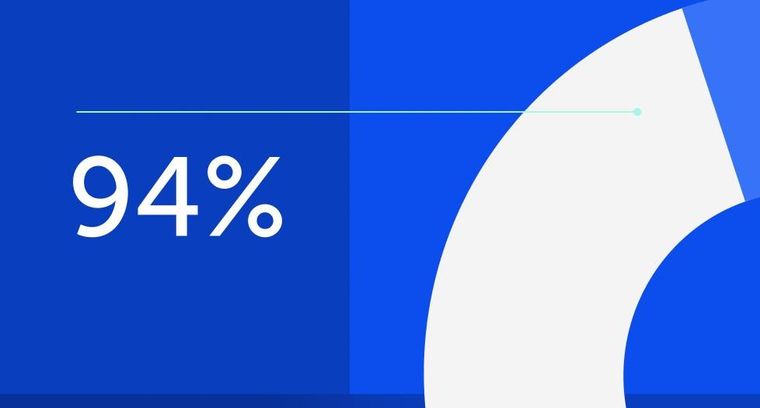
94% of researchers rate our articles as excellent or good
Learn more about the work of our research integrity team to safeguard the quality of each article we publish.
Find out more
REVIEW article
Front. Drug Deliv., 13 May 2022
Sec. Ophthalmic Drug Delivery
Volume 2 - 2022 | https://doi.org/10.3389/fddev.2022.899260
This article is part of the Research TopicOcular gene therapy: where we are and where to go?View all 4 articles
Retinal diseases lead to severe vision loss and are currently a major cause of vision impairment in industrialized countries. The significant number of genetic defects of the retina underlying these disorders, coupled to the absence of effective treatments, require new therapeutic solutions. Recent gene therapy developments in the field of ophthalmic research reveal the great potential of this approach. In recent years, non-viral vectors have been extensively studied due to their properties such as large gene packaging capacity and low immunogenicity. Hitherto, their development and optimisation for retinal gene therapy have been hindered by their inability to directly target retinal cells. The goal of this review is to summarize the most promising strategies to direct non-viral vectors for retinal cells to avoid off-target effects and promote their specific uptake, gene expression and overall efficiency.
Gene therapy, as initially defined by Friedmann and Roblin, refers to the delivery of exogenous genetic material into target cells to manipulate or modify the expression of a gene (Friedmann and Roblin, 1972). Significant advancements have been made in the field of gene therapy since the first clinical trial in the early 1990s, in particular for the treatment of eye diseases (Blaese et al., 1995), as the eye has several features that make it an ideal target for respect to gene therapy (Lee et al., 2019). First, it is easily accessible, and its compartmentalized anatomy allows for the targeting of various ocular tissues by altering the administration route. Furthermore, the blood-ocular barrier prevents systemic exposure of most drugs administered in the eye, conferring it an immune-privileged status (Niederkorn et al., 1981; Sonoda and Streilein, 1992). Recent developments in genomics and in the understanding of disease mechanisms have led to identification of new genes and mutations responsible for inherited and acquired eye diseases (Tan et al., 2021). To date, more than 300 genetic loci and 200 relevant genes associated with retinal diseases in humans have been identified (RetNet, 2022). Additionally, there are well-characterised animal models to study and develop new therapeutic strategies for several of these mutations. All these aspects confer the eye unique advantages as a relevant model system and target organ for gene therapy. Nevertheless, the eye has several dynamic and static barriers that difficult the delivery of transgenes to targeted ocular tissues, in particular the retina, which requires localised delivery (Patel et al., 2013; Suri et al., 2020). Subretinal and intravitreal injection are the most common routes of administration (Figure 1), and while the first is more technically challenging and targets the retinal pigment epithelium (RPE) and photoreceptors, the second is easier to perform and targets mostly the inner retina (Ziccardi et al., 2019).
FIGURE 1. Schematic representation of the common administration routes for gene therapies in the human eye. Created with BioRender.com.
Successful gene therapy requires an efficient and safe carrier to deliver the exogenous genetic material into target cells (Mulligan, 1993). The adeno-associated viruses (AAV) are among the most commonly used vectors due to their long-term transgene expression in diverse retinal cells (Liu et al., 2011). There are a variety of AAV-based gene delivery systems that have been developed and entered clinical practice (Maguire et al., 2019) but, despite their success, they present a major limitation: they can only carry genes up to 4.5 kb. Hence, for larger sized genes, alternatives are needed.
Non-viral vectors for ocular gene delivery have emerged as an alternative to viral vectors. Although they are still behind their viral counterparts in terms of efficiency, efforts have been made in vector design to optimize transgene expression (Conley et al., 2008). The safety profile and higher packaging capacity of genetic cargo of these systems confer them an advantage for gene therapy applications. Moreover, non-viral vectors are easier to manufacture on a large scale and hence more cost-effective than viral ones.
Non-viral vectors include gene delivery by both chemical carriers and physical methods (Ramamoorth and Narvekar, 2015). The first group can be divided into inorganic particles, polymeric (polyplexes), lipid-based (lipoplexes) and peptide-based particles (Midoux et al., 2009; Choi et al., 2013; Rajala et al., 2014; Hadianamrei and Zhao, 2022). These chemical systems have the advantage of being easily manipulated to change their properties depending on the target and purpose. Physical methods, on the other hand, rely on physical force to facilitate the intracellular delivery of genetic material and comprise, among others, electroporation, sonoporation, ballistic DNA injection and naked DNA delivery through needle injection (Yang et al., 1990; Heller et al., 2005; Newman and Bettinger, 2007; Gascón et al., 2013).
There are some challenges regarding the safety and efficiency of gene therapies that must be addressed before they reach the clinic. A successful gene therapy largely depends on the vector ability to target specific cells to achieve maximal therapeutic effect with minimal off-target effects. In the retina, this point is particularly important given the need to reach a large number of cells using a minimum injection volume to minimize tissue damage (Flannery et al., 1997). Initially, the targeting strategies were studied for application in cancer therapies (Santana-Armas and Tros de Ilarduya, 2021), but recent advances are also broadening the range of options available for areas such as ophthalmology (Cai et al., 2008). Cell specificity can be achieved by using different strategies: 1) electroporation performed directly on the target tissue; 2) incorporation of specific peptide ligands into polymer and lipid-based particles; 3) conjugation of specific polymers with particles and 4) transcriptional targeting using specific promoters (Mahato, 1999; Heller et al., 2005; Kachi et al., 2006; Laradji et al., 2021) (Figure 2). All these approaches will be addressed throughout this review in the context of retinal diseases.
FIGURE 2. Schematic representation of non-viral vectors and targeting methods for gene therapy of the retina. Created with BioRender.com.
In the quest for the safest and most effective gene therapy approach, tissue-specific strategies to avoid possible side effects of the expressed transgene play an important role. The main strategies to confer cell-specific expression of therapeutic transgenes to non-viral delivery methods, for retinal cells, will be addressed in this review.
The administration route of gene therapy vectors could be the difference between a non-targeted and a targeted delivery. For eye diseases several routes can be used ranging from systemic or topical administration to subconjunctival, intravitreal and subretinal injections (Figure 1). Depending on the disease and the affected ocular tissue, a different delivery route can be chosen to allow a more precise delivery of the genetic therapy.
The topical administration route is used mostly in the form of eye droplets to treat diseases of the anterior section of the eye as pre-corneal factors (solution drainage, blinking, among others) and anatomical barriers prevent the accessibility of the carriers to the posterior section of the eye (Gaudana et al., 2010). Delivery of SLN-based vectors to treat corneal inflammation have been achieved in mice; however, translation to the human eye may be hindered by the thickness of the human cornea (Vicente-Pascual et al., 2020; Amador et al., 2022).
In the last decade, intravitreal injection became one of the most used techniques to deliver therapeutics to the retina in clinical practice. It can be used to target the inner retina, optic nerve, and lens, however it has some drawbacks, as it can cause retinal detachment, haemorrhage, cataracts, and endophthalmitis (Gaudana et al., 2010). Notwithstanding, the injection of anti-VEGF (vascular endothelial growth factor) agents is the standard approach for treating retinal diseases such as diabetic retinopathy (DR) and age-related macular degeneration (Lai et al., 2015).
Subretinal injection is a very specific delivery technique that can be used to target cell types unreachable by any other delivery method, such as RPE and photoreceptors (PR) cells (Venkatesh et al., 2013; Fayyaz et al., 2020). Similar to other injection methods, it has some limitations: only a small volume can be injected, and there is an associated risk of haemorrhage and retinal detachment (Pierce and Bennett, 2015).
Electroporation is a physical method that uses short electric pulses to direct and deliver genetic material to tissues in a reversible manner by temporarily increasing the cell membrane permeability. This technique has been successfully used in neonatal and adult mice to deliver naked plasmids and non-viral vectors (Melo and Blackshaw, 2011; Nickerson et al., 2012). In combination with the different injection routes, specific cells such as photoreceptors, RPE and retinal ganglion cells can be targeted. For instance, by combining a subretinal injection of genetic material with electroporation, photoreceptors or RPE can be targeted, depending on the direction in which the electric pulses are applied (Venkatesh et al., 2013). Although a powerful tool in animal models, electroporation has a limited use in human clinical practice. In the posterior segment of the eye, specifically the retina, electroporation requires a more invasive surgery for placement of the electrodes, as proximity to the target cells is needed to produce a localized and effective electric field. This creates a challenge to translate electroporation from preclinical studies in rodents to human clinical practice. That being said, there is an active clinical trial (NCT03308045) to assess safety and tolerability of electroporation in establishing the ciliary muscle as a biofactory of therapeutic proteins, in which non-infectious uveitis (NIU) patients are being electroporated in the ciliary muscle with a plasmid encoding a chimeric TNF-α soluble receptor (hTNFR-Is) (Bloquel et al., 2006).
An interesting approach to promote cell-specific targeting is the use of small ligand molecules such as peptides and sugars. Targeting ligands have been used to direct genetic material to cancer and neuronal cells, and less extensively in other cell types, such as retinal cells. Hence, lessons can be learnt from the conjugation of non-viral vectors with ligands to target cancer cells, which is paramount to avoid off-target consequences of cancer therapies. An example is the conjugation of the poly (amidoamine) (PAMAM) dendrimer with transferrin, whose receptor is overexpressed in cancer cells. Urbiola et al. observed that an incorporation of 50% of transferrin led to an increase in transfection activity in CT26, HeLa, and HepG2 cancer cell lines (Urbiola et al., 2015). Another example is the overexpression of interleukin-12 (IL-12) which has proven to be a potent inducer of anti-tumour immunity (Harada et al., 1998). As demonstrated by Kim et al., in BALB/c mice injected with CT26 carcinoma cells, that mannose-coated chitosan polyplexes carrying the IL-12 gene were able to suppress tumour growth (Kim et al., 2006), thus confirming the potential of this approach for cellular targeting by non-viral vectors.
Interestingly, Kwon et al. observed that the electrostatic uptake of synthetic polymers such as polyethylenimine (PEI) was reduced in neuron-like cells in comparison with undifferentiated cells. To improve the uptake of PEI by neuronal cells, a 12-mer peptide, Tet1, possessing a C-terminal cysteine which has been described to have binding properties like the tetanus toxin, was covalently linked to PEI. Tet1-binding PEI polyplexes were able to improve gene expression levels in differentiated neuron-like PC-12 cells in comparison to unbound polyplexes (Kwon et al., 2008). In another study, the nontoxic Hc fragment of the tetanus toxin (TeNT) was shown to bind to presynaptic motor neuron terminals when injected intramuscularly (Price et al., 1975). Hence, Knight et al. used this knowledge to covalently bind TeNT peptide to polylysine (PLL), and TeNT-binding PLL polyplexes were able to target neuronal-derived cell lines in vitro (Knight et al., 1999).
In the retina, the use of ligands to target RPE cells is still limited to a small number of molecules. Folic acid or folate, one of the B vitamins, has been shown to interact specifically to RPE cells in the eye via folate-receptors (Bridges et al., 2002). Based on this interesting finding, binding folic acid to drug delivery carriers has been studied to target RPE cells. Suen and Chau demonstrated that folate-carriers had the capacity to deliver hydrophobic drugs in a prolonged and targeted delivery to RPE cells (Suen and Chau, 2013). These studies, although performed with pharmacological agents, support the hypothesis that folic acid can potentiate the targeting of non-viral vectors to RPE cells.
Hyaluronic acid (HA) is one of the most studied polymers for drug and gene delivery in the eye (Oliveira et al., 2014; Martens et al., 2015; Huang et al., 2017). It is well established that HA is a ligand for CD44 receptors that are expressed in RPE (Liu et al., 1997) and Müller cells (Shinoe et al., 2010). CD44 receptors have been described to be overexpressed in most retinal pathologies, and this can potentially improve the specificity of a non-viral therapeutic approach (Kuhrt et al., 1997; Huang et al., 2017). Additionally, HA is a major component of the vitreous humour, supporting its biocompatibility and suitability as gene delivery vehicle. Martens et al. demonstrated that despite their negative surface charge, HA-coated polyplexes were able to be internalized and transfect ARPE-19 cells, which are a RPE cell line (Martens et al., 2015). Similarly, Oliveira et al. also showed that the chemical conjugation of HA with chitosan was able to efficiently transfect ARPE-19 cells (Oliveira et al., 2014). Hyaluronic acid is usually associated with a cationic polymer to produce polyplexes with a positive surface charge capable of interacting with cell membranes. However, this association of cationic and HA polymers possibly decreases the capacity of HA to interact with the CD44 receptor, as it might be less exposed. Therefore, the use of HA alone to produce polyplexes with the capacity to complex and protect DNA could potentiate the HA interaction with CD44 receptors. To test this hypothesis, Oliveira et al. performed chemical modifications in HA and observed that an incorporation of 10% positive charges in the HA polymer structure was not sufficient to produce polyplexes with the necessary gene therapy characteristics (Oliveira et al., 2016). These results seem to suggest that a higher modification efficiency may be required to produce polyplexes with desired physicochemical properties. Hyaluronic acid can also be used to coat other non-viral vectors such as lipoplexes (HA-LIP), as demonstrated by an in vitro and in vivo study on a retina light damage model. HA-LIP carrying siRNA targeting caspase-3 were able to inhibit the apoptosis cascade thus preventing retinal degeneration (Ribeiro et al., 2021).
The distribution of HA-coated polyplexes in bovine retinas was evaluated ex-vivo by Huang et al. and showed the presence of polyplexes in the inner limiting membrane, the ganglion cell layer (GCL) and the RPE up to 4 h after exposition (Huang et al., 2017). Additionally, it was shown that the HA-coated polyplexes accumulated in areas of the retina with cells known to be enriched with CD44 receptors, RPE (Liu et al., 1997) and Müller cells (Shinoe et al., 2010). Hyaluronan-modified core-shell liponanoparticles carrying an anti-inflammatory drug were also shown to accumulate in RPE cells when injected by intravitreal administration route in Lewis rats. Instead, chitosan polyplexes and liponanoparticles without HA were not able to reach the RPE (Gan et al., 2013). These experiments performed in different animal models strongly suggest the targeting capacity of hyaluronic acid (HA) to retinal pigment epithelium cells. Supporting these findings, it was shown that non-viral vectors like hyaluronic acid (HA) and polylactide (PLA), upon intravitreal delivery, tend to travel through the retina and enter specific cells (Sakurai et al., 2001; Bourges et al., 2003; Huang et al., 2017). In their studies, Devoldere et al. showed that HA/mRNA-complexes when delivered through intravitreal injection were able to reach and accumulate in the inner limiting membrane (ILM). This study has shown that HA improved the intravitreal mobility of mRNA-complexes, but these complexes were not able to cross the ILM (Devoldere et al., 2019b). This limitation could be attributed to differences in the ILM of different animal models, as it is known that the ILM of rodents has a more simplified structure than those of humans (Peynshaert et al., 2019). Addiitiionally, differences in HA-complex formulations regarding surface charge impact the crossing of the ILM, as negatively charged complexes are more likely to cross the ILM (Koo et al., 2012). Although the process by which they navigate the retina is not yet fully understood, these findings can be used to tailor non-viral vectors.
The retinal distribution of polymers has been studied to understand if these materials can be used as non-viral vectors to target retinal cells. Polylactide (PLA) based polyplexes loaded with rhodamine (Rh-6G) and Nile red fluorochromes injected through intravitreal administration were shown to accumulate in the rod outer segments and RPE layer in the first 24 h (Bourges et al., 2003). A similar behaviour was observed for polystyrene nanospheres under 2 μm in size. After 2 months the nanospheres were found in the neural retina and RPE of rabbits following an intravitreal injection (Sakurai et al., 2001). Bourges et al. suggested that a transretinal movement of polyplexes occurs in vivo. Possible explanations range from a rupture of the ILM physiological barrier due to the presence of polymers, inflammation after the injection and modification of the permeability in response to the activation of microglia (Bourges et al., 2003). However, this needs further addressing and evaluation for other polymers.
Nevertheless, it is widely accepted that polymer vectors lack the cellular tropism observed for some viral vectors and would therefore benefit from other targeting strategies as described below. Since the retina is a tissue separated from the systemic circulation by the blood-retina barrier, the degradation and excretion are limited. Therefore, a major characteristic to consider is the ability of a polymer to be biodegradable inside the retina. Polymers, such as hyaluronic acid, chitosan, and poly (lactic-co-glycolic acid) (PLGA), are examples of biodegradable and biocompatible polymers that have been extensively tested and reviewed for gene and drug delivery to the retina (Tsai et al., 2018; Lynch et al., 2019).
One of the molecular strategies used in vector design to increase protein production and control the expression of therapeutic genes by plasmid DNA is the incorporation of specific promoters into the expression cassette. Promoters are genetic regions involved in transcription initiation and some are only active in specific cell types (Zheng and Baum, 2008). These DNA regulatory elements could be used to regulate the level of transgene expression and restrict it to a specific tissue, thus reducing off-target effects. Furthermore, several regulatory DNA elements (enhancers, silencers or insulators) can be used in addition to promoters to increase cell-type specificity (Nicoud et al., 2007; Toscano et al., 2011). In initial stages of gene therapy, transgenes were under the control of viral promoters such as cytomegalovirus (CMV) or simian virus 40 (SV40), which have demonstrated capacity to promote gene expression (Boshart et al., 1985; Schmidt et al., 1990; Makrides, 2003). However, in recent years, mammalian promoters have emerged, especially in cancer gene therapy, to direct therapeutic genes towards target cells (Yoon et al., 2018; Rama Ballesteros et al., 2020; Yan et al., 2020).
In the ophthalmic field, some groups have reported promising results using mammalian promoters to increase cell-specific gene expression, as summarized in Table 1. These promoters are usually evaluated and selected using reporter genes such as green fluorescent protein (GFP) or luciferase (Kachi et al., 2006; Wang et al., 2020) and ideally, the chosen promoters, should meet two criteria: they must regulate an endogenous gene that is expressed exclusively in target cells, and must promote robust expression of the reporter gene in those cells (Aguirre, 2017).
Much of the research in this area focuses on targeting photoreceptors, as a vast number of human retinal degenerations are primarily associated with dysfunction and death of these cells. Regarding photoreceptor-targeted gene expression, opsin promoters have been extensively studied. The rhodopsin promoter has been shown to drive strong gene expression with viral (Flannery et al., 1997; Matsuda and Cepko, 2004; Nicoud et al., 2007) and non-viral (Zack et al., 1991; Apaolaza et al., 2016; Wang et al., 2016) vectors in photoreceptors, when administered both by subretinal and intravitreal administration. It was also reported that this promoter was able to direct the expression of genes carried by PEGylated liposome-based nanoparticles specifically to the retina after intravenous administration (Zhang et al., 2003). In addition, promoters of other genes such as rhodopsin kinase (GRK1), RP1 axonemal microtubule associated (RP1) and cGMP phosphodiesterase (PDE) have been demonstrated to induce expression of reporter genes in photoreceptor cells (Nicoud et al., 2007; Beltran et al., 2017). The use of other opsin promoters to direct expression to photoreceptors using non-viral vectors was also reported by Apaolaza et al. The authors used a solid lipid nanoparticle carrying the retinoschisin (RS1) gene under the control of a murine opsin promoter and administered it by intravitreal administration in Rs1-deficient mice. Interestingly, the expression driven by the opsin promoter was confined to photoreceptors and the improvement observed in the retina was higher using this promoter than the ubiquitous CMV promoter, demonstrating the relevance of a cell-specific strategy (Apaolaza et al., 2016).
In some retinal pathologies, an even more specific targeting to limit the transgene expression to just one type of photoreceptors is required. For example, in achromatopsia it is important to achieve cone-specific expression (Ye et al., 2016) and some studies have shown this possibility by using a cone opsin promoter (Wang et al., 2016; Ye et al., 2016; Khabou et al., 2018). When studying cell-specific promoters it is important to be cautious when extrapolating and applying results obtained in healthy wild-type retinas to mutants and between different species. The expression level of a regulated gene in normal animals may be altered in mutants (Aguirre et al., 2014). Aguirre et al. and Beltran et al for example, have shown that even though the GRK1 promoter directed gene expression exclusively to rods in normal canine retinas, in mutated ones this vector drove expression also in cones (Beltran et al., 2010; Aguirre, 2017). The variability of promoter specificity between species was demonstrated by Ye et al. when they tried to express transgenes carried by AAV vectors and regulated by cone-specific promoters in different animal models. Indeed, they showed that the IRBP/GNAT2 promoter induced a strong expression restricted to photoreceptors in mice and canines, while in primate cones it was ineffective (Ye et al., 2016). A possible explanation for this divergence is that the DNA sequence of the promoter may not have some regulatory elements needed for expression in primate cones.
The implication of the retinal pigment epithelium (RPE) genetic defects in several retinal degenerative diseases, such as retinitis pigmentosa, age-related macular degeneration or Stargardt disease suggest that RPE could be an interesting target to gene therapies (Dougherty et al., 2008; Sparrow et al., 2010). Thus, in recent years, many promoters have been evaluated for their ability to drive specific expression in these cells. Kachi et al. studied two mammalian promoters of RPE cells, a promoter fragment of the vitelliform macular dystrophy 2 gene (VMD2) and another of Rpe65 gene and verified an increased and sustained expression for at least 56 and 112 days, respectively. This suggests that in addition to achieving cell-specific expression, it is also possible to obtain sustained transgene expression with mammalian promoters (Kachi et al., 2006). Interestingly, VMD2 and Rpe65 promoters have been the preferential promoters used to reach RPE specific expression; despite the promising results with the Rpe65 promoter (Kachi et al., 2009, 2006), VMD2 was shown to have higher specificity (Wang et al., 2019). This promoter drove RPE specific transduction of the reporter gene in either viral (Kachi et al., 2009; Wang et al., 2019; Wu et al., 2021) or non-viral (Esumi et al., 2004; Kachi et al., 2006; Wang et al., 2016) vectors.
In the retina field, most retinal degenerations initially affect photoreceptors and underlying RPE, and thus many therapeutic studies have been focused on targeting these cells; however, there are retinal disorders involving retinal ganglion cells, Müller cells, and bipolar cells that could benefit from targeted gene therapies as well (Hellström and Harvey, 2011; Devoldere et al., 2019a; Hulliger et al., 2020).
Retinal ganglion cells (RGCs) are substantially affected in patients with glaucoma, and their degeneration has also been reported in other diseases such as diabetic retinopathy, retinitis pigmentosa, or even neurodegenerative diseases. Intravitreal injection seems to be the most suitable administration route when targeting RGCs, with Wang et al. testing liposome-protamine-DNA (LPD) nanoparticles to deliver genes to RGCs through this administration method. They showed that it is possible to achieve RGC-specific expression of a reporter gene under the control of the thymocyte antigen promoter (Thy-1.2) (Wang et al., 2016). Other promoters from the gamma-synuclein (SNCG) and neurofilament heavy chain (Nefh) genes were tested, and both promoters, when conveyed in AAV vectors, were shown to successfully induce gene expression limited to retinal ganglion cells (Wang et al., 2016; Chaffiol et al., 2017; Hanlon et al., 2017).
Currently, there is limited research on the specific targeting of Müller cells; however, the use of glial promoters has been shown to be a promising approach. Greenberg et al., for example, have shown that lentiviral vectors with the Cluster of Differentiation 44 (CD44), glial fibrillary acidic protein (GFAP) or vimentin promoters, led to targeted expression in Müller cells after subretinal injection, with CD44 achieving the highest expression level (Greenberg et al., 2007). The GFAP promoter has been the most studied in this context and several other groups have verified its effectiveness (Kuzmanovic et al., 2003; Dorrell et al., 2009; Aartsen et al., 2010; Prentice et al., 2011). In prior studies the Mlc1 (megalencephalic leukoencephalopathy with the subcortical cysts 1) and RLBP1 (Retinaldehyde-binding protein 1) promoters have also shown to be of interest as specific tools to obtain targeted expression on Müller cells (Pellissier et al., 2014; Danjo et al., 2022). Furthermore, encouraging results were obtained using a construct carrying the cellular retinaldehyde binding protein (CRALBP) promoter upstream of the gene that was introduced by subretinal injection and electroporation into the retina (Matsuda and Cepko, 2004).
Since much of the early-stage processing of visual inputs occurs in retinal bipolar cells, their genetic modifications may be of value in various ophthalmic conditions. To reach these cells located in the inner nuclear layer of the retina, the promoter of glutamate metabotropic receptor 6 (Grm6), a gene expressed in these cells, has been studied. This promoter sequence was cloned into lentiviral vectors and shown to direct expression to bipolar cells when subretinal (Cronin et al., 2014) and intravitreal (Lu et al., 2016) administration were used. Cepko’s group has also obtained encouraging results in these cells by using a non-viral strategy combined with the calcium binding protein 5 (CABP5) promoter (Matsuda and Cepko, 2004).
Most recently, biotechnological advances created synthetic promoters capable of spatial and temporal control of transgene expression. This new approach has been used to enhance specificity for retinal cells. Jüttner et al., for example, have developed a library of 230 AAVs, each with a different synthetic promoter and showed that 14% of those drove successful targeting in mice (Jüttner et al., 2019). In their collection of promoters, it is possible to find some capable of targeting human cones (ProA7) or ganglion cells (ProC29), for example, which could be used to express optogenetic tools (Jüttner et al., 2019). For RPE cells, Johari et al presented a synthetic promoter that exhibited higher expression levels than VMD2 gene promoter in vitro (Johari et al., 2021).
Patients with retinal diseases would benefit greatly from the implementation of cell-specific promoters in gene therapy. The combination between different strategies could be relevant for developing and translating retinal gene therapies into clinics. In theory, if the use of a specific promoter is coupled with one of the previously mentioned targeting strategies, the overall efficacy of the therapy could be improved while reducing undesirable gene expression.
To increase the targeting capability of a non-viral vector, a combined strategy may be required to obtain an efficient and precise gene delivery to the retina. This can be achieved by combining a specific promoter or a targeting peptide with an adequate delivery route for non-viral vectors. Rajala et al. modified liposome-protamine-DNA (LPD) polyplexes with nuclear localization signal (NLS) and trans-activator of transcription (TAT) peptides, in combination with the CMV promoter and subretinal administration (Rajala et al., 2014). The authors have shown that when injected with these modified LPD polyplexes the RPE layer of BALB/c mice showed a strong green fluorescent protein (GFP) expression which was not observed in other retinal layers, thus confirming that RPE can be targeted. In another study, using a mouse model of retinal disease—the RPE65 KO mice—the expression of RPE65 LPD polyplexes-derived was able to reduce cone cell death and partially recover the function of photoreceptors, as observed in the electroretinogram (ERG) analysis (Rajala et al., 2014). In a follow-up and extended study, LPD polyplexes were combined with promoters from either mouse rhodopsin (rod photoreceptors), human red/green opsin (cone photoreceptors), thymocyte antigen (retinal ganglion cells), or vitelliform macular dystrophy (RPE cells). The injected eyes of the BALB/c mice presented a cell specific expression of GFP for the rod and cone photoreceptors, as well as ganglion and RPE cells. Additionally, LPD polyplexes did not affect the morphology of the retina and no inflammation was observed (Wang et al., 2016).
As in classical pharmacological approaches, the specific targeting of gene therapy approaches to certain tissues and cells is required to reduce undesired off-target effects. In this review we present an overview of targeting strategies used in the gene and drug delivery field using specific promoters, physical methods, polymer-specific characteristics and targeting ligands.
Overall, promoters have shown to be a viable and relatively simple option to restrict gene expression to retinal specific cells, to increase the expression of the protein of interest and to avoid unwanted effects on other cells; however, the knowledge of the elements of the promoter responsible for achieving the required level of expression in target cells need to be considered (Zheng and Baum, 2008). It is also important to choose a promoter with high activity in selected cells, as some reports have shown that specific promoters have much lower activity than conventional ones (Chen et al., 2018). The use of these genetic regions requires a prior careful evaluation in specific expression systems to understand if there are interactions between the promoter and the remaining genetic material of the vector, as this could affect their activity and specificity. It is also important to assess the vector activity in vitro and in adequate animal models (Zheng and Baum, 2008). An important limitation of using specific promoters is that it does not work for every type of genetic material. For instance, siRNA, oligonucleotides and mRNA do not benefit from this approach, which limits its use to genes and shRNA encoded in DNA sequences. Although further research is needed to overcome these challenges and meet the requirements already discussed, great progress has been made regarding the specificity for retinal cells. Furthermore, this approach has shown great potential and could be a powerful tool in a clinical application of retinal gene therapy.
In contrast to tissue-specific promoters of the eye, targeting ligands for non-viral delivery to the retina are scarce. Most notably is the use of folic acid to direct drugs into RPE cells which can possibly be adapted to deliver genetic material (Bridges et al., 2002; Suen and Chau, 2013). Several ligands have been described for cancer and neuronal targeting by non-viral vectors, such as sugars (e.g. mannose) and peptides (e.g. transferrin). Hence, further research is needed to find specific ligands that could be used to promote the delivery of genetic material to specific cells of the retina such as the RPE and photoreceptors.
Additionally, the route of administration has a significant impact on the outcome of the therapeutic approach. The target tissue, off-target effects, and immune response are three important factors to consider when developing a non-viral vector. In the eye, the intravitreal route is more useful to target the inner retina, namely the ganglion and Müller cells (Gaudana et al., 2010; Lai et al., 2015), while the subretinal route can more effectively target photoreceptors and RPE cells (Venkatesh et al., 2013; Fayyaz et al., 2020). In combination with physical methods such as electroporation, the targeting of therapeutic vectors can be greatly improved.
In summary, an efficient, retina-specific non-viral vector could include a combination of the targeting strategies discussed in this review. This requires careful consideration of the most appropriate administration route, the coupling of a targeting ligand or the choice of a polymer specific for a target cell or tissue, and a specific promoter that allows cell-specific gene expression. Altogether, these strategies can make the efficiency of these non-viral vectors meet the clinical needs.
DB, CF, and GS wrote the manuscript. AMLO revised the manuscript. GS provided funding and revised the manuscript.
The authors acknowledge the financial support of Fundação para a Ciência e Tecnologia (PTDC/BTM/ORG/28121/2017 and EXPL/BTM-ORG/0500/2021 to GS). iNOVA4Health—UID/Multi/04462/2020, a program financially supported by Fundação para a Ciência e Tecnologia/Ministério da Educação e Ciência, through national funds and co-funded by FEDER under the PT2020 Partnership Agreement is also acknowledged.
The authors declare that the research was conducted in the absence of any commercial or financial relationships that could be construed as a potential conflict of interest.
All claims expressed in this article are solely those of the authors and do not necessarily represent those of their affiliated organizations, or those of the publisher, the editors and the reviewers. Any product that may be evaluated in this article, or claim that may be made by its manufacturer, is not guaranteed or endorsed by the publisher.
Aartsen, W. M., van Cleef, K. W. R., Pellissier, L. P., Hoek, R. M., Vos, R. M., Blits, B., et al. (2010). GFAP-driven GFP Expression in Activated Mouse Müller Glial Cells Aligning Retinal Blood Vessels Following Intravitreal Injection of AAV2/6 Vectors. PLOS ONE 5, e12387. doi:10.1371/journal.pone.0012387
Aguirre, G. D. (2017). Concepts and Strategies in Retinal Gene Therapy. Invest. Ophthalmol. Vis. Sci. 58, 5399–5411. doi:10.1167/iovs.17-22978
Aguirre, G. D., Genini, S., Komaromy, A. M., Guziewicz, K. E., and Beltran, W. A. (2014). Disease-based Promoter Selection for Retinal Gene Therapy. Investigative Ophthalmol. Vis. Sci. 55, 838.
Amador, C., Shah, R., Ghiam, S., Kramerov, A. A., and Ljubimov, A. V. (2022). Gene Therapy in the Anterior Eye Segment. Cgt 22, 104–131. doi:10.2174/1566523221666210423084233
Apaolaza, P. S., del Pozo-Rodríguez, A., Solinís, M. A., Rodríguez, J. M., Friedrich, U., Torrecilla, J., et al. (2016). Structural Recovery of the Retina in a Retinoschisin-Deficient Mouse after Gene Replacement Therapy by Solid Lipid Nanoparticles. Biomaterials 90, 40–49. doi:10.1016/j.biomaterials.2016.03.004
Beltran, W. A., Boye, S. L., Boye, S. E., Chiodo, V. A., Lewin, A. S., Hauswirth, W. W., et al. (2010). rAAV2/5 Gene-Targeting to Rods:dose-dependent Efficiency and Complications Associated with Different Promoters. Gene Ther. 17, 1162–1174. doi:10.1038/gt.2010.56
Beltran, W. A., Cideciyan, A. V., Boye, S. E., Ye, G.-J., Iwabe, S., Dufour, V. L., et al. (2017). Optimization of Retinal Gene Therapy for X-Linked Retinitis Pigmentosa Due to RPGR Mutations. Mol. Ther. 25, 1866–1880. doi:10.1016/j.ymthe.2017.05.004
Blaese, R. M., Culver, K. W., Miller, A. D., Carter, C. S., Fleisher, T., Clerici, M., et al. (1995). T Lymphocyte-Directed Gene Therapy for ADA − SCID: Initial Trial Results after 4 Years. Science 270, 475–480. doi:10.1126/science.270.5235.475
Bloquel, C., Bejjani, R. A., Bigey, P., Bedioui, F., Doat, M., BenEzra, D., et al. (2006). Plasmid Electrotransfer of Eye Ciliary Muscle: Principles and Therapeutic Efficacy Using hTNF‐α Soluble Receptor in Uveitis. FASEB J. 20, 389–391. doi:10.1096/fj.05-4737fje
Boshart, M., Weber, F., Jahn, G., Dorschhler, K., Fleckenstein, B., and Schaffner, W. (1985). A Very Strong Enhancer Is Located Upstream of an Immediate Early Gene of Human Cytomegalovirus. Cell 41, 521–530. doi:10.1016/S0092-8674(85)80025-8
Bourges, J.-L., Gautier, S. E., Delie, F., Bejjani, R. A., Jeanny, J.-C., Gurny, R., et al. (2003). Ocular Drug Delivery Targeting the Retina and Retinal Pigment Epithelium Using Polylactide Nanoparticles. Invest. Ophthalmol. Vis. Sci. 44, 3562–3569. doi:10.1167/iovs.02-1068
Bridges, C. C., El-Sherbeny, A., Ola, M. S., Ganapathy, V., and Smith, S. B. (2002). Transcellular Transfer of Folate across the Retinal Pigment Epithelium. Curr. Eye Res. 24, 129–138. doi:10.1076/ceyr.24.2.129.8167
Cai, X., Conley, S., and Naash, M. (2008). Nanoparticle Applications in Ocular Gene Therapy. Vis. Res. 48, 319–324. doi:10.1016/j.visres.2007.07.012
Chaffiol, A., Caplette, R., Jaillard, C., Brazhnikova, E., Desrosiers, M., Dubus, E., et al. (2017). A New Promoter Allows Optogenetic Vision Restoration with Enhanced Sensitivity in Macaque Retina. Mol. Ther. 25, 2546–2560. doi:10.1016/j.ymthe.2017.07.011
Chen, C., Yue, D., Lei, L., Wang, H., Lu, J., Zhou, Y., et al. (2018). Promoter-Operating Targeted Expression of Gene Therapy in Cancer: Current Stage and Prospect. Mol. Ther. - Nucleic Acids 11, 508–514. doi:10.1016/j.omtn.2018.04.003
Choi, S., Yu, X., Jongpaiboonkit, L., Hollister, S. J., and Murphy, W. L. (2013). Inorganic Coatings for Optimized Non-viral Transfection of Stem Cells. Sci. Rep. 3, 1567. doi:10.1038/srep01567
Conley, S. M., Cai, X., and Naash, M. I. (2008). Nonviral Ocular Gene Therapy: Assessment and Future Directions. Curr. Opin. Mol. Ther. 10, 456–463.
Cronin, T., Vandenberghe, L. H., Hantz, P., Juttner, J., Reimann, A., Kacsó, Á. E., et al. (2014). Efficient Transduction and Optogenetic Stimulation of Retinal Bipolar Cells by a Synthetic Adeno‐associated Virus Capsid and Promoter. EMBO Mol. Med. 6, 1175–1190. doi:10.15252/emmm.201404077
Danjo, Y., Shinozaki, Y., Natsubori, A., Kubota, Y., Kashiwagi, K., Tanaka, K. F., et al. (2022). The Mlc1 Promoter Directs Müller Cell-specific Gene Expression in the Retina. Trans. Vis. Sci. Tech. 11, 25. doi:10.1167/tvst.11.1.25
de Melo, J., and Blackshaw, S. (2011). In Vivo Electroporation of Developing Mouse Retina. JoVE 1715, e2847. doi:10.3791/2847
Devoldere, J., Peynshaert, K., De Smedt, S. C., and Remaut, K. (2019a). Müller Cells as a Target for Retinal Therapy. Drug Discov. Today 24, 1483–1498. doi:10.1016/j.drudis.2019.01.023
Devoldere, J., Wels, M., Peynshaert, K., Dewitte, H., De Smedt, S. C., and Remaut, K. (2019b). The Obstacle Course to the Inner Retina: Hyaluronic Acid-Coated Lipoplexes Cross the Vitreous but Fail to Overcome the Inner Limiting Membrane. Eur. J. Pharm. Biopharm. 141, 161–171. doi:10.1016/j.ejpb.2019.05.023
Dorrell, M. I., Aguilar, E., Jacobson, R., Yanes, O., Gariano, R., Heckenlively, J., et al. (2009). Antioxidant or Neurotrophic Factor Treatment Preserves Function in a Mouse Model of Neovascularization-Associated Oxidative Stress. J. Clin. Invest. 119, 611–623. doi:10.1172/JCI35977
Dougherty, C. J., Smith, G. W., Dorey, C. K., Prentice, H. M., Webster, K. A., and Blanks, J. C. (2008). Robust Hypoxia-Selective Regulation of a Retinal Pigment Epithelium-specific Adeno-Associated Virus Vector. Mol. Vis. 14, 471–480.
Esumi, N., Oshima, Y., Li, Y., Campochiaro, P. A., and Zack, D. J. (2004). Analysis of the VMD2 Promoter and Implication of E-Box Binding Factors in its Regulation. J. Biol. Chem. 279, 19064–19073. doi:10.1074/jbc.M309881200
Fayyaz, A., Ranta, V.-P., Toropainen, E., Vellonen, K.-S., Ricci, G. D. A., Reinisalo, M., et al. (2020). Ocular Intracameral Pharmacokinetics for a Cocktail of Timolol, Betaxolol, and Atenolol in Rabbits. Mol. Pharm. 17, 588–594. doi:10.1021/acs.molpharmaceut.9b01024
Fenner, B. J., Tan, T. E., Barathi, A. V., Tun, S. B. B., Yeo, S. W., Tsai, A. S. H., et al. (2021). Gene-Based Therapeutics for Inherited Retinal Diseases. Front. Genet. 12, 794805. doi:10.3389/fgene.2021.794805
Flannery, J. G., Zolotukhin, S., Vaquero, M. I., LaVail, M. M., Muzyczka, N., and Hauswirth, W. W. (1997). Efficient Photoreceptor-Targeted Gene Expression In Vivo by Recombinant Adeno-Associated Virus. Proc. Natl. Acad. Sci. U.S.A. 94, 6916–6921. doi:10.1073/pnas.94.13.6916
Friedmann, T., and Roblin, R. (1972). Gene Therapy for Human Genetic Disease? Science 175, 949–955. doi:10.1126/science.175.4025.949
Gan, L., Wang, J., Zhao, Y., Chen, D., Zhu, C., Liu, J., et al. (2013). Hyaluronan-modified Core-Shell Liponanoparticles Targeting CD44-Positive Retinal Pigment Epithelium Cells via Intravitreal Injection. Biomaterials 34, 5978–5987. doi:10.1016/j.biomaterials.2013.04.035
Gaudana, R., Ananthula, H. K., Parenky, A., and Mitra, A. K. (2010). Ocular Drug Delivery. AAPS J. 12, 348–360. doi:10.1208/s12248-010-9183-3
Greenberg, K. P., Geller, S. F., Schaffer, D. V., and Flannery, J. G. (2007). Targeted Transgene Expression in Müller Glia of Normal and Diseased Retinas Using Lentiviral Vectors. Invest. Ophthalmol. Vis. Sci. 48, 1844–1852. doi:10.1167/iovs.05-1570
Hadianamrei, R., and Zhao, X. (2022). Current State of the Art in Peptide-Based Gene Delivery. J. Control. Release 343, 600–619. doi:10.1016/j.jconrel.2022.02.010
Hanlon, K. S., Chadderton, N., Palfi, A., Blanco Fernandez, A., Humphries, P., Kenna, P. F., et al. (2017). A Novel Retinal Ganglion Cell Promoter for Utility in AAV Vectors. Front. Neurosci. 11, 521. doi:10.3389/fnins.2017.00521
Harada, M., Tamada, K., Abe, K., Yasumoto, K., Kimura, G., and Nomoto, K. (1998). Role of the Endogenous Production of Interleukin 12 in Immunotherapy. Cancer Res. 58, 3073–3077.
Heller, L. C., Ugen, K., and Heller, R. (2005). Electroporation for Targeted Gene Transfer. Expert Opin. Drug Deliv. 2, 255–268. doi:10.1517/17425247.2.2.255
Hellstrom, M., and R. Harvey, A. (2011). Retinal Ganglion Cell Gene Therapy and Visual System Repair. Cgt 11, 116–131. doi:10.2174/156652311794940746
Huang, D., Chen, Y.-S., and Rupenthal, I. D. (2017). Hyaluronic Acid Coated Albumin Nanoparticles for Targeted Peptide Delivery to the Retina. Mol. Pharm. 14, 533–545. doi:10.1021/acs.molpharmaceut.6b01029
Hulliger, E. C., Hostettler, S. M., and Kleinlogel, S. (2020). Empowering Retinal Gene Therapy with a Specific Promoter for Human Rod and Cone ON-Bipolar Cells. Mol. Ther. - Methods & Clin. Dev. 17, 505–519. doi:10.1016/j.omtm.2020.03.003
Johari, Y. B., Mercer, A. C., Liu, Y., Brown, A. J., and James, D. C. (2021). Design of Synthetic Promoters for Controlled Expression of Therapeutic Genes in Retinal Pigment Epithelial Cells. Biotech Bioeng. 118, 2001–2015. doi:10.1002/bit.27713
Jüttner, J., Szabo, A., Gross-Scherf, B., Morikawa, R. K., Rompani, S. B., Hantz, P., et al. (2019). Targeting Neuronal and Glial Cell Types with Synthetic Promoter AAVs in Mice, Non-human Primates and Humans. Nat. Neurosci. 22, 1345–1356. doi:10.1038/s41593-019-0431-2
Kachi, S., Binley, K., Yokoi, K., Umeda, N., Akiyama, H., Muramatu, D., et al. (2009). Equine Infectious Anemia Viral Vector-Mediated Codelivery of Endostatin and Angiostatin Driven by Retinal Pigmented Epithelium-specific VMD2 Promoter Inhibits Choroidal Neovascularization. Hum. Gene Ther. 20, 31–39. doi:10.1089/hum.2008.046
Kachi, S., Esumi, N., Zack, D. J., and Campochiaro, P. A. (2006). Sustained Expression after Nonviral Ocular Gene Transfer Using Mammalian Promoters. Gene Ther. 13, 798–804. doi:10.1038/sj.gt.3302700
Khabou, H., Garita-Hernandez, M., Chaffiol, A., Reichman, S., Jaillard, C., Brazhnikova, E., et al. (2018). Noninvasive Gene Delivery to Foveal Cones for Vision Restoration. JCI Insight 3, e96029. doi:10.1172/jci.insight.96029
Kim, T. H., Jin, H., Kim, H. W., Cho, M.-H., and Cho, C. S. (2006). Mannosylated Chitosan Nanoparticle-Based Cytokine Gene Therapy Suppressed Cancer Growth in BALB/c Mice Bearing CT-26 Carcinoma Cells. Mol. Cancer Ther. 5, 1723–1732. doi:10.1158/1535-7163.MCT-05-0540
Knight, A., Carvajal, J., Schneider, H., Coutelle, C., Chamberlain, S., and Fairweather, N. (1999). Non-viral Neuronal Gene Delivery Mediated by the HC Fragment of Tetanus Toxin. Eur. J. Biochem. 259, 762–769. doi:10.1046/j.1432-1327.1999.00108.x
Koo, H., Moon, H., Han, H., Na, J. H., Huh, M. S., Park, J. H., et al. (2012). The Movement of Self-Assembled Amphiphilic Polymeric Nanoparticles in the Vitreous and Retina after Intravitreal Injection. Biomaterials 33, 3485–3493. doi:10.1016/j.biomaterials.2012.01.030
Kuhrt, H., Härtig, W., Grimm, D., Faude, F., Kasper, M., and Reichenbach, A. (1997). Changes in CD44 and ApoE Immunoreactivities Due to Retinal Pathology of Man and Rat. J. fur Hirnforschung 38, 223–229.
Kuzmanovic, M., Dudley, V. J., and Sarthy, V. P. (2003). GFAP Promoter Drives Müller Cell-specific Expression in Transgenic Mice. Invest. Ophthalmol. Vis. Sci. 44, 3606–3613. doi:10.1167/iovs.02-1265
Kwon, E. J., Bergen, J. M., Park, I. K., and Pun, S. H. (2008). Peptide-modified Vectors for Nucleic Acid Delivery to Neurons. J. Control. Release 132, 230–235. doi:10.1016/j.jconrel.2008.06.012
Lai, T. Y. Y., Liu, S., Das, S., and Lam, D. S. C. (2015). Intravitreal Injection-Technique and Safety. Asia-Pacific J. Ophthalmol. 4, 321–328. doi:10.1097/APO.0000000000000146
Laradji, A., Karakocak, B. B., Kolesnikov, A. V., Kefalov, V. J., and Ravi, N. (2021). Hyaluronic Acid-Based Gold Nanoparticles for the Topical Delivery of Therapeutics to the Retina and the Retinal Pigment Epithelium. Polymers 13, 3324. doi:10.3390/polym13193324
Lee, J. H., Wang, J.-H., Chen, J., Li, F., Edwards, T. L., Hewitt, A. W., et al. (2019). Gene Therapy for Visual Loss: Opportunities and Concerns. Prog. Retin. Eye Res. 68, 31–53. doi:10.1016/j.preteyeres.2018.08.003
Liu, M. M., Tuo, J., and Chan, C.-C. (2011). Gene Therapy for Ocular Diseases. Br. J. Ophthalmol. 95, 604–612. doi:10.1136/bjo.2009.174912
Liu, N. P., Roberts, W. L., Hale, L. P., Levesque, M. C., Patel, D. D., Lu, C. L., et al. (1997). Expression of CD44 and Variant Isoforms in Cultured Human Retinal Pigment Epithelial Cells. Invest. Ophthalmol. Vis. Sci. 38, 2027–2037.
Lu, Q., Ganjawala, T. H., Ivanova, E., Cheng, J. G., Troilo, D., and Pan, Z.-H. (2016). AAV-mediated Transduction and Targeting of Retinal Bipolar Cells with Improved mGluR6 Promoters in Rodents and Primates. Gene Ther. 23, 680–689. doi:10.1038/gt.2016.42
Lynch, C., Kondiah, P. P. D., Choonara, Y. E., du Toit, L. C., Ally, N., and Pillay, V. (2019). Advances in Biodegradable Nano-Sized Polymer-Based Ocular Drug Delivery. Polymers 11, 1371. doi:10.3390/polym11081371
Maguire, A. M., Russell, S., Wellman, J. A., Chung, D. C., Yu, Z.-F., Tillman, A., et al. (2019). Efficacy, Safety, and Durability of Voretigene Neparvovec-Rzyl in RPE65 Mutation-Associated Inherited Retinal Dystrophy. Ophthalmology 126, 1273–1285. doi:10.1016/j.ophtha.2019.06.017
Mahato, R. I. (1999). Non-Viral Peptide-Based Approaches to Gene Delivery. J. Drug Target. 7, 249–268. doi:10.3109/10611869909085509
Makrides, S. C. (2003). “Vectors for Gene Expression in Mammalian Cells,” in New Comprehensive Biochemistry, Gene Transfer and Expression in Mammalian Cells (Amsterdam: Elsevier), 9–26. doi:10.1016/S0167-7306(03)38002-0
Martens, T. F., Remaut, K., Deschout, H., Engbersen, J. F. J., Hennink, W. E., van Steenbergen, M. J., et al. (2015). Coating Nanocarriers with Hyaluronic Acid Facilitates Intravitreal Drug Delivery for Retinal Gene Therapy. J. Control. Release 202, 83–92. doi:10.1016/j.jconrel.2015.01.030
Matsuda, T., and Cepko, C. L. (2004). Electroporation and RNA Interference in the Rodent Retina In Vivo and In Vitro. Proc. Natl. Acad. Sci. U.S.A. 101, 16–22. doi:10.1073/pnas.2235688100
Midoux, P., Pichon, C., Yaouanc, J.-J., and Jaffrès, P.-A. (2009). Chemical Vectors for Gene Delivery: a Current Review on Polymers, Peptides and Lipids Containing Histidine or Imidazole as Nucleic Acids Carriers. Br. J. Pharmacol. 157, 166–178. doi:10.1111/j.1476-5381.2009.00288.x
Mulligan, R. C. (1993). The Basic Science of Gene Therapy. Science 260, 926–932. doi:10.1126/science.8493530
Newman, C. M. H., and Bettinger, T. (2007). Gene Therapy Progress and Prospects: Ultrasound for Gene Transfer. Gene Ther. 14, 465–475. doi:10.1038/sj.gt.3302925
Nickerson, J. M., Goodman, P., Chrenek, M. A., Bernal, C. J., Berglin, L., Redmond, T. M., et al. (2012). “Subretinal Delivery and Electroporation in Pigmented and Nonpigmented Adult Mouse Eyes,” in Retinal Development: Methods and Protocols, Methods in Molecular Biology. Editor S. Z. Wang (Totowa, NJ: Humana Press), 53–69. doi:10.1007/978-1-61779-848-1_4
Nicoud, M., Kong, J., Iqball, S., Kan, O., Naylor, S., Gouras, P., et al. (2007). Development of Photoreceptor-specific Promoters and Their Utility to Investigate EIAV Lentiviral Vector Mediated Gene Transfer to Photoreceptors. J. Gene Med. 9, 1015–1023. doi:10.1002/jgm.1115
Niederkorn, J., Streilein, J. W., and Shadduck, J. A. (1981). Deviant Immune Responses to Allogeneic Tumors Injected Intracamerally and Subcutaneously in Mice. Invest. Ophthalmol. Vis. Sci. 20, 355–363.
Oliveira, A. V., Bitoque, D. B., and Silva, G. A. (2014). Combining Hyaluronic Acid with Chitosan Enhances Gene Delivery. J. Nanomater. 2014, 1–9. doi:10.1155/2014/246347
Oliveira, A. V., Marcelo, A., Rosa da Costa, A. M., and Silva, G. A. (2016). Evaluation of Cystamine-Modified Hyaluronic Acid/chitosan Polyplex as Retinal Gene Vector. Mater. Sci. Eng. C 58, 264–272. doi:10.1016/j.msec.2015.08.047
Patel, A., Cholkar, K., Agrahari, V., and Mitra, A. K. (2013). Ocular Drug Delivery Systems: An Overview. Wjp 2, 47–64. doi:10.5497/wjp.v2.i2.47
Pellissier, L. P., Hoek, R. M., Vos, R. M., Aartsen, W. M., Klimczak, R. R., Hoyng, S. A., et al. (2014). Specific Tools for Targeting and Expression in Müller Glial Cells. Mol. Ther. - Methods & Clin. Dev. 1, 14009. doi:10.1038/mtm.2014.9
Peynshaert, K., Devoldere, J., Minnaert, A.-K., De Smedt, S. C., and Remaut, K. (2019). Morphology and Composition of the Inner Limiting Membrane: Species-specific Variations and Relevance toward Drug Delivery Research. Curr. Eye Res. 44, 465–475. doi:10.1080/02713683.2019.1565890
Pierce, E. A., and Bennett, J. (2015). The Status ofRPE65Gene Therapy Trials: Safety and Efficacy. Cold Spring Harb. Perspect. Med. 5, a017285. doi:10.1101/cshperspect.a017285
Prentice, H. M., Biswal, M. R., Dorey, C. K., and Blanks, J. C. (2011). Hypoxia-Regulated Retinal Glial Cell-specific Promoter for Potential Gene Therapy in Disease. Invest. Ophthalmol. Vis. Sci. 52, 8562–8570. doi:10.1167/iovs.10-6835
Price, D. L., Griffin, J., Young, A., Peck, K., and Stocks, A. (1975). Tetanus Toxin: Direct Evidence for Retrograde Intraaxonal Transport. Science 188, 945–947. doi:10.1126/science.49080
Rajala, A., Wang, Y., Zhu, Y., Ranjo-Bishop, M., Ma, J.-X., Mao, C., et al. (2014). Nanoparticle-Assisted Targeted Delivery of Eye-specific Genes to Eyes Significantly Improves the Vision of Blind Mice In Vivo. Nano Lett. 14, 5257–5263. doi:10.1021/nl502275s
Rama Ballesteros, A. R., Hernández, R., Perazzoli, G., Cabeza, L., Melguizo, C., Vélez, C., et al. (2020). Specific Driving of the Suicide E Gene by the CEA Promoter Enhances the Effects of Paclitaxel in Lung Cancer. Cancer Gene Ther. 27, 657–668. doi:10.1038/s41417-019-0137-3
Ramamoorth, M., and Narvekar, A. (2015). Non Viral Vectors in Gene Therapy- an Overview. Jcdr 9, GE01–GE06. doi:10.7860/JCDR/2015/10443.5394
RetNet(2022) RetNet Summaries [WWW Document], n.d. URL. AvaliableAt: https://sph.uth.edu/retnet/sum-dis.htm (Accessed 15 3, 22).
Ribeiro, M. C. S., de Miranda, M. C., Cunha, P. D. S., Andrade, G. F., Fulgêncio, G. D. O., Gomes, D. A., et al. (2021). Neuroprotective Effect of siRNA Entrapped in Hyaluronic Acid-Coated Lipoplexes by Intravitreal Administration. Pharmaceutics 13, 845. doi:10.3390/pharmaceutics13060845
Rodriguez, A., del, A., and Angeles, M. (2013). Non-Viral Delivery Systems in Gene Therapy. IntechOpen 17, 147–153. doi:10.5772/52704
R. Sparrrow, J., Hicks, D., and P. Hamel, C. (2010). The Retinal Pigment Epithelium in Health and Disease. Cmm 10, 802–823. doi:10.2174/156652410793937813
Sakurai, E., Ozeki, H., Kunou, N., and Ogura, Y. (2001). Effect of Particle Size of Polymeric Nanospheres on Intravitreal Kinetics. Ophthalmic Res. 33, 31–36. doi:10.1159/000055638
Santana-Armas, M. L., and Tros de Ilarduya, C. (2021). Strategies for Cancer Gene-Delivery Improvement by Non-viral Vectors. Int. J. Pharm. 596, 120291. doi:10.1016/j.ijpharm.2021.120291
Schmidt, E. V., Christoph, G., Zeller, R., and Leder, P. (1990). The Cytomegalovirus Enhancer: a Pan-Active Control Element in Transgenic Mice. Mol. Cell Biol. 10, 4406–4411. doi:10.1128/mcb.10.8.4406-4411.1990
Shinoe, T., Kuribayashi, H., Saya, H., Seiki, M., Aburatani, H., and Watanabe, S. (2010). Identification of CD44 as a Cell Surface Marker for Müller Glia Precursor Cells. J. Neurochem. 115, 1633–1642. doi:10.1111/j.1471-4159.2010.07072.x
Sonoda, Y., and Streilein, J. W. (1992). Orthotopic Corneal Transplantation in Mice-Evidence that the Immunogenetic Rules of Rejection Do Not Apply. Transplantation 54, 694–703. doi:10.1097/00007890-199210000-00026
Suen, W.-L. L., and Chau, Y. (2013). Specific Uptake of Folate-Decorated Triamcinolone-Encapsulating Nanoparticles by Retinal Pigment Epithelium Cells Enhances and Prolongs Antiangiogenic Activity. J. Control. Release 167, 21–28. doi:10.1016/j.jconrel.2013.01.004
Suri, R., Beg, S., and Kohli, K. (2020). Target Strategies for Drug Delivery Bypassing Ocular Barriers. J. Drug Deliv. Sci. Technol. 55, 101389. doi:10.1016/j.jddst.2019.101389
Toscano, M. G., Romero, Z., Muñoz, P., Cobo, M., Benabdellah, K., and Martin, F. (2011). Physiological and Tissue-specific Vectors for Treatment of Inherited Diseases. Gene Ther. 18, 117–127. doi:10.1038/gt.2010.138
Tsai, C.-H., Wang, P.-Y., Lin, I.-C., Huang, H., Liu, G.-S., and Tseng, C.-L. (2018). Ocular Drug Delivery: Role of Degradable Polymeric Nanocarriers for Ophthalmic Application. Ijms 19, 2830. doi:10.3390/ijms19092830
Urbiola, K., Blanco-Fernández, L., Navarro, G., Rödl, W., Wagner, E., Ogris, M., et al. (2015). Evaluation of Improved PAMAM-G5 Conjugates for Gene Delivery Targeted to the Transferrin Receptor. Eur. J. Pharm. Biopharm. 94, 116–122. doi:10.1016/j.ejpb.2015.05.007
Venkatesh, A., Ma, S., Langellotto, F., Gao, G., and Punzo, C. (2013). Retinal Gene Delivery by rAAV and DNA Electroporation. Curr. Protoc. Microbiol. 14, Unit 14D. doi:10.1002/9780471729259.mc14d04s28
Vicente-Pascual, M., Gómez-Aguado, I., Rodríguez-Castejón, J., Rodríguez-Gascón, A., Muntoni, E., Battaglia, L., et al. (2020). Topical Administration of SLN-Based Gene Therapy for the Treatment of Corneal Inflammation by De Novo IL-10 Production. Pharmaceutics 12, 584. doi:10.3390/pharmaceutics12060584
Wang, H., Kunz, E., Stoddard, G. J., Hauswirth, W. W., and Hartnett, M. E. (2019). Optimal Inhibition of Choroidal Neovascularization by scAAV2 with VMD2 Promoter-Driven Active Rap1a in the RPE. Sci. Rep. 9, 15732. doi:10.1038/s41598-019-52163-z
Wang, Q., Zhuang, P., Huang, H., Li, L., Liu, L., Webber, H. C., et al. (2020). Mouse γ-Synuclein Promoter-Mediated Gene Expression and Editing in Mammalian Retinal Ganglion Cells. J. Neurosci. 40, 3896–3914. doi:10.1523/JNEUROSCI.0102-20.2020
Wang, Y., Rajala, A., Cao, B., Ranjo-Bishop, M., Agbaga, M.-P., Mao, C., et al. (2016). Cell-Specific Promoters Enable Lipid-Based Nanoparticles to Deliver Genes to Specific Cells of the Retina In Vivo. Theranostics 6, 1514–1527. doi:10.7150/thno.15230
Wu, D. M., Ji, X., Ivanchenko, M. V., Chung, M., Piper, M., Rana, P., et al. (2021). Nrf2 Overexpression Rescues the RPE in Mouse Models of Retinitis Pigmentosa. JCI Insight 6, e145029. doi:10.1172/jci.insight.145029
Yan, M., Chen, J., Jiang, H., Xie, Y., Li, C., Chen, L., et al. (2020). Effective Inhibition of Cancer Cells by Recombinant Adenovirus Expressing EGFR-Targeting Artificial microRNA and Reversed-Caspase-3. PLOS ONE 15, e0237098. doi:10.1371/journal.pone.0237098
Yang, N. S., Burkholder, J., Roberts, B., Martinell, B., and McCabe, D. (1990). In Vivo and In Vitro Gene Transfer to Mammalian Somatic Cells by Particle Bombardment. Proc. Natl. Acad. Sci. U.S.A. 87, 9568–9572. doi:10.1073/pnas.87.24.9568
Ye, G.-J., Budzynski, E., Sonnentag, P., Nork, T. M., Sheibani, N., Gurel, Z., et al. (2016). Cone-Specific Promoters for Gene Therapy of Achromatopsia and Other Retinal Diseases. Hum. Gene Ther. 27, 72–82. doi:10.1089/hum.2015.130
Yoon, A.-R., Hong, J., Kim, M., and Yun, C.-O. (2018). Hepatocellular Carcinoma-Targeting Oncolytic Adenovirus Overcomes Hypoxic Tumor Microenvironment and Effectively Disperses through Both Central and Peripheral Tumor Regions. Sci. Rep. 8, 2233. doi:10.1038/s41598-018-20268-6
Zack, D. J., Bennett, J., Wang, Y., Davenport, C., Klaunberg, B., Gearhart, J., et al. (1991). Unusual Topography of Bovine Rhodopsin Promoter-IacZ Fusion Gene Expression in Transgenic Mouse Retinas. Neuron 6, 187–199. doi:10.1016/0896-6273(91)90355-4
Zhang, Y., Schlachetzki, F., Li, J. Y., Boado, R. J., and Pardridge, W. M. (2003). Organ-specific Gene Expression in the Rhesus Monkey Eye Following Intravenous Non-viral Gene Transfer. Mol. Vis. 9, 465–472.
Zheng, C., and Baum, B. J. (2008). “Evaluation of Promoters for Use in Tissue-specific Gene Delivery,” in Gene Therapy Protocols: Design and Characterization of Gene Transfer Vectors, Methods in Molecular BiologyTM. Editor J. M Le Doux (Totowa, NJ: Humana Press), 205–219. doi:10.1007/978-1-60327-248-3_13
Keywords: non-viral vectors, gene therapy, retina, cellular targeting, polymers.
Citation: Bitoque DB, Fernandes CF, Oliveira AML and Silva GA (2022) Strategies to Improve the Targeting of Retinal Cells by Non-Viral Gene Therapy Vectors. Front. Drug. Deliv. 2:899260. doi: 10.3389/fddev.2022.899260
Received: 18 March 2022; Accepted: 29 April 2022;
Published: 13 May 2022.
Edited by:
Heather Sheardown, McMaster University, CanadaReviewed by:
Alexander V Ljubimov, Cedars Sinai Medical Center, United StatesCopyright © 2022 Bitoque, Fernandes, Oliveira and Silva. This is an open-access article distributed under the terms of the Creative Commons Attribution License (CC BY). The use, distribution or reproduction in other forums is permitted, provided the original author(s) and the copyright owner(s) are credited and that the original publication in this journal is cited, in accordance with accepted academic practice. No use, distribution or reproduction is permitted which does not comply with these terms.
*Correspondence: Gabriela A. Silva, Z2FicmllbGEuc2lsdmFAbm1zLnVubC5wdA==
†These authors have contributed equally to this work
Disclaimer: All claims expressed in this article are solely those of the authors and do not necessarily represent those of their affiliated organizations, or those of the publisher, the editors and the reviewers. Any product that may be evaluated in this article or claim that may be made by its manufacturer is not guaranteed or endorsed by the publisher.
Research integrity at Frontiers
Learn more about the work of our research integrity team to safeguard the quality of each article we publish.