- 1Department of Surgical and Developmental Sciences, School of Dentistry, University of Minnesota, Minneapolis, MN, United States
- 2TDA Research Inc., Golden, CO, United States
- 3Minnesota Dental Research Center for Biomaterials and Biomechanics, Department of Restorative Sciences, School of Dentistry, University of Minnesota, Minneapolis, MN, United States
Current resin composites have favorable handling and upon polymerization initial physical properties that allow for efficient material replacement of removed carious tooth structure. Dental resin composites have long-term durability limitations due to the hydrolysis of ester bonds within the methacrylate-based polymer matrix. This article outlines the importance of ester bonds positioned internal to the carbon–carbon double bond in current methacrylate monomers. Water and promiscuous salivary/bacterial esterase activity can initiate ester bond hydrolysis that can sever the polymer backbone throughout the material. Recent studies have custom synthesized, with the latest advances in modern organic chemical synthesis, a novel molecule named ethylene glycol bis (ethyl methacrylate) (EGEMA). EGEMA was designed to retain the reactive acrylate units. Upon intermolecular polymerization of vinyl groups, EGEMA ester groups are positioned outside the backbone of the polymer chain. This review highlights an investigation into the degradation resistance of EGEMA using buffer, esterase, and microbial storage assays. Material samples of EGEMA had superior final physical and mechanical properties than traditional ethylene glycol dimethacrylate (EGDMA) in all degradation assays. Integrating bioinformatics-based biodegradation predictions to the experimental results of storage media analyzed by LC/GC-MS revealed that hydrolysis of EGEMA generated small amounts of ethanol while preserving the strength-bearing polymer backbone. Prior studies support an investigation into additional custom-synthesized methacrylate polymers with “flipped external” ester groups. The long-term goal is to improve clinical durability compared to current methacrylates while retaining the inherent advantages of acrylic-based chemistry, which may ease the implementation of these novel methacrylates into clinical practice.
Introduction
The majority of current resin-based composite restorations use methacrylates as a polymer matrix. Despite clinical improvements compared to older generations, current methacrylate-based materials display less than ideal long-term durability, especially in specific high-risk populations (1–5). For healthy young children with a history of decay in the primary dentition, the 24-month cumulative survival rate of a methacrylate composite restoration is 67%, with no significant differences seen between the numbers of surfaces in the restoration (6). For medically complex (ASA II) children, only 55% of composite restorations survive after 24 months (6). In recent years, pediatric restorative recommendations based on systematic reviews have shifted toward recommending full-coverage crowns, since current survival rates of methacrylate materials translate to primary teeth restorations failing before tooth exfoliation (7). Full-coverage crowns cemented by glass ionomer cement provide a barrier seal that allows for a higher success rate than multi-surface composite restorations (8). Full-coverage crowns can seal carious bacteria over a long-term period which allows a high success rate even in the case of incomplete caries removal (9). Current pediatric restorative guidelines recommend full-coverage crowns rather than composite restorations due to inherent weaknesses in the long-term adhesive seal of methacrylate-based resin composite materials (7).
Laboratory assessments of current methacrylate materials have identified several weaknesses that affect the long-term stability of current composite restorations (10–12). Potential factors that may influence secondary caries at the composite resin tooth interface include an absence of effective buffering of methacrylate materials to acidification (13), low fluoride release of the material (14), stresses from masticatory forces (15), and degradation of the polymer matrix compromising the marginal seal and structural integrity of the restoration-tooth interface (16). While fluoride release and improving buffer capacity can be addressed with the changes to the filler system in methacrylates (17, 18), degradation is directly related to the position of ester bonds in the current methacrylate monomers. Common monomers such as ethylene glycol dimethacrylate (EGDMA), triethylene glycol dimethacrylate (TEGDMA) 1,1,1-trimethylolethane trimethacrylate, 2-hydroxyethyl methacrylate (HEMA), 1,1,1-trimethylolpropane triacrylate, urethane dimethacrylate (UDMA), and bisphenol A glycidyl methacrylate (BisGMA) have internal ester bonds that irreversibly hydrolyze in the presence of saliva and oral bacteria. The hydrolysis of ester bonds severs the polymer matrix backbone structure in current methacrylates and reduces the overall bulk mechanical properties of the material.
An obvious solution to addressing methacrylate ester bond degradation is using a replacement chemistry that shares many of the short-term advantages while improving upon the long-term durability concern. Ether-based polymers have notable potential as being resistant to hydrolytic degradation (19). Vinyl sulfonamide and thiol-based resin polymers show higher toughness and lower water sorption, which can lead to degradation, compared to methacrylate-based resins (20). While these alternative chemistries may have reduced degradation effects through the removal of ester bonds, a comprehensive comparison to current methacrylate-based materials is still in development. In fact, one investigation into the polymerization kinetics of ether-base materials required the use of the diluents EGDMA/TEGDMA, which are highly prone to hydrolysis as hydrophilic monomers (21). Methacrylate-based materials have numerous short-term advantages. Methacrylates can be formulated to have favorable handling, esthetics, smell, few undesired side reactions, fast polymerization, and low-temperature generation (22, 23).
Another potential solution is re-designing the methacrylate polymer linkage system. For current methacrylates, the ester bond hydrolysis that severs the internal polymer backbone leads to a reduction in bulk mechanical and physical properties. A new approach is to design monomers that when they polymerize lack ester, carbonate, acetal, and anhydride bonds within their polymeric backbone sequence.
In this brief review, recent research on repositioning or “flipping” the ester bond to an external position will be summarized. The aim of the review is to highlight the current evidence on the potential benefits of methacrylate monomers with flipped external ester groups. This review will also introduce the flipped methacrylate design as a polymer that has potential use in high-caries-risk populations such as pediatric patients where there is a need to not only improve upon outcomes for resin composite restorations but also to increase durability for preventive sealants.
Novel Methacrylate Design and Initial Properties
EGDMA is used ubiquitously in dental materials. It is a hydrophilic monomer that is used as a diluent and mixed with larger strength-bearing hydrophobic monomers. EGDMA is commercially available and has been the starting point molecule for an investigation into the influence of ester bond positioning. Current methacrylate monomers such as EGDMA can polymerize via a free radical mechanism mediated by a photoinitiator/co-initiators system of camphorquinone (CQ), ethyl 4-dimethylamino benzoate (EDMAB), and diphenyliodonium hexafluorophosphate (DPIHP). CQ/EDMAB/DPIHP combination has been found to be a highly efficient photoinitiator system and is activated by high-intensity light with a wavelength range of 385–515 nm (24). EGDMA can polymerize through intermolecular polymerization of vinyl groups, in the presence of other EGDMA molecules (homo-polymerization) or different methacrylate monomers (co-polymerization) (25). After polymerization, the esters within EGDMA are positioned within or “internal” to polymeric backbone sequence shown as blue boxes (Figure 1A). If hydrolysis occurs at these internal ester bond linkages, the backbone of the polymer chain is severed.
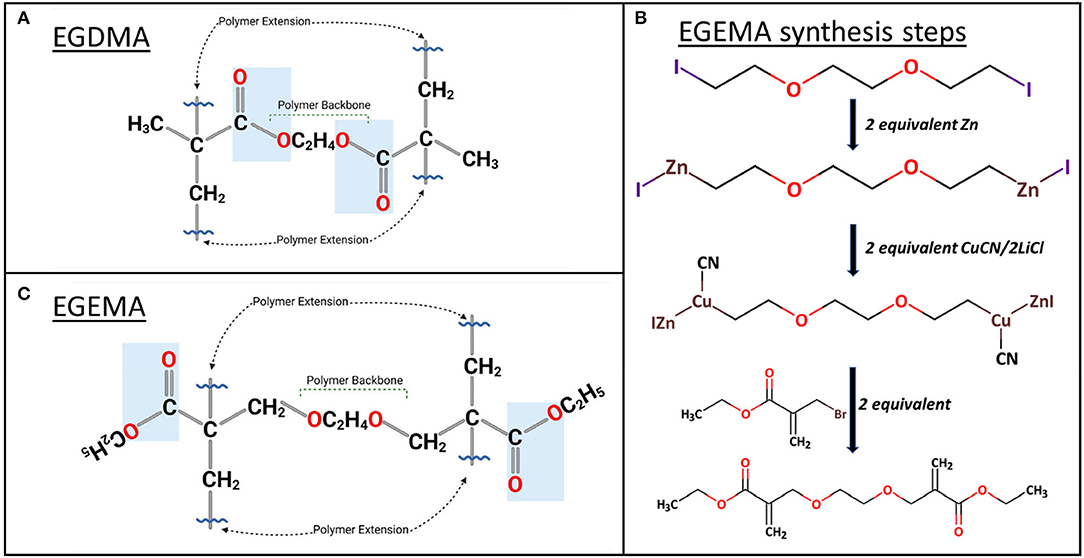
Figure 1. (A) molecular structure of EGDMA homo-polymerization where ester groups are within the polymer backbone. (B) EGEMA synthesis steps. (C) In EGEMA homo-polymerization, ester groups are external to the polymer backbone, flipped along the carbon axis, across the vinyl group.
Recent studies have custom synthesized a novel molecule named ethylene glycol bis (ethyl methacrylate) (EGEMA) with the latest advances in modern organic chemical synthesis (26, 27). Unlike EGDMA, EGEMA, which is a similar molecule with an alteration in the position of the ester bonds, is not commercially available. EGEMA was synthesized in a 1-pot 2-step reaction (Figure 1B) (28). While this may indicate a straightforward synthesis, key steps in the synthesis were unavailable when the first methylacrylate dental monomers were developed (29). Briefly, the synthesis reaction, performed under argon, begins with 1,2-bis(2-iodoethoxy) ethane. This molecule is metallated with zinc and then copper using organometallic transformations (26, 27). The Zn–Cu trans-metallated intermediate is allowed to react in situ with two equivalents of the electrophile ethyl 2-bromomethylacrylate to generate EGEMA (Figure 1C) (28).
The main difference between EGEMA and EGDMA is the position of the ester groups in the monomer (Figures 1C,A). This translated to differences in the ester group's relative position to the polymer backbone upon polymerization. After the polymerization of vinyl groups, EGEMA has ester groups that are “external” to the polymerizing chain shown with blue box in Figure 1C. The external position can also be termed a “flipped” ester design, since EGEMA is analogous to EGDMA with the ester group flipped along the carbon axis, across the vinyl group.
Recent studies demonstrated that EGEMA and EGDMA can be photo-polymerized with similar degrees of conversion after 40-second exposure to 385–515 nm high intensity (1000 mW/cm2) light (28, 30, 31). The two macromers used the same photoinitiator/co-initiators system of CQ/EDMAB/DPIHP. To simplify the assessment of EGEMA and EGDMA, the monomers were cured into 4.9 mm diameter and 2.6 mm thickness discs within a polytetrafluoroethylene (PTFE) mold. The cured discs were initially tested for bulk mechanical properties (Table 1). These assessments indicated that EGDMA had a statistically superior hardness and diametral tensile strength (DTS) to EGEMA under the same photo-curing parameters.
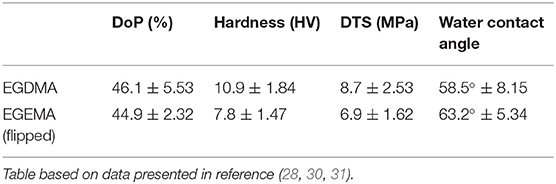
Table 1. Initial comparison between EGDMA (internal ester position) and EGEMA (flipped) examining degree of polymerization (DoP), hardness, diametral tensile strength (DTS), and water contact angle (hydrophilicity/hydrophobicity).
Degradation Assay Comparison
While EGDMA demonstrated initial higher mechanical properties after curing compared to EGEMA, this characterization did not predict the long-term durability trend of the two materials. Degradation assays examined EGDMA and EGEMA under various aqueous storage conditions. Materials were stored in solutions with increasing hydrolytic challenge to accelerate aging and investigate the hypothesis that ester bond positioning was a primary factor in the loss of mechanical strength. In order to interpret EGEMA and EGDMA results from these various degradation assays, it is essential to review the rationale for choosing specific degradation assays used in previous studies.
While there are numerous storage solutions that test dental material degradation potential using artificial aging models, phosphate buffered saline buffer (1xPBS) solutions at a resting saliva pH of 7.4 offer a starting point to understand hydrolysis of ester bonds. Materials can be stored at room temperature or at elevated temperatures. The advantage of elevated temperature storage is that higher temperature can accelerate material aging by increasing the rate of hydrolysis to shorten the study time (32). Material discs can be exposed to elevated temperatures which is based on an American Society for Testing and Materials (ASTM) protocol for the accelerated aging of medical devices and polymers (33). Based on ASTM F1980-16, material storage for 15 weeks at 55°C is equivalent to approximately 1 year at 37°C, which is the temperature within the oral cavity. Therefore, instead of storing material discs at 37°C for 1 year, experiments can store the materials at 55°C for 15 weeks and be expected to yield similar results. While this accelerated aging model is compatible with water or artificial saliva media, accelerated temperature aging in PBS can be compared with a second model of esterase enzymes suspended in 1x PBS (9 weeks).
An esterase storage model explores the direct mechanism of enzyme-mediated scission. There are several esterase enzymes that can be chosen for a model. There is evidence that esterases have different molecular specificity in methacrylate monomers, but the differences have been demonstrated only between molecules that have vast differences in size and hydrophobicity (12, 34). In the case of similar molecules, a Cholesterol esterase (CEase-Carboxyl ester lipase) derived from Pseudomonas sp. (EC 3.1.1.13) at 0.5 units/ml concentration in 1x PBS can be used. This CEase has reproducible esterase activity that is measurable with a colorimetric p-nitrophenol acetate assay (31). The CEase model is more severe for materials that contain ester bonds than the temperature accelerated model that examines the effects of accelerated water hydrolysis of ester bonds. Furthermore, examining the degradation effect size for a CEase and hydrolysis aging model provides direct evidence of the importance of ester bond positioning.
A third model of 9-week exposure to Streptococcus mutans (S. mutans; ATCC 700610/UA159) provides a measure of durability to an acidogenic bacterium. S. mutans can potentially degrade material discs via enzyme and acid formation. S. mutans produces a known esterase with measurable activity (30) and in previous works has shown to degrade conventional methacrylates (35–37). Acidification of S. mutans in Todd Hewitt media, which is supplemented with 0.3% yeast extract and 0.2% glucose, challenges material discs but also decreases the esterase activity substantially (30). In this model, fresh media is replaced daily. Esterases of S. mutans are more active near physiologically neutral pHs (37). This is the rationale for using PBS buffer solutions within the CEase model. CEase activity is mitigated at lower pHs, especially below a pHs of 5 (37). Also, for any bacterial model, there could potentially be unidentified mechanisms of degradation.
In reference to studies examining EGEMA and EGDMA in these three degradation models (hydrolytic, CEase, S. mutans), mechanical and physical properties changes occurred for both materials (Figure 2). However, the effects of degradation were more pronounced for EGDMA. EGDMA has a pronounced reduction in relative weight (Figure 2A) and diametral tensile strength (DTS) (Figure 2C) when stored in the presence of buffer, CEase, and S. mutans. There was a trend of larger reduction in relative weight and DTS with the 55°C accelerated aging, CEase, and S. mutans degradation models compared to 37°C storage. In the most severe model, CEase exposure for 9 weeks, the mean relative weight loss was 15.3% and 8.8% for EGDMA and EGEMA, respectively. The relative weight loss corresponded to higher water sorption (Figure 2D). Surface hydrophilicity (lower water contact angle) increased in all three degradation assays for both EGDMA and EGEMA. Less water uptake was measured for EGEMA than EGDMA discs in all three degradation studies. The significance of these results was that material degradation increased water sorption, which in turn, mediated more ester bond hydrolysis.
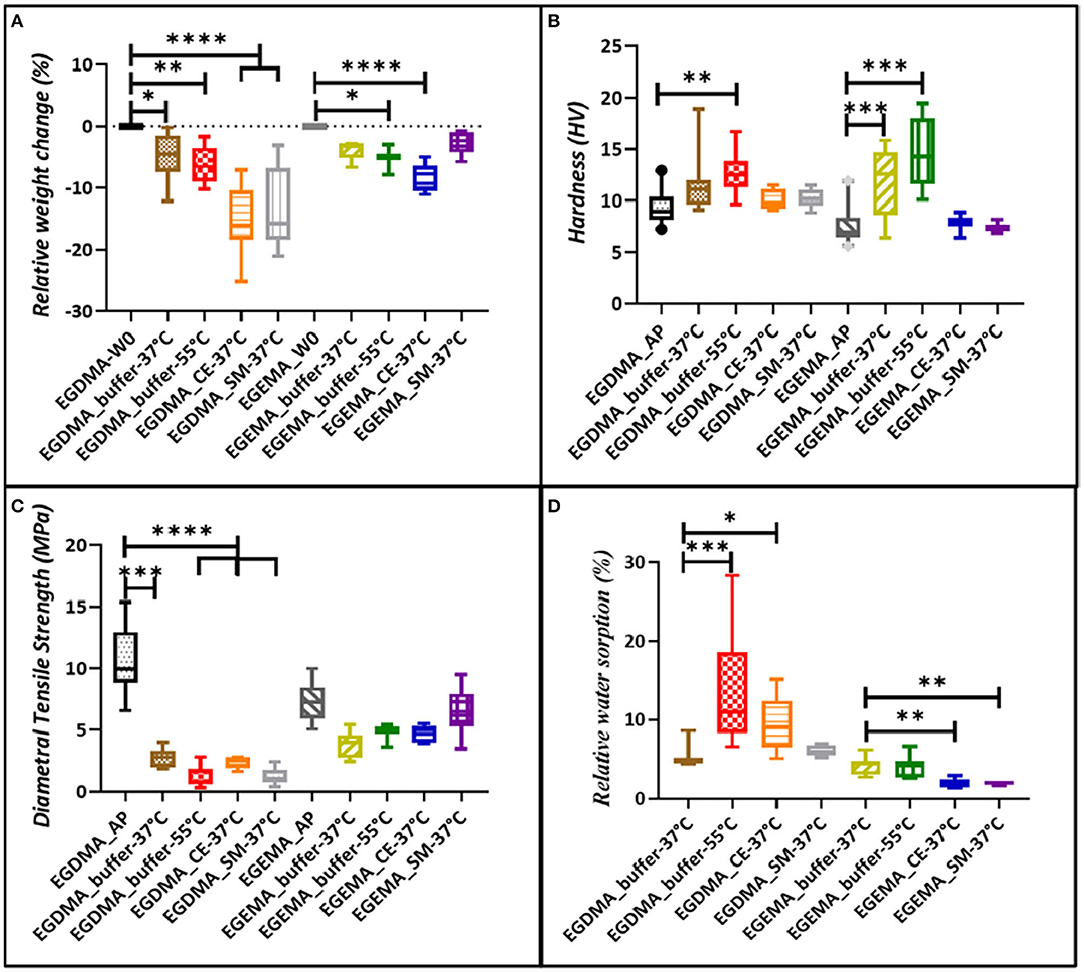
Figure 2. Relative weight change for sample discs incubated in buffer, CEase, and S. mutans cells, (A) followed by change in hardness (B) for same conditions, (C) represents changes in diametral tensile strength, and (D) represents relative water sorption. Graphs based on data presented in reference (28, 30, 31).
EGEMA discs were more resistant to degradation effects. EGEMA had higher load-bearing DTS values after the completion of the three degradation studies than EGDMA. This shows an inversion of the differences between the two materials, since initially EGDMA had a markedly higher DTS than EGEMA.
In the three degradation studies, hardness values were less intuitive to interpret, since there were several factors happening during polymer disc storage. EGEMA and EGDMA both underwent a dark/post cure while in the storage solutions. In these degradation studies, hardness was defined as “selective intact surface hardness” since after aqueous storage hardness measurement needed to be measured on intact smooth surfaces. The testing instrumentation (Micro surface Vickers Hardness Test) was not capable of measuring hardness on irregular surface roughness caused by hydrolysis degradation. Nonetheless, the hardness results were interpreted in the context that there were two competing factors affecting surface hardness outside of the restraint of choosing intact smooth surfaces to measure. One factor was that the surface was getting harder through a dark/post cure, and the other factor was that the storage solutions (buffer, CEase, S. mutans) were creating surface softening though ester bond hydrolysis. It is not surprising that surface properties showed differences between the temperature accelerated aging and CEase/S. mutans assays.
For the temperature aging experiments, EGDMA and EGEMA disc surfaces increased, albeit slightly, in hardness. For both materials, hardness did not increase in the CEase and S. mutans assays. For these later studies, the surface softening was likely more aggressive and competed with the post/dark cure to such a degree that no increase was seen in either material. The collective results indicate that EGEMA had surface softening from the hydrolysis of polymer side chains but degraded to a much lesser extent than EGDMA when examining the bulk properties such as weight and DTS. This is attributed to EGEMA maintaining the intermolecular bonds in the polymeric backbone sequence.
Collectively these results underscored the importance of evaluating the two materials after aging experiments rather than as an initial side by side analysis that could erroneously predict better long-term performance of EGDMA.
Cytocompatibility
The cytocompatibility assays of EGDMA and EGEMA indicate that both polymers have similar effects against oral keratinocytes. The data from metabolic activity assays (Figure 3) suggest both EGDMA and EGEMA support growth to a statistically significant degree more than a commercial dental sealant (HelioSeal) that is composed of a Bis-GMA and EGDMA mixture (38). This observation highlights the higher toxicity of Bis-GMA (39). EGDMA and EGEMA did not support the same level of cell growth and proliferation relative to a glass slide control. However, the glass slide control is a static surface that has limited leaching of unreacted monomers. Longer-term experiments of cytocompatibility are needed, after material aging, to determine how much the leaching of unreacted monomers contributes to the initial cytotoxicity. Prior in vitro studies examining traditional methacrylates have demonstrated that monomers have concentration-dependent cytotoxicity and can leach from polymerized material (39–41). Whether degradation by-products also yield sustained cytotoxic effects at expected low concentrations remains unanswered but as indicated below the degradation pathways of EGEMA and EGDMA during material aging experiments are fundamentally different. As was the case with initial mechanical and physical properties, EGEMA and EGDMA may have different cytocompatibility after aging, but this has not been tested to date. Additional cytotoxicity and biological effect experiments examining EGEMA vs. EGDMA have not been performed.
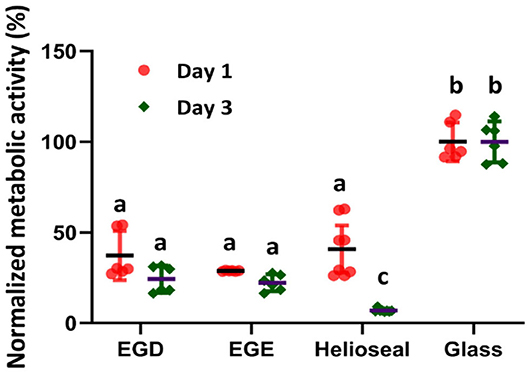
Figure 3. Metabolic activity of oral keratinocyte cells incubated on the EGDMA, EGEMA, HelioSeal (commercially available) discs and glass as control. Graph based on data repository reference (38).
Degradation Pathways
Physical characterization and mechanical property assessment revealed clear differences in terminal weight, DTS, and relative water sorption between EGEMA and EGDMA in the various degradation assays. These measurements supported the hypothesis that polymer backbone preservation occurred in EGEMA polymerization. To test the degradation chemical reactions in EGDMA and EGEMA, the 37°C buffer and CEase assay storage solutions were analyzed for degradation by-products with liquid/gas chromatography (LC/GC)/mass spectroscopy (MS) (31).
Elucidating the unique biodegradation pathways of EGEMA and EGDMA was accomplished by integrating bioinformatics-based biodegradation predictions into the experimental results of storage media analyzed by LC/GC-MS. After samples were exposed for 15 weeks in PBS buffer solution and 9 weeks of CEase, storage solution aliquots were analyzed with chromatographic separation followed by in-line mass spectrometry. The molecular structures of EGDMA and EGEMA were also queried via the EAWAG-Biocatalysis/Biodegradation (BB) Prediction Pathway System (PPS) (42). The bioinformatics PPS created a series of degradation pathway predictions based on rules-based assessment of bond scissions. The corresponding pathways create possible by-product molecules following pathways that are very likely, likely, or neutral. Based on experimental examples, degradation pathways that are considered very likely include biotransformations that occur in any biological system. In the case where the majority of bacteria contain enzymes that degrade a given bond, the definition of likely is indicated. Neutral pathways are common pathways where certain bacteria are implicated in bond degradation. The molar mass of predicted degradation by-products could be compared to LC/GC-MS experimental results. Further details about the methodology of this approach are found elsewhere (31).
LC/MS analysis of EGDMA and EGEMA polymer discs measured 9 and 12 unique degradation by-products respectively. LC/MS analysis of EGEMA included the very likely by-products of 2-hydroxyethyl 2-methylprop-2-enoate (HEMA) and methacrylic acid (Figure 4A). LC/MS analysis of EGEMA included the very likely by-products of 2-{[2-(3-ethoxy-2-methylidene-3-oxopropoxy) ethoxy] methyl} prop-2-enoic acid (Figure 4C).
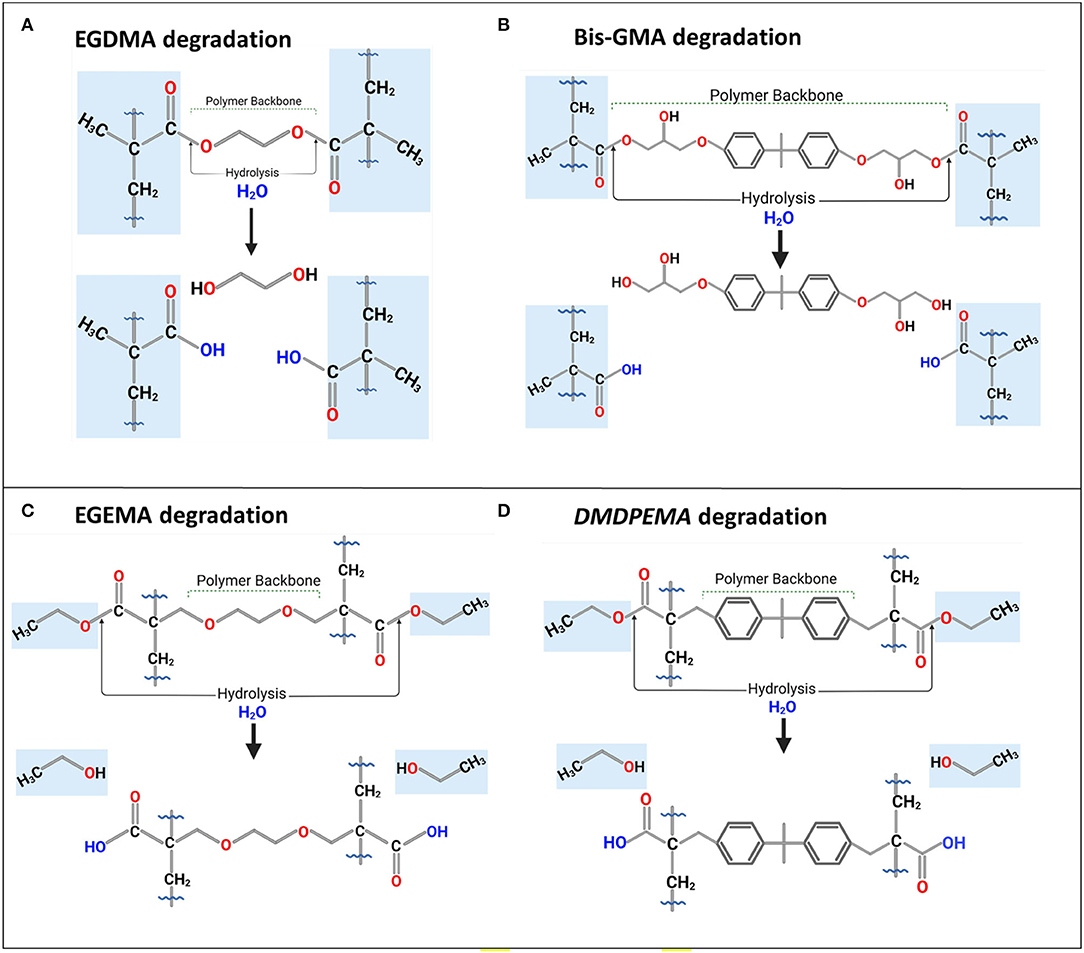
Figure 4. Degradation or bio-degradation of (A) EGDMA and (B) Bis-GMA with their backbone as by-products; their analogs, (C) EGEMA, and (D) DMDPEMA with ethanol as by-product and backbone preserved during hydrolysis. Figures in (A,C) based on figures in (28, 30, 31).
The low molecular weight by-products of ethylene glycol, in the case of the EGDMA pathway, and ethanol, in the case of EGEMA were not identified with LC-MS. It required GC-MS analysis to identify the very likely degradation by-products of ethylene glycol and ethanol that degraded from the two polymer discs. Ethanol was found in both 37°C buffer and CEase assay storage solutions, whereas ethylene glycol was found in greater frequency in the CEase assay which might be explained by the high rate of degradation in that assay compared with 15-week buffer storage.
LC/GC-MS, integrated with a bioinformatics PPS, demonstrated that polymer backbone degradation occurred during the degradation of EGDMA, whereas EGEMA, with the external or flipped position of ester groups to the polymer backbone, had severing of side chains with the polymer backbone intact. The different ester bond positioning changed the chemical degradation pathway, by-products, and durability of the polymer discs.
Future Directions With Novel Methacrylates
Altering the ester-bond linkage retains the benefits of methacrylate chemistry and the ease of commercial implementation while possibly increasing the clinical longevity of resin composite restorations. Near-term investigation into substituting EGEMA for EGDMA is immediately achievable since EGEMA can be polymerized with the same photoinitiator system. Investigating EGEMA vs. EGDMA is a starting point for the synthesis of many different flipped ester design methacrylates based on currently used methacrylates.
In terms of immediate improvement, the diluent EGDMA is quite hydrophilic and is likely a weak link within the polymer mixture of a resin composite system. The hydrophilicity enhances water sorption that can lead to ester bond hydrolysis. Since the synthesis reaction is worked out for EGEMA, future work can examine EGEMA substituting for EGDMA in an EGEMA/Bis-GMA resin mixture. This EGEMA/Bis-GMA mixture can be compared with the EGDMA/Bis-GMA mixture. Future work is needed to investigate subsurface degradation from water and also investigate if CEase penetration into EDGMA/EGEMA discs occurs through microcracks caused by polymerization. The degradation effects of CEase may be reduced when EGDMA and EGEMA are co-polymerized with strength-bearing Bis-GMA or a flipped ester design analog of Bis-GMA.
Dimethyl diphenylmethane ethyl methacrylate (DMDPEMA) is a flipped ester design analog of Bis-GMA that can be potentially blended with EGEMA. To achieve desirable handling properties, the addition of the low-viscosity diluent EGEMA will be necessary since DMDPEMA has a higher viscosity. The viscosity of DMDPEMA was not quantitatively measured yet, but it is qualitatively more viscous than EGEMA, and it is likely in the same order of magnitude as Bis-GMA. Bis-GMA needs to be blended with low-viscosity EGDMA, and future work can compare DMDPEMA/EGEMA with Bis-GMA/EGDMA blends. Future work is needed to investigate DMDPEMA degradation compared to Bis-GMA degradation. Bis-GMA degradation involves breakage of the polymeric backbone yielding a main by-product of bis-hydroxy-propoxy-phenyl propane (Bis-HPPP) and methacrylic acid (Figure 4B) (43). While DMDPEMA is significantly larger than EGEMA, the external ester group can undergo hydrolysis and yield an ethanol by-product just like EGEMA (Figure 4D). DMDPEMA hydrolysis likely preserves the polymeric backbone sequence. This requires further validation with LC/GC-MS. Based on the EGEMA degradation pathway and EAWAG-Biocatalysis/Biodegradation Prediction Pathway System, all flipped ester group designs are expected to generate the same side chain hydrolysis. The main by-product is expected to be ethanol for all the flipped ester group designs no matter how large the molecule (Figure 5). The polymeric backbone is expected to be preserved in DMDPEMA and all other flipped external ester group methacrylate-designed polymers. While long-term immersion in high concentrations (60–75%) of ethanol can cause hydrolysis in conventional methacrylates (32, 44), the low concentration of ethanol generated from EGEMA breakdown suggests minimal chemical degradation effects (31).
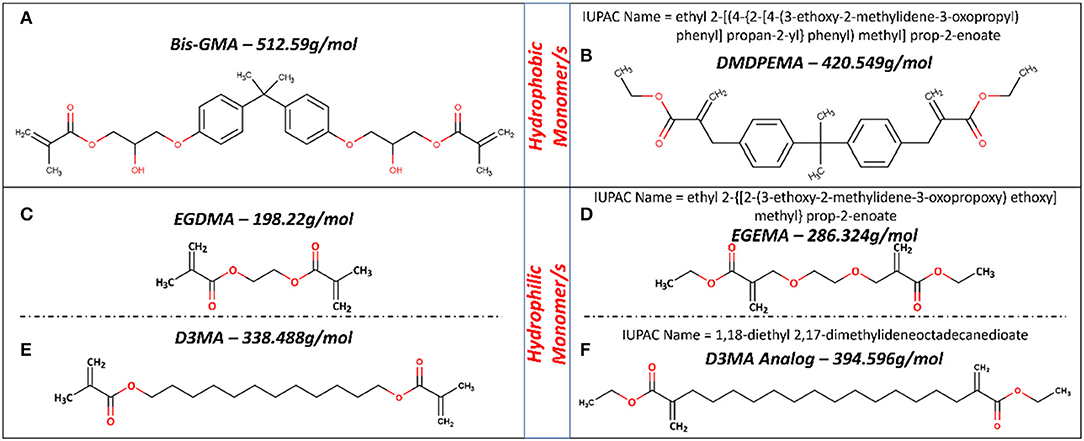
Figure 5. Molecular structure for hydrophobic (A,B) and hydrophilic (C–F) monomers used in dental formulations and their analog with flipped ester group design. Figures in (C,D) based on figures in (28, 30, 31).
The future direction of flipped ester group design polymers first requires investigation into the custom synthesis of these molecules. This will be a lengthy and ongoing area of investigation. After synthesizing and purifying these flipped ester groups, investigation needs to examine how the flipped design may in some cases create steric hindrances. There may be some polymer designs that are more favorable. Future examinations will address adding branch designs that may improve shrinkage, and based on traditional ester linkage methacrylates, branching can be designed to have comparable polymerization as linear monomers (52). Importantly, shrinkage examination will require both initial testing and post-degradation assay assessment since shrinkage stress can change after aqueous conditioning (10, 45).
Current methacrylate-based dental sealants have demonstrated low durability and retention (46–48). Investigating a replacement could be done with as little as two monomers, EGEMA and DMDPEMA. Once the DMDPEMA and EGEMA systems biocompatibilities have been confirmed through additional in vitro cell studies, immunological-based in vivo animal studies should be performed prior to human clinical trials. More work is needed to understand the biological properties and effects of methacrylate polymers with “flipped external” ester groups prior to clinical testing. For initial clinical testing, there may be benefits of optimizing dental sealants in pediatric populations as an initial clinical step. Dental sealants can be simply applied to acid-etched dental enamel. An EGEMA/DMDPEMA blend is expected to be transparent to near-infrared (1310 nm) light, and optical coherence tomography may be utilized to assess initial sealant adaptation and longitudinal assessment of marginal integrity (28, 49).
For dental restorative materials, there are several needed steps that include optimizing the polymer matrix with filler particles and developing novel dentin bonding agents. First, while metalloproteinases (MMPs) can degrade the dentin–collagen hybrid layer, dentin bonding agents contain the same internally positioned ester bonds that can degrade EGDMA and other composites. The degradation effects of dentin adhesive hydrolysis are substantial. Even with MMP inhibitor pretreatment, water storage reduces the microtensile bond strength of current adhesives and increases the marginal leakage by 41% vs. initial properties (50). Cohesive failure in adhesives is also due to water adsorption and hydrolytic degradation (51). Custom synthesis of external flipped ester designs of dentin bonding agents will allow further hypothesis testing on the stability of these polymers to esterases and hydrolytic challenge. Second, these flipped dentin bonding agents may benefit from branched or dendritic designs and may have reduced water adsorption and lower esterase vulnerability than linear flipped polymers due to cross-linked shielding (52). Third, dentin bonding agents with flipped designs are likely compatible with the CQ/EDMAB/DPIHP initiator system, which was found to work in EGEMA polymerization. CQ/EDMAB/DPIHP has been previously determined to be one of the most efficient initiator systems in a moist dentin environment (24). More work is needed to directly test moisture effects with flipped ester bond polymerization. Future experiments can also use MMP inhibitors and many of the aforementioned assays and characterization to investigate how dentin bonding could be optimized. Fourth, the addition of specific filler particles to EGEMA/DMDPEMA may have beneficial effects on improving water adsorption and degradation resistance (53). Fifth, extensive mechanical and physical property testing is needed in future studies examining filled composite systems and their compatible adhesive system. Future tests include measuring the extent of chain length branching, cross-linking density, shrinkage strain, and predicted shrinkage stress (38, 54).
The trajectory of research into flipped external ester group methacrylates can test these materials in high failure risk pediatric and adult dental patients. The traditional methacrylate design was optimized in a dental healthcare system that is currently changing. Clinical outcomes, rather than application time alone, are becoming an increasingly important metric for the dental profession and insurers (55). The cost of replacing failed resin composite systems is substantial and contributes to the increase in overall annual dental expenditures. One of the main benefits of changing the approach to ester-bond linkage design is the ease of commercial implementation while improving the clinical outcome and longevity of composite resin restorations.
Author Contributions
RB, DK, and RJ contributed to the conception and design of the manuscript. RB, IM, DK, and RJ collected articles and organized data for analysis. DK and RJ performed analysis. RJ wrote the first draft of the manuscript. DK, IM, and RB wrote sections of the manuscript. All authors contributed to manuscript revision, read, and approved the submitted version.
Funding
This work was supported by NIH/NIDCR 5R44DE024013-03. Parts of this work were carried out in the Characterization Facility, University of Minnesota, a member of the NSF - Funded Materials Research Facilities Network (www.mrfn.org) via the MRSEC program. The Hitachi SU8320 SEM specimen preparation system was provided by the NSF MRI DMR-1229263 program. The authors acknowledge the University of Minnesota funds received for the open access charge.
Conflict of Interest
RB has employment at TDA Research Inc.
The remaining authors declare that the research was conducted in the absence of any commercial or financial relationships that could be construed as a potential conflict of interest.
Publisher's Note
All claims expressed in this article are solely those of the authors and do not necessarily represent those of their affiliated organizations, or those of the publisher, the editors and the reviewers. Any product that may be evaluated in this article, or claim that may be made by its manufacturer, is not guaranteed or endorsed by the publisher.
Acknowledgments
We would like to thank Young Heo, Hooi Pin Chew, Conrado Aparicio, and Alex Fok for mechanical/physical characterization help and resources. We also acknowledge the open source resources of the EAWAG (http://eawag-bbd.ethz.ch/predict/) within the Federal Institutes of Technology ETH Zurich that utilizes the University of Minnesota biocatalysis/biodegradation database for biodegradation pathway prediction. Images rendering created in BioRender.com.
References
1. Pallesen U, van Dijken JWV, Halken J, Hallonsten A-L, Höigaard R. A prospective 8-year follow-up of posterior resin composite restorations in permanent teeth of children and adolescents in Public Dental Health Service: reasons for replacement. Clin Oral Investig. (2013) 18:819–27. doi: 10.1007/s00784-013-1052-x
2. Opdam NJM, Van De Sande FH, Bronkhorst E, Cenci MS, Bottenberg P, Pallesen U, et al. Longevity of posterior composite restorations: a systematic review and meta-analysis. J Dent Res. (2014) 93:943–9. doi: 10.1177/0022034514544217
3. Demarco FF, Corrêa MB, Cenci MS, Moraes RR, Opdam NJM. Longevity of posterior composite restorations: not only a matter of materials. Dent Mater. (2012) 28:87–101. doi: 10.1016/j.dental.2011.09.003
4. Bücher K, Tautz A, Hickel R, Kühnisch J. Longevity of composite restorations in patients with early childhood caries (ECC). Clin Oral Investig. (2014) 18:775–82. doi: 10.1007/s00784-013-1043-y
5. Kopperud SE, Espelid I, Tveit AB, Skudutyte-Rysstad R. Risk factors for caries development on tooth surfaces adjacent to newly placed class II composites–a pragmatic, practice based study. J Dent. (2015) 43:1323–9. doi: 10.1016/j.jdent.2015.08.013
6. Bayram M, Akgöl BB, Üstün N. Longevity of posterior composite restorations in children suffering from early childhood caries—results from a retrospective study. Clin Oral Investig. (2021) 25:2867–76. doi: 10.1007/s00784-020-03604-x
7. American Academy of Pediatric Dentistry. Pediatric Restorative Dentistry. The Reference Manual of Pediatric Dentistry. Chicago, ILL: American Academy of Pediatric Dentistry. (2021) p. 386–98.
8. Zahdan BA, Szabo A, Gonzalez CD, Okunseri EM, Okunseri CE. Survival rates of stainless steel crowns and multi-surface composite restorations placed by dental students in a pediatric clinic. J Clin Pediatr Dent. (2018) 42:167–72. doi: 10.17796/1053-4628-42.3.1
9. Innes NPT, Evans DJP, Bonifacio CC, Geneser M, Hesse D, Heimer M, et al. The hall technique 10 years on: questions and answers. Br Dent J. (2017) 222:478–83. doi: 10.1038/sj.bdj.2017.273
10. Park JW, Ferracane JL. Water aging reverses residual stresses in hydrophilic dental composites. J Dent Res. (2014) 93:195–200. doi: 10.1177/0022034513513905
11. Garcia-Godoy F, Krämer N, Feilzer AJ, Frankenberger R. Long-term degradation of enamel and dentin bonds: 6-year results in vitro vs. in vivo. Dent Mater. (2010) 26:1113–8. doi: 10.1016/j.dental.2010.07.012
12. Finer Y, Santerre JP. Biodegradation of a dental composite by esterases: dependence on enzyme concentration and specificity. J Biomater Sci Polym Ed. (2003) 14:837–49. doi: 10.1163/156856203768366558
13. Nedeljkovic I, De Munck J, Slomka V, Van Meerbeek B, Teughels W, Van Landuyt KL. Lack of buffering by composites promotes shift to more cariogenic bacteria. J Dent Res. (2016) 95:875–81. doi: 10.1177/0022034516647677
14. Vermeersch G, Leloup G, Vreven J. Fluoride release from glass-ionomer cements, compomers and resin composites. J Oral Rehabil. (2001) 28:26–32. doi: 10.1046/j.1365-2842.2001.00635.x
15. Carrera CA Li Y, Chen R, Aparicio C, Fok A, Rudney J. Interfacial degradation of adhesive composite restorations mediated by oral biofilms and mechanical challenge in an extracted tooth model of secondary caries. J Dent. (2017) 66:62–70. doi: 10.1016/j.jdent.2017.08.009
16. Huang B, Cvitkovitch DG, Santerre JP, Finer Y. Biodegradation of resin–dentin interfaces is dependent on the restorative material, mode of adhesion, esterase or MMP inhibition. Dent Mater. (2018) 34:1253–62. doi: 10.1016/j.dental.2018.05.008
17. Wei Su L, Lin DJ, Yen Uan J. Novel dental resin composites containing LiAl-F layered double hydroxide (LDH) filler: Fluoride release/recharge, mechanical properties, color change, and cytotoxicity. Dent Mater. (2019) 35:663–72. doi: 10.1016/j.dental.2019.02.002
18. Jang JH, Lee MG, Ferracane JL, Davis H, Bae HE, Choi D, et al. Effect of bioactive glass-containing resin composite on dentin remineralization. J Dent. (2018) 75:58–64. doi: 10.1016/j.jdent.2018.05.017
19. Gonzalez-Bonet A, Kaufman G, Yang Y, Wong C, Jackson A, Huyang G, et al. Preparation of dental resins resistant to enzymatic and hydrolytic degradation in oral environments. Biomacromolecules. (2015) 16:3381–8. doi: 10.1021/acs.biomac.5b01069
20. Sinha J, Dobson A, Bankhar O, Podgórski M, Shah PK, Zajdowicz SLW, et al. Vinyl sulfonamide based thermosetting composites via thiol-Michael polymerization. Dent Mater. (2020) 36:249–56. doi: 10.1016/j.dental.2019.11.012
21. Dickens SH, Stansbury JW, Choi KM, Floyd CJE. Photopolymerization Kinetics of Methacrylate Dental Resins. Macromolecules. (2003) 36:6043–53. doi: 10.1021/ma021675k
22. Ilie N, Hickel R. Resin composite restorative materials. Aust Dent J. (2011) 56:59–66. doi: 10.1111/j.1834-7819.2010.01296.x
23. Pratap B, Gupta RK, Bhardwaj B, Nag M. Resin based restorative dental materials: characteristics and future perspectives. Jpn Dent Sci Rev. (2019) 55:126–38. doi: 10.1016/j.jdsr.2019.09.004
24. Park J, Ye Q, Topp EM, Misra A, Kieweg SL, Spencer P. Effect of photoinitiator system and water content on dynamic mechanical properties of a light-cured bisGMA/HEMA dental resin. J Biomed Mater Res A. (2010) 93:1245. doi: 10.1002/jbm.a.32617
25. Hayakawa T, Takahashi K, Kikutake K, Yokota I, Nemoto K. Analysis of polymerization behavior of dental dimethacrylate monomers by differential scanning calorimetry. J Oral Sci. (1999) 41:9–13. doi: 10.2334/josnusd.41.9
26. Majid TN, Knochel P. A new preparation of highly functionaized aromatic and heteroaromatic zinc and copper organometallics. Tetrahedron Lett. (1990) 31:4413–6. doi: 10.1016/S0040-4039(00)97635-4
27. AchyuthaRao S, Tucker CE, Knochel P. Preparation of functionalized zinc and copper organometallics containing sulfur functionalities at the alpha or gamma position. Tetrahedron Lett. (1990) 31:7575–8. doi: 10.1016/S0040-4039(00)97302-7
28. Kumar D, Bolskar RD, Malone S, Mutreja I, Aparicio C, Jones RS, et al. Novel dental polymer with a flipped external ester group design that resists degradation via polymer backbone preservation. ACS Biomater Sci Eng. (2020) 6:5609–19. doi: 10.1021/acsbiomaterials.0c00947
29. Bayne SC. Beginnings of the dental composite revolution. J Am Dent Assoc. (2013) 144:880–4. doi: 10.14219/jada.archive.2013.0205
30. Kumar D, Ghose D, Bolskar RD, Mutreja I, Jones RS. A novel methacrylate derivative polymer that resists bacterial cell-mediated biodegradation. J Biomed Mater Res - Part B Appl Biomater. (2021) 110:991–1000. doi: 10.1002/jbm.b.34972
31. Kumar D, Ghose D, Mutreja I, Bolskar RD, Aparicio C, Jones RS. Utilizing a degradation prediction pathway system to understand how a novel methacrylate derivative polymer with flipped external ester groups retains physico-mechanical properties following esterase exposure. Dent Mater. (2022) 38:251–65. doi: 10.1016/j.dental.2021.12.008
32. Gornig DC, Maletz R, Ottl P, Warkentin M. Influence of artificial aging: mechanical and physicochemical properties of dental composites under static and dynamic compression. Clin Oral Investig. (2022) 26:1491–504. doi: 10.1007/s00784-021-04122-0
33. Devices SM. Standard Guide for Accelerated Aging of Sterile Barrier Systems for Medical. West Conshohoken, PA, USA: ASTM Int. (2012).
34. Finer Y, Jaffer F, Santerre JP. Mutual influence of cholesterol esterase and pseudocholinesterase on the biodegradation of dental composites. Biomaterials. (2004) 25:1787–93. doi: 10.1016/j.biomaterials.2003.08.029
35. Mushashe AM, de Almeida SA, Ferracane JL, Merritt J, Correr GM. Effect of biofilm exposure on marginal integrity of composite restoration. Am J Dent. (2020) 33:201.
36. Huang B, Sadeghinejad L, Adebayo OIA, Ma D, Xiao Y, Siqueira WL, et al. Gene expression and protein synthesis of esterase from Streptococcus mutans are affected by biodegradation by-product from methacrylate resin composites and adhesives. Acta Biomater. (2018) 81:158–68. doi: 10.1016/j.actbio.2018.09.050
37. Huang B, Siqueira WL, Cvitkovitch DG, Finer Y. Esterase from a cariogenic bacterium hydrolyzes dental resins. Acta Biomater. (2018) 71:330–8. doi: 10.1016/j.actbio.2018.02.020
38. Kumar D, Bolskar RD, Mutreja I, Jones RS. Investigating the cytocompatibility of a novel flipped ester group design polymer composites. Data Repository for U of M (DRUM). (2022). Available online at: https://hdl.handle.net/11299/227293
39. Kraus D, Wolfgarten M, Enkling N, Helfgen EH, Frentzen M, Probstmeier R, et al. In-vitro cytocompatibility of dental resin monomers on osteoblast-like cells. J Dent. (2017) 65:76–82. doi: 10.1016/j.jdent.2017.07.008
40. Geurtsen W. Biocompatibility of resin-modified filling materials. Crit Rev Oral Biol Med. (2000) 11:333–55. doi: 10.1177/10454411000110030401
41. Cândea Ciurea A, Surlin P, Stratul SI, Soancă A, Roman A, Moldovan M, et al. Evaluation of the biocompatibility of resin composite-based dental materials with gingival mesenchymal stromal cells. Microsc Res Tech. (2019) 82:1768–78. doi: 10.1002/jemt.23343
42. Gao J, Ellis LBM, Wackett LP. The university of minnesota biocatalysis/biodegradation database: improving public access. Nucleic Acids Res. (2010) 38:D488–91. doi: 10.1093/nar/gkp771
43. Santerte JP, Shajii L, Tsang H. Biodegradation of commercial dental composites by cholesterol esterase. J Dent Res. (1999) 78:1459–68. doi: 10.1177/00220345990780081201
44. McKinney JE, Wu W. Chemical softening and wear of dental composites. J Dent Res. (1985) 64:1326–31. doi: 10.1177/00220345850640111601
45. Bociong K, Szczesio A, Sokolowski K, Domarecka M, Sokolowski J, Krasowski M, et al. The influence of water sorption of dental light-cured composites on shrinkage stress. Materials. (2017) 10:1142. doi: 10.3390/ma10101142
46. Haznedaroglu E, Güner S, Duman C, Menteş A. A 48-month randomized controlled trial of caries prevention effect of a one-time application of glass ionomer sealant versus resin sealant. Dent Mater J. (2016) 35:532–8. doi: 10.4012/dmj.2016-084
47. Yun X, Li W, Ling C, Fok A. Effect of artificial aging on the bond durability of fissure sealants. J Adhes Dent. (2013) 15:251–8. doi: 10.3290/j.jad.a29014
48. Reić T, Galić T, Negovetić Vranić D. Retention and caries-preventive effect of four different sealants - A 2-year prospective split-mouth study. Int J Paediatr Dent. (2021). doi: 10.1111/ipd.12924. [Epub ahead of print].
49. Lenton P, Rudney J, Fok A, Jones RS. Clinical cross-polarization optical coherence tomography assessment of subsurface enamel below dental resin composite restorations. J Med Imaging. (2014) 1:16001. doi: 10.1117/1.JMI.1.1.016001
50. Shu C, Zheng X, Wang Y, Xu Y, Zhang D, Deng S. Captopril inhibits matrix metalloproteinase activity and improves dentin bonding durability. Clin Oral Investig. (2022) 26:3213–25. doi: 10.1007/s00784-021-04303-x
51. Oskoe SK, Drummond JL, Rockne KJ. The effect of esterase enzyme on aging dental composites. J Biomed Mater Res Part B Appl Biomater. (2019) 107:2178–84. doi: 10.1002/jbm.b.34313
52. Park JG, Ye Q, Topp EM, Spencer P. Enzyme-catalyzed hydrolysis of dentin adhesives containing a new urethane-based trimethacrylate monomer. J Biomed Mater Res B Appl Biomater. (2009) 91:562. doi: 10.1002/jbm.b.31430
53. Øysæd H, Ruyter IE. Water sorption and filler characteristics of composites for use in posterior teeth. J Dent Res. (1986) 65:1315–8. doi: 10.1177/00220345860650110601
54. Soh MS, Yap AUJ. Influence of curing modes on crosslink density in polymer structures. J Dent. (2004) 32:321–6. doi: 10.1016/j.jdent.2004.01.012
Keywords: dental, dental materials, physical properties, degradation, novel polymer, durability, methacrylate monomers, flipped ester group
Citation: Kumar D, Bolskar RD, Mutreja I and Jones RS (2022) Methacrylate Polymers With “Flipped External” Ester Groups: A Review. Front. Dent. Med. 3:923780. doi: 10.3389/fdmed.2022.923780
Received: 19 April 2022; Accepted: 20 May 2022;
Published: 10 June 2022.
Edited by:
James K. H. Tsoi, The University of Hong Kong, Hong Kong SAR, ChinaReviewed by:
Piyaphong Panpisut, Thammasat University, ThailandChristie Lung Ying Kei, The University of Hong Kong, Hong Kong SAR, China
Mohammed Hadis, University of Birmingham, United Kingdom
Copyright © 2022 Kumar, Bolskar, Mutreja and Jones. This is an open-access article distributed under the terms of the Creative Commons Attribution License (CC BY). The use, distribution or reproduction in other forums is permitted, provided the original author(s) and the copyright owner(s) are credited and that the original publication in this journal is cited, in accordance with accepted academic practice. No use, distribution or reproduction is permitted which does not comply with these terms.
*Correspondence: Robert S. Jones, cnNqb25lc0B1bW4uZWR1