- 1Respira Therapeutics, Inc., Burlingame, CA, United States
- 2Genentech, South San Francisco, CA, United States
Spray-dried formulations of a hydrophobic, crystalline drug, GDC-A, were prepared using the suspension-PulmoSphere™ technology. Increases in drug loading resulted in decreases in the primary particle size distribution and increases in tapped density. This enabled fine particle doses of up to 25 mg to be achieved with a portable dry powder inhaler from a size three capsule. The powders were physically and chemically stable, with no changes in physical form or degradants observed during processing or on storage in an open configuration at 40°C for 1 month. The potential benefits of the suspension-based spray drying process relative to solution-based spray drying in terms of stability, lung targeting, and safety/tolerability are discussed.
Introduction
Spray drying is a process for converting an atomized liquid feedstock into a dry powder (Vehring, 2008; Vehring et al., 2020). The nature of the feedstock and process conditions play a critical role in determining the size, morphology, physical form, and surface energy of the manufactured particles. For pulmonary delivery, these micromeritic properties are important in controlling the chemical stability, physical stability, manufacturability, aerosol performance, pharmacokinetics, and tolerability of the drug product (Weers and Miller, 2015; Chen et al., 2016; Sahakipijarn et al., 2020; Shetty et al., 2020). Spray drying is being increasingly used for the development of moderate and high dose dry powder formulations with total lung doses (TLD) between 1 and 100 mg (Brunaugh and Smyth, 2018; Sibum et al., 2018; Son et al., 2021).
GDC-A is a low molecular weight free base drug with a high melting temperature. It is highly crystalline, hydrophobic, moderately hygroscopic, and chemically stable with a target TLD between 5 and 20 mg. Pulmonary administration of high doses of a hydrophobic drug presents significant challenges.
Amorphous solid dispersions are often used to improve the bioavailability of poorly soluble hydrophobic drugs for oral administration (Paudel et al., 2013; Singh and Van den Mooter, 2016). In these formulations the hydrophobic drug and a polymer with a high glass transition temperature (Tg) are dissolved in a suitable organic solvent and spray-dried to form an amorphous dry powder. The resulting disordered glassy matrix improves the apparent solubility of the drug, thereby increasing bioavailability. In turn, the high viscosity of the amorphous phase inhibits molecular mobility, preventing crystallization of the drug. The drug loading in these formulations is typically 50% w/w or less, as a significant amount of polymer is needed to raise the Tg and improve the physical stability of the thermodynamically unstable amorphous phase.
Polymeric excipients are generally not used as glass-formers in pulmonary applications due to their slow clearance from the lungs. Small molecule glass formers (e.g., trehalose, sucrose, mannitol) could be utilized, but the low drug loading concerns remain, especially if additional shell-forming excipients (e.g., leucine, trileucine, phospholipids, fatty acid soaps) are needed to boost aerosol performance (Weers and Miller, 2015; Chen et al., 2016).
Alternatively, it may be possible to maintain the crystallinity of the drug substance by spray drying a suspension of the drug in a suitable non-solvent (Weers and Tarara, 2014; Weers et al., 2019). This manuscript will review considerations around the design of an inhaled GDC-A dry powder formulation using a suspension-based spray drying process, with the goal to optimize the physical stability, chemical stability, aerosol performance, pharmacokinetics, and tolerability of a GDC-A drug product.
Materials and Methods
GDC-A was obtained from the Small Molecule Process Chemistry Department (Genentech, Inc., South San Francisco, CA, United States). The micronized GDC-A drug substance has high purity (assay = 99.9%), low water content (0.99%), and a fine particle size distribution (Dv10 = 0.6 μm, Dv50 = 1.6 μm, and Dv90 = 3.4 μm). Extensive polymorph screening identified two solid state forms of GDC-A labeled Forms A and E. Form A is a non-stochiometric crystalline hydrate of GDC-A, while Form E is an anhydrate of GDC-A. Both forms A and E can be produced at room temperature depending on the crystallization solvent, relative humidity, and processing conditions. Form A was supplied for use in the present study.
Distearoylphosphatidylcholine (DSPC) was obtained from Lipoid GmbH (Ludwigschafen, Germany), calcium chloride dihydrate from Spectrum Chemical Manufacturing Corp. (New Brunswick, NJ, United States), and USP Perflubon (perfluorooctyl bromide, PFOB) from Exfluor Research Corp. (Round Rock, TX, United States).
Materials
The GDC-A drug product was prepared by spray-drying a liquid feedstock comprising a suspension of micronized GDC-A particles in an aqueous phase containing dispersed submicron PFOB-in-water emulsion droplets (Weers and Tarara, 2014; Weers et al., 2019). The emulsion droplets were prepared by first forming a dispersion of multilamellar vesicles (MLV) of DSPC and CaCl2 (2/1 mol/mol ratio) in hot water (T > 65°C) above the hydrated gel-to-liquid crystal phase transition (Tm) of the phospholipid. The phospholipid was dispersed with a high-shear mixer (UltraTurrax T-25, (IKA, Wilmington, NC, USA). PFOB was added to this vesicle dispersion while mixing to form a coarse PFOB-in-water emulsion. This emulsion was then homogenized using a high-pressure homogenizer (EmulsiFlex C5, Avestin, Inc., Ottawa, Canada) for ∼10 min at 5,000 psi, ∼10 min at 10,000 psi, ending with a single discrete pass at 17,000 psi. The resulting fine emulsion has droplet diameters between ∼200 and 500 nm (Weers and Tarara, 2014). The size of the emulsion droplets and as a result the size of the pores in the small porous particle post spray-drying can be controlled by varying the ratio of PFOB/DSPC. Droplets that are starved in DSPC will have a larger droplet size in order to maintain the area/molecule for DSPC at the PFOB/water interface (Weers and Tarara, 2014).
During formulation development, different approaches were used to incorporate GDC-A into the feedstock. Forming stable suspensions of hydrophobic drugs like GDC-A in water can be challenging, as they often wet poorly, preferring to agglomerate at the air-water interface (Figure 1A) or adhere to surfaces in the manufacturing equipment.
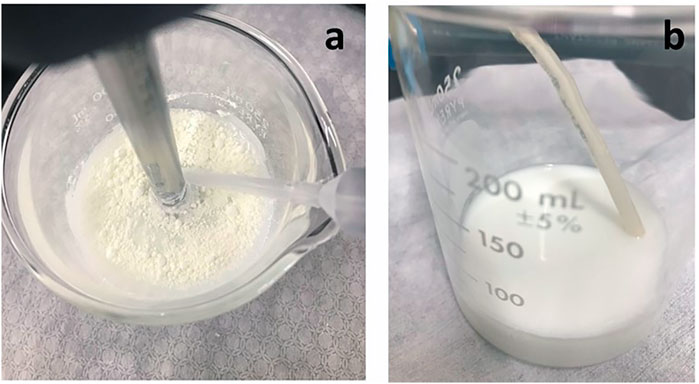
FIGURE 1. Images of GDC-A PulmoSphere feedstock. (A) image just after powder addition to PulmoSphere emulsion showing poorly wetting GDC-A powder at the air/water interface; (B) Suspended GDC-A in PulmoSphere emulsion showing the “milk-like” appearance of well-dispersed feedstock post-homogenization.
Owing to its low surface tension at the air/water interface (∼20 mN/m), DSPC is a good dispersant for hydrophobic drugs (Kabalnov et al., 1995). The challenge with DSPC is that it is poorly soluble in water (∼10–10 mol/L) (Marsh, 1990). When present in vesicles or emulsion droplets in a continuous water phase, molecular diffusion of DSPC molecules to a drug particle’s surface is exceedingly slow (Kabalnov et al., 1995). Hence, effective mixing of the phospholipid and drug is key to enabling preparation of a suitable suspension. This is not trivial. Fortunately, hydrophobic materials (e.g., leucine, phospholipids, cyclosporine, corticosteroids), are easily wetted and form stable suspensions in PFOB (Weers and Tarara, 2014; Weers et al., 2019; Miller et al., 2021). Hence, wetting the drug in PFOB (either in the coarse emulsion, or prior to emulsion formation in a solid-in-oil-in-water dispersion) reduces the risk that a poor suspension may be produced, especially at larger scales. In the present study, three methods for incorporation of the drug into the feedstock were studied. or the process designated as PSph A, the drug is dispersed in the fine emulsion. In PSph B, the drug is added to the lipid dispersion, while in PSph C the drug is dispersed in the PFOB in a solid-in-oil-in-water dispersion prior to fine emulsion formation. All three methods were able to effectively disperse the drug. The resulting complex dispersion comprising suspended drug particles in the oil-in-water emulsion was passed through a high-pressure homogenizer for a single discrete pass at 17,000 psi. The final aqueous feedstock comprising the suspended GDC-A particles and sub-micron emulsion droplets had an appearance that resembled milk, with no visible flocs of drug (Figure 1B).
Spray drying was conducted with a Büchi B-191 mini spray dryer (Flawil, Switzerland). For the purposes of this work, the atomization and drying processes were held constant, using parameters consistent with previous work on suspension-based PulmoSphere™ formulations (Weers et al., 2019). The spray-drying conditions utilized were an inlet temperature of 105°C, an outlet temperature of 60–65°C, an aspirator setting of 100%, an atomizer gas pressure of 70 psi, and a liquid feed rate of 5.0 ml/min. A custom-built (Adams and Chittenden, Berkeley, CA) glass cyclone was used. The aqueous feedstock was atomized with a twin fluid atomizer to produce 8–10 μm liquid droplets containing suspended drug particles and approximately one thousand emulsion droplets (Figure 2) (Ivey and Vehring, 2010; Weers et al., 2019). To prevent particle sedimentation having a negative impact on batch uniformity, the liquid feedstock was stirred during the spray drying process. During the initial moments of the drying process, the lower boiling process aid (water) begins to evaporate. This leads to a decrease in size of the atomized droplets, concentration of the slowly diffusing drug particles and emulsion droplets at the air-water interface, and the formation of a hollow core at the center of the droplet (Vehring, 2008; Ivey and Vehring, 2010; Weers and Tarara, 2014; Weers et al., 2019). Eventually, the second process aid (i.e., the oil phase in the dispersed emulsion droplets) evaporates, leaving behind pores where the sub-micron emulsion droplets were originally found. The process results in production of crystalline drug particles embedded in a nanostructured porous shell of phospholipid (Ivey and Vehring, 2010; Weers et al., 2019).
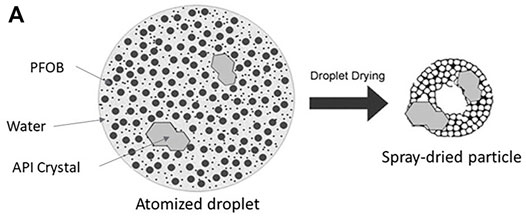
FIGURE 2. Schematic of an atomized droplet containing nanoemulsion droplets and suspended drug particles (also known as the suspension PulmoSphere™ manufacturing process) with Adapted from Ivey and Vehring (2010) and Weers et al. (2019).
For aerosol performance testing, the spray-dried GDC-A bulk powder was hand-filled into size 3, inhalation grade hydroxypropylmethylcellulose (HPMC) capsules (Quali-V®, Qualicaps, Whitsett, NC). The fill mass varied depending on the nature of the formulation. Powder consolidation within the capsule was achieved by gently tapping the capsule on a hard surface to enable increases in fill mass.
Methods
Determination of GDC-A content and purity in formulated bulk powder and aerosol test samples were determined by reverse phase high performance liquid chromatography (RP-HPLC) with detection at 220 nm. Samples were analyzed with an Agilent 1260 Infinity II HPLC system (Wilmington, DE, United States). Separation was achieved with Agilent InfinityLab Poroshell 120 EC-C18, 3.0 × 150 mm, 2.7 µm column (P/N 693,975–302) using a gradient method (solvent A = water with 0.025% trifluoroacetic acid (TFA); solvent B = acetonitrile with 0.025% TFA. GDC-A was quantified using a single-point calibration with a reference standard. Samples were extracted using water:acetonitrile 1:1 (v/v). The analyte retention time was 9.5 min, and the total run time was 22 min.
Primary particle size distributions were determined via laser diffraction (Sympatec GmbH, Clausthal-Zellerfeld, Germany). The Sympatec H3296 unit was equipped with an R2 lens, an ASPIROS micro dosing unit, and a RODOS/M dry powder-dispersing unit. Approximately 2–5 mg powder was filled into tubes and fed at 5 mm/s into a RODOS operated with 4 bar dispersion pressure and 65 mbar vacuum. Powders were introduced at an optical concentration of approximately 1–5% and data was collected over a measurement duration up to 15 s. Particle size distributions were calculated by the instrument software using the Fraünhofer model. Reported values represent the mean and standard deviation of three measurements.
Tapped densities were determined using a cylindrical cavity of known volume (0.593 cm3). Powder was filled into this sample holder using a microspatula. The sample cell was then gently tapped on a countertop. As the sample volume decreased, more powder was added to the cell. The tapping and addition of powder steps were repeated until the cavity was filled, and the powder bed no longer consolidated with further tapping. The tapped density is defined as the mass of this tapped bed of powder divided by the volume of the cavity.
X-ray powder diffraction (XRPD) patterns of selected powders were measured with a Rigaku Miniflex Model 600 diffraction system equipped with a D/Tex solid state “strip” detector. Each sample was prepared by packing bulk powder into a sample holder. Samples were scanned from 3° 2θ to 35° 2θ at a scan rate of 1° 2θ/min, using a Cu radiation source with a wavelength of 1.54 Å, operated at 40 kV and 15 mA.
The aerosol performance of spray-dried formulations of GDC-A were assessed with a variant of the RS01 dry powder inhaler, DPI (Plastiape, S.p.A., Osnago, Italy). The RS01 DPI is a portable, capsule-based DPI that is available with different resistances to airflow through the device. The variant utilized modifies the air inlet in the base assembly to increase device resistance to ∼0.12 cm H2O0.5 L−1 min.
Aerodynamic particle size distributions (APSD) were determined with a Next Generation Impactor (NGI) equipped with a USP induction port. No pre-separator is needed for engineered particles in the absence of carrier particles. The impactor stages were coated with a solution comprising 50% v/v glycerol, 5% v/v Tween 20, and 45% v/v water (1 ml of solution for stages 2–7 and 2 ml for stage 1 and MOC). Tests were conducted in accordance with USP 〈601〉 Aerosols ‘Aerodynamic Size Distribution, Apparatus six for Dry Powder Inhalers’ and Ph. Eur. 2.9.18 ‘Preparations for Inhalation; Aerodynamic Assessment of Fine Particles; Apparatus E’. APSD measurements were conducted at a volume of 4 L and a pressure drop of 4 kPa. This corresponds to a volumetric flow rate of about 54 L/min. Delivered doses (DD) are reported for NGI testing by summing the mass of drug in the throat and on the various stages in the impactor. GDC-A was extracted and quantitated using the RP-HPLC detailed above.
Delivered dose measurements were conducted with a dose uniformity sampling apparatus (DUSA), with quantitation of drug content using the RP-HPLC detailed above. A total of ten measurements were made for delivered dose uniformity (DDU) testing, and the mean DD and standard deviation were calculated.
Results
Impact of the Spray Drying Process on Drug Substance Properties
The physicochemical properties of spray-dried formulations of GDC-A are detailed in Table 1. Unless otherwise noted, the formulations within Table 1 were prepared at 10% v/v PFOB.
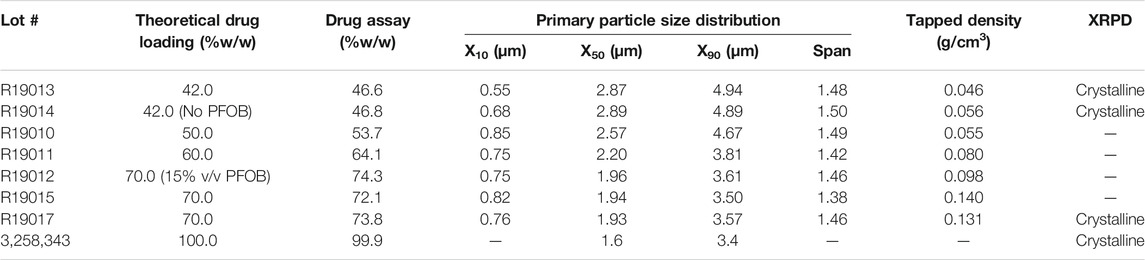
TABLE 1. Physicochemical properties of spray-dried PulmoSphere formulations of GDC-A. Unless noted, the PFOB content in the feedstock was 10% v/v.
Suspension-based PulmoSphere formulations are often enriched in drug content relative to their theoretical content, presumably due to selective loss (out the exhaust of the spray dryer) of small unilamellar vesicles (post-homogenization) that do not contain drug. For GDC-A, the degree of enrichment was between 3.0 and 11.4%, decreasing with increases in drug content. Studies with other suspension based PulmoSphere formulations suggest that the degree of enrichment for a given formulation and process is highly reproducible between batches.
The suspension-based PulmoSphere manufacturing process for GDC-A, including high-shear mixing, high-pressure homogenization and spray drying resulted in purity and related substance profiles that were identical to the neat drug. Chemical stability of the formulated GDC-A drug product was assessed by placing both neat drug substance and batch R19017 (70% API, PSph C) at 40 °C for 1 month in an open dish configuration. The purity of both the neat drug substance and batch R19017 remained at ∼100.0% (normalized peak area), and all peaks remained below the limit of quantitation of 0.5 ng. Moreover, no changes in drug content were observed for batch R19017.
XRPD results indicate that the suspension-based PulmoSphere manufacturing process preserves the crystalline nature (Form A) of GDC-A in the drug product (Figure 3). Each diffraction peak observed in the drug substance is also present in the spray-dried powder. In this example, the diffraction peaks of the spray-dried powder are intense due to the high drug loading (70% w/w). The additional peak observed at 21.2°2θ is due to the DSPC excipient (Sun et al., 1996; Miller et al., 2015). This corresponds to a d-spacing of ∼4.2 Å. This length scale is consistent with the chain-chain spacing in hydrated gel-phase DSPC. The high crystallinity of the formulated drug product is maintained over 26 months following room temperature storage in a dry box as evidenced by the overlapping XRPD pattern with the t = 0 sample.
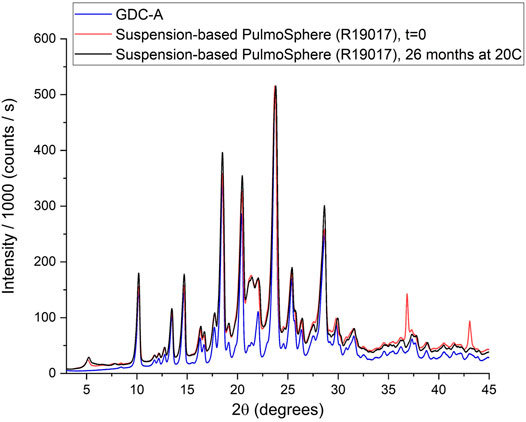
FIGURE 3. XRPD powder patterns of neat GDC-A and drug formulated in suspension-based PulmoSphere particles.
Impact of Increases in Drug Loading
Scanning electron microscopy (SEM) images of selected GDC-A formulations are detailed in Figure 4. As the drug loading is increased, there is a transition from particles that are PulmoSphere-like with a porous morphology (42% w/w GDC-A) to particles that have an appearance that is more consistent with the micronized API (70% w/w GDC-A).
The shift in particle morphology with increases in drug content is also reflected by linear decreases in the size and increases in tapped density of the GDC-A powders (Table 1; Figure 5). As the theoretical drug loading was increased from 42 to 70% w/w, the size of the spray-dried particles was markedly decreased from 2.9 to 1.9 μm for the X50, and from 4.9 to 3.6 μm for the X90. The tapped density of the powders was increased from 0.05 g/cm3 to 0.13 g/cm3. Hence, the less PulmoSphere excipient that is present, the smaller the particles and the greater the tapped density. As the drug loading increases, the particles increasingly resemble the neat drug crystals (as given by the data for the 100% API). At a 70% drug loading, the X50 remains about 0.4 μm larger than that of the neat drug, however. The increases in tapped density and decreases in primary particle size distribution at higher drug loadings both favor higher TLDs for GDC-A, provided that the particles can be adequately dispersed with a portable dry powder inhaler.
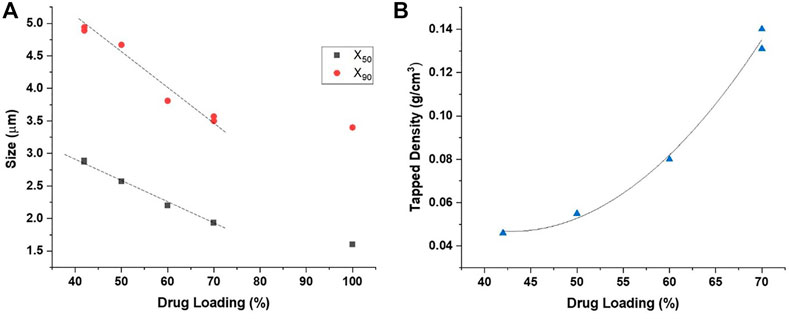
FIGURE 5. Impact of drug loading on the: (A) primary particle size distribution, and (B) tapped density of spray-dried formulations comprising GDC-A.
The aerosol performance was assessed with a Next Generation Impactor (4 kPa pressure drop with the RS01 DPI) for batches with varying drug content and fill mass (Table 2). A representative aerodynamic particle size distribution is presented for batch 19,015–1 in Figure 6. The impactor DD measurements were generally greater than 80% of the nominal dose. The MMAD of the powders decreased with increased drug loading, presumably driven by the decrease in primary particle size. For the 70% w/w GDC-A formulations (10% v/v PFOB), the MMAD values were in the range from 2.4 to 2.7 μm, with FPF<5µm values of 82–87% of the DD. This equates to an FPD<5µm of up to 25 mg from a size three capsule. This exceeded the target FPF<5µm of 5–20 mg. If comparable aerosol performance were observed in a multi-dose dry powder inhaler (MD-DPI) with a receptacle volume of 50 μL, an FPD<5µm of ∼4 mg could be achieved. This exceeds by an order of magnitude the highest FPD<5µm observed with MD-DPIs for the delivery of potent bronchodilators and inhaled corticosteroids for the treatment of asthma and chronic obstructive pulmonary disease.
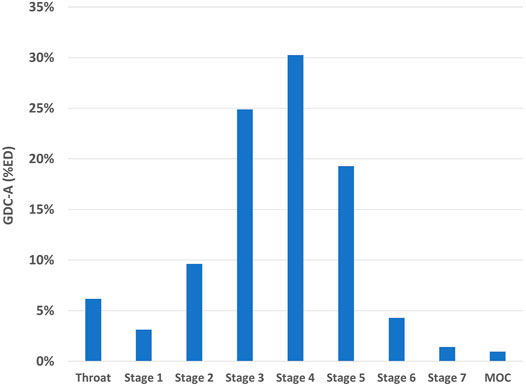
FIGURE 6. Aerodynamic particle size distribution of GDC-A PulmoSphere formulation (Batch R19015-1), as determined on a NGI operated at a flow rate of 54 L/min and a volume of 4 L.
In a separate experiment conducted at Genentech, the aerosol performance of neat GDC-A was assessed. The mean DD was just 48.0%. For the fraction of particles exiting the device, the MMAD was low (2.1 μm) and the FPF<5 μm expressed as a percentage of the DD was comparable to the PulmoSphere formulations (79.2%). Nonetheless, the low DD leads to a much lower FPF<5μm when expressed as a percentage of the nominal dose (i.e., 38.0 vs 71.0% R19017-3).
Delivered dose uniformity (DDU) of spray-dried GDC-A (Batch R19017) was determined at a 4 kPa pressure drop (N = 10). The mean delivered dose was 86.7% with a standard deviation of 4.4%; which suggests it would meet the FDA MDI/DPI guidance requirement for DDU.
Discussion
Benefits Relative to Solution-Based Spray Drying
Formulation of GDC-A as a crystalline solid using the suspension PulmoSphere process is expected to provide numerous benefits relative to formulation as an amorphous solid using conventional solution-based spray drying processes. These include improvements in physical and chemical stability, improvements in lung targeting due to the ability to sustain drug levels in the lungs, and minimization of potential tolerability issues associated with high dose delivery. These features are discussed in more detail below.
Improved physical and chemical stability
Maintaining physical and chemical stability of the drug substance is critical for spray-dried formulations over the life of the product (Weers and Miller, 2015; Chen et al., 2016; Shetty et al., 2020). Owing to the ultra-rapid timescale for droplet drying (i.e., milliseconds), drug that is dissolved in an aqueous feedstock and spray-dried will tend to be converted into amorphous domains in a spray-dried drug product (Vehring, 2008; Vehring et al., 2020). Even small amounts of amorphous content (∼1% w/w) can adversely impact chemical stability (Weers et al., 2019). The percentage of drug dissolved in the liquid feedstock (
Here,
Spray drying of solutions of GDC-A dissolved in an 80/20 w/w mixture of dichloromethane and methanol with DSPC as a shell-forming excipient (GDC-A/DSPC = 9/1 mol/mol) resulted in production of an amorphous solid (Shetty et al., 2021). When exposed to a temperature of 40 °C for a period of just 24 h, the amorphous solid partially recrystallized to Form E (16.3% w/w). The poor physical stability observed, albeit with no glass-forming excipients, points to the challenge of formulating GDC-A as an amorphous solid.
Improved lung targeting. GDC-A was engineered using inhaled-by-design principles to target the drug more effectively within the lungs, while minimizing off-target systemic effects. The features of the drug substance needed for optimal targeting to the lungs are opposite to those required for drugs administered orally (Yeadon, 2011). For an oral tablet, the goal is to maximize oral bioavailability and maintain drug concentrations in the systemic circulation for as long as possible. This is done by minimizing systemic clearance and binding to plasma proteins. In contrast, for an inhaled drug targeting the lungs, the goal is to minimize oral bioavailability as drug deposited in the mouth and throat will be swallowed and contribute to systemic effects if orally bioavailable. Another goal is to maximize the residence time in the lungs either by molecule engineering (i.e., a strategy with GDC-A), or by the development of inhaled sustained release dosage forms. Once absorbed, the goal is for the drug to be rapidly cleared and/or be bound to plasma proteins such that it does not contribute to systemic adverse events.
Owing to its low solubility, absorption of crystalline GDC-A from the lungs into the systemic circulation is expected to be dissolution limited. The Dose Number (Do) for an inhaled drug is given by Eq. (2) (Hastedt et al., 2016):
Where
Hence, increasing the residence time of GDC-A in the lungs may enable improved lung targeting and less frequent dosing. In contrast, the amorphous form of GDC-A spray-dried from an organic solvent would be expected to have a higher “apparent solubility” and more rapid dissolution (Almeida e Sousa et al., 2015), resulting in increased clearance rates and possibly the need for more frequent dose administration.
Improved dose consistency
In the early days of dry powder inhaler development, it was observed that neat, micronized drug particles had poor powder flow and fluidization properties. This was shown to negatively impact the precision in filling of powder into receptacles and in the emission of drug from the receptacle during inhalation of the dry powder. As a result, micronized drug particles were blended with coarse lactose carrier particles or pelletized into large agglomerates. These larger sized particles dramatically improved powder flow properties. Similar results were observed herein, where neat GDC-A powder was observed to have poor fluidization with a DD of just 48.0%.
It has been demonstrated that coating of crystalline drug particles with a shell-forming excipient not only improves powder flow and fluidization (as demonstrated herein), but it also leads to additional reductions in interpatient variability associated with dose delivery to the lungs (Son et al., 2021). The reductions in variability in lung delivery are the result of: 1) more effectively bypassing deposition in the upper respiratory tract where variability is driven by anatomical differences in the mouth and throat between individuals, and 2) decreases in the dependence of lung delivery with inspiratory flow rate. Due to its hydrophobic nature, neat GDC-A particles disperse effectively, but the neat drug particles would not be expected to balance the effects of increased flow rate on particle velocity and particle dispersion to achieve flow rate independent drug delivery (Miller et al., 2021). Indeed, high flow rate dependence has typically been observed with most neat drug formulations (Son et al., 2021).
Improved safety and tolerability
The decision to formulate the practically insoluble free base form of GDC-A may also have important implications from a safety/tolerability perspective, especially considering the dose requirements of the drug. It is worth noting that despite the slow clearance of drug from the lungs, both CIP and ABIP were well tolerated clinically (Kugler et al., 2007; Weers, 2019a).
Significant increases in adverse events associated with airway irritation (e.g., dyspnea, wheezing, bronchospasm, oropharyngeal pain, hoarseness, voice alteration, dysgeusia, and cough) have been observed as the nominal dose of inhaled therapeutics is increased beyond ∼10 mg (Sahakipijarn, et al., 2020; Chang et al., 2020). It has been hypothesized that the tolerability issues are associated with acute changes in ASL pH or ion composition (Sahakipijarn, et al., 2020; Chang et al., 2020; Godden et al., 1986; Lowry et al., 1988; Anderson, 1985). It has been suggested that the act of simply inhaling large masses of dry powders causes airway irritation irrespective of what is being inhaled (Hamed and Debonnett, 2017). However, a direct comparison of ciprofloxacin DPI (CIP) (Bayer, Berlin, Germany) and TOBI® Podhaler™ (Novartis, Basel, Switzerland) demonstrates that this is simply not the case (Sahakipijarn et al., 2020).
The aminoglycoside tobramycin has five primary amine groups. In TOBI Podhaler, free base tobramycin is titrated with sulfuric acid to form the salt. At neutral pH, approximately two–three of the amine groups have been titrated. The high dose of tobramycin (112 mg twice daily), and the large number of ions formed on dissolution, leads to acute changes in osmolality in airway surface liquid. This in turn results in increased airway irritation and sequelae including post-inhalation cough (Sahakipijarn et al., 2020). In contrast, formulation of ciprofloxacin as the zwitterion at neutral pH results in poor solubility and a low ion concentration despite the high dose (32.5 mg twice daily). The use of a poorly soluble neutral form of a drug and an insoluble phospholipid excipient minimizes acute changes in ASL osmolality and airway irritation, resulting in excellent tolerability with a low incidence of post-inhalation cough (Dorkin et al., 2015; McShane et al., 2018; Weers, 2019a). The poor solubility of GDC-A and use of a poorly soluble phospholipid excipient is expected to result in minimal airway irritation and tolerability issues.
The choice of using the free base form of GDC-A for development has another important implication from a tolerability perspective. GDC-A has two ionizable groups with pKa values of 2.7 and 8.9. Salt formation on the group with a low pKa could be problematic from a tolerability perspective. Formation of a stable salt typically requires that the ΔpKa between the basic group on the drug and the acid used to form the salt to be greater than ∼4 (Cruz-Capeza, 2012). Hence, a very strong acid, with a pKa <0 would be needed.
The form of the API that will ultimately be present in the lungs depends on the
where
Indeed, an increase in post-inhalation cough was observed for inhaled indacaterol maleate where disproportionation is favored (Donohue et al., 2011; Beasley et al., 2015). This was mitigated by using a weaker acid to form the salt (acetic acid) or by formation of a co-crystal (Beasley et al., 2015; Beeh et al., 2018). For the suspension-based PulmoSphere formulation of GDC-A, the drug is maintained as the free base. Hence, there is no potential for disproportionation to negatively impact ASL pH.
The PulmoSphere technology is currently utilized in three marketed products (TOBI Podhaler (TIP), Bevespi® Aerosphere, and Breztri® Aerosphere) (Geller et al., 2011; Miller et al., 2015; Doty et al., 2018; Israel et al., 2020). The excipient composition in each of these products is the same, a 2:1 M ratio of DSPC:CaCl2. The amount of excipient in the nominal dose depends on the potency of the drug and the nature of the delivery system. For the high-dose TOBI Podhaler drug product, the dose of excipients is ∼60 mg/day (Miller et al., 2015; Weers et al., 2019; Son et al., 2021).
Nonclinical studies with the PulmoSphere vehicle were conducted in rats and dogs at a dose of 12 mg/kg/day over a period of 26 weeks (Center for Drug Evaluation and Research Application Number 201688s000, 2016). No apparent toxicity was observed. The FDA Reviewer commented that there were: “No histopathologic changes in the respiratory tissues and no changes in the clinical chemistry parameters. DSPC and CaCl2 appear to be reasonably safe for repeated inhalation at the levels used in TIP”. The safety observed in animals has been substantiated over several years of experience in CF patients post-approval (Konstan et al., 2016; Hamed and Debonnett, 2017).
For GDC-A, the excipient dose is expected to be significantly less than in TOBI Podhaler. Assuming a 40 mg fill mass with a 70% drug loading of GDC-A administered once daily, the excipient burden would be 12 mg/day. This is about 5-fold less than the daily excipient dose in TOBI Podhaler.
Enabling High Dose Delivery
Son et al. categorized inhaled dry powders in terms of their TLD (Son et al., 2021). TLD values less than 1 mg were defined as low doses, TLD values between 1 and 20 mg were defined as moderate doses, those between 20 and 100 mg as high doses, and TLD values >100 mg were defined as ultrahigh doses. The GDC-A drug product with its target TLD of 5–20 mg falls within the moderate dose category. The results presented for the suspension PulmoSphere formulation herein demonstrate the ability of the suspension PulmoSphere powder to meet the target dose (based on using the FPD<5μm as a metric for TLD).
Son et al. also introduced a new metric for assessing high dose delivery termed the ‘product density’ (Son et al., 2021). The product density (
The three terms comprise: 1) the mass of powder (
For GDC-A batch R19015-2, a powder fill mass of 51.25 mg was achieved in a size three capsule (Vr = 0.30 ml), for a packing density of 170.8 mg/ml. This is greater than the packing density (131.1 mg/ml) achieved with the solution-based PulmoSphere formulation in the TOBI Podhaler drug product, and significantly greater than is achieved with the large porous particle formulation in Inbrija (52.6 mg/ml) (Geller et al., 2011; Son et al., 2021). It should be noted that the GDC-A powder was hand-filled versus the commercial products which are filled on a drum filler. With that said, our experience is that we are able to achieve even greater fill masses with a drum filler due to compression of the powder into pucks during filling. Hence, one would expect the improvements in packing density might be even greater. The drug loading in this formulation was 0.72 mg/mg, and the FPD<5μm was 0.68 mg/mg, resulting in a product density of 83.8 mg/ml. This is about 1.8-fold greater than is observed in TOBI Podhaler (47.1 mg/ml), and nearly 4-fold greater than Inbrija (22.1 mg/ml) (Son et al., 2021).
The achievable TLD from a single receptacle can be increased significantly by using larger sized capsules (assuming equivalent aerosol performance). For the product density of 83.8 mg/ml observed, the FPD<5μm increases to 31.0, 41.9, 57.0, and 79.6 mg in size 2 (Vr = 0.37 ml), size 1 (Vr = 0.50 ml), size 0 (Vr = 0.68 ml), and size 00 capsules (Vr = 0.95 ml), respectively. Perhaps of equal importance, an FPD<5μm of 4.2 mg can be achieved in a 50 μL blister, enabling moderate doses to be delivered in a multi-dose DPI. The potential fill mass in a size 00 capsule is ∼162 mg. This would require an inhaled volume of about 3.6 L to empty the powder contents, or between two and four inhalations depending on the patient population (Son et al., 2021).
This study did not explore drug loadings greater than 70% w/w. It remains to be seen whether the drug loading in suspension PulmoSphere powders can be increased further, and when the influence of the phospholipid shell on powder properties is lost. Buttini et al. demonstrated packing densities as high as 176.5 mg/ml and a product density of 104.4 mg/ml in spray-dried tobramycin base coated with sodium stearate (Buttini et al., 2018). They were able to deliver a TLD of ∼70 mg from a size 0 capsule. Pilcer et al. also achieved excellent aerosol performance with lipid-coated crystals of tobramycin base (Pilcer et al., 2009).
Hence, the formulation of crystalline drugs via suspension-based spray drying should be considered as a viable formulation alternative for the delivery of high doses of poorly soluble hydrophobic drugs, such as GDC-A.
Data Availability Statement
The original contributions presented in the study are included in the article/supplementary material, further inquiries can be directed to the corresponding authors.
Author Contributions
AE, AM, and JT defined the scope of the work and obtained funding for the study. TT, DM, JW, AE designed the study; TT, DM, and AEW executed powder manufacture and analytical testing; JW, TT, and DM interpreted the results and wrote the first draft of the paper. All authors reviewed the draft and provided comments.
Funding
Funding for the work presented herein was supplied by Genentech.
Conflict of Interest
AE, AM, and JT are employees of Genentech, receiving salaries and other compensation. TT, DM, AEW and JW conducted this work as a feasibility study while employees of Respira Therapeutics, Inc., and currently have no relationship with Genentech regarding the development of GDC-A.
Publisher’s Note
All claims expressed in this article are solely those of the authors and do not necessarily represent those of their affiliated organizations, or those of the publisher, the editors and the reviewers. Any product that may be evaluated in this article, or claim that may be made by its manufacturer, is not guaranteed or endorsed by the publisher.
References
Almeida e Sousa, L., Reutzel-Edens, S.M., Stephenson, G.A.,et al.(2015) Assessment of the Amorphous “Solubility” of a Group of Diverse Drugs Using New Experimental and Theoretical Approaches. Mol. Pharmaceutics 12, 484–495. doi:10.1021/mp500571m
Anderson, S. (1985). Issues in Exercise-Induced Asthma. J. Allergy Clin. Immunol. 76, 763–772. doi:10.1016/0091-6749(85)90745-6
Beasley, R. W., Donohue, J. F., Mehta, R., Nelson, H. S., Clay, M., Moton, A., et al. (2015). Effect of Once-Daily Indacaterol Maleate/mometasone Furoate on Exacerbation Risk in Adolescent and Adult Asthma: a Double-Blind Randomized Trial. BMJ Open. 5, e006131. doi:10.1036/bmjopen-2014-006131
Beeh, K. M., Kirsten, A.-M., Tanase, A.-M., Richard, A., Cao, W., Hederer, B., et al. (2018). Indacaterol Acetate/mometasone Furoate Provides Sustained Improvements in Lung Function Compared with Salmeterol Xinafoate/fluticasone Propionate in Patients with Moderate-To-Very-Severe COPD: Results from a Phase II Randomized, Double-Blind 12-week Study. Copd Vol. 13, 3923–3936. doi:10.2147/COPD.S179293
Brunaugh, A. D., and Smyth, H. D. C. (2018). Formulation Techniques for High Dose Dry Powders. Int. J. Pharmaceutics 547, 489–498. doi:10.1016/j.ijpharm.2018.05.036
Buttini, F., Balducci, A. G., Colombo, G., Sonvico, F., Montanari, S., Pisi, G., et al. (2018). Dose Administration Maneuvers and Patient Care in Tobramycin Dry Powder Inhalation Therapy. Int. J. Pharmaceutics 548, 182–191. doi:10.1016/j.ijpharm.2018.06.006
Center for Drug Evaluation and Research Application Number 201688s000 (2021). Pharmacology Reviews. Available at: https://www.accessdata.fda.gov/drugsatfda_docs/nda/2013/201688Orig1s000PharmR.pdf (Accessed November 21, 2021).
Chang, R. Y. K., Kwok, P. C. L., Ghassabian, S., Brannan, J. D., Koskela, H. O., and Chan, H. K. (2020). Cough as an Adverse Effect on Inhalation Pharmaceutical Products. Br. J. Pharmacol. 177, 4096–4112. doi:10.1111/bph.15197
Chen, L., Okuda, T., Lu, X.-Y., and Chan, H.-K. (2016). Amorphous Powders for Inhalation Drug Delivery. Adv. Drug Deliv. Rev. 100, 102–115. doi:10.1016/j.addr.2016.01.002
Cruz-Capeza, A. J. (2012). Acid-base Crystalline Complexes and the pKa Rule. Cryst. Eng. Comm. 14, 6362–6365.
Donohue, J., Singh, D., Kornmann, O., Lawrence, D., Lassen, C., and Kramer, B. (2011). Safety of Indacaterol in the Treatment of Patients with COPD. Copd 6, 477–492. doi:10.2147/COPD.S23816
Dorkin, H. L., Staab, D., Operschall, E., Alder, J., and Criollo, M. (2015). Ciprofloxacin DPI: a Randomized Placebo-Controlled, Phase IIb Efficacy and Safety Study on Cystic Fibrosis. BMJ Open Respir. Res. 2, e000100. doi:10.1136/bmjresp-2015-000100/2
Doty, A., Schroeder, J., Vang, K., Sommerville, M., Taylor, M., Flynn, B., et al. (2018). Drug Delivery from an Innovative LAMA/LABA Co-suspension Delivery Technology Fixed-Dose Combination MDI: Evidence of Consistency, Robustness, and Reliability. AAPS PharmSciTech. 19, 837–844. doi:10.1208/s12249-017-08910110.1208/s12249-017-0891-1
Fröhlich, E., Mercuri, A., Wu, S., and Salar-Behzadi, S. (2016). Measurements of Deposition, Lung Surface Area and Lung Fluid for Simulation of Inhaled Compounds. Front. Pharmacol. 7, 181. doi:10.3389/fphar.2016.00181
Geller, D. E., Weers, J., and Heuerding, S. (2011). Development of an Inhaled Dry-Powder Formulation of Tobramycin Using PulmoSphere Technology. J. Aerosol Med. Pulm. Drug Deliv. 24, 175–182. doi:10.1089/jamp.2010.0855
Godden, D. J., Borland, C., Lowry, R., and Higenbottam, T. W. (1986). Chemical Specificity of Coughing in Man. (Lond). 70, 301–306. doi:10.1042/cs0700301
Hamed, K., and Debonnett, L. (2017). Tobramycin Inhalation Powder for the Treatment of Pulmonary Pseudomonas aeruginosa Infection in Patients with Cystic Fibrosis: a Review Based on Clinical Evidence. Ther. Adv. Respir. Dis. 11, 193–209. doi:10.1177/1753465817691239
Hastedt, J. E., Bäckman, P., Clark, A. R., Doub, W., Hickey, A., Hochhaus, G., et al. (2016). Scope and Relevance of a Pulmonary Biopharmaceutical Classification System AAPS/FDA/USP Workshop March 16-17th, 2015 in Baltimore, MD. AAPS Open 2, 1. doi:10.1186/s41120-015-0002-x
Högger, P., Bonsmann, U., and Rohdewald, P. (1994). Efflux of Glucocorticoids from Human Lung Tissue to Human Plasma In Vitro. Eur. Respir. J. 7, 382s.
Israel, S., Kumar, A., DeAngelis, K., Aurivillius, M., Dorinsky, P., Roche, N., et al. (2020). Pulmonary Deposition of Budesonide/glycopyrronium/formoterol Fumarate Dihydrate Metered Dose Inhaler Formulated Using Co-suspension Delivery Technology in Healthy Male Subjects. Eur. J. Pharm. Sci. 153, 105472. doi:10.1016/j.ejps.2020.105472
Ivey, J. W., and Vehring, R. (2010). The Use of Modeling in spray Drying of Emulsions and Suspensions Accelerates Formulation and Process Development. Comput. Chem. Eng. 34, 1036–1040. doi:10.1016/j.compchemeng.2010.02.031
Kabalnov, A., Weers, J., Arlauskas, R., and Tarara, T. (1995). Phospholipids as Emulsion Stabilizers. 1. Interfacial Tensions. Langmuir 11, 2966–2974. doi:10.1021/LA00008A020
Konstan, M. W., Flume, P. A., Galeva, I., Wan, R., Debonnett, L. M., Maykut, R. J., et al. (2016). One-year Safety and Efficacy of Tobramycin Powder for Inhalation in Patients with Cystic Fibrosis. Pediatr. Pulmonol. 51, 372–378. doi:10.1002/ppul.23358
Kugler, A. R., Lee, J. D., Samford, L. K., Sahner, D. K., and Eldon, M. D. (2007). San Diego, 225–226.“Clinical Pharmacokinetics Following Multiple Doses of Amphotericin B Inhalation Powder (ABIP)”. in: Program and Abstracts of Focus on Fungal Infections (FoFI) 17
Lowry, R. H., Wood, A. M., and Higenbottam, T. W. (1988). Effects of pH and Osmolarity on Aerosol-Induced Cough in normal Volunteers. Clin. Sci. 74, 373–376. doi:10.1042/cs0740373
Martin, A. R., and Finlay, W. H. (2018). Model Calculations of Regional Deposition and Disposition for Single Doses of Inhaled Liposomal and Dry Powder Ciprofloxacin. J. Aerosol Med. Pulm. Drug Deliv. 31, 49–60. doi:10.1089/jamp.2017.1377
McShane, P. J., Weers, J. G., Tarara, T. E., Haynes, A., Durbha, P., Miller, D. P., et al. (2018). Ciprofloxacin Dry Powder for Inhalation (Ciprofloxacin DPI): Technical Design and Features of an Efficient Drug-Device Combination. Pulm. Pharmacol. Ther. 50, 72–79. doi:10.1016/j.pupt.2018.03.005
Miller, D. P., Tan, T., Tarara, T. E., Nakamura, J., Malcolmson, R. J., and Weers, J. G. (2015). Physical Characterization of Tobramycin Inhalation Powder: I. Rational Design of a Stable Engineered-Particle Formulation for Delivery to the Lungs. Mol. Pharmaceutics 12, 2582–2593. doi:10.1021/acs.molpharmaceut.5b00147
Miller, D. P., Tarara, T. E., and Weers, J. G. (2021). Targeting of Inhaled Therapeutics to the Small Airways: Nanoleucine Carrier Formulations. Pharmaceutics 13, 1855. doi:10.3390/pharmaceutics13111855
Paudel, A., Worku, Z. A., Meeus, J., Guns, S., and Van den Mooter, G. (2013). Manufacturing of Solid Dispersions of Poorly Water Soluble Drugs by spray Drying: Formulation and Process Considerations. Int. J. Pharmaceutics 453, 253–284. doi:10.1016/j.ijpharm.2012.07.015
Pilcer, G., Vanderbist, F., and Amighi, K. (2009). Spray‐dried Carrier‐free Dry Powder Tobramycin Formulations with Improved Dispersion Properties. J. Pharm. Sci. 98, 1463–1475. doi:10.1002/jps.21545
Sahakijpijarn, S., Smyth, H. D. C., Miller, D. P., and Weers, J. G. (2020). Post-inhalation Cough with Therapeutic Aerosols: Formulation Considerations. Adv. Drug Deliv. Rev. 165-166, 127–141. doi:10.1016/j.addr.2020.05.003
Shetty, N., Cipolla, D., Park, H., and Zhou, Q. T. (2020). Physical Stability of Dry Powder Inhaler Formulations. Expert Opin. Drug Deliv. 17, 77–96. doi:10.1080/17425247.2020.1702643
Shetty, N., Hou, J., Yanez, E., Shur, J., Cheng, J., Sun, C. C., et al. (in press). (2021). Effect of Lipidic Excipients on the Particle Properties and Aerosol Performance of High Drug Load spray Dried Particles for Inhalation. J. Pharm. Sci. doi:10.1016/j.xphs.2021.09.004
Sibum, I., Hagedoorn, P., de Boer, A. H., Frijlink, H. W., and Grasmeijer, F. (2018). Challenges for Pulmonary Delivery of High Powder Doses. Int. J. Pharmaceutics 548, 325–336. doi:10.1016/j.ijpharm.2018.07.008
Singh, A., and Van den Mooter, G. (2016). Spray Drying Formulation of Amorphous Solid Dispersions. Adv. Drug Deliv. Rev. 100, 27–50. doi:10.1016/j.addr.2015.12.010
Son, Y.-J., Miller, D. P., and Weers, J. G. (2021). Optimizing spray-dried Porous Particles for High Dose Delivery with a Portable Dry Powder Inhaler. Pharmaceutics 13, 1528. doi:10.3390/pharmaceutics13091528
Stass, H., Nagelschmitz, J., Willmann, S., Delesen, H., Gupta, A., and Baumann, S. (2013). Inhalation of a Dry Powder Ciprofloxacin Formulation in Healthy Subjects: A Phase I Study. Clin. Drug Investig. 33, 419–427. doi:10.1007/s40261-013-0082-0
Sun, W. J., Tristram-Nagle, S., Suter, R. M., and Nagle, J. F. (1996). Structure of Gel Phase Saturated Lecithin Bilayers: Temperature and Chain Length Dependence. Biophysical J. 71, 885–891. doi:10.1016/S0006-3495(96)79290-1
Thakral, N. K., and Kelly, R. C. (2017). Salt Disproportionation: A Material Science Perspective. Int. J. Pharmaceutics 520, 228–240. doi:10.1016/j.ijpharm.2017.02.001
Vehring, R. (2008). Pharmaceutical Particle Engineering via spray Drying. Pharm. Res. 25, 999–1022. doi:10.1007/s11095/-007-9475-110.1007/s11095-007-9475-1
Vehring, R., Snyder, H., and Lechuga‐Ballesteros, D. (2020). “Spray Drying,” in Drying Technologies for Biotechnology and Pharmaceutical Applications. Editors S. Ohtake, K. Izutsu, and D. Lechuga-Ballesteros (Weinheim, Germany: Wiley), 179–216. doi:10.1002/9783527802104.ch7
Weers, J. (2019a). Comparison of Phospholipid-Based Particles for Sustained Release of Ciprofloxacin Following Pulmonary Administration to Bronchiectasis Patients. Pulm. Ther. 5, 127–150. doi:10.1007/s41030-019-00104-6
Weers, J. G., and Miller, D. P. (2015). Formulation Design of Dry Powders for Inhalation. J. Pharm. Sci. 104, 3259–3288. doi:10.1002/jps.24574
Weers, J. G., Miller, D. P., and Tarara, T. E. (2019). Spray-dried PulmoSphere Formulations for Inhalation Comprising Crystalline Drug Particles. AAPS PharmSciTech 20, 103. doi:10.1208/s12249-018-1280-0
Weers, J., and Tarara, T. (2014). The PulmoSphere Platform for Pulmonary Drug Delivery. Ther. Deliv. 5, 277–295. doi:10.4155/tde.14.3
Wong, J. P., Yang, H., Blasetti, K. L., Schnell, G., Conley, J., and Schofield, L. N. (2003). Liposome Delivery of Ciprofloxacin against Intracellular Francisella Tularensis Infection. J. Controlled Release 92, 265–273. doi:10.1016/S0168-3659(03)00358-4
Keywords: PulmoSphere™, dry powder Inhalers, spray drying, high dose delivery, drug loading, GDC-A
Citation: Tarara TE, Miller DP, Weers AE, Muliadi A, Tso J, Eliahu A and Weers JG (2022) Formulation of Dry Powders for Inhalation Comprising High Doses of a Poorly Soluble Hydrophobic Drug. Front. Drug. Deliv. 2:862336. doi: 10.3389/fddev.2022.862336
Received: 25 January 2022; Accepted: 18 February 2022;
Published: 15 March 2022.
Edited by:
Philip Chi Lip Kwok, The University of Sydney, AustraliaReviewed by:
Zhenbo Tong, Southeast University, ChinaFrancesca Buttini, University of Parma, Italy
Nitesh K. Kunda, St. John’s University, United States
Copyright © 2022 Tarara, Miller, Weers, Muliadi, Tso, Eliahu and Weers. This is an open-access article distributed under the terms of the Creative Commons Attribution License (CC BY). The use, distribution or reproduction in other forums is permitted, provided the original author(s) and the copyright owner(s) are credited and that the original publication in this journal is cited, in accordance with accepted academic practice. No use, distribution or reproduction is permitted which does not comply with these terms.
*Correspondence: Avi Eliahu, ZWxpYWh1LmF2aUBnZW5lLmNvbQ==; Jeffry G. Weers, andlZXJzQGN5c3RldGljbWVkaWNpbmVzLmNvbQ==
†These authors share first authorship