- 1Department of Mechanical Engineering, University of AB, Edmonton, AB, Canada
- 2Proveris Scientific Corporation, Hudson, MA, United States
To date, in vitro estimation of doses delivered by an inhaler to the different major regions of the lung has required combining particle size measurements of the inhaled aerosol with in silico deposition models. Such a two step process is labor and time intensive. Here, we describe instead the development of an apparatus that allows direct estimation of regional lung deposition by measurement of doses collected on purpose-built metal grid filters that mimic tracheobronchial deposition efficiency. Placing these filters downstream of the Alberta Idealized Throat and upstream of a final filter allows collection of doses depositing in the extrathoracic, tracheobronchial and alveolar regions. Artificial electrostatic deposition on the metal tracheobronchial filters is prevented by a custom inline electrostatic neutralizer. We use the resulting apparatus to estimate regional deposition with a variety of dry powder inhalers during realistic, time-varying inhalation maneuvers and three pMDIs with a constant flow rate of 30 l/min. These results are compared to those obtained with the traditional two step approach that combines cascade impaction with a regional deposition model. Good agreement is found between the two approaches, indicating that the present direct method may be an efficient, time-saving alternative method for in vitro estimation of regional lung doses.
Introduction
During development and evaluation of inhaled pharmaceutical aerosols, benchtop experiments that discriminate between the dose depositing in the extrathoracic region versus the dose delivered to the lungs are commonly used (e.g., Ciciliani et al., 2020; Miller et al., 2021). This is because different in vivo effects may be ascribed to aerosol deposition in these different regions. By a similar argument, in some cases it may also be useful to discriminate between the tracheobronchial versus alveolar components of the total lung dose. Until recently, the simplest in vitro procedure for separating the lung dose into its morphological components was to measure the aerosol size distribution, typically using multistage cascade impaction, and input this into a computer model that provides estimates of regional lung deposition for the given particle size distribution and chosen inhalation pattern (e.g., Ruzycki et al., 2020; Miller et al., 2021). This labor-intensive task is complicated by the need for a mixing inlet when using realistic breathing patterns. A recently developed, simpler and less laborious approach is described here that instead collects the aerosol downstream of an extrathoracic replica directly onto filters that separately mimic tracheobronchial versus alveolar deposition.
Designing a Filter That Mimics Tracheobronchial Deposition
An essential component of the present approach is a filter that correctly captures the dependence of tracheobronchial deposition on particle size and flow rate. While extrathoracic replicas and absolute filters are readily available and routinely used, such is not the case for filters that capture tracheobronchial deposition. In particular, for inhaled pharmaceutical aerosols, the tracheobronchial region has much lower deposition efficiency than commercially available filters. Previous authors (Koehler and Volckens 2013) have used polyurethane foams and nylon meshes to mimic regional lung deposition curves of the ICRP model (1994). However, their apparatus was designed for use at a constant flow rate of 16.7 l/min, whereas inhalers are commonly tested at much higher, time varying flow rates. In addition, recovery of pharmaceutical ingredients from filters typically involves rinsing with organic solvents that can damage the organic filter media used by Koehler and Volckens (2013), besides interfering spectroscopically when assaying small quantities.
One solution to the above issues involves the design of custom stainless steel filter media. Aerosol filters present many small scale obstacles to a particle-containing air flow, often by having the air flow through a thin three-dimensional layer of fibers. The air flows around the fibers, while aerosol particles deposit on the fibers by one or more of the following mechanisms: impaction, interception, diffusion, sedimentation, electrostatics. Filter design involves choosing fiber diameters and fiber spacings to yield the desired filtration efficiency for flow rates, particle diameters and other parameters in the range of interest. This is normally done with the aid of single fiber filtration theory (Hinds 1999). However, in our case, we wish to mimic the filtration efficiency of the tracheobronchial region, which involves filter parameter values outside the range of existing single fiber theory equations (Tavernini et al., 2019). Thus, we instead used the computational fluid dynamics (CFD) package OpenFOAM (version 5.0, Bracknell, Berkshire, United Kingdom) to simulate the flow and particle motion through single layers of wire meshes. CFD was performed for particle diameters in the range of 0.5–10 μm, meshes with wire diameters of 20 and 28 μm with 30.5, 38.1, and 50.8 μm diameter openings, fluid velocities of 0.05–1.55 m/s, and a numerical grid of 1.9–3.2 million cells (see Tavernini et al., 2019 for further details). An empirical curve fit to the CFD results was then used to explore filtration efficiencies for multiple layers of wire meshes for a variety of filter designs. Predicted filtration was compared to average human tracheobronchial deposition given by various different authors (Stahlhofen et al., 1989; ICRP 1994; Javaheri et al., 2013; Asgharian et al., 2001). Using this approach, a two layer filter design with an upstream layer having 500 wires per inch and each wire having a diameter of 25 μm (i.e. 500 × 25 μm mesh), with the second layer consisting of 400 × 30 μm mesh was found to be optimal in mimicking deposition in the human tracheobronchial region for the parameter values noted above.
Part of the present filter design process included ensuring that filter efficiency was unaffected by time varying flow rates, thereby allowing the filter to correctly mimic tracheobronchial deposition when realistic inhalation breathing flows occur directly through the filter. This involved examination of the Reynolds and Strouhal numbers, and ensuring values were small enough to make unsteady flow effects negligible (Tavernini et al., 2021).
Experimental Measurement of Filtration Efficiency
The above noted filter design was built and its filtration efficiency measured experimentally using the setup shown in Figure 1. These experiments involved supplying nebulized jojoba oil aerosols from a plenum and comparing the signals from an Electrical Low Pressure Impactor (ELPI) when exposed to the test aerosol with and without the filter element. The aerosol was inhaled across the filter with various unsteady tidal breathing patterns supplied by an ASL 5000 Breathing Simulator (IngMar Medical, Pittsburgh, PA, United States), as well as various constant flow rates supplied by a vacuum pump. Comparing the signal received from the ELPI with and without the filter element allowed determination of the filtration efficiency for each particle size bin of the ELPI.
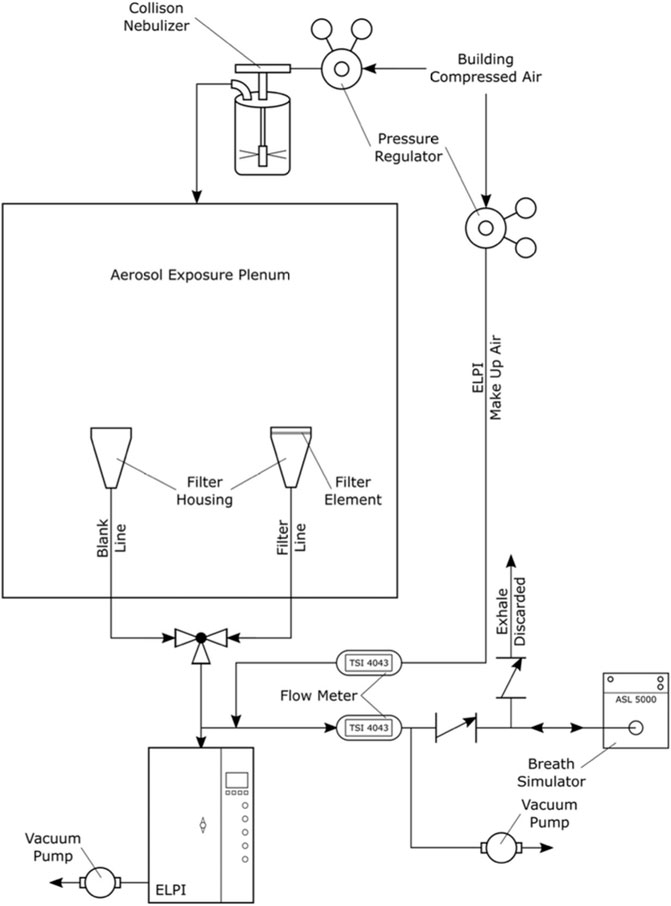
FIGURE 1. Schematic of experimental measurement to determine filtration efficiency of filter media. Used with permission from Tavernini et al. (2019).
Figure 2 shows measured filtration for the two layer stainless steel filter noted above, with both layers supplied by ASADA MESH Co. (Osaka, Japan), compared to that predicted by various theoretical deposition models for various breathing patterns. Data obtained at constant flow rates differed negligibly from the corresponding unsteady breathing patterns having the same average flow rate (Tavernini et al., 2019), indicating the filtration efficiencies were unaffected by unsteady flow rates, as was intended in their design. Good agreement is also seen between the measured filtration of the filter and the average tracheobronchial deposition values predicted by the various sources noted in Figure 2, particularly considering the large intersubject variability that is known to occur among the approximately 130 combined subjects of the in vivo scintigraphy studies used to validate the theoretical deposition models (Stahlhofen et al., 1989).
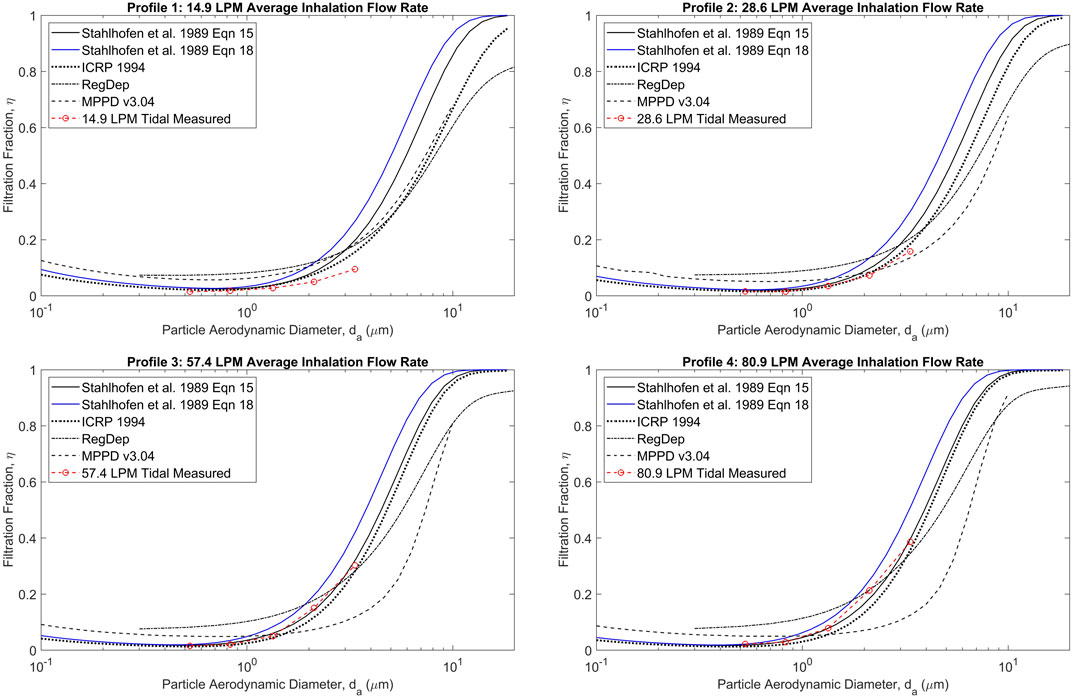
FIGURE 2. Measured filtration efficiency of jojoba oil test aerosols for the two layer stainless steel mesh filter compared with predicted values from various sources (Stahlhofen et al., 1989; ICRP 1994; RegDep from; Javaheri et al., 2013; MPPD v3.04 from; Asgharian et al., 2001) for a range of particle sizes and breathing patterns. Reprinted from Tavernini et al. (2019) with permission.
Benchtop Measurement of Regional Deposition With Inhalers
Having demonstrated the ability of the mesh filter to adequately mimic average tracheobronchial deposition with laboratory generated test aerosols, we proceeded to similarly examine its behavior with aerosols generated by various marketed inhalers. For this purpose, several additional considerations were necessary. First, when placing a filter immediately downstream of an extrathoracic replica, alterations to the filter intake flow path must be made to prevent the laryngeal jet from causing large differences in velocity at different locations across the face of the filter. Secondly, lumen diameters in the tracheobronchial region are typically more than a few millimeters, while the widths of the channels of the filter are less than 0.1 mm. Thus, the distance to an electrically conducting surface is many times lower for aerosol particles passing through the filter than in human conducting airways, which could artificially enhance electrostatic deposition on the filter in the case of sufficiently charged particles. Since electrostatic deposition is expected to be negligible in the human tracheobronchial region with pharmaceutical inhaler aerosols (Tavernini et al., 2021), a method for neutralizing the aerosol just prior to passing through the tracheobronchial filter is needed. Finally, large particles (e.g., 10 μm or larger in diameter) that occur in some dry powder inhaler formulations could bounce through the extrathoracic region and artificially increase the dose depositing on the tracheobronchial filter.
To address the above noted issues, a custom bipolar ion source was integrated with a liquid filled collection dish that served to both collect large particles and disrupt the laryngeal jet (Tavernini et al., 2021). The addition of electrostatic neutralization via a high voltage corona ionization source necessitated the use of an annular design for the filter region. Figure 3 shows the final design of the combined flow disruptor, large-particle collection dish and charge neutralizer apparatus. The voltage drop between the inner and outer electrodes was powered by 120 V AC, stepped up to 5 kV by a transformer, which produced an alternating bipolar corona discharge between the outer and inner electrodes. The collection dish was filled with a small amount of solvent to prevent particle bounce.
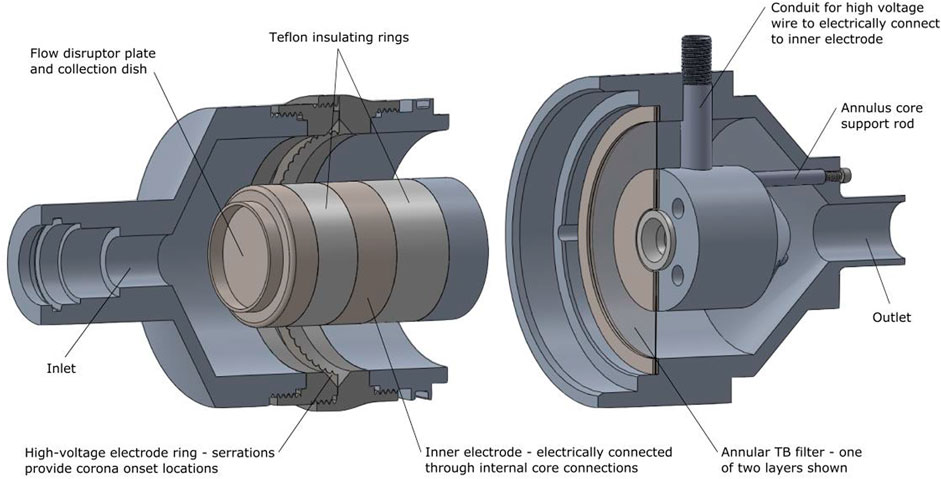
FIGURE 3. A schematic of the combined flow disruptor/large particle collection dish and aerosol neutralizer (left) is shown placed on its side and pulled apart from its downstream section that houses the tracheobronchial filter mimic (right). Reprinted from Tavernini et al. (2021) by permission from Springer Publishing.
The apparatus shown in Figure 3 was placed immediately downstream of an Alberta Idealized Throat (AIT). A final absolute filter downstream of the TB Filter housing collected an estimate for the alveolar dose. Four dry powder inhalers (Onbrez Breezhaler, indacaterol maleate, 97 μg; Flovent Diskus, fluticasone propionate, 250 μg; Pulmicort Turbuhaler, budesonide, 200 μg; Asmanex Twisthaler, mometasone furoate, 400 μg) were connected to the AIT and individual breathing patterns representative of those expected in vivo based on inhaler resistance (Delvadia et al., 2016) with each device were actuated by a custom-built breathing machine. Active pharmaceutical ingredient was rinsed from the AIT, the tracheobronchial filter, and alveolar filter and assayed by UV spectrophotometry. The same inhalers were also tested with the filters, flow disruptor and neutralizer instead replaced by a Next Generation Impactor (NGI). The particle size distribution measured by the NGI was then input into the regional lung deposition model of Javaheri et al. (2013) to predict tracheobronchial and alveolar doses to allow comparison to doses collected by the filters. Figure 4 shows the experimental setup for the two sets of measurements.
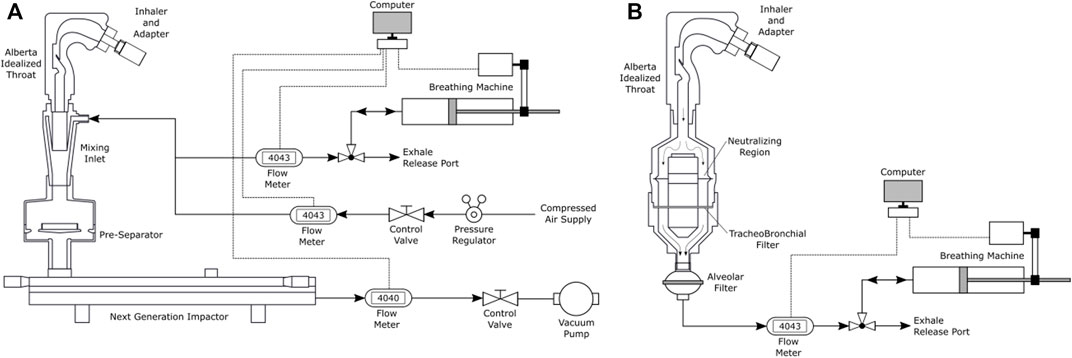
FIGURE 4. (A) Schematic of apparatus used here for the traditional two-step approach for determining regional deposition, involving cascade impaction with a mixing inlet during breath simulation followed by regional deposition estimation via in silico modeling. (B) Schematic of direct regional deposition filter measurement setup. Reprinted from Tavernini et al. (2021) by permission from Springer Publishing.
A similar set of experiments and calculations were performed with three metered dose inhalers (Ventolin HFA, salbutamol, 100 μg; QVAR, beclomethasone dipropionate, 80 μg; Asmanex HFA, mometasone furoate, 100 μg) with a constant inhalation flow rate of 30 l/min supplied by a vacuum pump, rather than the breathing machine shown in Figure 4. A constant flow rate was used with the pMDIs to avoid the need for robotic timing actuation equipment when a realistic breath pattern is used. In addition, for pMDI testing the inhalers were inserted into the extrathoracic replica with their mouthpieces horizontal (i.e. “transverse” rather the “coaxial” position shown in Figure 4 for the dry powder inhalers), since for high momentum pMDI aerosols Ruzycki et al. (2020) found the transverse position with the Alberta Idealized Throat improved agreement with in vivo extrathoracic deposition data.
Regional Deposition Estimates With Inhalers
Results of experiments performed using the methods described above are shown in Figure 5. Good agreement is seen between the two different approaches. Differences between the two approaches seen in Figure 5 may be primarily due to particle size dependent differences between the deposition efficiencies obtained with the tracheobronchial filter versus values predicted by the RegDep (Javaheri et al., 2013) in silico deposition model For example, as is seen in Figure 2, data points measured with the filter are somewhat lower than those predicted with RegDep for small particle diameters, but are higher at large particle sizes. Such differences are similar to the differences between different in silico deposition models (also seen in Figure 2), but these differences are considerably smaller than intersubject variability (Stahlhofen et al., 1989). Given these known differences and variations in regional deposition values, the present filter approach appears to provide acceptable estimates of average regional deposition.
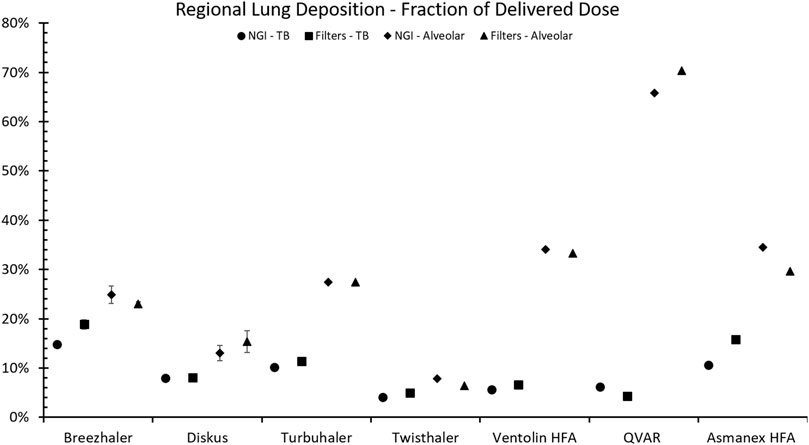
FIGURE 5. Comparison of amounts predicted to deposit in the tracheobronchial (TB) and alveolar regions using NGI impactor data combined with in silico regional deposition modeling (Javaheri et al., 2013) versus values collected directly on filters for four different DPIs operated with realistic breath patterns plus three pMDIs operated at a constant flow rate of 30 l/min. Error bars indicate standard deviation (n = 5) and are shown only when they are larger than the datapoint symbols.
Conclusions
We have developed an alternative benchtop approach to estimating regional deposition in the human respiratory tract, whereby custom designed metal filters are used to estimate tracheobronchial deposition. An upstream extrathoracic replica captures deposition there, and a final downstream filter captures the dose to the alveolar region. Assaying drug in the three collection regions is considerably simpler and less labor intensive than using a cascade impactor, and does not require an in silico model to predict regional lung deposition. Furthermore, no mixing inlet is required when using realistic breathing patterns. In spite of its relative simplicity, the filter approach gives tracheobronchial and alveolar deposition estimates that are in good agreement with data obtained by the more involved cascade impactor-in silico modeling approach, when applied to the four DPIs and three pMDIs considered here.
Data Availability Statement
The raw data supporting the conclusions of this article will be made available by the authors, without undue reservation.
Author Contributions
WF wrote the first draft of the manuscript, cosupervised ST and gave research and design input. DF supplied research guidance, arranged technical support, edited the manuscript and supplied supervisory support. ST implemented and performed the laboratory experiments, CFD simulations, analyzed the raw data, edited the manuscript and created the figures. AM cosupervised ST, edited the manuscript and gave research and design input.
Funding
This research was financially supported by Proveris Scientific Corporation and the Natural Sciences and Engineering Research Council of Canada (NSERC).
Conflict of Interest
Patents have been filed on the technology described in this work, which may financially benefit the authors. Author DF is employed by Proveris.
The remaining authors declare that the research was conducted in the absence of any commercial or financial relationships that could be construed as a potential conflict of interest.
Publisher’s Note
All claims expressed in this article are solely those of the authors and do not necessarily represent those of their affiliated organizations, or those of the publisher, the editors and the reviewers. Any product that may be evaluated in this article, or claim that may be made by its manufacturer, is not guaranteed or endorsed by the publisher.
Acknowledgments
The authors thank Milad Kiaee for assistance with computational fluid dynamics investigation of the filter design, and ASADA Mesh Company for supplying materials to construct the filter elements. Patents have been filed on the technology described in this work, which may financially benefit the authors.
References
Asgharian, B., Hofmann, W., and Bergmann, R. (2001). Particle Deposition in a Multiple-Path Model of the Human Lung. Aerosol Sci. Technology 34 (4), 332–339. doi:10.1080/02786820119122
Ciciliani, A.-M., Denny, M., Langguth, P., Voshaar, T., and Wachtel, H. (2020). Lung Deposition Using the Respimat Soft Mist Inhaler Mono and Fixed-Dose Combination Therapies: An In Vitro/In Silico Analysis. COPD: J. Chronic Obstructive Pulm. Dis. 18 (1), 91–100. doi:10.1080/15412555.2020.1853091
Delvadia, R. R., Wei, X., Longest, P. W., Venitz, J., and Byron, P. R. (2016). In VitroTests for Aerosol Deposition. IV: Simulating Variations in Human Breath Profiles for Realistic DPI Testing. J. Aerosol Med. Pulm. Drug Deliv. 29 (2), 196–206. doi:10.1089/jamp.2015.1215
Hinds, W. C. (1999). Aerosol Technology: Properties, Behavior, and Measurement of Airborne Particles. 2nd ed. New York: John Wiley and Sons.
International Commission on Radiological Protection (1994). Human Respiratory Tract Model for Radiological protection: A Report of a Task Group of the International Commission on Radiological Protection. Oxford: Pergamon.
Javaheri, E., Shemirani, F. M., Pichelin, M., Katz, I. M., Caillibotte, G., Vehring, R., et al. (2013). Deposition Modeling of Hygroscopic saline Aerosols in the Human Respiratory Tract: Comparison between Air and Helium-Oxygen as Carrier Gases. J. Aerosol Sci. 64, 81–93. doi:10.1016/j.jaerosci.2013.04.010
Koehler, K. A., and Volckens, J. (2013). Development of a Sampler to Estimate Regional Deposition of Aerosol in the Human Respiratory Tract. Ann. Occup. Hyg. 57 (9), 1138–1147. doi:10.1093/annhyg/met041
Miller, D. P., Tarara, T. E., and Weers, J. G. (2021). Targeting of Inhaled Therapeutics to the Small Airways: Nanoleucine Carrier Formulations. Pharmaceutics 13 (11), 1855. doi:10.3390/pharmaceutics13111855
Ruzycki, C. A., Murphy, B., Nathoo, H., Finlay, W. H., and Martin, A. R. (2020). Combined In Vitro-In Silico Approach to Predict Deposition and Pharmacokinetics of Budesonide Dry Powder Inhalers. Pharm. Res. 37, 209. doi:10.1007/s11095-020-02924-7
Stahlhofen, W., Rudolf, G., and James, A. C. (1989). Intercomparison of Experimental Regional Aerosol Deposition Data. J. Aerosol Med. 2 (3), 285–308. doi:10.1089/jam.1989.2.285
Tavernini, S., Farina, D. J., Martin, A. R., and Finlay, W. H. (2021). Using Filters to Estimate Regional Lung Deposition with Dry Powder Inhalers. Pharm. Res. 38, 1601–1613. doi:10.1007/s11095-021-03082-0
Keywords: in vitro, conducting airways, regional, lung, deposition
Citation: Finlay WH, Farina DJ, Tavernini S and Martin AR (2022) In Vitro Estimation of Tracheobronchial and Alveolar Doses Using Filters. Front. Drug. Deliv. 2:901289. doi: 10.3389/fddev.2022.901289
Received: 21 March 2022; Accepted: 12 April 2022;
Published: 04 May 2022.
Edited by:
Zhenbo Tong, Southeast University, ChinaReviewed by:
Xiaole Chen, Nanjing Normal University, ChinaYan Cui, Huazhong University of Science and Technology, China
Copyright © 2022 Finlay, Farina, Tavernini and Martin. This is an open-access article distributed under the terms of the Creative Commons Attribution License (CC BY). The use, distribution or reproduction in other forums is permitted, provided the original author(s) and the copyright owner(s) are credited and that the original publication in this journal is cited, in accordance with accepted academic practice. No use, distribution or reproduction is permitted which does not comply with these terms.
*Correspondence: Warren H. Finlay, d2FycmVuLmZpbmxheUB1YWxiZXJ0YS5jYQ==