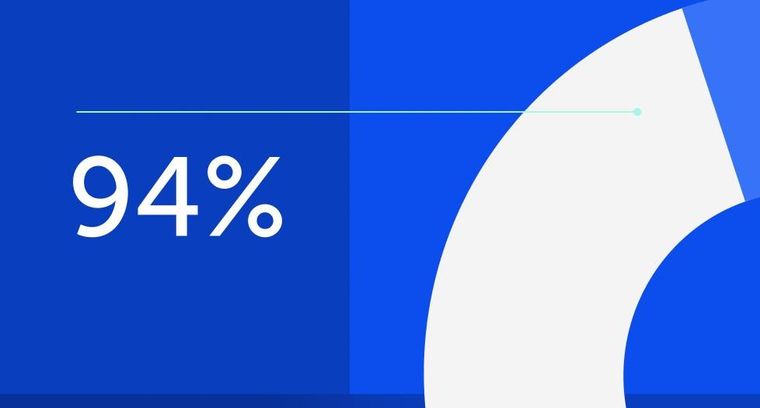
94% of researchers rate our articles as excellent or good
Learn more about the work of our research integrity team to safeguard the quality of each article we publish.
Find out more
OPINION article
Front. Drug Deliv., 22 March 2022
Sec. Respiratory Drug Delivery
Volume 2 - 2022 | https://doi.org/10.3389/fddev.2022.855234
This article is part of the Research TopicStrategies to overcome the barriers to effective inhaled treatmentsView all 7 articles
A commentary has been posted on this article:
Commentary: Suboptimal Inspiratory Flow Rates With Passive Dry Powder Inhalers: Big Issue or Overstated Problem?
The maximum inspiratory pressure (MIP) that a subject can achieve through the mouthpiece of a “passive” dry powder inhaler (DPI) is driven chiefly by their inspiratory muscle strength (Clark, 2015). Muscle strength increases with age, peaking at about age 25, plateauing until about age 40, after which it steadily decreases. Males achieve greater MIP values than females, and increases in disease severity may further reduce MIP. When using DPIs, patients rarely inhale with maximal effort, instead achieving peak inspiratory pressures (PIP) that are about 40–80% of their MIP (Clark, 2015 and references therein).
Based on these observations, current industry guidance is that passive DPIs are inherently flow rate dependent, and that young children and elderly patients may not be able to achieve the PIP or peak inspiratory flow rates (PIFR) necessary to effectively fluidize and disperse dry powders, especially during acute exacerbations (Laube et al., 2011).
For patients with COPD, it has been suggested that: “If the PIFR is less than 60 L min−1 the patient may not achieve optimal clinical benefit (with inhaled bronchodilators), and a different delivery system such as a metered dose or soft mist inhaler or nebulized therapy should be considered” (Mahler, 2017). Based on results of multiple breathing studies in COPD patients, it was further suggested that between 19 and 78% of stable outpatients, and 32–47% of in-patients prior to discharge after admission for an exacerbation, have a suboptimal PIFR <60 L min−1 (Mahler et al., 2013; Mahler, 2017; Mahler, 2020). More recently, Mahler has taken the argument one step further, suggesting that PIFR be used as a therapeutic biomarker to guide delivery system selection, while dropping the optimal flow rate for high resistance DPIs to 30 L min−1 (Mahler and Halpin, 2021).
This opinion reviews the available literature regarding flow rate dependence of inhaled bronchodilators when administered with passive DPIs for the treatment of asthma and COPD.
According to the ERS/ISAM Guidance on inhalation therapies (Laube et al., 2011): “Each DPI has a minimum threshold energy below which deagglomeration is inefficient, resulting in a reduced emitted dose (ED) with a high mass median aerodynamic diameter (MMAD) and small fine particle dose less than 5 μm (FPD < 5μm). Below the minimum threshold energy, the patient will receive no, or very little, therapeutic effect from the drug”.
The Guidance further states that: “The turbulent energy (in a DPI) is the product of the patient’s inhalation flow
Stated another way, the flow rate through a DPI is proportional to the square root of the pressure drop the patient achieves across it, with the constant of proportionality being the device resistance.
Different DPIs contain design elements, such as grids, orifices and swirl chambers, that present varying degrees of airflow resistance. It has been suggested in numerous publications regarding passive DPIs that subjects must inhale with enough flow to overcome the resistance of the device (Ghosh et al., 2017; Mahler, 2017; Baloira et al., 2021). This along with the proposed optimal PIFR being independent of device resistance suggests that achieving a flow rate of 60 L min−1 is necessary for effective powder fluidization and dispersion from a passive DPI. This suggestion is fundamentally incorrect. For higher resistance inhalers, effective powder dispersion and drug delivery can be achieved at flow rates much less than 60 L min−1, or even 30 L min−1 for that matter. Similarly, the effectiveness of powder dispersion and the minimum PIP cannot be established a priori from the product of flow rate and device resistance, as the degree of dispersion depends on key design features of both the formulation and device.
Establishing the minimally acceptable flow rate or pressure drop for an inhaled dry powder requires linking the results of three independent experimental evaluations: 1) measurement of the inspiratory flow profiles of subjects (i.e., breathing studies) when using the DPI. The use of PIP enables a single metric that is independent of device resistance to describe the inspiratory effort that a subject provides (Clark et al., 2020); 2) measurement of the impact of a subject’s inspiratory flow profile on drug delivery to the lungs; 3) measurement of the impact of regional deposition of the drug within the respiratory tract on clinical outcomes.
To determine whether decreases in the total lung dose (TLD) of the drug are critical, one must understand where on the sigmoidal dose-response curve the dose sits. If the TLD sits at the top of the curve, some reduction in TLD may be possible without sacrificing efficacy. Inhaled bronchodilators are often described as having a high therapeutic index, thereby enabling higher doses to be administered without fear of serious adverse events. Hence, the determination of the minimum threshold energy should rely first and foremost on clinical measures of efficacy as a function of flow rate. Unfortunately, this type of data is in short supply, with most of it generated more than 25 years ago on first-generation DPI products comprising short-acting bronchodilators. Nonetheless, it is this data that has shaped current industry perceptions regarding passive DPIs.
In the absence of clinical efficacy, it is impossible to know whether measures of drug delivery will correlate with clinical outcomes. If differences in TLD are to be relied upon, it is preferred that these measurements come from in vivo clinical studies (i.e., imaging or charcoal block pharmacokinetics methods). Alternatively anatomical throat models (e.g., the Alberta Idealized Throat, AIT), particularly when using realistic inspiratory flow profiles, have demonstrated good in vitro/in vivo correlations (Zhang et al., 2007; Byron et al., 2010; Delvadia et al., 2012; Olsson et al., 2013; Finlay and Martin, 2015; Weers et al., 2015; Ruzycki et al., 2019; Newman and Chan, 2020; Ruzycki et al., 2020). The last choice for estimates of TLD should be cascade impactors. As pointed out by Dolovich et al., “cascade impactors are not lung simulators”, and in vivo deposition of the inhaled drug dose and ultimately the clinical response to the inhaled dose are far more complicated (Dolovich et al., 2018). Unfortunately, most of the conclusions to date regarding the optimal PIFR of DPIs have been based on FPD<5μm, a metric which provides a misleading picture of flow rate dependence with DPIs (Weers and Clark, 2017; Weers, 2018; Weers et al., 2019b). Moreover, use of a single metric like PIFR or PIP to describe drug delivery omits many other critical factors including inhaled volume, ramp rate to peak flow, and other patient related features including the geometry of their airways and degree of airway obstruction or gas trapping, to name a few).
To achieve drug delivery to the lungs, particles must bypass deposition in the upper respiratory tract (URT). It is well accepted that particle deposition within the URT occurs primarily by inertial impaction (Stahlhofen et al., 1989; Darquenne, 2020). The Stokes number (Stk) defines the tendency that a particle will diverge from the airflow and deposit by inertial impaction in the URT (Equation 1):
Here
Pharmaceutical impactors are designed to separate particles onto stages based on
Use of
To achieve lung deposition that is independent of flow rate in vivo, it is
The magnitude of flow rate dependence can be estimated using a metric termed the Q index (Weers and Clark, 2017). The Q index represents a normalized measure of the percentage change in TLD between pressure drops of 1 and 6 kPa. The sign indicates whether the TLD increases (+) or decreases (-) with increasing PIP. Q index values between 0 and 15% are deemed to be indicative of low flow rate dependence, between 15 and 40% as moderate, and >40% as high flow rate dependence. If a Next Generation Impactor (NGI) is used to estimate the Q index, deposition on stage 3 to filter (i.e., FPDS3-F) may be used as an approximation of the TLD.
It is sometimes stated that active devices such as pMDIs and SMIs are flow rate independent (Ghosh et al., 2017). While it is true that these devices do not depend on the patient’s inspiratory effort to create the aerosol, it is incorrect to suggest that they provide flow rate independent dose delivery to the lungs. In fact, active devices are inherently flow rate dependent, because while they create a constant aerodynamic particle size distribution independent of the patient, patients inhale the aerosol at different flow rates, resulting in a negative flow rate dependence. This was exemplified in clinical studies for numerous active delivery systems including the Exubera® DPI, Spiros™ DPI, and Respimat® SMI (Hill et al., 1996; Weers and Clark, 2017; Weers, 2018; Weers et al., 2019b; Weers et al., 2019c; Ciciliani et al., 2021)). Metered dose inhalers may show less flow dependence than other active delivery systems due to interactions of the plume with the entraining air flow.
Paradoxically, the only portable inhalers capable of achieving true flow rate independent dose delivery to the lungs are passive dry powder inhalers. Indeed, carrier-free dry powder formulations comprising spray-dried porous particles have repeatedly demonstrated the ability to balance the two competing effects with passive DPIs with increasing
For early DPI products such as the Ventolin® Rotacaps®, Intal® Spinhaler®, Foradil® Aerolizer®, and Bricanyl® Turbuhaler®, large decreases in
With this as background, let’s examine the flow rate dependence of several marketed DPIs utilized for the treatment of asthma and COPD. For this discussion, we have selected four representative DPIs with marked variations in their resistance to airflow.
Unfortunately, there are no measures of efficacy as a function of variation in flow rate available for the Breezhaler, so the determination of the minimum PIFR or PIP must rely on aerosol performance metrics and breathing studies only.
As discussed above, a common misconception with DPIs is that: “In vitro testing of various drugs has demonstrated that higher flow rates generate smaller particle sizes, thereby allowing for better drug deposition in the lungs” (Ghosh et al., 2017). As formulations of lactose blends have advanced with the addition of fine lactose and/or force control agents, the magnitude of the decrease in particle size with variations in flow rate has diminished, with lactose blends now showing positive, negative and even flow rate independent drug delivery (Hamilton et al., 2015; Weers and Clark, 2017).
Indeed, a moderate negative flow rate dependence was observed for OnBrez® Breezhaler® (Q index = -31.0%) (Weers and Clark, 2017), with increases in TLD of indacaterol (as determined in the AIT model) from 29% of the nominal dose at a PIP of 6 kPa (128 L min−1), to 33% at 4 kPa (104 L min−1), to 40% at 2 kPa (73 L min−1), to 42% at 1 kPa (53 L min−1) (Ung et al., 2014; Weers et al., 2015). Given that the highest TLD is observed at 1 kPa, it seems likely that drug delivery will continue to be acceptable at PIPs well below 1 kPa. As anticipated, measures based on FPD<5μm are misleading, showing a small in vitro flow rate dependence with increases in FPD<5μm with increasing
Pavkov et al. studied the breathing profiles of 26 COPD patients with the Breezhaler device (Pavkov et al., 2010). The PIFR decreased with increases in disease severity, from 103 L min−1 for subjects with mild disease to 84 L min−1 for subjects with very severe disease. Across the 26 subjects, PIFR values ranged from 52 to 133 L min−1, corresponding to PIP values ranging from about 1 to 6 kPa.
Altman et al. measured the PIFRs achieved with Breezhaler in 97 COPD patients (Altman et al., 2018). The mean PIFR was 108 ± 23 L min−1 with a range from 54 to 156 L min−1. Optimal flow was defined by Haidl et al. based on the Pavkov results as being 50 L min−1 (Haidl et al., 2016). This flow rate corresponds to a PIP of 0.90 kPa, i.e., consistent with a 1 kPa cutoff proposed by Clark et al. for effective delivery (Clark et al., 2020). Hence, all subjects in the breathing studies described above achieved the PIP needed for effective drug delivery.
Two additional questions worthy of reflection are: 1) Should patients be instructed to inhale forcefully with the Breezhaler, as this is likely to increase URT deposition and lower the TLD? 2) If someone inhales optimally at a PIP of 1 kPa, will this be judged as a critical error in observational studies?
Nielsen et al. studied the clinical effects of the long-acting β-agonist salmeterol delivered with the Diskus DPI in children ages 8–15 years diagnosed with exercise-induced asthma (Nielsen et al., 1998). Changes in FEV1 were measured during exercise challenges at 1 and 12-h post-administration. Subjects inhaled either placebo, or 50 mg of salmeterol at 30 L min−1 or 90 L min−1. The drop in FEV1 during exercise was significantly less for the subjects receiving inhaled salmeterol compared to placebo at both timepoints. No difference in the protection afforded by salmeterol was observed on the low-flow rate day, as compared with the high flow-rate day, resulting in a minimum effective PIP of about 0.58 kPa (30 L min−1).
In the same study, Nielsen et al. demonstrated that 99% of children between the ages of 3 and 10 years were able to achieve a PIFR of at least 30 L min−1, with 26% of subjects exceeding a flow rate of 90 L min−1 (PIP = 5.2 kPa) (Nielsen et al., 1998). Numerous other breathing studies conducted in asthma subjects have demonstrated that children above the age of three can achieve a PIFR of at least 30 L min−1 even during periods of acute wheeze (Bentur et al., 2004; Amirav et al., 2005; Baba et al., 2011).
Additional breathing studies have been conducted in COPD patients with the Diskus DPI (Van der Palen, 2003; Broeders et al., 2004; Mahler et al., 2014; Sharma et al., 2017; Ghosh et al., 2019). In one study, Sharma et al. measured a mean PIFR of 71 ± 22 L min−1 (PIP = 3.3 kPa) in 268 subjects with COPD following an exacerbation (Sharma et al., 2017). In this group, 31% of subjects had a PIFR less than 60 L min−1 (2.3 kPa), i.e., less than what is optimal according to Mahler’s convention (Mahler, 2017; Mahler and Halpin, 2021). Only 5.6% of subjects had a PIFR less than 40 L min−1 (PIP = 1.0 kPa). No difference in readmission rates were observed for the group classified as having suboptimal PIFR (<60 L min−1) versus the group having optimal PIFR (Sharma et al., 2017).
In another study, Ghosh et al. determined the PIFR of 66 COPD patients with the Diskus DPI (Ghosh et al., 2019). The median PIFR was 64 L min−1, with a range from 43 to 75 L min−144% of subjects were assessed as having suboptimal PIFR less than 60 L min−1, with none having a PIFR <30 L min−1.
Broeders et al. followed the changes in PIFR during an acute exacerbation of COPD in 15 subjects (Broeders et al., 2004). The mean PIFR was 86 ± 6.6 (range: 44–131) L min−1 during the acute phase on Day 1. Mean PIFR increased to 95 ± 7.9 (range: 49–140) L min−1 during the convalescence period on Day 5, and to 101 ± 7.6 (range: 51–134) L min−1 on Day 50 (stable phase). Again, none of these subjects had a PIFR <30 L min−1, even during periods of acute exacerbation.
Ung et al. determined the TLD of Advair Diskus at PIPs of 4 and 2 kPa using the AIT model (Ung and Chan, 2016). For salmeterol, the TLD decreased from 17 to 16% with decreases in PIP (Q index = +13.9%). Over the same range of PIP values, the TLD of fluticasone propionate decreased from 21 to 19% (Q index = +21.7%). These results suggest TLD values of about 15 and 17% for salmeterol and fluticasone, respectively at a 1 kPa pressure drop (extrapolated). Impactor results presented by Kamin et al. (42) suggest that drug delivery is maintained down to flow rates as low as 18 L min−1 (0.25 kPa) (Kamin et al., 2002). This coupled with results from other groups (43,44), suggests that there will be no significant drop in drug delivery at a PIFR of 30 L min−1 (Chrystyn, 2007; Buttini et al., 2016).
Haidl et al. defined the optimal flow rate with the Diskus DPI as being a PIFR ≥30 L min−1, corresponding to a PIP of ∼0.6 kPa (Haidl et al., 2016).
By most accounts, the Turbuhaler device with its pelletized formulation technology has the greatest flow rate dependence of any commercial DPI. The mean Q index for six independent studies was +65.4 ± 10.1% (Weers and Clark, 2017). There are at least five clinical studies that have explored the impact of inspiratory flow rate on the therapeutic effect of terbutaline delivered from the Turbuhaler (Dolovich et al., 1988; Engel et al., 1990; Pedersen et al., 1990; Newman et al., 1991; Drblik et al., 2003). Numerous other breathing studies provide guidance on the PIFR and PIP that patients typically achieve with the reservoir-based device (Brown et al., 1995; Persson et al., 1997; Dewar et al., 1999; Broeders et al., 2004; Amirav et al., 2005; Al-Showair et al., 2007; Von Berg et al., 2007; Baba et al., 2011).
Pedersen et al. studied the influence of inspiratory flow rate on improvements in lung function following administration of 0.25 mg of terbutaline to fourteen asthmatic children with a mean age of 7.4 years (range: 4–13 years) (Pedersen et al., 1990). All inhalations resulted in significant improvements in FEV1 relative to baseline. Bronchodilation increased with increases in mean PIFR between 13 and 30 L min−1. No additional improvement in bronchodilation was observed when the mean PIFR was increased to 60 L min−1 relative to that achieved at 30 L min−1 (PIP ∼0.88 kPa).
Pedersen et al. also conducted breathing studies in 265 healthy children between the ages of 3.5 and 15 years, and in 34 asthmatic children between the ages of 4 and 13 years during an episode of acute wheeze (Pedersen et al., 1990). For healthy children below the age of 6, 15/57 (26%) did not achieve a PIFR of 28 L min−1, whereas 207/208 (99.5%) of children ages 6 and older did. For the acute asthma subjects (ages 4 and 5), 6/15 (40%) could not achieve a PIFR of 28 L min−1 (mean: 24 L min−1), whereas 17/19 (89%) of children between the ages of 6 and 13 did (mean: 39 L min−1). It was concluded that children ages 6 and above could achieve effective bronchodilation at flow rates of 30 L min−1 and above.
In another study, Newman et al. utilized gamma scintigraphy to determine deposition of terbutaline in the respiratory tract of ten asthmatic adults between the ages of 22 and 78 years (Newman et al., 1991). Lung deposition was reduced from 16.8% at a mean PIFR of 56.6 L min−1, to 9.1% at a PIFR of 27.9 L min−1. Despite the significant reduction in TLD, no differences in bronchodilation were observed between the “fast” and “slow” PIFRs. This suggests that a TLD of 9.1% sits on the flat part of the dose response curve. This points to the challenge with using deposition results only in choosing an adequate lung dose (Nagel et al., 2021).
Dolovich et al. also observed comparable changes in FEV1 following inhalation of terbutaline at 60 and 30 L min−1 with the Turbuhaler in adult asthmatic patients (Dolovich et al., 1988). So too did Engel et al., who observed comparable bronchodilation between PIFRs of 34 and 88 L min−1 in ten adult asthmatics (mean age of 25 year, range 19–40 year) (Engel et al., 1990).
Finally, Drblik et al. compared the efficacy of terbutaline delivered by Turbuhaler with that of a pressurized metered dose inhaler coupled with a Nebuhaler spacer in 112 children (ages 6–16 years) during acute asthma attacks (Drblik et al., 2003). No significant differences were observed between the two treatments, with improvements in FEV1 by 49 and 50% at 60 min, respectively. They further showed that the improvements in FEV1 with the Turbuhaler were independent of PIFR above 30 L min−1, the minimum PIFR achieved by all 112 subjects in the study. In this study, switching to an active device provided no additional clinical benefit.
Numerous other studies have examined the inspiratory flow profiles of COPD patients with the Turbuhaler both during stable disease and during acute exacerbations. For example, Broeders et al. studied the impact of an acute exacerbation on the PIFR generated with the Turbuhaler in 15 subjects with COPD (Broeders et al., 2004). The mean PIFR on Day 1 (acute phase) was 59 ± 4.7 (range: 33–88) L min−1. It improved to 67 ± 5.0 (range: 34–96) L min−1 on Day 5 (convalescence stage), and to 72 ± 5.2 (range: 39–93) L min−1 on Day 50 (stable phase). All of the subjects achieved a PIFR of at least 33 L min−1.
In total, these studies suggest that efficacy with the Turbuhaler is maintained down to a PIFR of 30 L min−1 (0.88 kPa), and that some degree of bronchodilation is maintained down to PIFR as low as 13 L min−1 (0.17 kPa).
Despite the clinical results demonstrating equivalent bronchodilation down to 30 L min−1, Haidl et al. suggest that the optimal PIFR for Turbuhaler is above 60 L min−1, with borderline performance between 30 and 60 L min−1 (Haidl et al., 2016). If the criterion is “little or no clinical effect” as defined by Laube et al., then the minimum PIP should be ∼0.88 kPa, if not lower (Laube et al., 2011). Virtually all subjects over the age of 6 can achieve this pressure drop, even during acute exacerbations.
It has been suggested in numerous publications that subjects must inhale with enough flow to overcome the internal resistance of the device (Ghosh et al., 2017; Mahler, 2017). It is further stated that “high internal resistance impacts the PIFR generated and needed for drug dispersion” (Ghosh et al., 2017). To reiterate, the resistance of a DPI and the PIFR achievable through the device tells one nothing about the dispersion forces generated within the device. In fact, the reason that many DPIs have a high internal resistance is that they contain design elements (e.g., orifices, swirl flow) that increase dispersion forces and particle velocity within the inhaler. For example, despite having a significantly lower PIFR, particles exiting the mouthpiece of the high resistance Handihaler DPI have a two-fold higher velocity and greater turbulence than do particles exiting the low resistance Breezhaler DPI (Weers et al., 2019a).
High flow rates are not a prerequisite for effective drug delivery to the lungs. Recent studies with a high resistance variant of the RS01 DPI (R = 0.051 kPa0.5 L−1 min) showed TLD values in the AIT of 93%, with flow rate independent delivery of an inhaled corticosteroid between PIP values of 1 (19.6 L min−1) and 6 kPa (48.0 L min−1) (Q index = -3.9%) (Miller et al., 2021).
Like the Breezhaler, Spiriva Handihaler shows a negative flow rate dependence between PIPs of 2 and 6 kPa (Ung et al., 2014). The TLD in Spiriva Handihaler as determined with the AIT model increased from 16% of the nominal dose at 47 L min−1 (6 kPa), to 19% at 39 L min−1 (4 kPa), to 20% at 28 L min−1 (2 kPa). This equates to a Q index of -31.0%. The extrapolated TLD value at a PIP of 1 kPa is 22%. The TLD values measured with the Alberta Idealized Throat were comparable to previous in vivo studies using gamma scintigraphy, where Brand et al. observed about 20% lung deposition for Spiriva Handihaler in COPD patients independent of the degree of disease severity (Brand et al., 2013).
Chodosh et al. determined FPD<5μm values for Spiriva Handihaler of 2.94, 3.92, 4.21, 4.56, 4.37 mg at PIFRs of 20, 28.3, 40, 50, and 60 L min−1, respectively (Chodosh et al., 2001). The relatively constant FPD<5μm values noted between 28.3 and 60 L min−1 is consistent with the negative flow rate dependence observed in the anatomical throat model. A drop of ∼25% in FPD<5μm was observed between flow rates of 28.3 and 20 L min−1. Based on these results, the TLD value at 20 L min−1 is likely to be less than the extrapolated value from the AIT data detailed above. The drop in FPD<5μm is balanced to some degree by the low PIFR, likely resulting in a TLD value comparable to those observed at the flow rates between 40 and 50 L min−1. Hence, effective drug delivery is anticipated down to PIFRs of about 20 L min−1 corresponding to a PIP of ∼1 kPa.
Ghosh et al. determined the PIFR of 66 COPD patients with the In-Check DIAL when using the high resistance Handihaler DPI (Ghosh et al., 2019). The median PIFR was 36 L min−1 with a range from 28 to 39 L min−1. Suboptimal delivery, defined as PIFR <30 L min−1 encompassed 32% of the subjects.
Altman et al. [32] studied the breathing profiles of 97 COPD patients with the Handihaler device. The mean PIFR was 49 ± 9 L min−1, with PIFRs ranging from 22 to 70 L min−1 (Altman et al., 2018). Hence, all subjects in the Ghosh and Altman studies exceeded a 1 kPa pressure drop.
In the conclusion to their study, Altman et al. stated: “The results showed that mean PIFR values increased, and mean pressure drop values at the PIFR decreased with decreasing airflow resistance of the inhalers. Patients with COPD were able to inhale with the least inspiratory effort and generate the highest mean PIFR value through the Breezhaler inhaler when compared with the Ellipta inhaler and the HandiHaler inhaler” (Altman et al., 2018). This statement implies that higher PIFRs are somehow beneficial, which is clearly not the case with the Breezhaler or the Handihaler, and many other newer generation formulations. It also implies that patients must give greater effort when using a high resistance DPI. In fact, patients tend to give more effort naturally when inhaling against a higher resistance for the same instruction set (De Koning et al., 2002; Tiddens et al., 2006; Azouz et al., 2015; Weers et al., 2019c; Sahay et al., 2021). For lower resistance DPIs (e.g., Rotahaler®), there is often far greater variability in PIFR with more patients achieving suboptimal PIFR (Pedersen, 1986; Weers and Clark, 2017; Weers et al., 2019c). Having patients supply greater and more consistent effort for a given instruction is a good thing from a “human factors” perspective, increasing the probability for effective drug delivery at low PIP. The best form of instruction is one that need not be given because the subjects perform the inspiratory maneuver naturally.
Haidl et al. suggested that the minimum required PIFR for Handihaler is 20 L min−1, but that the optimal PIFR is 30 L min−1 (Haidl et al., 2016). This is based primarily on the misleading lower FPD<5μm observed at 20 L min−1. In the spirit of the minimum threshold energy concept where PIPs less than the minimum threshold energy result in little or no therapeutic benefit, a PIP of 1 kPa is deemed to be appropriate.
Overall, the 1 kPa cutoff proposed by Clark et al. provides an acceptable metric for the minimum threshold energy or PIP for effective drug delivery with passive dry powder inhalers of asthma/COPD therapeutics (Clark et al., 2020). Moreover, most subjects above a target age in children can achieve a PIP of 1 kPa, even during acute exacerbations. As such, the high percentage of suboptimal inhalations suggested by Mahler, Ghosh, and others is overstated (Ghosh et al., 2017; Mahler, 2017; Mahler and Halpin, 2021).
One study that is used as support for the 60 L min−1 cutoff was conducted by Loh et al. (Loh et al., 2017). In this retrospective analysis, 123 COPD patients were subdivided into two groups based upon their PIFR when using the In-Check dial measured on the no resistance setting. 52% of the subjects at the time of discharge following a pulmonary exacerbation had a PIFR <60 L min−1. When compared to the optimal group, the suboptimal group had fewer days to all-cause admission (65.5 vs 101 days, p = 0.009). Ghosh et al. questioned whether the results may be more related to the disease process, and that PIFR may merely be a marker of exacerbation severity (Ghosh et al., 2017). Another curious aspect of this study is that the 60 L min−1 flow rate corresponds to a PIP of just 0.09 kPa on the no resistance (R ∼ 0.005 kPa0.5 L−1 min) setting. No other breathing study has suggested that COPD patients achieve this low of a PIP even during an acute exacerbation. Hence, it is difficult to assess what these results mean in the context of identifying an optimal PIFR.
It is important to point out that use of PIP as opposed to PIFR allows for the impact of device resistance to be accounted for in a single metric. The challenge with using PIFR is illustrated in Figure 1. For a 60 L min−1 PIFR, the corresponding PIP is nearly 10 kPa with Handihaler versus just 1.3 kPa with Breezhaler. Most COPD patients cannot achieve a PIP of 10 kPa, whereas most can achieve a PIP of 1.3 kPa. The percentage of subjects considered as having suboptimal delivery by Mahler is directly related to the PIP they are being asked to achieve. The reliance on flow rate as opposed to pressure drop and aerodynamic size as opposed to impaction parameter leads to many misconceptions about how we design, develop, and ultimately use passive DPIs in clinical practice.
FIGURE 1. Plot of the PIFR versus PIP for four portable dry powder inhalers with varying device resistance. The circles on each curve represent the minimum PIP or PIFR for effective drug delivery based on the data presented herein. The triangles represent the corresponding PIP values for individual inhalers at a PIFR of 60 L min−1.
The pharmaceutical industry has recently focused on reducing interpatient variability in drug delivery of inhaled aerosols by increasing patient adherence to the prescribed treatment regimen, and by reducing patients’ inability to correctly utilize their DPI (e.g., to achieve an optimal PIFR) (Smith et al., 2010; Newman, 2017). An additional challenge, and one that is largely unaddressed in current marketed asthma/COPD products, is that patients can utilize their device correctly and be fully adherent to the treatment regimen, and still achieve little or no benefit from the drug due to the anatomical features of their mouth and throat. This is especially problematic for current DPIs, as a high percentage of the delivered dose (50–90%) is deposited in the URT.
Borgström et al. demonstrated that for TLD values between 10 and 30% of the metered dose, that mean interpatient variability in TLD is between 30 and 50% (Borgström et al., 2006). Moreover, Stahlhofen et al. showed that individual patients may deposit anywhere from between 5 and 90% of their dose in the URT over a narrow range of
Let’s put the magnitude of the mean interpatient variability related to oropharyngeal filtering of particles in context relative to the measured flow rate dependence. Using the measured Q index values, the percent changes in TLD between 1.0 and 6.0 kPa were: 2.9, 13.9, 25 and 31% for bronchodilators administered with the Genuair, Diskus, Handihaler and Breezhaler DPIs respectively (Weers and Clark, 2017). These results suggest that the flow rate dependence results in variability in TLD that is comparable or lower than the variability associated with oropharyngeal filtering of particles. Not surprisingly, the outlier here is Bricanyl Turbuhaler where the Q index is 62%. Yet this drug showed no impact of flow rate on improvements in FEV1 down to a PIP of 1 kPa, presumably due to being on the flat part of the dose response curve. While pelletized formulations are the poster child for flow rate dependence with passive DPIs, they represent the exception rather than the standard for what is possible today, even with lactose blends.
The low degree of throat deposition for nebulizers relative to passive DPIs may lead to the potential for lower variability in TLD in vivo, and consequently improved outcomes in head-to-head comparisons for reasons that have nothing to do with flow rate dependence as suggested (Mahler et al., 2014; Mahler et al., 2019a). Rather this may be due to differences in variability associated with URT deposition. Or alternatively, it may have to do simply with the fact that different drugs are used in the comparison. Correlation does not necessarily imply causation.
In addition to its impact on interpatient variability in dose delivery, the high URT deposition observed with current marketed DPI formulations can also lead to increases in local adverse events (e.g., candidiasis, hoarseness, throat pain), as well as systemic adverse events for drugs with oral bioavailability. The high variability in TLD shifts the dose response curve to the right, necessitating higher nominal doses to achieve the same therapeutic effect. This is especially important in pediatric patients, where the risk of adverse events leads to decreases in adherence to therapy. Indeed, many of the limitations regarding DPIs, as delineated by Laube et al., are not inherent limitations of DPIs, but rather are shaped by the formulation technologies utilized for delivery of therapeutics for asthma and COPD that deposit most of their dose in the URT, or are inherently flow rate dependent (i.e., pelletized formulations) (Laube et al., 2011).
It is been 20 years since Duddu et al. and DeLong et al. demonstrated that porous particle formulations could deliver 60–70% of the nominal dose into the lungs independent of PIFR, with interpatient variability in TLD of just 12% between pressure drops of 0.2 and 10 kPa (Duddu et al., 2002; DeLong et al., 2005). Today formulations are available that can push the TLD to ∼90%, while optimizing delivery to both the large and small airways (Weers et al., 2019c; Miller et al., 2021). Even though particle engineering solutions exist to dramatically decrease URT deposition and reduce variability associated with oropharyngeal filtering of particles, flow rate dependence and co-formulation effects, all DPI products for the administration of asthma/COPD therapeutics remain lactose blends and pelletized formulations.
When it was discovered that chlorofluorocarbons (CFC) depleted stratospheric ozone, regulators called for a phase-out of CFC propellants. The pharmaceutical industry responded rapidly with the introduction of hydrofluoroalkane (HFA) propellants. The CFC to HFA conversion necessitated a rework of the modern pMDI, including development of new metering valves, new can coatings, dose counters, and new formulation technologies including solution pMDIs and PulmoSphere™ (re-branded Aerosphere®) formulations. One can see the beginnings of a similar transition for new propellants with low global warming potential.
No such regulatory pressure exists for DPIs. The Entrenched Player’s Dilemma is the choice faced by existing businesses in a changing marketplace. According to Tapscott ‘‘The commercial success of their products in the marketplace increases their dependency on them. Making radical changes in the product’s capabilities could cannibalize sales or lead to costly realignments of strategy and business infrastructure. The result is that entrenched players are generally not motivated to develop or deploy disruptive technologies.’’ (Tapscott and Williams, 2006). This in part helps to explain why engineered particles have yet to be commercialized in the asthma/COPD space.
There have been mixed messages regarding the importance of reducing variability in drug delivery for asthma/COPD therapeutics. On the one hand, there are a large number of references that suggest that the youngest and oldest of patients may not be able to use a passive dry powder inhaler because they cannot achieve the optimal PIFR needed for effective drug delivery. On the other hand, it has been argued that the high therapeutic index associated with asthma/COPD therapeutics has enabled products to be formulated at the top of the dose response curve, such that some reduction in TLD is possible without sacrificing efficacy. This argument has been utilized to quash development of new technologies that dramatically reduce variability in drug delivery of these therapeutics.
As the market moves towards generics in the asthma/COPD space, there is no driver for large pharmaceutical companies to make the investment to advance new technologies. Perhaps this is precisely the time for a new player to push the envelope and make improved DPI products, especially if one believes that variability in dose delivery to the lungs negatively impacts outcomes for some patients.
The author confirms being the sole contributor of this work and has approved it for publication.
JW author is employed by cystetic Medicines, Inc.
The reviewer (IG) declared a past co-authorship with one of the author (JW).
All claims expressed in this article are solely those of the authors and do not necessarily represent those of their affiliated organizations, or those of the publisher, the editors and the reviewers. Any product that may be evaluated in this article, or claim that may be made by its manufacturer, is not guaranteed or endorsed by the publisher.
Al-Showair, R. A. M., Tarsin, W. Y., Assi, K. H., Pearson, S. B., and Chrystyn, H. (2007). Can All Patients with COPD Use the Correct Inhalation Flow with All Inhalers and Does Training Help? Respir. Med. 101, 2395–2401. doi:10.1016/j.rmed.2007.06.008
Altman, P., Wehbe, L., Dederichs, J., Guerin, T., Ament, B., Moronta, M. C., et al. (2018). Comparison of Peak Inspiratory Flow Rate via the Breezhaler, Ellipta and HandiHaler Dry Powder Inhalers in Patients with Moderate to Very Severe COPD: a Randomized Cross-Over Trial. BMC Pulm. Med. 18, 100. doi:10.1186/s12890-018-0662-0
Amirav, I., Newhouse, M. T., and Mansour, Y. (2005). Measurement of Peak Inspiratory Flow with In-Check Dial Device to Simulate Low-Resistance (Diskus) and High-Resistance (Turbuhaler) Dry Powder Inhalers in Children with Asthma. Pediatr. Pulmonol. 39, 447–451. doi:10.1002/ppul.20180
Auty, R. M., Brown, K., Neale, M. G., and Snashall, P. D. (1987). Respiratory Tract Deposition of Sodium Cromoglycate Is Highly Dependent upon Technique of Inhalation Using the Spinhaler. Br. J. Dis. Chest 81, 371–380. doi:10.1016/0007-0971(87)90186-0
Azouz, W., Chetcuti, P., Hosker, H. S. R., Saralaya, D., Stephenson, J., and Chrystyn, H. (2015). The Inhalation Characteristics of Patients when They Use Different Dry Powder Inhalers. J. Aerosol Med. Pulm. Drug Deliv. 28, 35–42. doi:10.1089/jamp.2013.1119
Baba, K., Tanaka, H., Nishimura, M., Yokoe, N., Takahashi, D., Yagi, T., et al. (2011). Age-Dependent Deterioration of Peak Inspiratory Flow with Two Kinds of Dry Powder Corticosteroid Inhalers (Diskus® and Turbuhaler®) and Relationships with Asthma Control. J. Aerosol Med. Pulm. Drug Deliv. 24, 293–301. doi:10.1089/jamp.2010.0868
Baloira, A., Abad, A., Fuster, A., García Rivero, J. L., García-Sidro, P., Márquez-Martín, E., et al. (2021). Lung Deposition and Inspiratory Flow Rate in Patients with Chronic Obstructive Pulmonary Disease Using Different Inhalation Devices: a Systematic Literature Review and Expert Opinion. COPD Vol. 16, 1021–1033. doi:10.2147/copd.s297980
Bentur, L., Mansour, Y., Hamzani, Y., Beck, R., Elias, N., and Amirav, I. (2004). Measurement of Inspiratory Flow in Children with Acute Asthma. Pediatr. Pulmonol. 38, 304–307. doi:10.1002/ppul.20109
Borgström, L., Olsson, B., and Thorsson, L. (2006). Degree of Throat Deposition Can Explain the Variability in Lung Deposition of Inhaled Drugs. J. Aerosol Med. 19, 473–483. doi:10.1089/jam.2006.19.473
Brand, P., Meyer, T., Weuthen, T., Timmer, W., Berkel, E., Wallenstein, G., et al. (2013). Lung Deposition of Radiolabeled Tiotropium in Healthy Subjects and Patients with Chronic Obstructive Pulmonary Disease. J. Clin. Pathol. 47, 1335–1341. doi:10.1177/0091270006295788
Broeders, M. E. A. C., Molema, J., Hop, W. C. J., Vermue, N. A., and Folgering, H. T. M. (2004). The Course of Inhalation Profiles during an Exacerbation of Obstructive Lung Disease. Respir. Med. 98, 1173–1179. doi:10.1016/j.rmed.2004.04.010
Brown, P. H., Ning, A. C., Greening, A. P., McLean, A., and Crompton, G. K. (1995). Peak Inspiratory Flow through Turbuhaler in Acute Asthma. Eur. Respir. J. 8, 1940–1941. doi:10.1016/S0954-6111(99)90316-510.1183/09031936.95.08111940
Buttini, F., Brambilla, G., Copelli, D., Sisti, V., Balducci, A. G., Bettini, R., et al. (2016). Effect of Flow Rate on In Vitro Aerodynamic Performance of NEXThaler in Comparison with Diskus and Turbohaler Dry Powder Inhalers. J. Aerosol Med. Pulm. Drug Deliv. 29, 167–178. doi:10.1089/jamp.2015.1220
Byron, P. R., Hindle, M., Lange, C. F., Longest, P. W., McRobbie, D., Oldham, M. J., et al. (2010). In Vivo-In Vitro Correlations: Predicting Pulmonary Drug Deposition from Pharmaceutical Aerosols. J. Aerosol Med. Pulm. Drug Deliv. 23 (Suppl. 2), S–59. doi:10.1089/jamp.2010.0846
Chodosh, S., Flanders, J. S., Kesten, S., Serby, C. W., Hochrainer, D., and Witek, T. J. (2001). Effective Delivery of Particles with the HandiHaler Dry Powder Inhalation System over a Range of Chronic Obstructive Pulmonary Disease Severity. J. Aerosol Med. 14, 309–315. doi:10.1089/089426801316970268
Chrystyn, H. (2007). The DiskusTM: a Review of its Position Among Dry Powder Inhaler Devices. Int. J. Clin. Pract. 61, 1022–1036. doi:10.1111/j.1742-1241.2007.01382.x
Ciciliani, A.-M., Denny, M., Langguth, P., Voshaar, T., and Wachtel, H. (2021). Lung Deposition Using the Respimat Soft Mist Inhaler Mono and Fixed-Dose Combination Therapies: An In Vitro/In Silico Analysis. J. Chronic Obstructive Pulm. Dis. 18, 91–100. doi:10.1080/15412555.2020.1853091
Clark, A. R., Chambers, C. B., Muir, D., Newhouse, M. T., Paboojian, S., and Schuler, C. (2007). The Effect of Biphasic Inhalation Profiles on the Deposition and Clearance of Coarse (6.5 μM) Bolus Aerosols. J. Aerosol Med. 20, 75–82. doi:10.1089/jam.2006.0557
Clark, A. R., and Hollingworth, A. M. (1993). The Relationship between Powder Inhaler Resistance and Peak Inspiratory Conditions in Healthy Volunteers - Implications for In Vitro Testing. J. Aerosol Med. 6, 99–110. doi:10.1089/jam.1993.6.99
Clark, A. R., Weers, J. G., and Dhand, R. (2020). The Confusing World of Dry Powder Inhalers: It Is All about Inspiratory Pressures, Not Inspiratory Flow Rates. J. Aerosol Med. Pulm. Drug Deliv. 33, 1–11. doi:10.1089/jamp.2019.1556
Clark, A. (2015). The Role of Inspiratory Pressures in Determining the Flow Rates Though Dry Powder Inhalers; A Review. Curr. Pharm. Des. 21, 3974–3983. doi:10.2174/1381612821666150820105800
Darquenne, C. (2020). Deposition Mechanisms. J. Aerosol Med. Pulm. Drug Deliv. 33, 181–185. doi:10.1089/jamp.2020.29029.cd
De Koning, J. P., Van der Mark, T. W., Coenegracht, P. M. J., Tromp, T. F. J., and Frijlink, H. W. (2002). Effect of an External Resistance to Airflow on the Inspiratory Flow Curve. Int. J. Pharmaceutics 234, 257–266. doi:10.1016/s0378-5173(01)00969-3
DeLong, M., Wright, J., Dawson, M., Meyer, T., Sommerer, K., and Dunbar, C. (2005). Dose Delivery Characteristics of the AIR Pulmonary Delivery System over a Range of Inspiratory Flow Rates. J. Aerosol Med. 18, 452–459. doi:10.1089/jam.2005.18.452
Delvadia, R. R., Longest, P. W., and Byron, P. R. (2012). In Vitro Tests for Aerosol Deposition. I: Scaling a Physical Model of the Upper Airways to Predict Drug Deposition Variation in Normal Humans. J. Aerosol Med. Pulm. Drug Deliv. 25, 32–40. doi:10.1089/jamp.2011.0905
Dewar, M. H., Jamieson, A., McLean, A., and Crompton, G. K. (1999). Peak Inspiratory Flow through Turbuhaler in Chronic Obstructive Airways Disease. Respir. Med. 93, 342–344. doi:10.1016/s0954-6111(99)90316-5
Dolovich, M. B., Vanzielegham, M., and Hidinger, J. H. (1988). Influence of Inspiratory Flow Rate on the Response of Terbutaline Sulphate Inhaled via the Turbuhaler. Am. Rev. Respir. Dis. 133, A433.
Drblik, S., Lapierre, G., Thivierge, R., Turgeon, J., Gaudreault, P., Cummins-McManus, B., et al. (2003). Comparative Efficacy of Terbutaline Sulphate Delivered by Turbuhaler Dry Powder Inhaler or Pressurised Metered Dose Inhaler with Nebuhaler Spacer in Children during an Acute Asthmatic Episode. Arch. Dis. Child. 88, 319–323. doi:10.1136/adc.88.4.319
Duddu, S. P., Sisk, S. A., Walter, Y. H., Tarara, T. E., Trimble, K. R., Clark, A. R., et al. (2002). Improved Lung Delivery from a Passive Dry Powder Inhaler Using an Engineered PulmoSphere® Powder. Pharm. Res. 19, 689–695. doi:10.1023/a:1015322616613
Engel, T., Heinig, J. H., Madsen, F., and Nikander, K. (1990). Peak Inspiratory Flow and Inspiratory Vital Capacity of Patients with Asthma Measured with and without a New Dry-Powder Inhaler Device (Turbuhaler). Eur. Respir. J. 3, 1037–1041. PMID: 2289551.
Finlay, W., and Martin, A. (2015). Evaluating Inhaler Performance Using Idealized Throats. Eur. Pharm. Rev. 20, 55–57.
Ghosh, S., Ohar, J. A., and Drummond, M. B. (2017). Peak Inspiratory Flow Rate in Chronic Obstructive Pulmonary Disease: Implications for Dry Powder Inhalers. J. Aerosol Med. Pulm. Drug Deliv. 30, 381–387. doi:10.1089/jamp.2017.1416
Ghosh, S., Pleasants, R. A., Ohar, J. A., Donohue, J. F., and Drummond, M. B. (2019). Prevalence and Factors Associated with Suboptimal Peak Inspiratory Flow Rates in COPD. COPD Vol. 14, 585–595. doi:10.2147/COPD.S195438
Haidl, P., Heindl, S., Siemon, K., Bernacka, M., and Cloes, R. M. (2016). Inhalation Device Requirements for Patients' Inhalation Maneuvers. Respir. Med. 118, 65–75. doi:10.1016/j.rmed.2016.07.013
Hamilton, M., Leggett, R., Pang, C., Charles, S., Gillett, B., and Prime, D. (2015). In Vitro Dosing Performance of the ELLIPTA Dry Powder Inhaler Using Asthma and COPD Patient Inhalation Profiles Replicated with the Electronic Lung (eLung). J. Aerosol Med. Pulm. Drug Deliv. 28, 498–506. doi:10.1089/jamp.2015.1225
Haynes, A., Geller, D., Weers, J., Ament, B., Pavkov, R., Malcolmson, R., et al. (2016). Inhalation of Tobramycin Using Simulated Cystic Fibrosis Patient Profiles. Pediatr. Pulmonol. 51, 1159–1167. doi:10.1002/ppul.23451
Hill, M., Vaughan, L., and Dolovich, M. (1996). Dose Targeting for Dry Powder Inhalers. Proc. Respir. Drug Deliv. V. 1, 197–208.
Hillyer, E. V., Price, D. B., Chrystyn, H., Martin, R. J., Israel, E., van Aalderen, W. M. C., et al. (20182018). Harmonizing the Nomenclature for Therapeutic Aerosol Particle Size: A Proposal. J. Aerosol Med. Pulm. Drug Deliv. 31 (2), 111–113. doi:10.1089/jamp.2017.1396
Kamin, W. E. S., Genz, T., Roeder, S., Scheuch, G., Trammer, T., Juenemann, R., et al. (2002). Mass Output and Particle Size Distribution of Glucocorticosteroids Emitted from Different Inhalation Devices Depending on Various Inspiratory Parameters. J. Aerosol Med. 15, 65–73. doi:10.1089/08942680252908593
Laube, B. L., Janssens, H. M., de Jongh, F. H. C., Devadason, S. G., Dhand, R., Diot, P., et al. (2011). What the Pulmonary Specialist Should Know about the New Inhalation Therapies. Eur. Respir. J. 37, 1308–1417. doi:10.1183/09031936.00166410
Loh, C. H., Peters, S. P., Lovings, T. M., and Ohar, J. A. (2017). Suboptimal Inspiratory Flow Rates Are Associated with Chronic Obstructive Pulmonary Disease and All-Cause Readmissions. Ann. ATS 14, 1305–1311. doi:10.1513/AnnalsATS.201611-903OC
Mahler, D. A., and Halpin, D. M. G. (2021). Peak Inspiratory Flow as a Predictive Therapeutic Biomarker in COPD. Chest 160, 491–498. doi:10.1016/j.chest.2021.03.049
Mahler, D. A., Ohar, J. A., Barnes, C. N., Moran, E. J., Pendyala, S., and Crater, G. D. (2019a). Nebulized versus Dry Powder Long-Acting Muscarinic Antagonist Bronchodilators in Patients with COPD and Suboptimal Peak Inspiratory Flow Rate. J. Chron. Obstruct. Lug Dis. 6, 321–331. doi:10.15326/jcopdf.6.4.2019.0137
Mahler, D. A. (2017). Peak Inspiratory Flow Rate as a Criterion for Dry Powder Inhaler Use in Chronic Obstructive Pulmonary Disease. Ann. ATS 14, 1103–1107. doi:10.1513/AnnalsATS.201702-156PS
Mahler, D. A. (2020). The Role of Inspiratory Flow in Selection and Use of Inhaled Therapy for Patients with Chronic Obstructive Pulmonary Disease. Respir. Med. 161, 105857. doi:10.1016/j.rmed.2019.105857
Mahler, D. A., Waterman, L. A., and Gifford, A. H. (2013). Prevalence and COPD Phenotype for a Suboptimal Peak Inspiratory Flow Rate against the Simulated Resistance of the Diskus Dry Powder Inhaler. J. Aerosol Med. Pulm. Drug Deliv. 26, 174–179. doi:10.1089/jamp.2012.0987
Mahler, D. A., Waterman, L. A., Ward, J., and Gifford, A. H. (2014). Comparison of Dry Powder versus Nebulized Beta-Agonist in Patients with COPD Who Have Suboptimal Peak Inspiratory Flow Rate. J. Aerosol Med. Pulm. Drug Deliv. 27, 103–109. doi:10.1089/jamp.2013.1038
Marple, V. A., Olson, B. A., Santhanakrishnan, K., Roberts, D. L., Mitchell, J. P., and Hudson-Curtis, B. L. (2004). Next Generation Pharmaceutical Impactor: a New Impactor for Pharmaceutical Inhaler Testing. Part III. Extension of Archival Calibration to 15 L/min. J. Aerosol Med. 17, 335–343. doi:10.1089/jam.2004.17.335
Marple, V. A., Roberts, D. L., Romay, F. J., Miller, N. C., Truman, K. G., Van Oort, M., et al. (2003). Next Generation Pharmaceutical Impactor (A New Impactor for Pharmaceutical Inhaler Testing). Part I: Design. J. Aerosol Med. 16, 283–299. doi:10.1089/089426803769017659
Martin, A. R., and Finlay, W. H. (2007). A General, Algebraic Equation for Predicting Total Respiratory Tract Deposition of Micrometer-Sized Aerosol Particles in Humans. J. Aerosol Sci. 38, 246–253. doi:10.1016/j.jaerosci.2006.11.002
Miller, D. P., Tarara, T. E., and Weers, J. G. (2021). Targeting of Inhaled Therapeutics to the Small Airways: Nanoleucine Carrier Formulations. Pharmaceutics 13, 1855. doi:10.3390/pharmaceutics13111855
Nagel, M. W., Suggett, J. A., and Mitchell, J. P. (2021). Is a Pressure Drop ≥ 10 cm H2O with Any Dry Powder Inhaler (DPI) a Reasonable Threshold above Which a Patient Should Receive an Adequate Lung Dose: Method Validation and Experience with a Sample Cohort of COPD Patients. Drug Deliv. Lungs 32. (abstract).
Newman, S. P., and Chan, H.-K. (2020). In Vitro-In Vivo Correlations (IVIVCs) of Deposition for Drugs Given by Oral Inhalation. Adv. Drug Deliv. Rev. 167, 135–147. doi:10.1016/j.addr.2020.06.023
Newman, S. P. (2017). Drug Delivery to the Lungs: Challenges and Opportunities. Ther. Deliv. 8, 647–661. doi:10.4155/tde-2017-0037
Newman, S. P., Morén, F., Trofast, E., Talaee, N., and Clarke, S. W. (1991). Terbutaline Sulphate Turbuhaler: Effect of Inhaled Flow Rate on Drug Deposition and Efficacy. Int. J. Pharm. 74, 209–213. doi:10.1016/0378-5173(91)90239-K
Nielsen, K. G., Auk, I. L., Bojsen, K., Ifversen, M., Klug, B., and Bisgaard, H. (1998). Clinical Effect of Diskus Dry-Powder Inhaler at Low and High Inspiratory Flow-Rates in Asthmatic Children. Eur. Respir. J. 11, 350–354. doi:10.1183/09031936.98.11020350
Nielsen, K. G., Skov, M., Klug, B., Ifversen, M., and Bisgaard, H. (1997). Flow-dependent Effect of Formoterol Dry-Powder Inhaled from the Aerolizer™. Eur. Respir. J. 10, 2105–2109. doi:10.1183/09031936.97.10092105
Olsson, B., Borgström, L., Lundbäck, H., and Svensson, M. (2013). Validation of a General In Vitro Approach for Prediction of Total Lung Deposition in Healthy Adults for Pharmaceutical Inhalation Products. J. Aerosol Med. Pulm. Drug Deliv. 26, 355–369. doi:10.1089/jamp.2012.0986
Pavkov, R., Mueller, S., Fiebich, K., Singh, D., Stowasser, F., Pignatelli, G., et al. (2010). Characteristics of a Capsule Based Dry Powder Inhaler for the Delivery of Indacaterol. Curr. Med. Res. Opin. 26, 2527–2533. doi:10.1185/03007995.2010.518916
Pedersen, S., Hansen, O. R., and Fuglsang, G. (1990). Influence of Inspiratory Flow Rate upon the Effect of a Turbuhaler. Arch. Dis. Child. 65, 308–310. doi:10.1136/adc.65.3.308
Persson, G., Olsson, B., and Soliman, S. (1997). The Impact of Inspiratory Effort on Inspiratory Flow through Turbuhaler in Asthmatic Patients. Eur. Respir. J. 10, 681–684. PMID: 9073005.
Ruzycki, C. A., Martin, A. R., and Finlay, W. H. (2019). An Exploration of Factors Affecting In Vitro Deposition of Pharmaceutical Aerosols in the Alberta Idealized Throat. J. Aerosol Med. Pulm. Drug Deliv. 32, 405–417. doi:10.1089/jamp.2019.1531
Ruzycki, C. A., Murphy, B., Nathoo, H., Finlay, W. H., and Martin, A. R. (2020). Combined In Vitro-In Silico Approach to Predict Deposition and Pharmacokinetics of Budesonide Dry Powder Inhalers. Pharm. Res. 37, 209. doi:10.1007/s11095-020-02924-7
Sahay, S., Holy, R., Lyons, S., Parsley, E., Maurer, M., and Weers, J. (2021). Impact of Human Behavior on Inspiratory Flow Profiles in Patients with Pulmonary Arterial Hypertension Using AOS Dry Powder Inhaler Device. Pulm. Circ. 11, 1–9. doi:10.1177/2045894020985345
Sharma, G., Mahler, D. A., Mayorga, V. M., Deering, K. L., Harshaw, Q., and Ganapathy, V. (2017). Prevalence of Low Peak Inspiratory Flow Rate at Discharge in Patients Hospitalized for COPD Exacerbation. J. Copd F 4, 217–224. doi:10.15326/jcopdf.4.3.2017.0183
Smith, I. J., Bell, J., Bowman, N., Everard, M., Stein, S., and Weers, J. G. (2010). Inhaler Devices: what Remains to Be Done? J. Aerosol Med. Pulm. Drug Deliv. 23, S–25. doi:10.1089/jamp.2010.0853
Stahlhofen, W., Rudolf, G., and James, A. C. (1989). Intercomparison of Experimental Regional Aerosol Deposition Data. J. Aerosol Med. 2, 285–308. doi:10.1089/jam.1989.2.285
Stass, H., Nagelschmitz, J., Kappeler, D., Sommerer, K., Patzlaff, A., and Weimann, B. (2019). Ciprofloxacin Dry Powder for Inhalation: Inspiratory Flow in Patients with Non-cystic Fibrosis Bronchiectasis. J. Aerosol Med. Pulm. Drug Deliv. 32, 156–163. doi:10.1089/jamp.2018.1464
Tapscott, D., and Williams, A. D. (2006). Wilkinomics: How Mass Collaboration Changes Everything. New York: The Penguin Group.
Tiddens, H. A., Geller, D. E., Challoner, P., Speirs, R. J., Kesser, K. C., Overbeek, S. E., et al. (2006). Effect of Dry Powder Inhaler Resistance on the Inspiratory Flow Rates and Volumes of Cystic Fibrosis Patients of Six Years and Older. J. Aerosol Med. 19, 456–465. doi:10.1089/jam.2006.19.456
Ung, K. T., and Chan, H.-K. (2016). Effects of Ramp-Up of Inspired Airflow on In Vitro Aerosol Dose Delivery Performance for Certain Dry Powder Inhalers. Eur. J. Pharm. Sci. 84, 46–54. doi:10.1016/j.ijpharm.2016.07.07310.1016/j.ejps.2016.01.005
Ung, K. T., Rao, N., Weers, J. G., Clark, A. R., and Chan, H.-K. (2014). In Vitro Assessment of Dose Delivery Performance of Dry Powders for Inhalation. Aerosol Sci. Technol. 48, 1099–1110. doi:10.1080/02786826.2014.962685
Van der Palen, J. (2003). Peak Inspiratory Flow through Diskus and Turbuhaler, Measured by Means of a Peak Inspiratory Flow Meter (In-Check DIAL). Respir. Med. 97, 285–289. doi:10.1053/rmed.2003.1289
Von Berg, A., Kremer, H.-J., Ellers-Lenz, B., Conrad, F., Erb, K., Maus, J., et al. (2007). Peak Inspiratory Flow Rates Generated through the Novolizer and the Turbuhaler Dry Powder Inhaler Devices by Children with Stable Asthma. J. Aerosol Med. 20, 50–58. doi:10.1089/jam.2006.0558
Weers, J., and Clark, A. (2017). The Impact of Inspiratory Flow Rate on Drug Delivery to the Lungs with Dry Powder Inhalers. Pharm. Res. 34, 507–528. doi:10.1007/s11095-016-2050-x
Weers, J. G., Bell, J., Chan, H.-K., Cipolla, D., Dunbar, C., Hickey, A. J., et al. (2010). Pulmonary Formulations: what Remains to Be Done? J. Aerosol Med. Pulm. Drug Deliv. 23 (Suppl. 2), S–5. doi:10.1089/jamp.2010.0838
Weers, J. G., Clark, A. R., Rao, N., Ung, K., Haynes, A., Khindri, S. K., et al. (2015). In Vitro-In Vivo Correlations Observed with Indacaterol-Based Formulations Delivered with the Breezhaler. J. Aerosol Med. Pulm. Drug Deliv. 28, 268–280. doi:10.1089/jamp.2014.1178
Weers, J. G., Miller, D. P., and Tarara, T. E. (2019a). Spray-dried PulmoSphere Formulations for Inhalation Comprising Crystalline Drug Particles. AAPS PharmSciTech 20, 1–15. doi:10.1208/s12249-018-1280-0
Weers, J., Rao, N., and Kadrichu, N. (2019b). Is Aerodynamic Diameter a Good Metric for Understanding Regional Deposition? Proc. Respir. Drug Deliv. Europe 1, 59–66.
Weers, J. G., Son, Y.-J., Glusker, M., Haynes, A., Huang, D., Kadrichu, N., et al. (2019c). Idealhalers versus Realhalers: Is it Possible to Bypass Deposition in the Upper Respiratory Tract? J. Aerosol Med. Pulm. Drug Deliv. 32, 55–69. doi:10.1089/jamp.2018.1497
Weers, J. (2018). Regional Deposition of Particles within the Respiratory Tract Should Be Linked to Impaction Parameter, Not Aerodynamic Size. J. Aerosol Med. Pulm. Drug Deliv. 31, 116–118. doi:10.1089/jamp.2018.1452
Weers, J., Ung, K., Le, J., Rao, N., Ament, B., Axford, G., et al. (2013). Dose Emission Characteristics of Placebo PulmoSphereParticles Are Unaffected by a Subject's Inhalation Maneuver. J. Aerosol Med. Pulm. Drug Deliv. 26, 56–68. doi:10.1089/jamp.2012.0973
Keywords: peak inspiratory flow rate, peak inspiratory pressure, minimum threshold energy, optimal flow rate, asthma, COPD
Citation: Weers J (2022) Suboptimal Inspiratory Flow Rates With Passive Dry Powder Inhalers: Big Issue or Overstated Problem?. Front. Drug. Deliv. 2:855234. doi: 10.3389/fddev.2022.855234
Received: 14 January 2022; Accepted: 04 March 2022;
Published: 22 March 2022.
Edited by:
Philip Chi Lip Kwok, The University of Sydney, AustraliaReviewed by:
Andrew Martin, University of Alberta, CanadaCopyright © 2022 Weers. This is an open-access article distributed under the terms of the Creative Commons Attribution License (CC BY). The use, distribution or reproduction in other forums is permitted, provided the original author(s) and the copyright owner(s) are credited and that the original publication in this journal is cited, in accordance with accepted academic practice. No use, distribution or reproduction is permitted which does not comply with these terms.
*Correspondence: Jeffry Weers, andlZXJzQGN5c3RldGljbWVkaWNpbmVzLmNvbQ==
Disclaimer: All claims expressed in this article are solely those of the authors and do not necessarily represent those of their affiliated organizations, or those of the publisher, the editors and the reviewers. Any product that may be evaluated in this article or claim that may be made by its manufacturer is not guaranteed or endorsed by the publisher.
Research integrity at Frontiers
Learn more about the work of our research integrity team to safeguard the quality of each article we publish.