogen activator inhibitor-1, which may represent an important factor in clinical application (Shekhar et al., 2019).
Ultrasound-responsive microbubbles can also serve to deliver therapeutic biologics to the cells. An example of this type of application was reported by Gill et al., who utilized ultrasound-mediated liposome/microbubble transfection do deliver miR-133 mimics to HL-1 cardiomyocytes in vitro (Gill et al., 2014). The microbubble formulations (either complexed with, or encapsulating miR-133) were shown to improve intracellular delivery and efficiently reduce cardiomyocyte hypertrophy.
An interesting approach to local thrombolysis has been developed using tPA-loaded magnetic nanoparticles in combination with external magnetic field. Of note, this type of carrier can serve to realize two distinct functionalities, namely magnetic targeting and magnetically-activable release. Hu et al. produced APTES-functionalized superparamagnetic iron oxide nanorods loaded with 6% tPA, which were guided to the thrombus site in vitro and exposed to a rotating magnetic field (20 Hz, 3 mT). In the presence of magnetic field, accelerated release of adsorbed tPA from the surface of the particles was observed due to distortion and diffusion, contributing to significantly higher thrombolysis speed as compared with samples without magnetic stimulation (Hu et al., 2016). In line with this, Moghanizadeh et al. developed iron oxide nanoparticles with silica shell, that were loaded with streptokinase. These particles were subsequently used in vitro to test the influence of magnetic field intensity on clot dissolution speed, showing that thrombolysis rate increases with the growing intensity of static magnetic field, and that deeper clot penetration is possible using these nanocarriers (Moghanizadeh et al., 2021).
The field of drug delivery systems that respond to specific stimuli is rapidly growing and its potential in the management of CVD starts gaining attention. Improved efficacy of the drugs and reduced adverse effects, in parallel with improved control over the time and dose of released pharmacologic agents constitute considerable advantages over the current therapies. In particular, harnessing multiple stimuli may in the future provide a solution to control the complex pathophysiological processes occurring in advanced atherosclerotic plaque, or in the regions of ischemia/reperfusion injury, limiting the diseases development and improving tissue regeneration in an adequate spatiotemporal manner. Nonetheless, the clinical translation of the promising candidate carriers will certainly be challenging. The material-related and technical challenges include the choice of bio/nanomaterials to create safe, biodegradable and non-immunogenic carriers, the reproducibility, upscaling requirements and cost of the manufacturing process. Further, ensuring a long-term stimuli-sensitive release of drugs is an obstacle for carrier design and requires special solutions to prevent burst release and rapid drug depletion. Besides, multiple biological hurdles must be solved before the respective carriers enter the regulatory approval pathway. Among these, hemodynamics-related difficulties of drug delivery to atherosclerotic plaques must be considered, as the retention time of the carriers in the arteries may not be sufficient to permit the access to the lesion via luminal endothelium. In this context, advantage could be taken of specific, either molecular or magnetic, targeting to avoid scavenging of carriers by reticuloendothelial system and allow their effective accumulation in the plaque or ischemic region. Alternatively, utilizing long-circulating nanosized carriers could enable their entry into plaque via the leaky neovessels and their retention by EPR effect. Another challenge is related to the selection of the timing for drug administration. While the acute ischemic events call for immediate interventions, appropriate timing is a particularly difficult issue in case of atherosclerotic plaques, which in the majority of cases progress asymptomatically. However, in symptomatic patients at risk of plaque instability, the drug delivery systems that respond to specific (potentially multiple) stimuli would be very beneficial, as these systems could release/activate the drug exactly when needed and ideally, to a needed degree.
Taken together, on-demand drug delivery is often the key to the therapeutic success, but is very limited without the ability of nanocarriers to sense and respond to the diseased microenvironment. Although the clinical translation of complex drug-delivery nanosystems may be challenging, achieving a precise control over therapeutic agents, in terms of targeting, retention and stimuli-sensitive release over long-term periods would be of particular importance in order to regulate the biological repair process and promote tissue regeneration in CVD.
IC: writing and editing the text; HG: writing and figure preparation; EE: writing and review.
This work was supported by the German Federal Ministry of Education and Research (BMBF) within the ERA-Net project “MAGNA” (Grant No. 01DJ21004).
The authors declare that the research was conducted in the absence of any commercial or financial relationships that could be construed as a potential conflict of interest.
All claims expressed in this article are solely those of the authors and do not necessarily represent those of their affiliated organizations, or those of the publisher, the editors and the reviewers. Any product that may be evaluated in this article, or claim that may be made by its manufacturer, is not guaranteed or endorsed by the publisher.
Bandiera, A., Catanzano, O., Bertoncin, P., Bergonzi, C., Bettini, R., and Elviri, L. (2021). 3D-printed Scaffold Composites for the Stimuli-Induced Local Delivery of Bioactive Adjuncts. Biotechnol. Appl. Biochem. 2245, 1–12. doi:10.1002/bab.2245
Bugger, H., and Pfeil, K. (2020). Mitochondrial ROS in Myocardial Ischemia Reperfusion and Remodeling. Biochimica Biophysica Acta (BBA) - Mol. Basis Dis. 1866, 165768. doi:10.1016/j.bbadis.2020.165768
Carbone, F., Teixeira, P. C., Braunersreuther, V., Mach, F., Vuilleumier, N., and Montecucco, F. (2015). Pathophysiology and Treatments of Oxidative Injury in Ischemic Stroke: Focus on the Phagocytic NADPH Oxidase 2. Antioxidants Redox Signal. 23, 460–489. doi:10.1089/ars.2013.5778
Chang, L.-H., Chuang, E.-Y., Cheng, T.-M., Lin, C., Shih, C.-M., Wu, A. T., et al. (2021). Thrombus-specific Theranostic Nanocomposite for Codelivery of Thrombolytic Drug, Algae-Derived Anticoagulant and NIR Fluorescent Contrast Agent. Acta Biomater. 134, 686–701. doi:10.1016/j.actbio.2021.07.072
Cicha, I., Wörner, A., Urschel, K., Beronov, K., Goppelt-Struebe, M., Verhoeven, E., et al. (2011). Carotid Plaque Vulnerability. Stroke 42, 3502–3510. doi:10.1161/strokeaha.111.627265
Collins, R., Reith, C., Emberson, J., Armitage, J., Baigent, C., Blackwell, L., et al. (2016). Interpretation of the Evidence for the Efficacy and Safety of Statin Therapy. Lancet 388, 2532–2561. doi:10.1016/s0140-6736(16)31357-5
De Silva, T. M., and Miller, A. A. (2016). Cerebral Small Vessel Disease: Targeting Oxidative Stress as a Novel Therapeutic Strategy? Front. Pharmacol. 7, 61. doi:10.3389/fphar.2016.00061
Dou, Y., Chen, Y., Zhang, X., Xu, X., Chen, Y., Guo, J., et al. (2017). Non-proinflammatory and Responsive Nanoplatforms for Targeted Treatment of Atherosclerosis. Biomaterials 143, 93–108. doi:10.1016/j.biomaterials.2017.07.035
Falk, E., Nakano, M., Bentzon, J. F., Finn, A. V., and Virmani, R. (2013). Update on Acute Coronary Syndromes: The Pathologists' View. Eur. Heart J. 34, 719–728. doi:10.1093/eurheartj/ehs411
Ferdinand, P., and Roffe, C. (2016). Hypoxia after Stroke: A Review of Experimental and Clinical Evidence. Exp Trans Stroke Med 8, 9. doi:10.1186/s13231-016-0023-0
Gandhi, A., and Akholkar, P. (2015). Metabolic Acidosis in Acute Myocardial Infarction. Int. J. Adv. Med. 2, 260–263. doi:10.18203/2349-3933.ijam20150556
Gill, S.-L., O'neill, H., Mccoy, R. J., Logeswaran, S., O'brien, F., Stanton, A., et al. (2014). Enhanced Delivery of microRNA Mimics to Cardiomyocytes Using Ultrasound Responsive Microbubbles Reverses Hypertrophy in an Iin-Vvitro Model. Thc 22, 37–51. doi:10.3233/thc-130772
Grochowski, C., Litak, J., Kamieniak, P., and Maciejewski, R. (2018). Oxidative Stress in Cerebral Small Vessel Disease. Role of Reactive Species. Free Radic. Res. 52, 1–13. doi:10.1080/10715762.2017.1402304
Hu, J., Huang, W., Huang, S., Zhuge, Q., Jin, K., and Zhao, Y. (2016). Magnetically Active Fe3O4 Nanorods Loaded with Tissue Plasminogen Activator for Enhanced Thrombolysis. Nano Res. 9, 2652–2661. doi:10.1007/s12274-016-1152-4
Karimi, M., Sahandi Zangabad, P., Baghaee-Ravari, S., Ghazadeh, M., Mirshekari, H., and Hamblin, M. R. (2017). Smart Nanostructures for Cargo Delivery: Uncaging and Activating by Light. J. Am. Chem. Soc. 139, 4584–4610. doi:10.1021/jacs.6b08313
Kleven, R. T., Karani, K. B., Hilvert, N., Ford, S. M., Mercado-Shekhar, K. P., Racadio, J. M., et al. (2021). Accelerated Sonothrombolysis with Definity in a Xenographic Porcine Cerebral Thromboembolism Model. Sci. Rep. 11, 3987. doi:10.1038/s41598-021-83442-3
Korin, N., Kanapathipillai, M., Matthews, B. D., Crescente, M., Brill, A., Mammoto, T., et al. (2012). Shear-Activated Nanotherapeutics for Drug Targeting to Obstructed Blood Vessels. Science 337, 738–742. doi:10.1126/science.1217815
Korin, N. (2015). Mechanoresponsive Nanotherapeutic for Localized Drug Delivery to Flow Obstructed Blood Vessels. Ther. Deliv. 6, 895–897. doi:10.4155/tde.15.36
Kurihara, O., Takano, M., Soeda, T., Fracassi, F., Araki, M., Nakajima, A., et al. (2021). Degree of Luminal Narrowing and Composition of Thrombus in Plaque Erosion. J. Thromb. Thrombolysis 51, 143–150. doi:10.1007/s11239-020-02159-8
Li, L., Wang, Y., Guo, R., Li, S., Ni, J., Gao, S., et al. (2020). Ginsenoside Rg3-Loaded, Reactive Oxygen Species-Responsive Polymeric Nanoparticles for Alleviating Myocardial Ischemia-Reperfusion Injury. J. Control. Release 317, 259–272. doi:10.1016/j.jconrel.2019.11.032
Li, Y., Li, L., Wang, K., Wu, P., and Cui, Y. (2021). Investigation on Risk Stratification and the Prognostic Value of Hs-TnT Combined with MMP-2 in Patients with Acute Coronary Syndrome. Biomed. Res. Int. 2021, 1040171. doi:10.1155/2021/1040171
Lin, Y., Liu, J., Bai, R., Shi, J., Zhu, X., Liu, J., et al. (2020). Mitochondria-Inspired Nanoparticles with Microenvironment-Adapting Capacities for On-Demand Drug Delivery after Ischemic Injury. ACS Nano 14, 11846–11859. doi:10.1021/acsnano.0c04727
Liu, C.-H., Hsu, H.-L., Chen, J.-P., Wu, T., and Ma, Y.-H. (2019). Thrombolysis Induced by Intravenous Administration of Plasminogen Activator in Magnetoliposomes: Dual Targeting by Magnetic and Thermal Manipulation. Nanomedicine Nanotechnol. Biol. Med. 20, 101992. doi:10.1016/j.nano.2019.03.014
Liu, M., Galli, G., Wang, Y., Fan, Q., Wang, Z., Wang, X., et al. (2020). Novel Therapeutic Targets for Hypoxia-Related Cardiovascular Diseases: The Role of HIF-1. Front. Physiol. 11, 774. doi:10.3389/fphys.2020.00774
Lv, W., Xu, J., Wang, X., Li, X., Xu, Q., and Xin, H. (2018). Bioengineered Boronic Ester Modified Dextran Polymer Nanoparticles as Reactive Oxygen Species Responsive Nanocarrier for Ischemic Stroke Treatment. Acs Nano 12, 5417–5426. doi:10.1021/acsnano.8b00477
Ma, B., Xu, H., Zhuang, W., Wang, Y., Li, G., and Wang, Y. (2020). ROS Responsive Nanoplatform with Two-Photon AIE Imaging for Atherosclerosis Diagnosis and "Two-Pronged" Therapy. Small 16, e2003253. doi:10.1002/smll.202003253
Mccarthy, J. R., Korngold, E., Weissleder, R., and Jaffer, F. A. (2010). A Light-Activated Theranostic Nanoagent for Targeted Macrophage Ablation in Inflammatory Atherosclerosis. Small 6, 2041–2049. doi:10.1002/smll.201000596
Moghanizadeh, A., Ashrafizadeh, F., Varshosaz, J., and Ferreira, A. (2021). Study the Effect of Static Magnetic Field Intensity on Drug Delivery by Magnetic Nanoparticles. Sci. Rep. 11, 18056. doi:10.1038/s41598-021-97499-7
Mu, D., Li, J., Qi, Y., Sun, X., Liu, Y., Shen, S., et al. (2020). Hyaluronic Acid-Coated Polymeric Micelles with Hydrogen Peroxide Scavenging to Encapsulate Statins for Alleviating Atherosclerosis. J. Nanobiotechnol. 18, 179. doi:10.1186/s12951-020-00744-w
Namgung, R., Nam, S., Kim, S. K., Son, S., Singha, K., Kwon, J.-S., et al. (2009). An Acid-Labile Temperature-Responsive Sol-Gel Reversible Polymer for Enhanced Gene Delivery to the Myocardium and Skeletal Muscle Cells. Biomaterials 30, 5225–5233. doi:10.1016/j.biomaterials.2009.05.073
Nederhoed, J. H., Tjaberinga, M., Otten, R. H. J., Evers, J. M., Musters, R. J. P., Wisselink, W., et al. (2021). Therapeutic Use of Microbubbles and Ultrasound in Acute Peripheral Arterial Thrombosis: A Systematic Review. Ultrasound Med. Biol. 47, 2821–2838. doi:10.1016/j.ultrasmedbio.2021.06.001
Newby, A. C. (2006). Do metalloproteinases Destabilize Vulnerable Atherosclerotic Plaques? Curr. Opin. Lipidol. 17, 556–561. doi:10.1097/01.mol.0000245262.48258.b4
Olmez, I., and Ozyurt, H. (2012). Reactive Oxygen Species and Ischemic Cerebrovascular Disease. Neurochem. Int. 60, 208–212. doi:10.1016/j.neuint.2011.11.009
Öörni, K., Rajamäki, K., Nguyen, S. D., Lähdesmäki, K., Plihtari, R., Lee-Rueckert, M., et al. (2015). Acidification of the Intimal Fluid: The Perfect Storm for Atherogenesis. J. Lipid Res. 56, 203–214. doi:10.1194/jlr.r050252
Pala, R., Anju, V., Dyavaiah, M., Busi, S., and Nauli, S. M. (2020). Nanoparticle-Mediated Drug Delivery for the Treatment of Cardiovascular Diseases. Ijn 15, 3741–3769. doi:10.2147/ijn.s250872
Peters, E. B., Tsihlis, N. D., Karver, M. R., Chin, S. M., Musetti, B., Ledford, B. T., et al. (2019). Atheroma Niche-Responsive Nanocarriers for Immunotherapeutic Delivery. Adv. Healthc. Mat. 8, e1801545. doi:10.1002/adhm.201801545
Ridker, P. M., Macfadyen, J. G., Thuren, T., Everett, B. M., Libby, P., Glynn, R. J., et al. (2017). Effect of Interleukin-1β Inhibition with Canakinumab on Incident Lung Cancer in Patients with Atherosclerosis: Exploratory Results from a Randomised, Double-Blind, Placebo-Controlled Trial. Lancet 390, 1833–1842. doi:10.1016/S0140-6736(17)32247-X
Rockson, S. G., Lorenz, D. P., Cheong, W.-F., and Woodburn, K. W. (2000). Photoangioplasty. Circulation 102, 591–596. doi:10.1161/01.cir.102.5.591
Rodrigo, R., Fernandez-Gajardo, R., Gutierrez, R., Matamala, J., Carrasco, R., Miranda-Merchak, A., et al. (2013). Oxidative Stress and Pathophysiology of Ischemic Stroke: Novel Therapeutic Opportunities. Cnsnddt 12, 698–714. doi:10.2174/1871527311312050015
Sadeghi, M. R., Shirani, E., Tafazzoli-Shadpour, M., and Samaee, M. (2011). The Effects of Stenosis Severity on the Hemodynamic Parameters-Assessment of the Correlation between Stress Phase Angle and Wall Shear Stress. J. Biomechanics 44, 2614–2626. doi:10.1016/j.jbiomech.2011.08.017
Sellner, S., Kocabey, S., Zhang, T., Nekolla, K., Hutten, S., Krombach, F., et al. (2017). Dexamethasone-conjugated DNA Nanotubes as Anti-inflammatory Agents in Vivo. Biomaterials 134, 78–90. doi:10.1016/j.biomaterials.2017.04.031
Shekhar, H., Bader, K. B., Huang, S., Peng, T., Huang, S., Mcpherson, D. D., et al. (2017). In Vitrothrombolytic Efficacy of Echogenic Liposomes Loaded with Tissue Plasminogen Activator and Octafluoropropane Gas. Phys. Med. Biol. 62, 517–538. doi:10.1088/1361-6560/62/2/517
Shekhar, H., Kleven, R. T., Peng, T., Palaniappan, A., Karani, K. B., Huang, S., et al. (2019). In Vitro characterization of Sonothrombolysis and Echocontrast Agents to Treat Ischemic Stroke. Sci. Rep. 9, 9902. doi:10.1038/s41598-019-46112-z
Shen, J.-W., Li, C., Yang, M.-Y., Lin, J.-F., Yin, M.-D., Zou, J.-J., et al. (2022). Biomimetic Nanoparticles: U937 Cell Membranes Based Core-Shell Nanosystems for Targeted Atherosclerosis Therapy. Int. J. Pharm. 611, 121297. doi:10.1016/j.ijpharm.2021.121297
Stroes, E. S. G., Stiekema, L. C. A., and Rosenson, R. S. (2022). PCSK9 Inhibitors: Pharmacology, Adverse Effects, and Use. [Online]. Netherlands: Wolters Kluwer. Available at: https://www.uptodate.com/contents/pcsk9-inhibitors-pharmacology-adverse-effects-and-use?search=pcsk9-inhibitors-pharmacology-adve&source=search_result&selectedTitle=1∼37&usage_type=default&display_rank=1[Accessed 2022].
Thrysøe, S. A., Oikawa, M., Yuan, C., Eldrup, N., Klaerke, A., Paaske, W. P., et al. (2010). Longitudinal Distribution of Mechanical Stresses in Carotid Plaques of Symptomatic Patients. Stroke 41, 1041–1043. doi:10.1161/STROKEAHA.109.571588
Vivekananthan, D. P., Penn, M. S., Sapp, S. K., Hsu, A., and Topol, E. J. (2003). Use of Antioxidant Vitamins for the Prevention of Cardiovascular Disease: Meta-Analysis of Randomised Trials. Lancet 361, 2017–2023. doi:10.1016/s0140-6736(03)13637-9
Waksman, R., Leitch, I. M., Roessler, J., Yazdi, H., Seabron, R., Tio, F., et al. (2006). Intracoronary Photodynamic Therapy Reduces Neointimal Growth without Suppressing Re-endothelialisation in a Porcine Model. Heart 92, 1138–1144. doi:10.1136/hrt.2005.073486
Wang, L. L., Chung, J. J., Li, E. C., Uman, S., Atluri, P., and Burdick, J. A. (2018). Injectable and Protease-Degradable Hydrogel for siRNA Sequestration and Triggered Delivery to the Heart. J. Control. Release 285, 152–161. doi:10.1016/j.jconrel.2018.07.004
Woods, J. J., Cao, J., Lippert, A. R., and Wilson, J. J. (2018). Characterization and Biological Activity of a Hydrogen Sulfide-Releasing Red Light-Activated Ruthenium(II) Complex. J. Am. Chem. Soc. 140, 12383–12387. doi:10.1021/jacs.8b08695
Wu, T., Chen, X., Wang, Y., Xiao, H., Peng, Y., Lin, L., et al. (2018). Aortic Plaque-Targeted Andrographolide Delivery with Oxidation-Sensitive Micelle Effectively Treats Atherosclerosis via Simultaneous ROS Capture and Anti-inflammation. Nanomedicine Nanotechnol. Biol. Med. 14, 2215–2226. doi:10.1016/j.nano.2018.06.010
Yao, S. Y., Shen, M. L., Li, S. J., Wu, X. D., Zhang, M. M., Ma, L. N., et al. (2020). Application of a Mechanically Responsive, Inflammatory Macrophage-Targeted Dual-Sensitive Hydrogel Drug Carrier for Atherosclerosis. Colloids Surfaces B Biointerfaces 186, 110718. doi:10.1016/j.colsurfb.2019.110718
Zalba, G. (2021). Oxidative Stress in Vascular Pathophysiology: Still Much to Learn. Antioxidants 10, 673. doi:10.3390/antiox10050673
Zhao, Y., Gao, H., He, J., Jiang, C., Lu, J., Zhang, W., et al. (2018). Co-delivery of LOX-1 siRNA and Statin to Endothelial Cells and Macrophages in the Atherosclerotic Lesions by a Dual-Targeting Core-Shell Nanoplatform: A Dual Cell Therapy to Regress Plaques. J. Control. Release 283, 241–260. doi:10.1016/j.jconrel.2018.05.041
Keywords: stimuli-responsive drug release, smart drug delivery, biosensing drug carriers, light-activated drugs, atherosclerosis, ischemia-reperfusion, stroke, thrombosis
Citation: Genç H, Efthimiadou E and Cicha I (2022) On-Demand Drug Delivery: Recent Advances in Cardiovascular Applications. Front. Drug. Deliv. 2:913225. doi: 10.3389/fddev.2022.913225
Received: 05 April 2022; Accepted: 18 May 2022;
Published: 06 June 2022.
Edited by:
Chih-Chia Huang, National Cheng Kung University, TaiwanReviewed by:
Tsung-Lin Yang, Taipei Medical University Hospital, TaiwanCopyright © 2022 Genç, Efthimiadou and Cicha. This is an open-access article distributed under the terms of the Creative Commons Attribution License (CC BY). The use, distribution or reproduction in other forums is permitted, provided the original author(s) and the copyright owner(s) are credited and that the original publication in this journal is cited, in accordance with accepted academic practice. No use, distribution or reproduction is permitted which does not comply with these terms.
*Correspondence: Iwona Cicha, SXdvbmEuQ2ljaGFAdWstZXJsYW5nZW4uZGU=
†ORCID: Iwona Cicha, orcid.org/0000-0002-7399-5307
Disclaimer: All claims expressed in this article are solely those of the authors and do not necessarily represent those of their affiliated organizations, or those of the publisher, the editors and the reviewers. Any product that may be evaluated in this article or claim that may be made by its manufacturer is not guaranteed or endorsed by the publisher.
Research integrity at Frontiers
Learn more about the work of our research integrity team to safeguard the quality of each article we publish.