- 1Department of Dentistry, Faculty of Medicine and Dentistry, University of Alberta, Edmonton, AB, Canada
- 2Faculty of Dentistry, Alexandria University, Alexandria, Egypt
The majority of dental, periodontal, and craniofacial tissues are derived from the neural crest cells and ectoderm. Neural crest stem cells are pluripotent, capable of differentiating into a variety of cells. These cells can include osteoblasts, odontoblasts, cementoblasts, chondroblasts, and fibroblasts, which are responsible for forming some of the tissues of the oral and craniofacial complex. The hard tissue forming cells deposit a matrix composed of collagen and non-collagenous proteins (NCPs) that later undergoes mineralization. The NCPs play a role in the mineralization of collagen. One such category of NCPs is the small integrin-binding ligand, the N-linked glycoprotein (SIBLING) family of proteins. This family is composed of dentin sialophosphosprotein (DSPP), osteopontin (OPN), dentin matrix protein 1 (DMP1), bone sialoprotein (BSP), and matrix extracellular phosphoglycoprotein (MEPE). The SIBLING family is known to have regulatory effects in the mineralization process of collagen fibers and the maturation of hydroxyapatite crystals. It is well established that SIBLING proteins have critical roles in tooth development. Recent literature has described the expression and role of SIBLING proteins in other areas of the oral and craniofacial complex as well. The objective of the present literature review is to summarize and discuss the different roles the SIBLING proteins play in the development of dental, periodontal, and craniofacial tissues.
Introduction
Hard Tissues and NCPs
Most of the hard tissues of the dental–periodontal complex and craniofacial structure develop from neural crest cells and ectoderm (1). Teeth are composed of enamel, dentin, and pulp. Teeth are supported by the periodontium, which is formed by alveolar bone, cementum, periodontal ligament (PDL), and gingiva (2). The dental-periodontal structure contains all forms of hard tissues found in the body: bone, cementum, dentin, and enamel. Such hard tissues are composed of organic and inorganic matter. The inorganic being mostly hydroxyapatite (HA) crystals, and the organic being composed of collagenous and non-collagenous proteins (NCPs), which are essential in making the framework for the inorganic matter to mineralize (3, 4).
NCPs found in the organic matter of hard tissues are known to be regulators of the mineralization of collagen fibers and of crystal growth. NCPs play an important role in the mineralization process of enamel, dentin, cementum, and bone during development. The SIBLING (small integrin-binding ligand N-glycosylated) family is one category of NCPs with notable expression in these tissues (5, 6).
SIBLING Proteins
The SIBLING family includes dentin matrix protein 1 (DMP1), osteopontin (OPN), bone sialoprotein (BSP), dentin sialophosphoprotein (DSPP), and matrix extracellular phosphoglycoprotein (MEPE) (6). These proteins are all located on chromosome 4 in humans and the family is known to have a regulatory function in mineralization and interactions with HA (5). Another common feature ofthe SIBLING family members is post-translational modifications (PTM), a process in which enzymatic modifications of the proteins occur following synthesis (5, 7). All SIBLINGs also contain an Arg-Gly-Asp (RGD) integrin-binding motif in their structure which promotes cell attachment, differentiation, and migration, as well as intracellular signaling by binding to cell surface receptors (8). It is currently accepted that the SIBLINGs pathway of action includes activation of matrix metalloproteinases (MMPs) that mediate extracellular matrix (ECM) processes (9).
DSPP is the most abundant NCP in the dentin ECM. DSPP is inactive in its full form, it undergoes PTM to cleave into two proteins: dentin phosphoprotein (DPP) (10) and dentin sialoprotein (DSP) (11). DSPP products are known to be essential during the development of dentin, and recent studies have described their role in different craniofacial tissues (12–18).
DMP1 is a product of chondrocytes and osteocytes. It is not usually found in its intact form but rather in one of its cleaved products: an N-terminal peptide, a C-terminal peptide, and a glycosylated N-terminal protein. The intact protein and its glycosylated forms appear to inhibit mineralization. However, the phosphorylated cleaved fragments can promote mineralization (19). Studies have described the expression and role of DMP1 in oral and craniofacial tissues (20–22).
OPN is the most abundant and widely distributed SIBLING protein. It acts as an inhibitor of mineralization, and when it is highly phosphorylated, it can promote HA formation. OPN is also involved in the recruitment of osteoclasts and in regulating the immune response (23). OPN plays an important role in regulating the mineralization of the hard tissues of the craniofacial complex (24, 25).
BSP is a HA nucleator, facilitating osteoblast differentiation and maturation, therefore, stimulating mineralization (26). BSP is mostly expressed in bone-forming cells, with lower expression levels in other mineralized tissues such as dentin (27, 28). BSP promotes cellular adhesion and interactions between cells and matrixes, acting in bone formation and remodeling (29).
MEPE expression has been identified in the dental pulp and in predentin, and it is considered to be involved in the mineralization process (30, 31). MEPE is expressed at a very early stage and only expressed during embryonic development and postnatally on days 5–9 in predentin and day 2 in osteoblasts (32, 33). Much remains unclear regarding MEPE pathways of action.
Extensive research has been published regarding the expression and roles of SIBLING proteins in the oral and craniofacial complex. However, much knowledge about their mechanisms of action is still to be discovered. The objective of the present review is to summarize the current knowledge of SIBLING protein's role in oral and craniofacial development.
Sibling Roles in Dental, Periodontal, and Craniofacial Development
Most of the periodontium develops from the dental follicle that is derived from the neural crest cells. During tooth development, odontoblasts and dental pulp are originated from the dental papilla. Meanwhile, the periodontium components, cementum, periodontal ligament, and alveolar bone, are formed from the dental follicle. The development potential of the dental follicle has been investigated in studies that transplanted the tooth bud to sites known to be incapable of forming mineralized tissue. For example, after tooth bud transplantation to the anterior chamber of the eye, root formation with cementum and alveolar bone formation were observed (34, 35). Even though the dental follicle proper has all precursors needed for cementum, bone, and periodontal ligament formation, fibroblasts from the perifollicular mesenchyme proliferate during root development and contribute to the PDL fibroblast pool. Perifollicular mesenchyme and perivascular cells may also contribute to originating osteoblasts of the alveolar bone (36).
Tooth
There are four stages of tooth development: dental lamina, bud stage, cap stage, and bell stage (37, 38). Dental hard tissue formation starts at the early bell stage, and the most prominent cells that govern this process are odontoblasts and ameloblasts (37, 38). Odontoblasts are responsible for dentin production and are originated from ectomesenchymal cells adjacent to the dental epithelium. Ameloblasts are responsible for enamel production and are originated from inner enamel epithelium cells (39).
Predentin and osteoid are precursors of their corresponding mineralized tissues, dentin, and bone. In their unmineralized phase they lie between the mineralizing front and their depositing cells and are transformed in their mineralized phase as HA crystals are deposited. The biomineralization process involves multiple interactions between different molecules, among those, are type I collagen and NCPs (40, 41). Some of the roles of SIBLING proteins in dental tissues are discussed next.
DSP and DPP were initially thought to be dentin specific, “but recently their expression has also been shown to be present in bone and cementum” (15, 16). Human studies “have shown” that DSPP gene mutations are related to dentinogenesis imperfecta (DGI) and dentin dysplasia (DD); dentin development disorders that result in dentin hypomineralization and consequently, early tooth loss (12, 13). Mice studies also confirmed that Dspp knockout (KO) mice have hypomineralized dentin “that resembles” human DGI. The knockout phenotype presents teeth that are weaker than normal and more susceptible to rapid wear and breakage, with enlarged pulp chambers and widened predentin zone (42, 43). At 1 month of age, a “shell tooth” appearance of the teeth can be noticed, with enlarged pulp chambers. At 3 and 6 months of age extensive destruction of the tooth crown can be noticed, mostly due to the fragility of the dentin (42, 43). Examples of this tooth phenotype are illustrated in Figures 1 and 2.
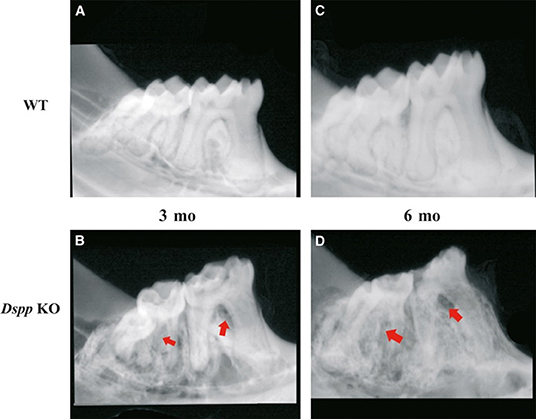
Figure 1. X-ray images of mice mandibles from 3- and 6-month old WT (A,C) and Dspp KO (B,D). Figures 3B,D show reduced dentin layer thickness, with enlargement of the pulp and destruction of the periodontium (red arrows) and crown. Figure from Gibson et al. (58).
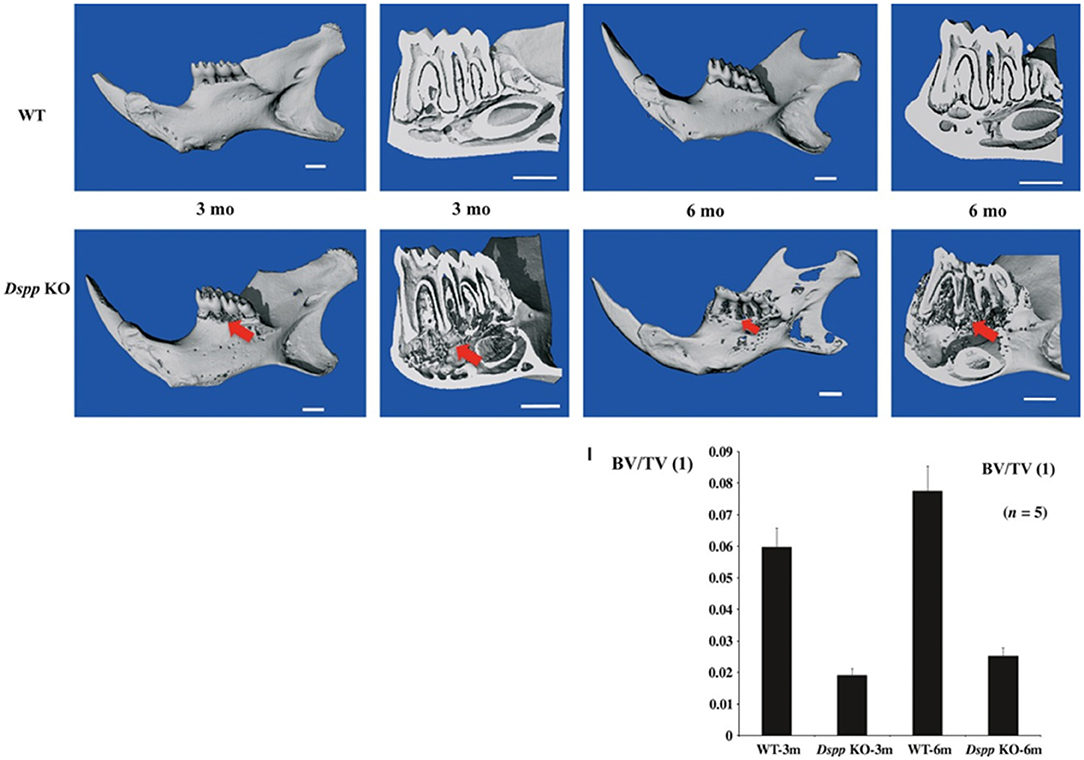
Figure 2. Micro-CT images of mice mandibles from 3- and 6-month-old WT, Dspp KO. Dspp KO images show destruction of the alveolar bone (red arrows). Bone volume fraction (BV/TV) quantitative analysis shows the impact of the loss of function of DSPP in the alveolar bone. Figure from Gibson et al. (58).
DMP1 was also initially identified in teeth and later in bone (44, 45). DMP1 is present mostly in the peritubular region of dentin and the pulp, and it plays a role in dentin mineralization and the differentiation of odontoblasts (46). Previous in vivo studies have shown that Dmp1 KO animals express hypomineralization of the dentin with widened predentin and reduced dentin layer, caused by an impairment of the conversion of predentin to dentin. Also, the dentin tubules in Dmp1 KO models lack organization and number of branches compared to control models of the same age (47, 48). A previous study also suggested that DMP1 can regulate crystal size and organize crystal alignment in the dentin (20). Examples of Dmp1 KO tooth phenotypes are illustrated in Figures 3–5.
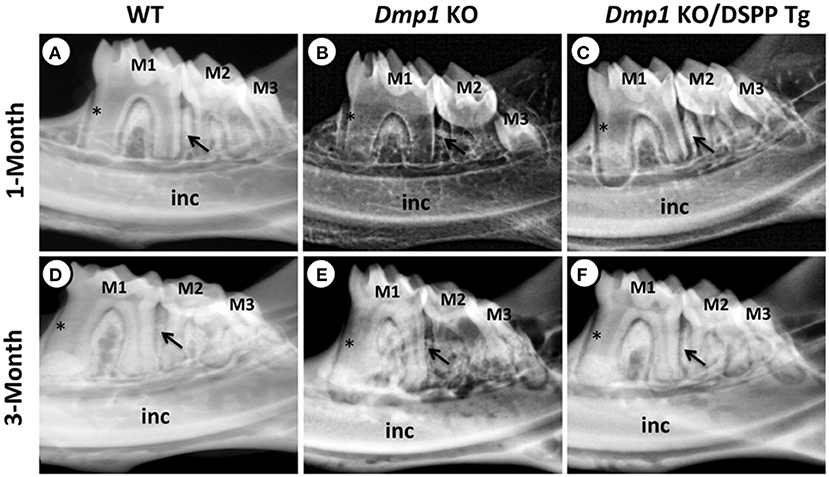
Figure 3. (A–F) X-ray images of mandibles from 1- and 3-month old WT (A,D), Dmp1 KO (B,E), and Dmp1 KO mice with transgenic expression of DSPP (Dmp1 KO/DSPP Tg) (C,F). (A,D) Normal dentin layer thickness and alveolar bone opacity. (B,E) Increased radiolucency of the molars and alveolar bone, with highest impact in 1 month of age. (B) “Shell tooth” appearance of the M1. (E) Evidence of periodontal destruction (black arrow). (C,F) Show similar morphology compared to (A,B). Overall, WT and Dmp1 KO/DSPP Tg express a thicker dentin layer compared to Dmp1 KO (*). M1, First molar; M2, Second molar; M3, Third molar; Inc, Incisors. Figure from Gibson et al. (91).
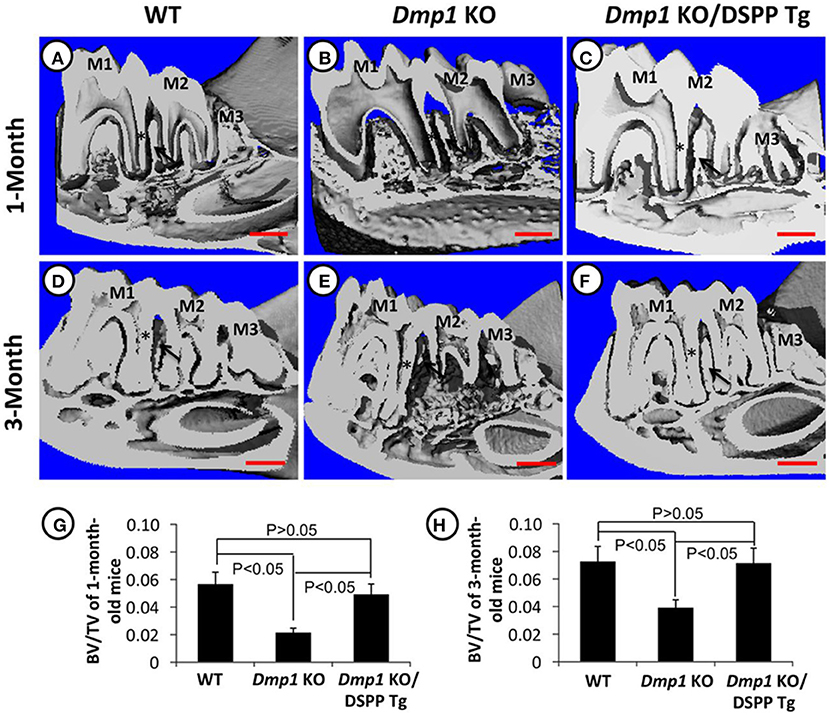
Figure 4. (A–H) Micro-CT images of mandibles from 1- and 3-month old WT (A,D), Dmp1 KO (B,E), and Dmp1 KO mice with transgenic expression of DSPP (Dmp1 KO/DSPP Tg) (C,F). Destruction of the periodontium and enlargement of the pulp can be noticed in (B,E) (black arrows) BV/TV analyses show the impact of Dmp1 KO, as well as the rescue potential of DSPP in Dmp1 KO background (G,H). As seen in X-rays, WT and Dmp1 KO/DSPP Tg express a thicker dentin layer compared to Dmp1 KO (*). M1, First molar; M2, Second molar; M3, Third molar. Figure from Gibson et al. (91).
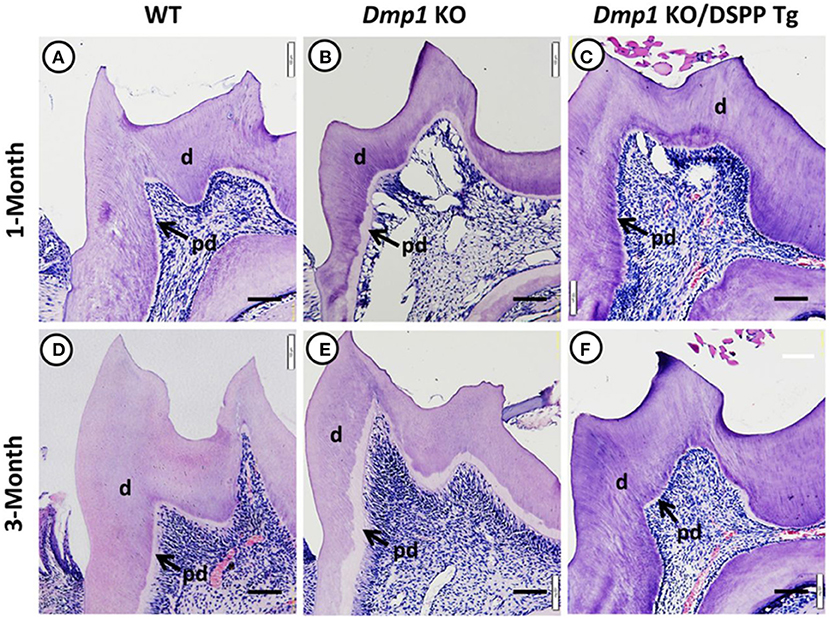
Figure 5. Hematoxylin and eosin staining images of molars from 1- and 3-month old WT (A,D), Dmp1 KO (B,E), and Dmp1 KO mice with transgenic expression of DSPP (Dmp1 KO/DSPP Tg) (C,F). (B,E) Widened predentin (pd) zones and enlarged pulp chambers with reduced dentin (d) layer thickness. Figure from Gibson et al. (91).
OPN is a phosphorylated sialoprotein. It is expressed in predentin, mantle dentin, dentin-cementum junction, and tertiary dentin (25). It is an element of the ECM of teeth. It functions as an inhibitor of mineralization and mediator of interfacial adhesion (31, 49–51). Proper OPN function is closely related to the expression of the Alpl gene, which is responsible for the function of tissue-non-specific alkaline phosphatase (TNAP). TNAP dephosphorylates OPN, therefore the loss of function of TNAP leads to increased OPN concentration in the ECM. Molars and incisors of Alpn KO mice showed hypomineralization of root dentin, with delayed conversion of predentin into dentin, as mentioned previously. The same models also expressed lack of organization of the odontoblast layer of the pulp (49).
BSP is present in reactionary dentin and in the mineralization front (52). BSP is reportedly specific to mineralizing tissues, such as bone, dentin, cementum, and ameloblasts (52, 53). BSP expression is highest in zones where bone is being formed or remodeled (54). However, it has been found that BSP absence did not cause observational defects in dentin mineralization, development, or cellular organization, and therefore, BSP has no critical impact in dentinogenesis, and its absence could possibly be compensated by other SIBLINGs (55). Recent discoveries by Vijaykumar et al. showed that BSP-GFPtpz+ cells are present in in vitro mineralization of pulp cells and in reparative dentin formation (56).
MEPE has been suggested to possibly play roles in dentinogenesis, osteogenesis, and pulpal homeostasis (57). In dentin, MEPE might be able to delay mineralization through negative regulation of odontoblasts during their immature stage, and during the formation of dentinal tubules. It also has been suggested that MEPE might inhibit the growth of HA crystals in the matrix of dentin (57). Another study by Gullard et al. showed that. Mepe−/− mice molars had thicker predentin, dentin, and enamel and lower expression of SIBLING transcripts compared to WT, and also suggested that MEPE might play a role in the maintenance of non-mineralized matrix (58).
Gingiva
Gingiva is composed of gingival epithelium and the gingival connective tissue, both of different tissue origins. The gingival epithelium originates from the thickened reduced enamel epithelium which is formed as an erupting tooth approaches the oral epithelium. As tooth eruption proceeds, the reduced enamel epithelium fuses with the oral epithelium and forms the junctional epithelial cells (36).
Fibroblasts from the gingival connective tissue are developed from the perifollicular mesenchyme, which is a derivative of the stomodeal mesoderm. Unlike periodontal ligament fibroblasts, gingival fibroblasts do not meet the tooth surface. New gingival fibroblasts are originated from the proliferation of undifferentiated perivascular cells (36).
OPN is not normally detected in healthy mucosa (59). However, it is upregulated in the presence of malignant lesions such as oral squamous cell carcinoma, or premalignant lesions, such as leukoplakia (60). DSPP absence has also been related to detachment of junctional epithelium in mice, leading to periodontal disease. However, this is accompanied by bone destruction and the gingival impacts might be secondary to that (61). To the best of the authors' knowledge, limited research is available regarding the role of SIBLING proteins in gingival tissues. Future research would be needed to further understand impacts of their absence in these tissues.
PDL
The development of the PDL is related to tooth root formation. Mesenchymal cells of the perifollicular mesenchyme initialize the synthesis and deposit of collagen fibers and glycoproteins in the developing PDL (62–64). A unique feature of the PDL is that both developing and mature PDL contain undifferentiated stem cells (65–67). OPN, BSP, and DMP1 play a major role in PDL development.
OPN is responsible for preventing ectopic calcification in soft tissues (68–72). In accordance with that, Ibsp−/− mice showed increased density in the PDL compared to control. In the absence of OPN, a five-fold increase in inorganic pyrophosphate (PPi) was reported, possibly working to repress cementum growth, compensating the absence of OPN (73).
The periodontal ligament contains periodontal ligament stem cells (PDLSCs) that are responsible for maintaining tissue homeostasis (74). George et al. investigated the mechanisms by which PDLSCs differentiate into osteoblasts. This study demonstrated that DMP1 is transported to the nucleus of PDLSCs where it promotes osteogenic differentiation (75). Another study also pointed out that the PDL of Dmp1 KO mice “expresses fewer” and irregularly shaped fibroblasts compared to WT (76).
The loss of BSP function has been shown to impact the PDL attachment, affecting Sharpey's fiber attachment and causing disarray of the PDL collagen fibers (55). However, this study pointed out that the integrity of the fibers in knockout models was similar to wild type (WT) at 14 days post-natal (dpn), and disorganization of the fibers could be noticed at 26 dpn, when bone and cementum destruction caused by the absence of BSP had already taken place. Therefore, it must be noted that the PDL impacts might be secondary to destruction of the PDL attachment interfaces.
A study by Gibson et al. showed how the loss of DSPP leads to periodontal disease in mice, including bacterial infiltration in the PDL, which is related to detachment of the PDL. However, it was noted the PDL impacts could be secondary to cementum and alveolar bone loss (61). The histology of this PDL phenotype is illustrated in Figure 6.
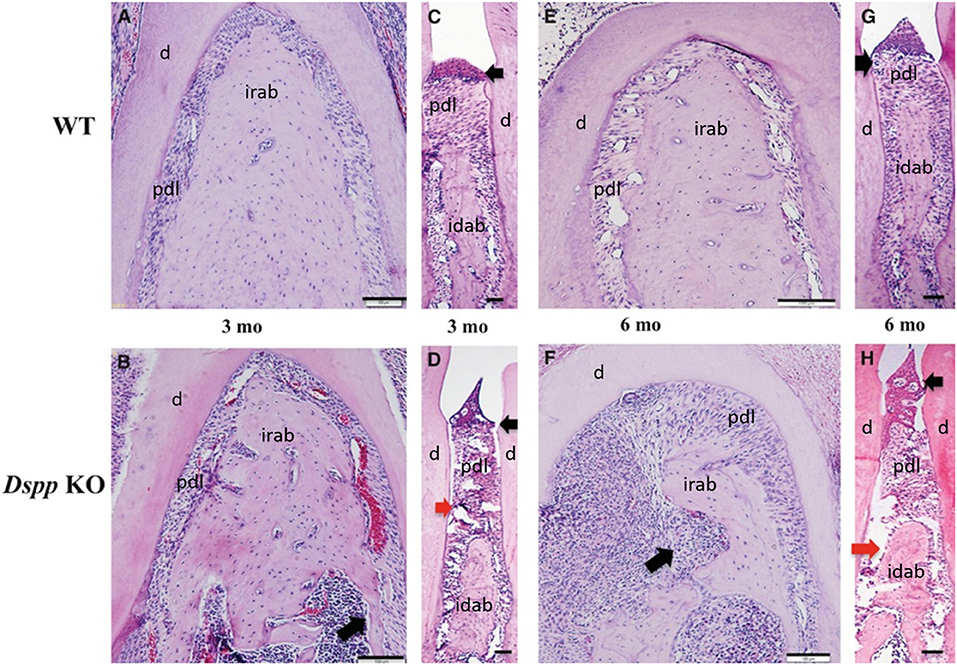
Figure 6. Hematoxylin and eosin staining images of alveolar bone from 3- and 6-month old WT (A,C,E,G), Dspp KO (B,D,F,H). (B,D,F,H) Destruction of the alveolar bone, with apical migration of the junctional epithelium (black arrows) and destruction of the PDL (red arrows) in (D,H), and inflammatory process in the alveolar bone (long black arrows) in (F). Idab, Interdental alveolar bone; irab, inter-radicular alveolar bone; d, dentin; pdl, periodontal ligament. Figure adapted from Gibson et al. (58).
Alveolar Bone
At the late bell stage of tooth development, bony septa, and bony bridge start to form and separate tooth germs from one another, initiating a socket for each tooth (77). As the root develops, the alveolar process follows the increase in height. While cells in the dental follicle start differentiating into fibroblasts and cementoblasts, some cells from the dental follicle differentiate into osteoblasts that form the alveolar bone proper (77–80). Therefore, the morphology of each individual root determines the structure of the alveolar bone proper. The spongy bone and cortical walls of the alveolar process compose the periosteal bone (77).
Successful tooth eruption requires remodeling of the alveolar bone process, where the gubernacular canal must be opened by osteoclastic activity to allow the developing tooth to erupt (81, 82). The dental follicle has been found to be essential for bone resorption through the eruption pathway as well as new bone formation apically to the erupting tooth (83, 84).
OPN is known to be expressed in the periodontium during tooth development (23). In a study, at 14 days post-natal, the Spp1 gene was observed in osteoblasts of the alveolar bone and new cementoblasts near the apical root. “It was concluded that lack of OPN in mice promotes more rapid formation of cellular cementum with higher mineral density compared to the control.” Observations in the mandibular bone showed that osteoblasts appear normal in number and location, suggesting that OPN acts directly in bone matrix mineralization. OPN also functions as an osteoclast recruiter for bone remodeling during unloading conditions in the tail suspension mouse model (85–87). Spp1−/− osteoclasts present defects such as hypomobility and decreased resorption (88, 89). In the alveolar bone, OPN was shown to be important in force-induced bone resorption, such as orthodontic movement (90).
A previous study has observed alveolar bone loss in Dspp−/− mice, with a significant decrease in bone volume/ trabecular volume (BV/TV), compared to control (91). DSPP absence has also been associated with severe alveolar bone loss and bone porosities in mice, as well as infiltration of inflammatory cells in the alveolar bone (61). The histology of this alveolar bone phenotype is illustrated in Figure 6.
The alveolar bone forms by intramembranous ossification, a previous study has described that BSP absence in mice is related to high osteoid concentration resulting in delayed mineralization in the mandible and alveolar bone when compared to WT (55). It has also been reported that Ibsp−/− mice models presented twice the number of osteoclasts in their alveolar bone compared to WT models at 26 dpn, and 8-fold the amount by 60 dpn (55). This study suggested that BSP impacts the osteoid mineralization phase directly during intramembranous ossification, and therefore has a direct role during HA crystal development and maturation.
Literature has already described that DMP1 is important for the conversion of osteoblasts into mature osteocytes (92, 93). DMP1 absence was also found to severely impact the formation of alveolar bone, forming porosities in the bone and disorganization of osteocyte lacunae and reduced canaliculi. A previous study investigated the pathway of action of DMP1 in the bone by investigating its impact on odontoblast differentiation markers, COL1A1 and BSP, and found that Dmp1 KO mice had reduced expression of “these markers and led” to periodontal defects in mice. It is also worth noting that this study found that transgenic DSPP expression was capable of rescuing the osteoblast and odontoblast differentiation impairments in Dmp1 KO mice, as illustrated in Figures 3–5 (94). Dmp1 KO also results in increased FGF23 expression, which is associated with hypophosphatemia in mice and autosomal recessive hypophosphatemic rickets in humans (OMIM 241520) (95, 96).
MEPE expression has also been identified in osteocytes and osteoblasts (30, 31). Previous studies showed that the knockout of Mepe gene in mice was related to increased bone formation and bone mass, as well as higher mineralization and increased osteoblastic markers. MEPE's exact role in mineralization is still unclear, studies have suggested that different terminals of Mepe gene have different functions. A fragment from the COOH- terminal has been linked to inhibition of mineralization in vitro, while, a fragment from the N-terminal has been associated with accelerated mineralization (30, 31).
Cementum
Cementum composition is similar to bone, being 50% inorganic, mostly HA crystals, and the remaining is composed of collagen and NCPs (74). Cementum can be divided into two main forms. Acellular cementum, which serves as an attachment base for the tooth, and cellular cementum, which serves as an active responder to tooth movement and damage. The complete pathway of cementum development is still currently being researched (74). BSP, DMP1, DSP, and OPN are known to be present in cementum.
As mentioned before, acellular cementum is essential for anchoring the PDL fibers to the tooth root surface. The first developmental disease known to affect cementum was hypophosphatasia (HPP; OMIM 241500, 241510, 146300), which causes loss of function of the ALPL gene, which encodes tissue-nonspecific alkaline phosphatase (TNAP) (97, 98). This results in increased concentrations of PPi, which is a potent inhibitor of mineralization. This leads to skeletal defects and loss of acellular cementum formation and function, and therefore further leads to PDL detachment and tooth loss (99–105).
The ECM of cementum is rich in BSP and OPN. Developmental studies of Ibsp−/− mice “have shown that this mutation causes a reduction in the formation” and function of acellular cementum, resulting in dysfunctional PDL attachment (55, 106, 107). Similarity in defective cementum phenotypes between Ibsp−/− and Alpl−/− was investigated. It was concluded that Ibsp KO mice feature a 2-fold increase in circulating PPi, elevated OPN expression, and altered mRNA expression of PPi regulators ALPL, SPP1, and ANK in the periodontium. In vitro studies also showed that Ibsp KO cementoblasts exhibit significantly decreased mineralization capacity and increased PPi (108). The loss of BSP function has also been related to absence of acellular cementum in mice (55).
DSPP has been found to have an active role in cementogenesis. DSPP absence has been related to severe cementum loss in first molars and incisors of 3 month-old mice. This study also suggested that the cementum malformation might be related to postnatal function loss of cementoblasts (61). Similar to DSPP, DMP1 absence has also been found to be related to defective cementum formation of 3 month-old mouse models. This study described the acellular cementum of Dmp1 KO mice to be thinner and with less cementoblasts, which were irregularly shaped. The cementocyte lacuna was rough, and the cementum formation rate was irregular in KO models, which suggested impaired calcospherite maturation (76).
Craniofacial Skeleton
Most of the cranium is formed via intramembranous ossification. In this type of ossification, bone development occurs directly from mesenchymal connective tissue (109). Similar to the alveolar bone, osteoid is transformed into bone through the action of NCPs, especially SIBLING proteins.
A previous study found that osteoblasts derived from the skull of mice missing the DSPP protein deposited less mineral content compared to osteoblasts from WT mice, a phenotype of Dspp KO skull is illustrated in Figure 7 (90).
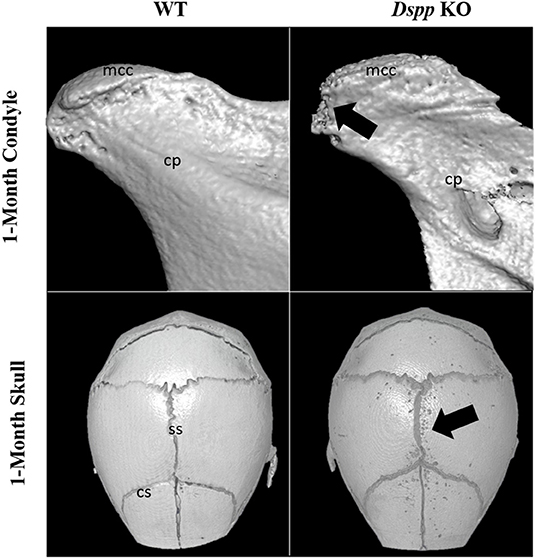
Figure 7. Micro-CT images of mandibular condyles and skulls of 1-month old WT and Dspp KO mice. The KO mice show porosities on the MCC surface, as well as around cranial sutures (black arrows). Mcc, mandibular condylar cartilage; cp, condylar process; ss, sagittal suture; cs, coronal suture.
BSP potentially plays a role in maintaining tissue homeostasis by remodeling via osteoclastogenic activity (110). BSP has also been suggested to play a critical role during the intramembranous ossification of the calvaria, with delayed mineralization represented by wider sutures in Ibsp-/- mice compared to WT during development (55).
A recent study found that the absence of the proteoglycan form of DMP1 (DMP1-PG) could potentially cause premature, narrow, and irregular fusion of cranial sutures. The study suggested that in the absence of DMP1-PG, the differentiation of bone mesenchymal stem cells into preosteoblasts was accelerated, leading to premature mineral deposition (111).
It has been previously shown that the loss of OPN results in impaired osteoclast activity, which leads to impaired bone resorption (88). A more recent publication has outlined that mechanical stress in the sagittal cranial suture of neonatal mice leads to modified expression of OPN, suggesting that OPN is active in the osteoblast differentiation process in cranial sutures (112). Another study has suggested that BSP and OPN have a functional overlap, as they have the potential to compensate for each other's absence in response to a hormonal challenge (113).
Mandibular Condyle
Literature has described the expression of SIBLING proteins in the mandibular condylar cartilage (MCC) and suggested they are active during chondrogenesis (6). IHC results showed DSPP, DMP1, BSP, and OPN expression in different layers of the MCC during development. DSPP absence has been shown to impact the development of the condyle at early ages (6).
DMP1 cleavage has been shown to be essential during chondrogenesis of the MCC. A study has shown that the absence of DMP1-PG could be related to accelerated osteoarthritis in the TMJ cartilage (22). Similarly, a recent study has shown that the post translation modification of DSPP is also essential for the development of the MCC. The same study found that Dspp−/− mice showed a reduced volume of subchondral bone in the condyle compared to WT, an example of this phenotype is illustrated in Figure 7 (114).
Conclusion
Some SIBLING proteins were first discovered in isolated tissues, for example, DSPP and DMP1 in dentin. However, recent studies have brought to light a much wider distribution of the expression of SIBLING proteins in the body. Such distributed expression “suggests that there may be” be many undiscovered roles and pathways of SIBLING protein action. The present review focused on summarizing the expression and roles of SIBLING proteins in oral and craniofacial tissues. Studies included in this review mostly discussed the regulatory function that SIBLING proteins have in mineralization and the impacts of their absences. DMP1, DSPP, and BSP as positive regulators of mineralization, and OPN and MEPE as negative regulators. Some studies have also suggested that SIBLING proteins might be able to partially compensate for the absence of one another.
Further research on the role of SIBLING proteins during periodontal and craniofacial development, as well as which proteins are able to compensate for others absences, could expand our knowledge of the pathways of mineralization and provide potential insight into pathways of rescuing craniofacial defects.
Author Contributions
CF, NA, and MG: study conception and design and draft manuscript. CF: data collection. All authors reviewed the results and approved the final version of the manuscript.
Conflict of Interest
The authors declare that the research was conducted in the absence of any commercial or financial relationships that could be construed as a potential conflict of interest.
Publisher's Note
All claims expressed in this article are solely those of the authors and do not necessarily represent those of their affiliated organizations, or those of the publisher, the editors and the reviewers. Any product that may be evaluated in this article, or claim that may be made by its manufacturer, is not guaranteed or endorsed by the publisher.
References
1. Nanci A. General Embryology. In: Nanci A, editor. Ten Cate's Oral Histology. (Eighth Edition). St. Louis, MO: Mosby. (2013). p. 14–25. doi: 10.1016/B978-0-323-07846-7.00002-1
2. Hughes FJ. Periodontium and periodontal disease. In: Vishwakarma A, Sharpe P, Shi S, Ramalingam M, editors. Stem Cell Biology and Tissue Engineering in Dental Sciences. Amsterdam, Netherlands: Elsevier Inc. (2015) p. 433–4.
3. Boskey AL. Biomineralization: an overview. Connect Tissue Res. (2003) 44 Suppl 1:5–9. doi: 10.1080/03008200390152007
4. George A, Veis A. Phosphorylated proteins and control over apatite nucleation, crystal growth, and inhibition. Chem Rev. (2008) 108:4670–93. doi: 10.1021/cr0782729
5. Huang B, Sun Y, Maciejewska I, Qin D, Peng T, McIntyre B, et al. Distribution of SIBLING proteins in the organic and inorganic phases of rat dentin and bone. Eur J Oral Sci. (2008) 116:104–12. doi: 10.1111/j.160722.2008.00522.x
6. Fisher LW, Fedarko NS. Six genes expressed in bones and teeth encode the current members of the SIBLING family of proteins. Connect Tissue Res. (2003) 1:33–40. doi: 10.1080/03008200390152061
7. Sun Y, Gandhi V, Prasad M, Yu W, Wang X, Zhu Q, et al. Distribution of small integrin-binding ligand, N-linked glycoproteins. (SIBLING) in the condylar cartilage of rat mandible. Int J Oral Maxillofac Surg. (2010) 39:272–81. doi: 10.1016/j.ijom.2009.12.017
8. Fisher LW, Torchia DA, Fohr B, Young MF, Fedarko NS. Flexible structures of SIBLING proteins, bone sialoprotein, and osteopontin. Biochem Biophys Res Commun. (2001) 280:460–5. doi: 10.1006/bbrc.2000.4146
9. Fedarko NS, Jain A, Karadag A, Fisher LW. Three small integrin binding ligand N-linked glycoproteins. (SIBLINGs) bind and activate specific matrix metalloproteinases. FASEB J. (2004) 18:734–6. doi: 10.1096/fj.03-0966fje
10. Veis A, Perry A. The phosphoprotein of the dentin matrix. Biochemistry. (1967) 6:2409–2416. doi: 10.1021/bi00860a017
11. Linde A, Bhown M, Butler WT. Non-collagenous proteins of rat dentin. Evidence that phosphoprotein is not covalently bound to collagen. Biochim Biophys Acta Protein Structure. (1981) 667:341–50. doi: 10.1016/0005-2795(81)90200-2
12. Xiao S, Yu C, Chou X, Yuan W, Wang Y, Bu L, et al. Dentinogenesis imperfecta 1 with or without progressive hearing loss is associated with distinct mutations in DSPP. Nat Genet. (2001) 27:201–4. doi: 10.1038/84848
13. Zhang X, Zhao J, Li C, Gao S, Qiu C, Liu P, et al. DSPP mutation in dentinogenesis imperfecta Shields type II. Nat Genet. (2001) 27:151–2. doi: 10.1038/84765
14. Yamakoshi Y. Dentinogenesis and dentin sialophosphoprotein. (DSPP). J Oral Biosci. (2009) 51:134–42. doi: 10.1016/s1349-0079(09)80021-2
15. Qin C, Brunn JC, Cadena E, Ridall A, Tsujigiwa H, Nagatsuka H, et al. The expression of dentin sialophosphoprotein gene in bone. J Dent Res. (2002) 81:392–4. doi: 10.1177/154405910208100607
16. Baba O, Qin C, Brunn JC, Jones JE, Wygant JE, McItyre BW, et al. Detection of dentin sialoprotein in rat periodontium. Eur J Oral Sci. (2004) 112:163–70. doi: 10.1111/j.0909-8836.2004.00110.x
17. Prasad M, Zhu Q, Sun Y, Wang X, Kulkarni A, Boskey A, et al. Expression of dentin sialophosphoprotein in non-mineralized tissues. J Histochem Cytochem. (2011) 59:1009–21. doi: 10.1369/0022155411423406
18. Zhang R, Chen FM, Zhao SL, Xiao Mz, Smith AJ, Feng JQ. Expression of dentine sialophosphoprotein in mouse nasal cartilage. Arch Oral Biol. (2012) 57:607–13. doi: 10.1016/j.archoralbio.2011.10.012
19. He G, Gajjeraman S, Schultz D, Cookson D, Qin C, Butler WT, et al. Spatially and temporally controlled biomineralization is facilitated by interaction between self-assembled dentin matrix protein 1 and calcium phosphate nuclei in solution. Biochemistry. (2005) 44:16140–8. doi: 10.1021/bi051045l
20. Beniash E, Deshpande AS, Fang PA, Lieb NS, Zhang X, Sfeir CS. Possible role of DMP1 in dentin mineralization. J Struct Biol. (2011) 174:100–6. doi: 10.1016/j.jsb.2010.11.013
21. Jani PH, Gibson MP, Liu C, Zhang H, Wang X, Lu Y, et al. Transgenic expression of Dspp partially rescued the long bone defects of Dmp1-null mice. Matrix Biol. (2016) 52:95–112. doi: 10.1016/j.matbio.2015.12.001
22. Weng Y, Liu Y, Du H, Li L, Jing Q, Zhang X, et al. Glycosylation of DMP1 is essential for chondrogenesis of condylar cartilage. J Dent Res. (2017) 96:1535–45. doi: 10.1177/0022034517717485
23. Foster BL, Ao M, Salmon CR, Chavez MB, Kolli TN, Tran AB, et al. Osteopontin regulates dentin and alveolar bone development and mineralization. Bone. (2018) 107:196–207. doi: 10.1016/j.bone.2017.12.004
24. Boskey AL, Spevak L, Paschalis E, Doty SB, McKee MD. Osteopontin deficiency increases mineral content and mineral crystallinity in mouse bone. Calcif Tissue Int. (2002) 71:145–54. doi: 10.1007/s00223-001-1121-z
25. Sodek J, Ganss B, McKee MD. Osteopontin. Crit Rev Oral Biol Med. (2000) 11:279–303. doi: 10.1177/10454411000110030101
26. Gordon JA, Tye CE, Sampaio AV, Underhill TM, Hunter GK, Goldberg HA. Bone sialoprotein expression enhances osteoblast differentiation and matrix mineralization in vitro. Bone. (2007) 41:462–73. doi: 10.1016/j.bone.2007.04.191
27. Hunter GK, Goldberg HA. Nucleation of hydroxyapatite by bone sialoprotein. PNAS USA. (1993) 90:8562–5. doi: 10.1073/pnas.90.18.8562
28. Baht G, Hunter G, Goldberg H. Bone sialoprotein–collagen interaction promotes hydroxyapatite nucleation. Matrix Biol. (2008) 27:600–8. doi: 10.1016/j.matbio.2008
29. Ganss B, Kim RH, Sodek J. Bone sialoprotein. Crit Rev Oral Biol Med. (1999) 10:79–98. doi: 10.1177/10454411990100010401
30. Lu C, Huang S, Miclau T, Helms JA, Colnot C. Mepe is expressed during skeletal development and regeneration. Histochem Cell Biol. (2004) 121:493–9. doi: 10.1007/s00418-004-0653-5
31. Staines KA, MacRae VE, Farquharson C. The importance of the SIBLING family of proteins on skeletal mineralization and bone remodelling. J Endocrinol. (2012) 214:241–55. doi: 10.1530/JOE-12-0143
32. Linde A. Dentin matrix proteins: composition and possible functions in calcification. Anat Rec. (1989) 224:154–66. doi: 10.1002/ar.1092240206
33. Goldberg M. Dentin structure composition and mineralization. Front Biosci. (2011) 3:711–35. doi: 10.2741/e281
34. Yoshikawa DK, Kollar EJ. Recombination experiments on the odontogenic roles of mouse dental papilla and dental sac tissues in ocular grafts. Arch Oral Biol. (1981) 26:303–7. doi: 10.1016/0003-9969(81)90051-0
35. Palmer RM, Lumsden AGS. Development of periodontal ligament and alveolar bone in homografted recombinations of enamel organs and papillary, pulpal and follicular mesenchyme in the mouse. Arch Oral Biol. (1987) 32:281–9. doi: 10.1016/0003-9969(87)90022-7
36. Cho MI, Garant PR. Development and general structure of the periodontium. Periodontology. (2000) 24:9–27. doi: 10.1034/j.1600-0757.2000.2240102.x
37. Hovorakova M, Lesot H, Peterka M, Peterkova R. Early development of the human dentition revisited. J Anat. (2018) 233:135–45. doi: 10.1111/joa.12825
38. Jheon AH, Seidel K, Biehs B, Klein OD. From molecules to mastication: the development and evolution of teeth. Wiley Interdiscip Rev Dev Biol. (2013) 2:165–82. doi: 10.1002/wdev.63
39. Matalova E, Lungova V, Sharpe P. Development of Tooth and Associated Structures. In: Vishwakarma A, Sharpe P, Shi S, Ramalingam M, editors. Stem Cell Biology and Tissue Engineering in Dental Sciences. Cambridge, MA: Academic Press. (2015). p. 335–46. doi: 10.1016/B978-0-12-397157-9.00030-8
40. Yu T, Volponi AA, Babb R, An Z, Sharpe PT. Stem cells in tooth development, growth, repair, and regeneration. Curr Top Dev Biol. (2015) 115:187–212. doi: 10.1016/bs.ctdb.2015.07.010
41. Linde A, Goldberg M. Dentinogenesis. Crit Rev Oral Biol Med. (1993) 4:679–728. doi: 10.1177/10454411930040050301
42. Sreenath T, Thyagarajan T, Hall B, Longenecker G, D'Souza R, Hong S, et al. Dentin sialophosphoprotein knockout mouse teeth display widened predentin zone and develop defective dentin mineralization similar to human dentinogenesis imperfecta type III. J Biol Chem. (2003) 278:24874–80. doi: 10.1074/jbc.m303908200
43. Verdelis K, Ling Y, Sreenath T, Haruyama N, MacDougall M, Meulen MCH, et al. DSPP effects on in vivo bone mineralization. Bone. (2008) 43:983–90. doi: 10.1016/j.bone.2008.08.110
44. George A, Sabsay B, Simonian PAL, Veis A. Characterization of a novel dentin matrix acidic phosphoprotein. Implications for induction of biomineralization. J Biol Chem. (1993) 268:12624–30. doi: 10.1016/s0021-9258(18)31434-0
45. Macdougall M, Gu TT, Luan X, Simmons D, Chen J. Identification of a novel isoform of mouse dentin matrix protein 1: spatial expression in mineralized tissues. J Bone Miner Res. (1998) 13:422–431. doi: 10.1359/jbmr.1998.13.3.422
46. Almushayt A, Narayanan K, Zaki AE, George A. Dentin matrix protein 1 induces cytodifferentiation of dental pulp stem cells into odontoblasts. Gene Ther. (2006) 13:611–20. doi: 10.1038/sj.gt.3302687
47. Lu Y, Ye L, Yu S, Zhang S, Xie Y, McKee MD, et al. Rescue of odontogenesis in Dmp1-deficient mice by targeted re-expression of DMP1 reveals roles for DMP1 in early odontogenesis and dentin apposition in vivo. Dev Biol. (2007) 303:191–201. doi: 10.1016/j.ydbio.2006.11.001
48. Ling Y, Rios HF, Myers ER, Lu Y, Feng JQ, Boskey AL. DMP1 depletion decreases bone mineralization in vivo: an FTIR imaging analysis. J Bone Miner Res. (2005) 20:2169–77. doi: 10.1359/JBMR.050815
49. Narisawa S, Yadav MC, Millán JL. In vivo overexpression of tissue-nonspecific alkaline phosphatase increases skeletal mineralization and affects the phosphorylation status of osteopontin. J Bone Miner Res. (2013) 28:1587–98. doi: 10.1002/jbmr.1901
50. Foster BL, Nagatomo KJ, Tso HW, Tran AB, Nociti Jr FH, Narisawa S, et al. Tooth root dentin mineralization defects in a mouse model of hypophosphatasia. J Bone Miner Res. (2013) 28:271–282. doi: 10.1002/jbmr.1767
51. Gericke A, Qin C, Spevak L, Fujimoto Y, Butler WT, Sorensen ES, et al. Importance of phosphorylation for osteopontin regulation of biomineralization. Calcif Tissue Int. (2005) 77:45–54. doi: 10.1007/s00223-004-1288-1
52. Oldberg A, Franzen A, Heinegard D. The primary structure of a cell-binding bone sialoprotein. J Biol Chem. (1988) 263:19430–2. doi: 10.1016/S0021-9258(19)77651-0
53. Chen J, McCulloch CAG, Sodek J. Bone sialoprotein in developing porcine dental tissues: Cellular expression and comparison of tissue localization with osteopontin and osteonectin. Arch Oral Biol. (1993) 38:241–9. doi: 10.1016/0003-9969(93)90034-J
54. Kawaguchi H, Ogawa T, Kurihara H, Nanci A. Immunodetection of noncollagenous matrix proteins during periodontal tissue regeneration. J Periodont Res. (2001) 36:205–13. doi: 10.1034/j.1600-0765.2001.036004205.x
55. Foster BL, Ao M, Willoughby C, Soenjaya Y, Holm E, Lukashova L, et al. Mineralization defects in cementum and craniofacial bone from loss of bone sialoprotein. Bone. (2015) 78:150–64. doi: 10.1016/j.bone.2015.05.007
56. Vijaykumar A, Dyrkacz P, Vidovic-Zdrilic I, Maye P, Mina M. Expression of BSP-GFPtpz Transgene during Osteogenesis and Reparative Dentinogenesis. J Dent Res. (2020) 99:89–97. doi: 10.1177/0022034519885089
57. Wang H, Kawashima N, Iwata T, Xu J, Takahashi S, Sugiyama T, et al. Differentiation of odontoblasts is negatively regulated by MEPE via its C-terminal fragment. Biochem Biophys Res Commun. (2010) 398:406–12. doi: 10.1016/j.bbrc.2010.06.085
58. Gullard A, Gluhak-Heinrich J, Papagerakis S, Sohn P, Unterbrink A, Chen S, et al. Localization in the craniofacial complex and function in tooth dentin formation. J Histochem Cytochem. (2016) 64:224–36. doi: 10.1369/0022155416635569
59. Devoll RE Li W, Woods KV, Pinero GJ, Butler WT, Farach-Carson MC, et al. Osteopontin. (OPN) distribution in premalignant and malignant lesions of oral epithelium and expression in cell lines derived from squamous cell carcinoma of the oral cavity. J Oral Pathol Med. (2007) 28:97–101. doi: 10.1111/j.1600-0714.1999.tb02004.x
60. Chien CY, Su CY, Chuang HC, Fang FM, Huang HY, Chen CH, et al. Comprehensive study on the prognostic role of osteopontin expression in oral squamous cell carcinoma. Oral Oncol. (2009) 45:798–802. doi: 10.1016/j.oraloncology.2008.12.006
61. Gibson MP, Zhu Q, Liu Q, D'Souza RN, Feng JQ, Qin C. Loss of dentin sialophosphoprotein leads to periodontal diseases in mice. J Periodont Res. (2013) 48:221–7. doi: 10.1111/j.16000765.2012.01523.x
62. Cho MI, Garant PR. Radioautographic study of [3H]mannose utilization during cementoblast differentiation, formation of acellular cementum, and development of periodontal ligament principal fibers. Anat Rec. (1989) 223:209–22. doi: 10.1002/ar.1092230214
63. Cho MI, Garant PR. Expression and role of epidermal growth factor receptors during differentiation of cementoblasts, osteoblasts, and periodontal ligament fibroblasts in the rat. Anat Rec. (1996) 245:342–60. doi: 10.1002/(sici)1097-0185(199606)245:2<342::aid-ar16>3.0.co;2-p
64. Freeman E, Ten Cate AR. Development of the Periodontium: An Electron Microscopic Study. J Periodontol. (1971) 42:387–95. doi: 10.1902/jop.1971.42.7.387
65. McCulloch CAG. Progenitor cell populations in the periodontal ligament of mice. Anat Rec. (1985) 211:258–62. doi: 10.1002/ar.1092110305
66. McCulloch CAG. Basic considerations in periodontal wound healing to achieve regeneration. Periodontology. (1993) 1:16–25. doi: 10.1111/j.1600-0757.1993.tb00203.x
67. McCulloch CAG, Nemeth E, Lowenberg B, et al. Paravascular cells in endosteal spaces of alveolar bone contribute to periodontal ligament cell populations. Anat Rec. (1987) 219:233–42. doi: 10.1002/ar.1092190304
68. Steitz SA, Speer MY, McKee MD, Liaw L, Almeida M, Yang H, et al. Osteopontin inhibits mineral deposition and promotes regression of ectopic calcification. Am J Pathol. (2002) 161:2035–46. doi: 10.1016/S0002-9440(10)64482-3
69. Speer MY, McKee MD, Guldberg RE, Liaw L, Yang HY, Tung E, et al. Inactivation of the osteopontin gene enhances vascular calcification of matrix Gla protein-deficient mice: evidence for osteopontin as an inducible inhibitor of vascular calcification in vivo. Exp Med. (2002) 196:1047–55. doi: 10.1084/jem.20020911
70. Speer M, Chien Y, Quan M, Yang HY, Vali H, McKee MD, et al. Smooth muscle cells deficient in osteopontin have enhanced susceptibility to calcification in vitro. Cardiovasc Res. (2005) 66:324–33. doi: 10.1016/j.cardiores.2005.01.023
71. Wesson JA. Osteopontin Is a Critical Inhibitor of Calcium Oxalate Crystal Formation and Retention in Renal Tubules. J Am Soc Nephrol. (2003) 14:139–47. doi: 10.1097/01.asn.0000040593.93815.9d
72. Mo L, Liaw L, Evan AP, Sommer AJ, Lieske JC, Wu XR. Renal calcinosis and stone formation in mice lacking osteopontin, Tamm-Horsfall protein, or both. Am J Physiol Renal Physiol. (2007) 293:F1935–43. doi: 10.1152/ajprenal.00383.2007
73. Harmey D, Johnson KA, Zelken J, Camacho NP, Hoylaerts MF, Noda M, et al. Elevated skeletal osteopontin levels contribute to the hypophosphatasia phenotype in Akp2/mice. J. Bone Miner. Res. (2006) 21:1377–1386. doi: 10.1359/jbmr.060619
74. Nanci A. General embryology. In: Nanci A, editor. Ten Cate's Oral Histology. (Eighth Edition). St. Louis, MO: Mosby. (2013) p. 205–32. doi: 10.1016/B978-0-323-07846-7.000094
75. Merkel A, Chen Y, George A. Endocytic trafficking of DMP1 and GRP78 complex facilitates osteogenic differentiation of human periodontal ligament stem cells. Front Physiol. (2019) 10:1–14. doi: 10.3389/fphys.2019.01175
76. Ye L, Zhang S, Ke H, Bonewald LF, Feng JQ. Periodontal breakdown in the Dmp1 null mouse model of hypophosphatemic rickets. J Dent Res. (2008) 87:624–9. doi: 10.1177/154405910808700708
77. Schroeder HE. Development, structure, and function of periodontal tissues. In: The Periodontium Handbook of Microscopic Anatomy. Springer, Berlin, Heidelberg. (1986). p. 23–323. doi: 10.1007/978-3-642-71261-6_4
78. Tencate AR, Mills C. The development of the periodontium: the origin of alveolar bone. Anat Rec. (1972) 173:69–77. doi: 10.1002/ar.1091730106
79. Hoffman RL. Bone formation and resorption around developing teeth transplanted into the femur. American Crit Rev Oral Biol Med. (1966) 118:91–102. doi: 10.1002/aja.1001180106
80. TenCate AR, Mills C, Solomon G. The development of the periodontium. A transplantation and autoradiographic study. Anat Rec. (1971) 170:365–79. doi: 10.1002/ar.1091700312
81. Cahill DR. Eruption pathway formation in the presence of experimental tooth impaction in puppies. Anat Rec. (1969) 164:67–77. doi: 10.1002/ar.1091640105
82. Cahill DR, Marks SC. Tooth eruption: evidence for the central role of the dental follicle. J Oral Pathol Med. (1980) 9:189–200. doi: 10.1111/j.1600-0714.1980.tb00377.x
83. Larson EK, Cahill DR, Gorski JP, Marks Jr SC. The effect of removing the true dental follicle on premolar eruption in the dog. Arch Oral Biol. (1994) 39:271–5. doi: 10.1016/0003-9969(94)90116-3
84. Brunski JB. Biomechanical factors affecting the bone-dental implant interface. Clin Mater. (1992) 10:153–201. doi: 10.1016/0267-6605(92)90049-y
85. Rittling SR, Matsumoto HN, Mckee MD, Nanci A, An XR, Novick KE, et al. Mice lacking osteopontin show normal development and bone structure but display altered osteoclast formation in vitro. J Bone Miner Res. (1998) 13:1101–1111. doi: 10.1359/jbmr.1998.13.7.1101
86. Ishijima M, Rittling SR, Yamashita T, Tsuji K, Kurosawa H, Nifuji A, et al. Enhancement of osteoclastic bone resorption and suppression of osteoblastic bone formation in response to reduced mechanical stress do not occur in the absence of osteopontin. Exp Med. (2001) 193:399–404. doi: 10.1084/jem.193.3.399
87. Ishijima M, Tsuji K, Rittling SR, Yamashita T, Kurosawa H, Denhardt DT, et al. Resistance to unloading-induced three-dimensional bone loss in osteopontin-deficient mice. J Bone Miner Res. (2002) 17:661–7. doi: 10.1359/jbmr.2002.17.4.661
88. Chellaiah MA, Hruska KA. The integrin {alpha}v {beta}3 and CD44 regulate the actions of osteopontin on osteoclast motility. Calcif Tissue Int. (2003) 72:197–205. doi: 10.1007/s00223-002-1025-6
89. Chellaiah MA, Kizer N, Biswas R, Alvarez U, Strauss-Schoenberger J, Rifas L, et al. Osteopontin deficiency produces osteoclast dysfunction due to reduced CD44 surface expression. Mol Biol Cell. (2003) 14:173–89. doi: 10.1091/mbc.e02-06-0354
90. Chung CJ, Soma K, Rittling SR, Denhardt DT, Hayata T, Nakashima K, et al. OPN deficiency suppresses appearance of odontoclastic cells and resorption of the tooth root induced by experimental force application. J Cell Physiol. (2007) 214:614–20. doi: 10.1002/jcp.21250
91. Chen Y, Zhang Y, Ramachandran A, George A, DSPP. Is essential for normal development of the dental-craniofacial complex. J Dent Res. (2016) 95:302–10. doi: 10.1177/0022034515610768
92. Feng JQ, Ward LM, Liu S, Lu Y, Xie Y, Yuan B, et al. Loss of DMP1 causes rickets and osteomalacia and identifies a role for osteocytes in mineral metabolism. Nat Genet. (2006) 38:1310–5. doi: 10.1038/ng1905
93. Qin C, D'Souza R, Feng JQ. Dentin matrix protein 1. (DMP1): new and important roles for biomineralization and phosphate homeostasis. J Dent Res. (2007) 86:1134–41. doi: 10.1177/154405910708601202
94. Gibson MP, Zhu Q, Wang S, Liu Q, Liu Y, Wang X, et al. The rescue of dentin matrix protein 1. (DMP1)-deficient tooth defects by the transgenic expression of dentin sialophosphoprotein. (DSPP) indicates that DSPP is a downstream effector molecule of DMP1 in dentinogenesis. J Biol Chem. (2013) 10:7204–14. doi: 10.1074/jbc.M112.445775
95. Ichikawa S, Gerard-O'Riley RL, Acton D, McQueen AK, Strobel IE, et al. A mutation in the Dmp1 gene alters phosphate responsiveness in mice. Endocrinology. (2017) 158:470–6. doi: 10.1210/en.2016-1642
96. Beck-Nielsen SS, Mughal Z, Haffner D, Nilsson O, Levtchenko E, Ariceta G, et al. FGF23 and its role in X-linked hypophosphatemia-related morbidity. Orphanet J Rare Dis. (2019) 14:58. doi: 10.1186/s13023-019-1014-8
97. Millan JL, Whyte MP. Alkaline phosphatase and hypophosphatasia. Calcif Tissue Int. (2016) 98:398–416. doi: 10.1007/s00223-015-0079-1
98. Bruckner RJ, Rickles NH, Porter DR. Hypophosphatasia with premature shedding of teeth and aplasia of cementum. Oral Surg Oral Med Oral Pathol. (1962) 15:1351–69. doi: 10.1016/0030-4220(62)90356-0
99. Foster BL, Ramnitz MS, Gafni RI, Burke AB, Boyce AM, Lee JS, et al. Rare bone diseases and their dental, oral, and craniofacial manifestations. J Dent Res. (2014) 93:7S−19S. doi: 10.1177/0022034514529150
100. Zweifler LE, Patel MK, Nociti Jr FH, Wimer HF, Millan JL, Sommerman MJ, et al. Counter-regulatory phosphatases TNAP and NPP1 temporally regulate tooth root cementogenesis. Int J Oral Sci. (2015) 7:27–41. doi: 10.1038/ijos.2014.62
101. McKee MD, Nakano Y, Masica DL, Gray JJ, Lemire I, Heft R, et al. Enzyme replacement therapy prevents dental defects in a model of hypophosphatasia. J Dent Res. (2011) 90:470–6. doi: 10.1177/0022034510393517
102. Beertsen W, VandenBos T, Everts V. Root development in mice lacking functional tissue non-specific alkaline phosphatase gene: inhibition of acellular cementum formation. J Dent Res. (1999) 78:1221–9. doi: 10.1177/00220345990780060501
103. Van Den Bos T. G., Handoko A, Niehof Ryan LM, Coburn SP, Whyte MP, et al. Cementum and dentin in hypophosphatasia. J Dent Res. (2005) 84:1021–5. doi: 10.1177/154405910508401110
104. Foster BL, Kuss P, Yadav MC, Kolli TN, Narisawa S, Lukashova L, et al. Conditional Alpl ablation phenocopies dental defects of hypophosphatasia. J Dent Res. (2017) 96:81–91. doi: 10.1177/0022034516663633
105. Yadav MC, Oliveira RC, Foster BL, Fong H, Cory E, Narisawa S, et al. Enzyme replacement prevents enamel defects in hypophosphatasia mice. J Bone Miner Res. (2012) 27:1722–1734. doi: 10.1002/jbmr.1619
106. Foster BL, Soenjaya Y, Nociti Jr FH, Holm E, Zerfas PM, Wimer HF, et al. Deficiency in acellular cementum and periodontal attachment in BSP null mice. J Dent Res. (2013) 92:166–72. doi: 10.1177/0022034512469026
107. Foster BL, Nagatomo KJ, Nociti Jr FH, Fong H, Dunn D, Tran AB, et al. Central role of pyrophosphate in acellular cementum formation. PLoS ONE. (2012) 6:e38393. doi: 10.1371/journal.pone.0038393
108. Soenjaya Y, Foster BL, Nociti Jr FH, Ao M, Holdsworth DW, Hunter MJ, et al. Mechanical forces exacerbate periodontal defects in Bsp-null mice. J Dent Res. (2015) 94:1276–85. doi: 10.1177/0022034515592581
109. Jin SW, Sim KB, Kim SD. Development and growth of the normal cranial vault: an embryologic review. J Korean Neurosurg Soc. (2016) 59:192. doi: 10.3340/jkns.2016.59.3.192
110. Ikegame M, Ejiri S, Okamura H. Expression of non-collagenous bone matrix proteins in osteoblasts stimulated by mechanical stretching in the cranial suture of neonatal mice. J Histochem Cytochem. (2019) 2:107–16. doi: 10.1369/0022155418793588
111. Ross FP, Chappel J, Alvarez JI, Sander D, Butler WT, Farach-Carson MC, et al. Interactions between the bone matrix proteins osteopontin and bone sialoprotein and the osteoclast integrin alpha v beta 3 potentiate bone resorption. J Biol Chem. (1993) 268:9901–7.
112. Cai M, Li J, Yue R, Wang Z, Sun Y. Glycosylation of DMP1 maintains cranial sutures in mice. J Oral Rehabil. (2020) 47:19–28. doi: 10.1111/joor.12881
113. Bouleftour W, Bouet G, Granito RN, Thomas M, Linossier MT, Vanden-Bossche A, et al. Blocking the expression of both bone sialoprotein. (BSP) and osteopontin. (OPN) impairs the anabolic action of PTH in mouse calvaria bone. J Cell Physiol. (2015) 230:568–77. doi: 10.1002/jcp.24772
Keywords: SIBLING proteins, periodontal, craniofacial, dentin, PDL
Citation: Figueredo CA, Abdelhay N and Gibson MP (2022) The Role of SIBLING Proteins in Dental, Periodontal, and Craniofacial Development. Front. Dent. Med. 3:898802. doi: 10.3389/fdmed.2022.898802
Received: 17 March 2022; Accepted: 23 May 2022;
Published: 24 June 2022.
Edited by:
Brian L. Foster, The Ohio State University, United StatesReviewed by:
Elizabeth Guirado, University of Illinois at Chicago, United StatesCopyright © 2022 Figueredo, Abdelhay and Gibson. This is an open-access article distributed under the terms of the Creative Commons Attribution License (CC BY). The use, distribution or reproduction in other forums is permitted, provided the original author(s) and the copyright owner(s) are credited and that the original publication in this journal is cited, in accordance with accepted academic practice. No use, distribution or reproduction is permitted which does not comply with these terms.
*Correspondence: Monica P. Gibson, bWdpYnNvbkB1YWxiZXJ0YS5jYQ==