- 1School of Dentistry, College of Biomedical and Life Sciences, Cardiff Institute for Tissue Engineering and Repair, Cardiff University, Cardiff, United Kingdom
- 2School of Medicine, College of Biomedical and Life Sciences, Cardiff Institute for Tissue Engineering and Repair, Cardiff University, Cardiff, United Kingdom
Mesenchymal stromal cells (MSCs) have long been the focus for regenerative medicine and the restoration of damaged or aging cells throughout the body. However, the efficacy of MSCs in cell-based therapy still remains unpredictable and carries with it enumerable risks. It is estimated that only 3-10% of MSCs survive transplantation, and there remains undefined and highly variable heterogeneous biological potency within these administered cell populations. The mode of action points to secreted factors produced by MSCs rather than the reliance on engraftment. Hence harnessing such secreted elements as a replacement for live-cell therapies is attractive. Extracellular vesicles (EVs) are heterogenous lipid bounded structures, secreted by cells. They comprise a complex repertoire of molecules including RNA, proteins and other factors that facilitate cell-to-cell communication. Described as protected signaling centers, EVs can modify the cellular activity of recipient cells and are emerging as a credible alternative to cell-based therapies. EV therapeutics demonstrate beneficial roles for wound healing by preventing apoptosis, moderating immune responses, and stimulating angiogenesis, in addition to promoting cell proliferation and differentiation required for tissue matrix synthesis. Significantly, EVs maintain their signaling function following transplantation, circumventing the issues related to cell-based therapies. However, EV research is still in its infancy in terms of their utility as medicinal agents, with many questions still surrounding mechanistic understanding, optimal sourcing, and isolation of EVs for regenerative medicine. This review will consider the efficacy of using cell-derived EVs compared to traditional cell-based therapies for bone repair and regeneration. We discuss the factors to consider in developing productive lines of inquiry and establishment of standardized protocols so that EVs can be harnessed from optimal secretome production, to deliver reproducible and effective therapies.
Introduction
Extracellular vesicles (EVs), including exosomes, ectosomes, and apoptotic bodies, represent intercellular messengers secreted by cells to deliver biological signals. They were first biochemically assessed in the 1980s as cell-secreted vesicles (1–3) and initially dismissed as cell debris. However, a seminal discovery by Raposo et al. (4) identified their capacity to transmit signals form cell-to-cell, and to stimulate functional responses. This was a critical step in our appreciation of EVs as fundamental components for intercellular communication.
All EVs consist of an outer lipid bilayer, heavily decorated with membrane proteins, and encapsulating within the vesicle lumen an assortment of molecular constituents such as proteins, lipids, DNA and RNA (5). Nevertheless, their structures can be diverse and include unilamellar small vesicles (30-150 nm), multilamellar vesicles and sometimes other larger and irregular structures, all recognized as components of the vesicle secretome (6). These structures, their intracellular origins and mechanisms of regulation are incompletely understood, yet there is a wealth of examples describing intercellular transfer of such vesicles to diverse recipient cell types, eliciting complex cellular responses. Vesicle-mediated communication, and the underpinning mechanisms are highly complex, involving binding and signaling events at the recipient cell surface as well as vesicle internalization, principally via endocytosis and intracellular processing (7).
It is within this context, as important and naturally occurring signaling centers, the investigation of EV biology is becoming a new “hot topic” in regenerative medicine. Understanding and then harnessing this signaling potential for medical applications is very exciting, and there are various studies advocating the potential of EVs for restoring tissue and organ damage and enhancing regeneration in numerous diseases (8). This review therefore aims to summarize the available evidence on the efficacy of utilizing cell-derived EVs in promoting bone repair and regeneration and their advantages compared to conventional cell-based therapies and the technical challenges that need to be recognized if we are to achieve successful clinical translation.
The Major Classes of EVs
Under this generic term, EVs are broadly divided into three subtypes: exosomes, microvesicles (or ectosomes) and apoptotic bodies. These categories consider principally the cellular origins of the vesicles with full acknowledgment that there are overlaps in terms of the biophysical properties, molecular cargo and potentially function across these highly diverse vesicle types (Figure 1). Although, the molecular composition and RNA profiles of these classes of extracellular vesicles are distinct (9), this can be exploited to identify subtypes. Whilst exosomes and ectosomes may be produced under constitutive / steady-state conditions, or in response to exogenous stimuli, apoptotic structures are, naturally, a function of programmed cell death and do not normally feature when dealing with cell populations with high viability. Vesicle output from any system is inevitably dynamic in terms of the quantity and molecular composition of EVs, and this would indeed be consistent with their roles in delivering intercellular communication and/or in maintaining cellular integrity through the elimination of unwanted material. When considering their potential for tissue repair and regeneration, all three subsets have been identified as plausible candidates.
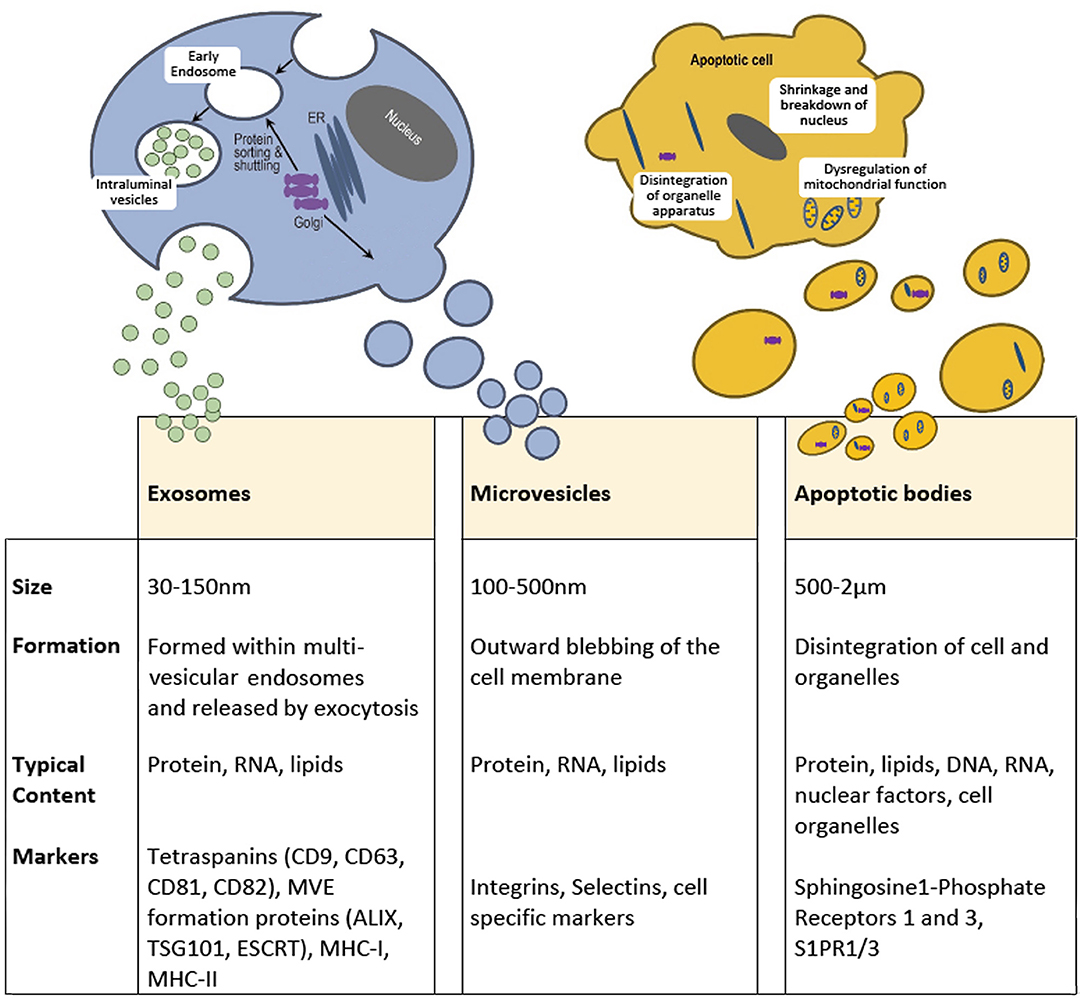
Figure 1. Extracellular vesicles are broadly divided into three subtypes based on size, formation, and content. Exosomes range from 30 to 150 mn and originate within multivesicular endosomes and carry protein, RNA and lipids. Microvesicles or Ectosomes, are often but not always larger and form through an outward budding of the plasma membrane and also contain protein, RNA and lipids. Microvesicles express surface markers specific to the membranous elements of the cell of origin, including integrins and selectins. Apoptotic bodies are highly diverse and can be a few microns in diameter but are a property of dying cells and therefore contain cell debris, organelles, nuclear factors, alongside protein, mRNA and lipids. The molecular composition of these classes of extracellular vesicle are distinct.
Exosomes, arise from intraluminal vesicles (ILVs) of multivesicular endosomes (MVE), compartments that are sometimes termed multivesicular bodies (MVB). These EVs are size limited by virtue of their endosomal origins and are typically described as within a range of 30-150 nm in diameter (10). Exosomes are formed by the inward budding of the limiting membrane of the MVE, the intracellular sorting organelles involved in protein sorting, recycling, storage, and release. Transportation of MVEs to the plasma membrane ultimately leads to exocytosis involving fusion with the plasma membrane (11). Endosomal sorting mechanisms will guide the content and release of exosomes, which may be constitutive or in response to cellular activation by extracellular signals (12). The most common transmembrane markers to identify exosomes come from the tetraspanin family of proteins (13). Specific cellular machinery, e.g., ALIX, ESCRT and TSG101, is required for formation of MVEs and can be useful markers for characterization. Microvesicles on the other hand are formed by the outward budding of the plasma membrane and are typically larger than exosomes, from 100 nm up to 500 nm in diameter (10). Cargo is directed to the site of microvesicles biogenesis by vacuolar trafficking of a cargo to the plasma membrane; a process that involves an increase in intracellular calcium that activates cell membrane proteins such as calpain (12). Plasma membrane budding occurs in specific regions of the plasma membrane, such as in regions enriched in cholesterol, as a loss in cellular cholesterol results in an inhibition of microvesicle release (14, 15). Actin-myosin contractile machinery is then utilized to facilitate the release of these microvesicles into the extracellular space. Studies have indicated there is an enrichment of protein content within EVs compared to the parent cell (16). However, following release, selective shedding of proteins can further modify the surface and intra-vesicle content compared to the parent cell (17). Of note, osteoblast-like cells have been shown to release EVs, that are concentrated in calcium and inorganic phosphate along with calcium binding proteins, generating embryo crystals that release their content within the collagen scaffold leading to deposition of hydroxyapatite (18, 19).
Finally, apoptotic bodies are released by dying cells into the extracellular space and are the largest of the EVs described here, ranging from 500 nm to 2 μm in diameter. Unlike exosomes and microvesicles, apoptotic bodies contain intact organelles, micronuclei, chromatin, and cytosol portions, in addition to DNA fragments and degraded proteins (20). Unique protein markers have been proposed to be induced by cell damage and can be used to identify apoptotic bodies (21). After their release into the extracellular space, apoptotic bodies are classically described to be phagocytosed by immune surveillant cells such as macrophages, finalizing the process for removal of damaged or senescent cells. However, apoptotic bodies have also been shown to be engulfed by other cell types, including endothelial cells promoting angiogenesis (22) and osteoblasts during formation of alveolar bone in rat maxilla, via activation of Wnt/β-catenin pathways (23). Indeed, apoptotic bodies from osteoclasts have been identified to be taken up by pre-osteoblasts promoting differentiation (24), whilst apoptotic bodies derived from osteocytes have been reported to stimulate osteoclast formation (25). This suggests that apoptotic bodies play divergent roles in the repair and regeneration of tissues, dependent upon their source and nature of origin.
While EVs are sub-classified as either exosomes, microvesicles, or apoptotic bodies, they are often difficult to separate due to overlap in their size ranges, markers, and cargos. Thus, in this review, we will solely use the term EV and include characterization details were available.
Limitations of MSC Cell-Based Therapies
Results from numerous clinical studies indicate the huge therapeutic potential for using multipotent mesenchymal stromal cells (MSCs), in what are collectively referred to, albeit often inaccurately as, “stem” cell-based therapies for regenerative medicine (26). MSCs can be isolated from a range of connective tissues, and most frequently from bone marrow, adipose tissue, umbilical cord blood and the dental pulp. However, despite this, the clinical application of MSCs in “stem” cell-based therapies has raised various drawbacks; most notably that obtaining adult MSCs can be invasive and comes with risks of morbidity or pain to the patient/donor. Induced pluripotent stem cells (iPSCs), which involves the reprogramming of adult somatic cells to a pluripotent state, by insertion of genes for specific transcription factors of c-Myc/Klf4/Oct4/Sox2 or Lin28/Nanog/Oct4/Sox2, can be a useful alternative (27). Their subsequent differentiation to defined MSC cell types gets around the issue of adult MSC cell harvesting.
Critically, the MSCs isolated (either adult MSC or iPSC derived) need to be able to replace the damaged cells in the patient and therefore must be able to differentiate into the desired cell types in a tightly controlled manor. However, one of the main safety concerns with cell-based therapy is the problem of uncontrolled differentiation into incorrect linages (28). MSC populations are highly heterogenous in nature, therefore for all tissue donor sources, the harvested transplanted cells represent an undefined and highly variable mix of multi-, tri-, bi-potent progenitor cells, committed uni-potent precursor cells and differentiated cells (29, 30). The biological response and duration of each population subset in achieving a terminally differentiated cells would expectantly be different within the transplanted site, thus resulting in an unpredictable efficacy, influencing clinical success. Results obtained from various in vitro studies have expressed concerns regarding the possibility of adult MSCs differentiating into ectodermal and endodermal cells (31). Meanwhile, other reports have highlighted data concerning the spontaneous differentiation of MSCs into unwanted cell types post-transplantation (32, 33). Additionally, safety concerns surrounding the genomic instability of genetically reprogrammed iPSC-MSCs have further hindered their clinical translation. The expression of recognized oncogenes is thought to increase during the reprogramming process and contribute to the role iPSCs play in tumorigenicity (34, 35), despite multiple studies reporting no observable teratogenic effects of iPSC-MSCs in vivo (36, 37). Nevertheless, the development of effective methods for the generation of well-characterized MSC cell populations that have predictable differentiation pathways remains challenging in the field of regenerative medicine (38).
Indeed, the efficiency of MSC cell-based therapies has been called into question, given the high capital investment and cost of cell culture in producing a sufficient number of cells required to bring about an efficacious action for such treatments. Studies using MSC transplantation for the treatment of spinal cord injury have shown the transplanted cells do not survive long term and disappear several weeks after transplantation (39). In fact, of the cells transplanted during MSC cell-based therapy, it is predicted that only 3-10% of cells actually survive the transplantation process (40, 41). Issues of aging and collection of cells associated systemic diseases, such as osteoporosis and type 2 diabetes, can further impair the regenerative capacity of MSCs (42).
An additional, but important consideration for cell-based therapies, is immunological tolerance between the transplanted MSCs and the patient, which still remains to be addressed (43). Numerous studies have identified that MSCs can exhibit both pro-inflammatory and anti-inflammatory phenotypes, dependent upon the extracellular signaling cues of the healing environment, thereby modulating the immune responses [reviewed by (44)]. Initially, activation of Toll-like receptors (TLR2 and TLR4) by bacterial factors such as lipopolysaccharide and peptidoglycan promote the formation of the pro-inflammatory MSC1 phenotype, which are essential for the triggering early immune responses leading to the recruitment and activation of innate and adaptive immune cells. The activation of lymphocytes and M1 macrophages is essential for the healing process, where the secretion of pro-inflammatory cytokines such as TNFα, IFNλ, IL-1α, and IL-1β or exposure to double stranded RNA, triggers a switch to the MSC2 anti-inflammatory phenotype. This latter MSC phenotype is essential for controlling lymphocyte proliferation, formation of Tregs and switch of macrophages to the M2 anti-inflammatory phenotype. Consequently, balancing of this pro- and anti-inflammatory MSC phenotype is central to achieving effective immune control of tissue repair and regeneration and an inappropriate signaling environment would be detrimental in the repair process; whether due to inability to form MSC1 phenotype and hence inability to trigger the switch to MSC2 phenotype, or prolonged stimulation of MSC1 phenotype stimulating pro-inflammatory lymphocyte and M1 macrophage activity. Indeed, it is interesting to note that preliminary studies have reported that EVs from numerous cell sources can provide an immunomodulatory role via mechanisms that stimulate anti-inflammatory responses (45). In fact, the administration of patient MSC-derived EVs to treat graft vs. host disease, successfully reduced the pro-inflammatory immune response, demonstrating the efficacy of EV administration as an immune supressing medicine (46). From this understanding, it is important to recognize the possible dangers that EV-treatments pose as a potential immune suppressor. Studies in this field are very much in their infancy, but it is clear that characterizing the phenotype of the MSC would be critical to producing a favorable response.
Assessing the EVs Cargo for Bone Repair and Regeneration
As previously eluded to, one of the first descriptions of EVs was the observed release of matrix vesicles or microvesicles by osteoblasts and hypertrophic chondrocytes during bone development. Readily seen using electron microscopy, these microvesicles were originally discovered at the initial sites of mineral formation in the growth plate, prior to matrix mineralization, where they are regarded to play an important role in extracellular matrix mineralization (47). The microvesicles, released from the plasma membrane, are rich for calcium and phosphate which form embryo crystals nurturing hydroxyapatite nucleation and crystal growth. In addition, they have been shown to contain tissue non-specific alkaline phosphatase (TNAP) and phosphodiesterase (NPP1/PC-1) which have been proposed collaborative roles that regulates the onset of calcification. Meanwhile annexins have also been identified, representing a family of proteins that bind calcium and play a critical role in initiating the mineralization process.
More recently the role of EVs in intercellular communication during bone remodeling has been realized. EVs released from various bone cells encapsulate proteins that regulate bone remodeling. These include osteogenic factors bone morphogenic proteins (BMPs) and Alkaline phosphatase (ALP), and factors that stimulate osteoclast 453 differentiation such as Receptor activator of nuclear factor kappa-B ligand (RANKL) and its receptor RANK (48). Studies in this area are summarized in Table 1. However, the mechanism of EV bioactivity is now recognized as highly complex and extends beyond these regulatory growth factors. Studies with BMSC derived EVs show a loss in osteogenic potential upon in vitro miRNA depletion via prolonged incubation with RNases, highlighting the role of miRNA in bone formation (72). Indeed, a number of microRNAs (miRNA) and long non-coding RNA (lncRNA) have been reported to play an important role in osteoblastic differentiation; forming highly complex regulatory networks that further controls osteogenic gene expression. Specifically, crosstalk between miRNA and lncRNA has been identified in osteogenic pathways such as TGF-β/BMP-SMAD-dependent and Wnt/β-catenin signaling, through which they can modulate cell differentiation, including the osteogenesis of BMSCs (73).
Studies have also looked at EVs produced by other cells involved in bone remodeling, such as osteocytes (representing terminally differentiated osteoblasts) and osteoclasts. Osteoclasts communicate with osteoblasts through the secretion of micro-RNA enriched exosomes, containing specifically miR-214, which is then taken up by osteoblasts to inhibit their function and regulate their activity (74). Further studies have demonstrated the inhibitory effects on osteoblasts of osteoclast-derived exosomal miR-214 both in vitro and in vivo (60). Additionally, terminally differentiated osteocytes secrete exosomes containing miR-29, miR-484 and miR-221 (56). When co-cultured with osteoblasts, these osteocyte derived exosomes become localized in the nucleus of recipient osteoblast cells and stimulate their differentiation (57).
Additionally, many immune cells, such as T cells, dendritic cells, and monocytes, are known to play important regulatory roles in bone remodeling. Studies investigating the effect of EVs released by these immune cells are in their infancy and currently inconclusive. Studies have shown that dendritic-cell-derived exosomes are endocytosed by hBMSCs and promote their recruitment and migration, although these exosomes did not impact on hBMSCs proliferation or osteogenic differentiation (75). Exosomes from monocytes, however, have been shown to stimulate the osteogenic differentiation of BMSCs, implicating immune derived EVs in the transition from the inflammatory stage to the regenerative stage of bone injury repair (76).
A common consensus has thus emerged that the effects of MSCs during regenerative treatments is mediated largely by their paracrine actions. Indeed, the studies discussed above have demonstrated the osteogenic and regenerative effects of various cell types can be mimicked by the EVs produced by the parent cells. It is the secretions of growth factors, cytokines, and regulatory RNAs contained within the EVs that mediate and promote tissue repair, rather than the engraftment of the mesenchymal progenitor cells and their subsequent differentiation at the sites of injury (77–80). It therefore stands to reason that finding ways to take advantage of these EVs is a worthwhile pursuit in the quest for improved therapeutics for bone regeneration and repair. Significantly, molecular analysis has frequently revealed that the presence of miRNAs, growth factors and cytokines, is higher within the secreted EV compared to the cell lysates of the parent cell (81). Due to this enrichment of bioactive factors, it could be suggested that treatments involving the administration of EVs are likely to be more effective over the transplantation of cells. Of note, studies by Osugi et al. have demonstrated that bone repair within a calvarial defect model was more extensive in experimental groups treated with conditioned media collected from human MSCs when compared with the transplantation of human BMSCs (82). Further investigation suggested that IGF-1 and VEGF were two paracrine signaling factors in the conditioned media that acted on the endogenous cell populations in the injured rat to mediate bone repair (83). Moreover, by selection of defined cell sub-populations and controlled and systematic modulation of culture conditions, EV production and content can be planned to provide a powerful and desirable cellular response. EVs offer potential to circumvent issues presented by transplanted cells which can change their biological phenotype following placement in a wound healing environment, compounded by poor cell survival where release of apoptotic bodies can in turn unpredictably induce either a pro-inflammatory extracellular signaling milieu through the transfer of their cargo to recipient dendritic cells or suppress the immune system (84). Consequently, compared to the direct use of mesenchymal cell populations for regenerative medicine and due to their small size and relative ease of handling, predictions are made that EVs can be produced on a large scale at a lower cost (85).
Isolation of EVs
As the excitement surrounding EVs and their regenerative potential has greatly increased over the past decade, achieving a harmony surrounding the appropriate experimental conditions in which to harvest EVs has come under scrutiny, but there is currently no accepted consensus. Factors that alter the quality and quantity of EVs produced by cells in vitro are summarized in Figure 2. A number of protocols have been developed, some of which may be suitable for EV production at scale and in a manner that is compatible with regulatory authority requirements. A position statement by the International Society for Extracellular Vesicles (ISEV) details many common issues in suitably defining the EV product (86, 87). Critical issues surround assessment for the reproducibility of EV isolates, and measures of biological potency and purity. However, the culture environment can be a critical and often under-appreciated determinant of cell status, and hence can be highly impactful on the quantity and molecular composition of EVs being secreted. Culture supplements, cell confluence, time in culture, nutritional and oxygenation conditions, pH and a range of other variables are known influences on EV production. Developing systems, therefore, that offer the capacity to produce EVs at scale, in a highly stable and well-defined culture environment, are non-trivial challenges. Similarly, EV isolation methods capable of handling often large volumes of cell conditioned media can influence the nature of the EV populations being concentrated and regulatory agencies are indeed concerned with the standardization and traceability of such workflows. Ensuring batch reproducibility and indeed, assays to confirm consistent EV production are essential elements if EV are to be used clinically.
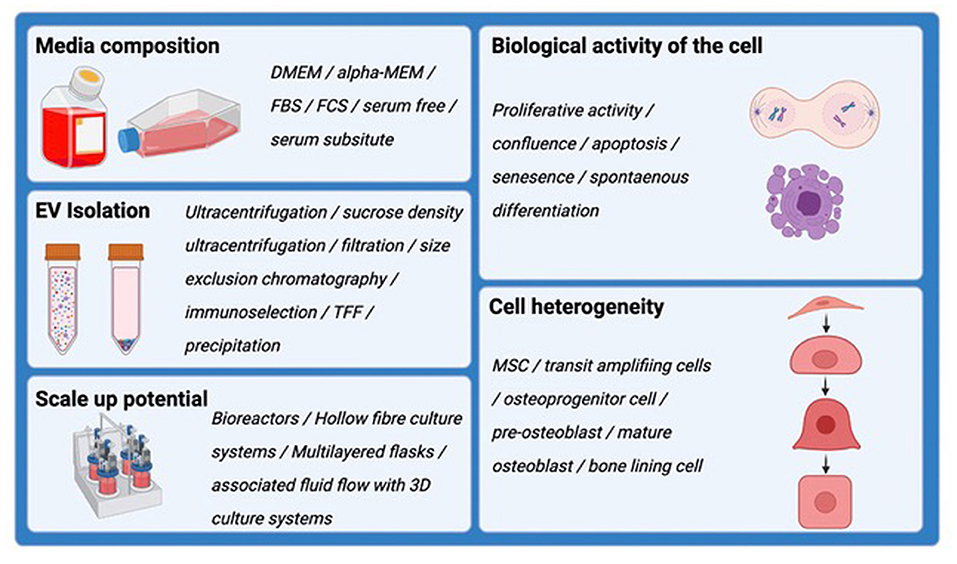
Figure 2. Schematic demonstrating the factors that alter the quality and quantity of EVs produced by cells in vitro. These should all be considered when harvesting EVs for downstream analysis and therapeutic application.
Formulation of the Conditioned Media
One challenge in the analysis of EVs isolated from conditioned medium is contamination by avesicular components of the cell secretome; even the composition of the basal cell media should be considered since varying concentration of cytokines and proteins have been reported among the MSC secretome produced when cultured in different media (88). Currently there are no studies directly comparing the effect of different basal media on the therapeutic effects of the EVs isolated from mesenchymal progenitor cells (89), and this is perhaps surprising.
Most of the in vitro cell culture performed involves supplementation with fetal bovine serum (FBS) as it contains many essential nutrients required for cell growth and survival. However, despite being used for decades, FBS has never been fully characterized, and with this bears several disadvantages when it comes to the use of serum in cell culture for downstream clinical application. From a biosafety concern, FBS may contain adverse factors, like endotoxin, mycoplasma, viral contaminants or prion proteins, that can be transmitted to the patient during cell therapy, causing detrimental immune reactions, rejection of the cell therapy and possible infections (90). Additionally, in the field of EV research, there is a concern as FBS is the source of a large variety of contaminants including exogenous FBS-derived EVs, lipoproteins, and protein complexes. This makes interpretation of results particularly difficult as the final EV isolation will contain a mixture of EVs from the cultured cells and components from the FBS. If FBS is used, the biological effects that are reported in the literature could falsely imply that the EVs, cytokines or growth factors that are eliciting this response are produced by the cell under investigation, when it is the presence of FBS that is playing an important role. This has been highlighted previously when investigating the angiogenic properties of conditioned media from BMSCs. One study demonstrated that conditioned media promoted the proliferation of endothelial cells (91), while a second study did not (81). The difference between these two studies was the presence of FBS; 10% FBS is the first study, and 0.1% FBS in the second study. In this example, the FBS used contained angiogenic factors such as VEGF, and this was, in part, the source of the pro-angiogenic potential of the conditioned media.
EV-depleted FBS can be used as an alternative, however it is important to note that EV-depleted FBS is not EV-free, but EV-reduced (92). The standard way of producing EV-depleted FBS is though high-speed ultracentrifugation, removal of the EV-containing pellet, and subsequently only using the supernatant to supplement media. However, this supernatant is often less than ideal as many growth factors and other proteins are likely to be removed during the ultracentrifugation (93). Studies investigating breast carcinoma cells lines demonstrated that cells were capable of forming 3D colonies in standard FBS media, but could only form monolayers in EV-depleted media (94). Meanwhile, the proliferation of human cardiac progenitors is reduced in EV-depleted FBS (95). Strikingly, both of these studies demonstrated that the addition of the pelleted EVs from the FBS could rescue these changes in migration and proliferation in a dose dependant manor. Investigation of myoblast cell lines from mice (C2C12), rats (L6) and primary human myoblasts found not only were proliferation rates reduced, but genes related to EV formation and EV trafficking were reduced when these cells were cultured using EV-depleted FBS compared to non-EV depleted (96). Conversely, one study investigating human adipose derived MSCs found no difference in proliferation, cell morphology, or osteogenic differentiation capacity, in cells grown in EV-depleted FBS compared to non-EV depleted (97). Nevertheless, the use of EV-depleted FBS has been shown impact cell growth, behavior and phenotype, likely due to removal of FBS-derived EVs and other co-isolated particles (98). Consequently, the cell-derived EVs could have altered characteristics and functionalities. Therefore, the use of FBS, even after EV-depletion, likely results in major consequences and the potential for greater misinterpretations of downstream EV analysis.
A common approach to circumvent the issue of inefficient EV-depletion is to grow cells using serum-free conditions, offering the advantage that the culture preparation is far more simple and defined. Of course, with a complete lack of FBS, the same pitfalls seen with EV-depleted FBS, such as changes in cell physiology and EV release, have been reported with serum free methods (99, 100). Supplementing serum free media with a cocktail of growth factors may be a solution to this problem (92, 101, 102), although the possibility for co-purification of the growth factors interacting with surface components of the EV should be investigated. Unfortunately, additional pitfalls to using serum-free media have been widely reported in the literature. Aggressively switching from normal FBS conditioned to serum free conditions may cause cell stress, which could in turn result in the interference of signaling cascades associated with EV biogenesis (99, 103). Washing cells with PBS prior to the switch to serum free media has been suggested to induce severe modifications in the phenotype of human MSC derived adipocytes compared to a more subtle switch to serum free media over 4 days, without a PBS wash (104). Additionally, washing cells more than three times, reduced the number of viable cells after the wash step (104). Furthermore, serum deprivation may induce cell death, leading to the release of apoptopic bodies or associate death ligands, and hence alter the quantity, quality and protein/cargo composition of in vitro derived EVs (105).
Alternatives to FBS have also been proposed, such as human sera or platelet lysates and various chemically defined serum free media, that can provide the nutrients cells need for in vitro culture (106). Studies found that hBMMSCs retained their morphology, phenotype, viability and differentiation potential when cultured in serum free media containing human platelet lysate. Additionally, these cells were enriched in EVs and the EV-RNA profiles remained unchanged (107). However, platelets can produce their own exogenous EVs, and therefore the ISEV discourages the use of platelet lysates to prevent this contamination, instead opting to promote entirely serum-free methods (86). Importantly, as yet there are no known studies that highlight the effect of these FBS alternatives on the biological properties of the conditioned media, or the effects on the cells and their production of EVs (89). Currently, ISEV recommends strict reporting of the culture media used when collecting EVs, the method of EV-depletion, and any changes in cell physiology. Furthermore, the use of proper unconditioned medium controls in order to assess the amount of exogenous EV contamination is fundamental to establish appropriate background standards (86).
Batch Culture for EV Production
Increasing the yield of EVs isolated is an obvious high priority for their clinical use in EV-based therapies. Several methods of scalable biomanufacturing processes for EV production have been reported in the literature, although it is as yet unknown how these various methods may change the cellular function and therefore production and/or composition of EVs.
Bioreactors have been used for the generation of clinical scale qualities of cells and in recent years this technology has been applied for the large-scale production of EVs. Hollow fiber bioreactors, in which cells are seeded into cylindrical hollow fibers through which media is continuously circulated, result in a high surface area for cell seeding (billions as opposed to millions in a standard culture flask). These bioreactors result in the production of 4-times more EVs compared to a traditional 2D flask (108). However, this continuous flow of media over the cells could results in flow-derived shear stress, which has been postulated to alter EV secretion and intravesicular cargo content (109). Additionally, studies comparing bioreactors to standard 2D flasks have shown EVs generated in these bioreactors have a different size profile (108). It is not yet known whether these changes in EV composition and size could lead to a reduced therapeutic potency, but is something that should be considered when thinking about large scale EV production.
Similarly, some laboratories use multi-layered culture flasks to increase the number of cells producing EVs (110). One caveat of this method is the flasks offer little advantage in terms of cell density; creating high volumes of conditioned media for subsequent processing, and ultimately these approaches have limited scalability. High density culture is therefore an advantage in terms of a tractable solution to vesicle yield. Typically EVs are harvested from cell cultures at 60-90% confluence (100). However, depending on cell types, culturing cells to high confluence can alter their biological properties. Contact inhibition at higher densities can trigger quiescence (111) which may influence their secretion of EVs and other paracrine factors. MSCs grown to high densities show up to 100-fold decrease in EV production compared to MSCs seeded at lower densities, although no change in EV bioactivity was observed (112). Additionally, the characteristics and gene expression profiles of BMSCs change as confluence increases (113). This may be because as cellular proliferation decreases, the secretome of the BMSC can change. There is now a consensus of opinion that down regulation of cell proliferation is pre-requisite for osteoblasts differentiation (114, 115) but high seeding densities can also promote adipocytic differentiation (116, 117). Consequently, the potential to change the differentiation status of a vesicle producing cell would very likely change the composition and subsequent functional potency of the EVs. It is also important to note that components such as ALP are down-regulated when MSCs enter the M phase during the cell cycle (118) which could translate to altered ALP levels in the EV. Therefore, harvesting of EV whilst actively proliferating or upon reaching high confluence should be fully assessed for impact on the EV cargo.
Long term in vitro cell expansion of MSCs has been shown to result in changes to gene expression (119). Yet, given the limited number of MSCs found in bone marrow for example, expansion is required in order to obtain the necessary quantities needed for EV production. Such changes in gene expression have been shown to influence MSC differentiation potential (120). As these cells age in vitro they eventually enter senescence, at which point they begin to lose their plasticity and genetic stability. Furthermore, EV cargo may be altered with cell age, as studies have shown the reparative capacity of MSC-derived EVs decreases with increasing cell passage (112). Hence the drive toward a scalable, high density cell culture system inevitably alters the phenotype of the cell and hence compromises the nature and potency of the EV being produced. Attending to these challenges by finding the optimal balance between cell density and phenotype retention will be important for advancing the EV therapeutic space.
EV Enrichment From Conditioned Media
The purification methods used to extract EVs from conditioned media also has a baring on EV yield. Currently EVs are isolated by a variety of methods such as ultracentrifugation, size exclusion chromatography, precipitation, immunoaffinity and varied forms of filtration. Many EV isolation methods are complex multistep processes, through which there are many stages where EVs can be lost, or damaged, or altered. As a result, this is thought to result in low yields and ultimately may misrepresent the true EV population in conditioned media (121–123). For research laboratories differential ultracentrifugation is often the favored method of EV isolation, probably due to historical reliance on this approach, and its relative simplicity. Pre-clearing requires some initial centrifugation steps to removed live cells (300-400xg for 5-10 mins) and dead cells (2,000xg for 10 mins). The supernatant may then be purified using micro-filtration with pore sizes ranging from 0.1 to 0.8 μm, to eliminate remaining structures in the secretome such as large vesicles, prior to ultracentrifugation at >100,000 xg for 1-3 h (93). The resulting pellet containing the EVs is then resuspended and can be further purified through additional PBS washes. It is worth noting that these additional purification steps will decrease yield due to incomplete sedimentation and aggregation in the pellet (124). Ultracentrifugation is a long and expensive process with limited scalability, and the estimated isolation yield from conditioned media has been reported to be as low as 5-25%, although the accuracy of these numbers remains problematic (125). Additionally, size based isolation via micro-filtration can result in low yield due to large quantities of EVs and contaminants adhering to and clogging the filters (126).
Density gradient ultracentrifugation, in which a viscous medium is used to isolate distinct populations of EVs based on vesicle density, are largely analytical approaches that can provide EV isolates of high purity, as co-isolation of contaminants can be minimized. Conversely, this method is complex, laborious, and expensive, and totally unsuited to up-scaling. Alternatively, aqueous two-phase systems (ATPSs) can be used to separate EVs based on their precipitation with hydrophilic polymers such as polyethylene glycol (PEG) and dextran (DEX). Studies found that EVs biased affinity to DEX-rich bases resulted in a four-times higher recovery rate compared to ultracentrifugation (127). This method of EV isolation is second in its popularity to ultracentrifugation (128) as it is fast and relatively simple, therefore making it ideal for the processing of a large number of samples. EV precipitation is accompanied by co-precipitation of non-EV nucleoproteins and proteins such as albumin and immune complexes (129). These impurities can be removed from the sample with subsequent centrifugation or filtration (130). Therefore, when it comes to choosing a method for EV isolation many factors need to be considered, such as cost, quantity of conditioned media, and purpose.
Influence of Cell Populations Chosen to Isolated EVs
MSCs can be found in all tissues, both in the adult and embryo, and choosing which cells to work with for cell-based therapies has required consideration surrounding ease of isolation, the use of autologous vs. allogenic cell transplants, and the limitations that come with such decisions. Similar decisions will also need to be made when considering the cell source for EV production, as the content will vary depending on the origin and age of the cell sourced.
To address this question, we should consider prior research that recognizes the heterogeneity of MSC populations being investigated for use in cell-based therapies and lessons learnt here. Both BMSCs and DPSCs contain individual subpopulations with different proliferation rates and differentiation capabilities (131–133). Daughter cells destined for differentiation undergo several rapid divisions as transit amplifiers (TA) that become progressively more lineage restricted as they divide (134). An important requirement for regenerative medicine and the exploitation of MSCs is the ability to expand these cells in vitro. It may therefore appear sensible to select highly proliferative cells, but, with extensive cell expansion, comes altered cell behavior and potential for spontaneous differentiation of MSCs down the osteoblast, chondrocytes and neuronal lineages (135–137).
Numerous studies investigating the heterogeneity of DPSCs have revealed potential protocols for the sorting of MSC subpopulations. Of note, DPSCs with greater multipotentiality and high proliferative capacities had longer telomeres, exhibited prolonged purported “stem” cell marker expression, and lacked CD271 expression; whilst other DPSC subpopulations presented with early onset senescence, “stem” cell marker loss, shorter telomeres and positive CD271 expression (138). This demonstrates telomere length and CD271 expression could be used to selectively screen and isolate multipotent DPSC populations with higher proliferative capacity and multipotent differentiation potential. Similarly, using fibronectin adherence to select for more immature MSC cell types has been used for both BMSC and DPSCs (139, 140).
Studies should thus compare the regenerative capacity of the more multipotent cell types with those that are identifiable as lineage restricted uni-potent cells. Indeed, it has been proposed that it is these lineage restricted cells that are possibly the first responders during the repair of tissues such as bone and dentine (141, 142). Conversely, multipotent MSCs may find a broader application for tissue regeneration therapies in general, where they demonstrate greater ability to differentiate into a wider range of tissue types. This question has possibly not yet been fully addressed, but a similar approach should be taken when selecting appropriate cells from which to harvest EVs. One study examining the time-course secretion of exosomes by hMSCs across the entire process of osteogenic differentiation found that only exosomes derived from the late stages of osteogenic differentiation were able to induce ECM mineralization in treated hMSCs (143). Micro-RNA analysis of the exosomes produced during the time course of osteogenic differentiation found higher levels of miRNAs that favored osteogenic differentiation in exosomes derived from the later osteogenic stages. This demonstrates that MSCs secrete exosomes with different biological properties depending on their stage in the differentiation process. Similarly, EVs derived from BMSCs and placental stem cells that had been differentiated for 21 days in osteogenic media (late-stage differentiation) were able to achieve faster differentiation rates compared to those derived from earlier stages (7 days in osteogenic media) (144). The above studies are of consequential value when selecting and expanding cells from which to harvest EVs as one must ensure a homogenous population of cells, the differentiation status of which is known. This is important to ensure reproducible results and the production of EVs with predictable biological properties. Although cell-free therapies mean painful and expensive cell isolation procedures may not be required, this ultimately depends on which cells produce EVs with the necessary cargo, meaning some of the issues related to cell-based therapies could still apply.
Considerations and Challenges in the Therapeutic Application of EVs
To successfully translate EV products into clinical applications it is essential that the bioactivity of the products is reproducible and meets pre-defined quantitative criteria of identity and potency before being used in a clinical capacity. Different manufacturing processes, discussed above, will no doubt generate EV products with qualitative and quantitative differences in identity and potency. The implications surrounding these issues are discussed in detail in the following reviews (145–147). There is currently no consensus on the best way to produce therapeutic EVs, on top of which there is also a lack of standardized quality assurance assays to ensure batch consistency and EV quality. Critically, this has led to discrepancies and controversies surrounding the biology, function, and therapeutic potency of EVs produced from the same cell type. Fingerprinting assays offer a way to establish batch-to-batch consistency by examining a narrowly defined set of molecular markers that are expected to be either present or absent in the EV isolation (148). However, these fingerprint assays are yet to be standardized. The identity of the EVs remains partially unknown, especially the nature of their therapeutically active components. This presents another major barrier to the clinical translation of EVs to therapeutics in defining their potency metrics, which is needed to establish how well a substance elicits a desired therapeutic action. There are several methods to quantify EV doses, and the most common are based on the number of parent cells, EV protein cargo/protein concentration, and EV number and size using specialized nanoparticle tracking analysis. However, dose, frequency, and route of administration varies considerably. It has been reported that EVs have a short in vivo half-life and that their effects could be short-lived, therefore repeat doses are required to maintain a therapeutic effect (149). Moreover, as EVs are so intrinsically complex, with thousands of varying cargos, they have the potential to influence a diverse range of biological activity. With such a broad variety of (heterogenous) cell sources from which to harvest EVs, establishing mode of action is challenging. In fact, EV isolations have been shown to act both directly and indirectly by modulating various cell types (150), therefore the therapeutic targets of these EVs may involve cells or tissues that are beyond the actual site of injury.
Utilizing EVs for Bone Regenerative Medicine
As discussed earlier, EVs participate in the normal mineral formation during bone growth and development (151). With the recent advancements in EV research and their application as an alternative to MSC cell-based therapy for regenerative medicine, many researchers have investigated the potential therapeutic applications of EVs in bone repair and the treatment of various bone diseases.
EVs derived from various mesenchymal progenitor cell sources have been investigated in their capacity to accelerate bone regeneration, both in vitro and in vivo. The authors refer the reader to a comprehensive review by Murali and Holmes (152). Human BMSC (hBMSC) derived EVs are the most frequently used MSC-type in pre-clinical studies for bone regeneration therapies. One study demonstrates hBMSC derived EVs stimulate osteogenic gene expression and differentiation both in vitro and in vivo (54). In vitro, the expression of osteoblast specific genes coding for Runx2, osteocalcin and osteopontin, are upregulated in osteoblasts that receive treatment with BMSC-derived EVs. In vivo, using a hydrogel delivery system, BMSC-derived EVs promote the regeneration of critical sized calvarial defects in rats to a higher degree than those that did not receive EV treatment. BMSC derived EVs have subsequently been shown to accelerate bone regeneration and vascularisation in other murine and rodent models (153–155). Similarly, human iPSC-MSCs derived EVs, when implanted into rat calvaria defect models, show dose-dependent bone regeneration, vascularisation, and expression of osteogenic markers OCN and OPN (71, 156).
Other sources of MSCs have also been explored as a source of osteogenic EVs for fracture repair and bone regeneration. Transplantation of umbilical cord MSC (uMSC) derived EVs into a rat femoral fracture markedly enhanced angiogenesis and bone healing processes (150), increasing local expression of critical fracture healing factors Wnt3a and β-catenin (157). Studies investigating exosomes derived from uMSCs that have been pre-differentiated down the osteogenic lineage in vitro prior to EV harvest, also induce bone regeneration when seeded into rat radial bone defect (158). EVs obtained from adipose tissue-derived MSCs (AD-MSCs) have successfully stimulated osteogenic differentiation in murine mouse models in vivo (159, 160), although recent studies have indicated the regenerative potential of AD-MSC-EVs has similar potency to transplanted AD-MSCs (161). Recent in vitro studies have highlighted the ability of exosomes secreted by hAD-MSCs, combined with PLA-based scaffolds, to stimulate the osteogenic commitment of hAD-MSCs (162).
It should not be forgotten that bone regeneration also requires the stimulation of angiogenesis, alongside the differentiation of osteoblasts. Indeed, microvesicles and exosomes from MSCs have been shown to stimulate blood vessel formation in vivo and in vitro, which in turn could lead to improvements in bone regeneration (163). Studies with hBMSC derived exosomes have elegantly demonstrated that exosomes stimulated MSCs to express both osteogenic and angiogenic genes, and in the presence of an angiogenesis inhibitor, these MSCs lost their ability to form bone in vivo (164, 165). Additionally, perivascular “stem” cells within the walls of blood vessels have been shown to participate in endogenous bone repair (166). EVs derived from human perivascular cells increased bone mineral deposition and the expression of osteogenic factors Runx2 and Osterix in BMSCs cells under osteogenic conditions in vitro. In a calvarial defect mouse model, in which human perivascular-EVs were percutaneously injected twice a week for 4 weeks, mice presented with an increase in osteoblastic proliferation at the edge of the bone defect and an increase in bone deposition compared to mice injected with a PBS control (167).
In an interesting approach, functionally engineered EVs have been generated from human BMSCs that constitutively express BMP2 to produce a cellular source resembling differentiating osteoprogenitor (168). EVs derived from BMP2 over-expressing hBMSCs promote greater bone regeneration in rat calvarial defects in vivo, compared to conventional hMSC-EVs. Of note, EVs from undifferentiated hMSCs demonstrated limited regenerative capacity in vivo, highlighting the importance of selecting appropriate EV sources for clinical application.
Assessing EV Function in the Treatment of Systemic Bone Disease
Osteoporosis is a common age-related bone disease associated with reduced bone mass and impaired bone microarchitecture that increases the risk of fragility fractures, typically in the vertebrae, hip, femoral neck, proximal humerus, and wrist (169). It is estimated there are more than 200 million people worldwide suffering from osteoporosis (170), and with an ever-increasing aging population it is clear osteoporosis will have a huge medical and socioeconomic effect in the future. Since MSC cell-derived EVs have gained attention as novel cell-free therapeutic, the effects of EVs on osteoporosis pathogenesis has been widely investigated in the literature and a selection of these studies are discussed below.
In vivo studies have demonstrated intravenous injection of EVs derived from human umbilical cord blood plasma into the tail vein of senile osteoporotic mice, over the course of 2 months, successfully attenuated bone loss. EV treatment resulted in increased trabecular and cortical bone mass, enhanced osteoblast formation, and reduced osteoclast formation. In vitro studies carried out alongside revealed the pro-osteogenic effect of these EVs was mediated by the shuttling of miR-3960 from the EVs into target cells (171). Numerous studies have shown the involvement of miR-3960 in osteoblast differentiation (172, 173).
Meanwhile, the effects of EVs from adipose tissue-derived “stem” cells (ACS-EVs) in an ovariectomy (OVX)-induced osteoporosis mouse model has also been investigated (174). Intravenous injection of either ASCs or ASC-EVs both resulted in increased bone volume, bone mineral density, trabecular thickness and trabecular number, compared to PBS controls. In vitro studies found EVs produced by ASCs were highly enriched for osteoprogenrin, without which anti-osteoclastogenic effects were not seen. Furthermore, as there was no significant difference between OVX mice treated with ASCs, compared to those treated with the EVs isolated from these MSCs, this further supports the use of EVs as an effective therapeutic alternative to cell-based therapies in the treatment of osteoporosis.
Human urine-derived “stem” cells (UrineSCs) are also gaining traction in the field of EV biology and regenerative medicine as an easy and reproducible source for EVs (UrineSC-EVs). Injection of UrineSC-EVs into osteoporotic mice enhanced osteoblastic differentiation resulting in inhibited bone loss and increased bone strength (175). Importantly, this study compared the anti-osteoporotic effects of human USC-EVs taken from various donors, of different ages, genders and from patients with or without osteoporosis. Not only were the yields of UrineSCs the same across all donors, the anti-osteoporotic effects were not affected by the age, gender or health condition of the donor.
Recent advances in bioengineering have seen the use of “click chemistry” to combine EVs with alendronate, an anti-osteoporotic drug that specifically binds to the surface of bone (176). “Click chemistry” is a term introduced in 2001 to describe organic reactions that produce high yields of molecular products composed of two or more covalently linked molecular components without the production of any byproducts (177). This combined Ale-EV results in a powerful synthetic tool that can target bone specifically, with improved bone targeting capabilities compared to standard MSC derived EVs when injected into the tail vein of an ovariectomy (OVX)-induced osteoporosis rat model. Treatment with Ale-EVs promoted bone regeneration in these rats, protecting them against osteoporosis more effectively than with either aledondronate or MSC derived EVs alone (176). This certainly highlights the advantages of combining EVs with anti-osteoporotic drugs.
Discussion and Concluding Remarks
Within this review we have explored the application of EVs in regenerative medicine as an alternative to cell-based therapies. The research discussed indicates a promising therapeutic potential for EV bioengineering and their application for improving the success and predictability of bone regeneration and osteoporosis therapies. Among the excitement generation for using EVs as therapeutic agents, key considerations for their generation, isolation, characterization and therapeutic delivery are crucial to provide valuable advancement of knowledge that would promote the efficacious translation for clinical benefit (see Table 2).
A brief look at the literature can instantly highlight a lack of consensus concerning EV isolation methods and EV characterization still remains. Current methodologies favored by research laboratories largely involves a series of micro-filtration steps, followed by ultra-centrifugation. However, these methods are entirely unsuited to up-scaling, a requirement which is vital for their success in future clinical application. Large scale batch production of EVs using high density culture systems such as hollow fiber bioreactors offer a plausible solution, however these come with their own caveats. In addition, contamination with avesicular components, be it from the basal cell media, or supplementation with FBS/EV-depleted FBS/FBS-alternatives, still poses a dilemma, as culture systems free from FBS inevitably alter cell physiology. As yet, an effective way to produce, separate and purify EVs in large quantities, without altering phenotype, has not been found. Finding the optimal balance between EV quantity and phenotype retention is an important and challenging prospect that is vital for the advancement of EV therapies.
To translate EV-based therapy into clinics, several hurdles would have to be overcome to guarantee the acquisition of safe therapeutics and the prevention of harmful side effects. Currently, the mechanism by which EVs instigate bone repair and regeneration has not been fully elucidated. To further complicate matters, many cell products are composed of a heterogeneous mixture of cells, and as such identification of the EVs responsible for a proposed biological function remains a major challenge. For future EV-therapeutics to be safe and effective, it is therefore necessary to not only determine a potential cell selection criteria for optimal secretome production, but to also clarify the transport, uptake and metabolic kinetics of the EVs in question. In addition, EV therapeutic dosage remains unclear, and it is not yet known if excessive EV treatment could result in detrimental side effects and tissue damage. Therefore, to apply EV therapy to the clinical setting, these important and challenging questions first need to be addressed. Only then can EVs be used safely in their clinical application.
In conclusion, although challenges and difficulties remain, EVs still show great potential in the regeneration of bone tissue. The research and preclinical studies presented in this review demonstrate the promise that EVs embody and their significance in the future of regenerative medicine and their application to the treatment of bony defects and disease.
Author Contributions
All authors have contributed relevant background research knowledge and expertise during the manuscript preparation, during discussion of findings and formulation of conclusion statements.
Funding
This work was supported by the Dunhill Medical Trust [grant number RPGF1806\43].
Conflict of Interest
The authors declare that the research was conducted in the absence of any commercial or financial relationships that could be construed as a potential conflict of interest.
Publisher's Note
All claims expressed in this article are solely those of the authors and do not necessarily represent those of their affiliated organizations, or those of the publisher, the editors and the reviewers. Any product that may be evaluated in this article, or claim that may be made by its manufacturer, is not guaranteed or endorsed by the publisher.
References
1. Trams EG, Lauter CJ, Salem JN, Heine U. Exfoliation of membrane ecto-enzymes in the form of micro-vesicles. Biochim Biophys Acta Biomembr. (1981) 1:63. doi: 10.1016/0005-2736(81)90512-5
2. Harding C, Stahl PD. Transferrin recycling in reticulocytes: pH and iron are important determinants of ligand binding and processing. Biochem Biophys Res Commun. (1983) 2:650-8. doi: 10.1016/0006-291X(83)91776-X
3. Pan B, Johnstone R. Fate of the transferrin receptor during maturation of sheep reticulocytes in vitro: selective externalization of the receptor. Cell. (1983) 33:967–78. doi: 10.1016/0092-8674(83)90040-5
4. Raposo G, Nijman HW, Stoorvogel W, Liejendekker R, Harding CV, Melief CJ, et al. B lymphocytes secrete antigen-presenting vesicles. J Exp Med. (1996) 3:1161–72. doi: 10.1084/jem.183.3.1161
5. Valadi H, Ekström K, Bossios A, Sjöstrand M, Lee JJ, Lötvall JO. Exosome-mediated transfer of mRNAs and microRNAs is a novel mechanism of genetic exchange between cells. Nat Cell Biol. (2007) 6:654–9. doi: 10.1038/ncb1596
6. Zabeo D, Cvjetkovic A, Lässer C, Schorb M, Lötvall J, Höög JL. Exosomes purified from a single cell type have diverse morphology. J Extracell Vesicles. (2017) 1:1329476. doi: 10.1080/20013078.2017.1329476
7. Yáñez-Mó M, Siljander PRM, Andreu Z, Bedina Zavec A, Borràs FE, Buzas EI, et al. Biological properties of extracellular vesicles and their physiological functions. J Extracell Vesicles. (2015) 1:27066. doi: 10.3402/jev.v4.27066
8. Jong OGD, Balkom BWMV, Schiffelers RM, Bouten CVC, Verhaar MC. Extracellular vesicles: potential roles in regenerative medicine. Front immunol. (2014) 5:608. doi: 10.3389/fimmu.2014.00608
9. Crescitelli R, Lässer C, Szabó TG, Kittel A, Eldh M, Dianzani I, et al. Distinct RNA profiles in subpopulations of extracellular vesicles: apoptotic bodies, microvesicles and exosomes. J Extracell Vesicles. (2013) 2. doi: 10.3402/jev.v2i0.20677
10. Doyle LM, Wang MZ. Overview of extracellular vesicles, their origin, composition, purpose, and methods for exosome isolation and analysis. Cells. (2019) 8:727. doi: 10.3390/cells8070727
11. van Niel G, D'Angelo G, Raposo G. Shedding light on the cell biology of extracellular vesicles. Nat Rev Mol Cell Biol. (2018) 4:213–28. doi: 10.1038/nrm.2017.125
12. Stahl PD, Raposo G. Extracellular vesicles: exosomes and microvesicles, integrators of homeostasis. Physiology. (2019) 3:169–77. doi: 10.1152/physiol.00045.2018
13. Andreu Z, Yáñez-Mó M. Tetraspanins in extracellular vesicle formation and function. Front Immunol. (2014) 5:442. doi: 10.3389/fimmu.2014.00442
14. Pfrieger FW, Vitale N. Cholesterol and the journey of extracellular vesicles. J Lipid Res. (2018) 12:2255–61. doi: 10.1194/jlr.R084210
15. Abdullah M, Nakamura T, Ferdous T, Gao Y, Chen Y, Zou K, et al. Cholesterol regulates exosome release in cultured astrocytes. Front Immunol. (2021) 12:722581. doi: 10.3389/fimmu.2021.722581
16. Escola JM, Kleijmeer MJ, Stoorvogel W, Griffith JM, Yoshie O, Geuze HJ. Selective enrichment of tetraspan proteins on the internal vesicles of multivesicular endosomes and on exosomes secreted by human B-lymphocytes. J Biol Chem. (1998) 32:20121–7. doi: 10.1074/jbc.273.32.20121
17. Del Conde I, Shrimpton CN, Thiagarajan P, López JA. Tissue-factor-bearing microvesicles arise from lipid rafts and fuse with activated platelets to initiate coagulation. Blood. (2005) 5:1604–11. doi: 10.1182/blood-2004-03-1095
18. Ansari S, de Wildt BWM, Vis MAM, de Korte CE, Ito K, Hofmann S, et al. Matrix vesicles: role in bone mineralization and potential use as therapeutics. Pharmaceuticals. (2021) 4:289. doi: 10.3390/ph14040289
19. Davies OG, Cox SC, Azoidis I, McGuinness AJA, Cooke M, Heaney LM, et al. Osteoblast-derived vesicle protein content is temporally regulated during osteogenesis: implications for regenerative therapies. Front Bioeng Biotechnol. (2019) 7:392. doi: 10.3389/fbioe.2019.00392
20. Battistelli M, Falcieri E. Apoptotic bodies: particular extracellular vesicles involved in intercellular communication. Biology. (2020) 1:21. doi: 10.3390/biology9010021
21. Kakarla R, Hur J, Kim YJ, Kim J, Chwae Y-J. Apoptotic cell-derived exosomes: messages from dying cells. Exp Mol Med. (2020) 1:1–6. doi: 10.1038/s12276-019-0362-8
22. Liu H, Liu S, Qiu X, Yang X, Bao L, Pu F, et al. Donor MSCs release apoptotic bodies to improve myocardial infarction via autophagy regulation in recipient cells. Autophagy. (2020) 12:2140–55. doi: 10.1080/15548627.2020.1717128
23. Liu D, Kou X, Chen C, Liu S, Liu Y, Yu W, et al. Circulating apoptotic bodies maintain mesenchymal stem cell homeostasis and ameliorate osteopenia via transferring multiple cellular factors. Cell Res. (2018) 9:918–33. doi: 10.1038/s41422-018-0070-2
24. Ma Q, Liang M, Wu Y, Ding N, Duan L, Yu T, et al. Mature osteoclast-derived apoptotic bodies promote osteogenic differentiation via RANKL-mediated reverse signaling. J Biol Chem. (2019) 29:11240–7. doi: 10.1074/jbc.RA119.007625
25. Yuan FL, Wu QY, Miao ZN, Xu MH, Xu RS, Jiang DL, et al. Osteoclast-derived extracellular vesicles: novel regulators of osteoclastogenesis and osteoclast-osteoblasts communication in bone remodeling. Front Physiol. (2018) 9:628. doi: 10.3389/fphys.2018.00628
26. Patel DM, Shah J, Srivastava AS. Therapeutic potential of mesenchymal stem cells in regenerative medicine. Stem Cells Int. (2013) 2013:496218. doi: 10.1155/2013/496218
27. Takahashi K, Tanabe K, Ohnuki M, Narita M, Ichisaka T, Tomoda K, et al. Induction of pluripotent stem cells from adult human fibroblasts by defined factors. Cell. (2007) 5:861–72. doi: 10.1016/j.cell.2007.11.019
28. Drela K, Stanaszek L, Nowakowski A, Kuczynska Z, Lukomska B. Experimental strategies of mesenchymal stem cell propagation: adverse events and potential risk of functional changes. Stem Cells Int. (2019) 2019:7012692. doi: 10.1155/2019/7012692
29. Russell KC, Phinney DG, Lacey MR, Barrilleaux BL, Meyertholen KE, O'Connor KC. In vitro high-capacity assay to quantify the clonal heterogeneity in trilineage potential of mesenchymal stem cells reveals a complex hierarchy of lineage commitment. Stem Cells. (2010) 4:788–98. doi: 10.1002/stem.312
30. Fox JM, Chamberlain G, Ashton BA, Middleton J. Recent advances into the understanding of mesenchymal stem cell trafficking. Br J Haematol. (2007) 6:491–502. doi: 10.1111/j.1365-2141.2007.06610.x
31. Kim EY, Lee K-B, Yu J, Lee JH, Kim KJ, Han K-W, et al. Neuronal cell differentiation of mesenchymal stem cells originating from canine amniotic fluid. Human Cell. (2014) 2:51–8. doi: 10.1007/s13577-013-0080-9
32. Breitbach M, Bostani T, Roell W, Xia Y, Dewald O, Nygren JM, et al. Potential risks of bone marrow cell transplantation into infarcted hearts. Blood. (2007) 4:1362–9. doi: 10.1182/blood-2006-12-063412
33. Yoon Y-S, Park J-S, Tkebuchava T, Luedeman C, Losordo DW. Unexpected severe calcification after transplantation of bone marrow cells in acute myocardial infarction. Circulation. (2004) 25:3154–7. doi: 10.1161/01.CIR.0000134696.08436.65
34. Klimczak M. Review oncogenesis and induced pluripotency – commonalities of signalling pathways. Contemp Oncol. (2015) 19:16–21. doi: 10.5114/wo.2014.47133
35. Wuputra K, Ku C-C, Wu D-C, Lin Y-C, Saito S, Yokoyama KK. Prevention of tumor risk associated with the reprogramming of human pluripotent stem cells. J Exp Clin Cancer Res. (2020) 39:100. doi: 10.1186/s13046-020-01584-0
36. Sun Y-Q, Deng M-X, He J, Zeng Q-X, Wen W, Wong DSH, et al. Human pluripotent stem cell-derived mesenchymal stem cells prevent allergic airway inflammation in mice. Stem Cells. (2012) 12:2692–9. doi: 10.1002/stem.1241
37. Hynes K, Bright R, Marino V, Ng J, Verma PJ, Gronthos S, et al. Potential of iPSC-derived mesenchymal stromal cells for treating periodontal disease. Stem Cells Int. (2018) 2018:2601945. doi: 10.1155/2018/2601945
38. Wernig M, Zhao JP, Pruszak J, Hedlund E, Fu D, Soldner F, et al. Neurons derived from reprogrammed fibroblasts functionally integrate into the fetal brain and improve symptoms of rats with Parkinson's disease. PNAS. (2008) 15:5856–61. doi: 10.1073/pnas.0801677105
39. Ide C, Nakai Y, Nakano N, Seo TB, Yamada Y, Endo K, et al. Bone marrow stromal cell transplantation for treatment of sub-acute spinal cord injury in the rat. Brain Res. (2010) 1332:32–47. doi: 10.1016/j.brainres.2010.03.043
40. Smith EJ, Stroemer RP, Gorenkova N, Nakajima M, Crum WR, Tang E, et al. Implantation site and lesion topology determine efficacy of a human neural stem cell line in a rat model of chronic stroke. Stem Cells. (2012) 4:785–96. doi: 10.1002/stem.1024
41. Eggenhofer E, Benseler V, Kroemer A, Popp F, Geissler E, Schlitt H, et al. Mesenchymal stem cells are short-lived and do not migrate beyond the lungs after intravenous infusion. Front immunol. (2012) 3:297. doi: 10.3389/fimmu.2012.00297
42. Boyette LB, Tuan RS. Adult stem cells and diseases of aging. J Clin Med. (2014) 1:88–134. doi: 10.3390/jcm3010088
43. Zakrzewski W, Dobrzyński M, Szymonowicz M, Rybak Z. Stem cells: past, present, and future. Stem Cell Res Ther. (2019) 8:135. doi: 10.1186/s13287-019-1165-5
44. Davies LC, LeBlanc K. Research advances in tissue regeneration by dental pulp stem cells. In: Waddington RJ, Sloan AJ, editors. Tissue Engineering and Regeneration in Dentistry: Current Strategies. Chichester; West Sussex: Wiley Blackwell (2016). p. 20–49.
45. Le Blanc K, Davies LC. MSCs-cells with many sides. Cytotherapy. (2018) 3:273–8. doi: 10.1016/j.jcyt.2018.01.009
46. Kordelas L, Rebmann V, Ludwig AK, Radtke S, Ruesing J, Doeppner TR, et al. MSC-derived exosomes: a novel tool to treat therapy-refractory graft-versus-host disease. Leukemia. (2014) 4:970–3. doi: 10.1038/leu.2014.41
47. Anderson HC. Vesicles associated with calcification in the matrix of epiphyseal cartilage. J Cell Biol. (1969) 1:59–72. doi: 10.1083/jcb.41.1.59
48. Liu M, Sun Y, Zhang Q. Emerging role of extracellular vesicles in bone remodeling. J Dent Res. (2018) 97:859–68. doi: 10.1177/0022034518764411
49. Zhang X, Wang W, Wang Y, Zhao H, Han X, Zhao T, et al. Extracellular vesicle-encapsulated miR-29b-3p released from bone marrow-derived mesenchymal stem cells underpins osteogenic differentiation. Front Cell Dev Biol. (2021) 8:1404. doi: 10.3389/fcell.2020.581545
50. Liu H, Sun Q, Wan C, Li L, Zhang L, Chen Z. MicroRNA-338-3p regulates osteogenic differentiation of mouse bone marrow stromal stem cells by targeting Runx2 and Fgfr2. J Cell Physiol. (2014) 10:1494–502. doi: 10.1002/jcp.24591
51. Lin C, Yu S, Jin R, Xiao Y, Pan M, Pei F, et al. Circulating miR-338 Cluster activities on osteoblast differentiation: potential diagnostic and therapeutic targets for postmenopausal osteoporosis. Theranostics. (2019) 13:3780–97. doi: 10.7150/thno.34493
52. Xie Y, Chen Y, Zhang L, Ge W, Tang P. The roles of bone-derived exosomes and exosomal microRNAs in regulating bone remodelling. J Cell Mol Med. (2017) 5:1033–41. doi: 10.1111/jcmm.13039
53. Xu J-F, Yang G-h, Pan X-H, Zhang S-J, Zhao C, Qiu B-S, et al. Altered microRNA expression profile in exosomes during osteogenic differentiation of human bone marrow-derived mesenchymal stem cells. PLoS ONE. (2014) 12:e114627. doi: 10.1371/journal.pone.0114627
54. Qin Y, Wang L, Gao Z, Chen G, Zhang C. Bone marrow stromal/stem cell-derived extracellular vesicles regulate osteoblast activity and differentiation in vitro and promote bone regeneration in vivo. Sci Rep. (2016) 1:21961. doi: 10.1038/srep21961
55. Luo ZW, Li FX, Liu YW, Rao SS, Yin H, Huang J, et al. Aptamer-functionalized exosomes from bone marrow stromal cells target bone to promote bone regeneration. Nanoscale. (2019) 43:20884–92. doi: 10.1039/C9NR02791B
56. Sato M, Suzuki T, Kawano M, Tamura M. Circulating osteocyte-derived exosomes contain miRNAs which are enriched in exosomes from MLO-Y4 cells. Biomed Rep. (2017) 2:223–31. doi: 10.3892/br.2016.824
57. Qin Y, Peng Y, Zhao W, Pan J, Ksiezak-Reding H, Cardozo C, et al. Myostatin inhibits osteoblastic differentiation by suppressing osteocyte-derived exosomal microRNA-218: a novel mechanism in muscle-bone communication. J Biol Chem. (2017) 26:11021–33. doi: 10.1074/jbc.M116.770941
58. Chen C, Wang D, Moshaverinia A, Liu D, Kou X, Yu W, et al. Mesenchymal stem cell transplantation in tight-skin mice identifies miR-151-5p as a therapeutic target for systemic sclerosis. Cell Res. (2017) 4:559–77. doi: 10.1038/cr.2017.11
59. Fulzele S, Mendhe B, Khayrullin A, Johnson M, Kaiser H, Liu Y, et al. Muscle-derived miR-34a increases with age in circulating extracellular vesicles and induces senescence of bone marrow stem cells. Aging. (2019) 6:1791–803. doi: 10.18632/aging.101874
60. Li D, Liu J, Guo B, Liang C, Dang L, Lu C, et al. Osteoclast-derived exosomal miR-214-3p inhibits osteoblastic bone formation. Nat Commun. (2016) 1:10872. doi: 10.1038/ncomms10872
61. Martin PJ, Haren N, Ghali O, Clabaut A, Chauveau C, Hardouin P, et al. Adipogenic RNAs are transferred in osteoblasts via bone marrow adipocytes-derived extracellular vesicles (EVs). BMC Cell Biol. (2015) 1:10. doi: 10.1186/s12860-015-0057-5
62. Davis C, Dukes A, Drewry M, Helwa I, Johnson MH, Isales CM, et al. MicroRNA-183-5p Increases with age in bone-derived extracellular vesicles, suppresses bone marrow stromal (stem) cell proliferation, and induces stem cell senescence. Tissue Eng Part A. (2017) 21-22:1231–40. doi: 10.1089/ten.tea.2016.0525
63. Baglio SR, Rooijers K, Koppers-Lalic D, Verweij FJ, Pérez Lanzón M, Zini N, et al. Human bone marrow- and adipose-mesenchymal stem cells secrete exosomes enriched in distinctive miRNA and tRNA species. Stem Cell Res Ther. (2015) 6:127. doi: 10.1186/s13287-015-0116-z
64. Liu Y, Zou R, Wang Z, Wen C, Zhang F, Lin F. Exosomal KLF3-AS1 from hMSCs promoted cartilage repair and chondrocyte proliferation in osteoarthritis. Biochem J. (2018) 22:3629–38. doi: 10.1042/BCJ20180675
65. Cui Y, Fu S, Sun D, Xing J, Hou T, Wu X. EPC-derived exosomes promote osteoclastogenesis through LncRNA-MALAT1. J Cell Mol Med. (2019) 6:3843–54. doi: 10.1111/jcmm.14228
66. Zhang Y, Chen B, Li D, Zhou X, Chen Z. LncRNA NEAT1/miR-29b-3p/BMP1 axis promotes osteogenic differentiation in human bone marrow-derived mesenchymal stem cells. Pathol Res Pract. (2019) 3:525–31. doi: 10.1016/j.prp.2018.12.034
67. Huynh N, VonMoss L, Smith D, Rahman I, Felemban MF, Zuo J, et al. Characterization of regulatory extracellular vesicles from osteoclasts. J Dent Res. (2016) 6:673–9. doi: 10.1177/0022034516633189
68. Nahar NN, Missana LR, Garimella R, Tague SE, Anderson HC. Matrix vesicles are carriers of bone morphogenetic proteins (BMPs), vascular endothelial growth factor (VEGF), and noncollagenous matrix proteins. J Bone Miner Metab. (2008) 5:514–9. doi: 10.1007/s00774-008-0859-z
69. Xiao Z, Camalier CE, Nagashima K, Chan KC, Lucas DA, Cruz MJDL, et al. Analysis of the extracellular matrix vesicle proteome in mineralizing osteoblasts. J Cell Physiol. (2007) 2:325–35. doi: 10.1002/jcp.20826
70. Ramachandran A, Ravindran S, Huang C-C, George A. TGF beta receptor II interacting protein-1, an intracellular protein has an extracellular role as a modulator of matrix mineralization. Sci Rep. (2016) 1:37885. doi: 10.1038/srep37885
71. Zhang J, Liu X, Li H, Chen C, Hu B, Niu X, et al. Exosomes/tricalcium phosphate combination scaffolds can enhance bone regeneration by activating the PI3K/Akt signaling pathway. Stem Cell Res Ther. (2016) 1:136. doi: 10.1186/s13287-016-0391-3
72. Otsuru S, Desbourdes L, Guess AJ, Hofmann TJ, Relation T, Kaito T, et al. Extracellular vesicles released from mesenchymal stromal cells stimulate bone growth in osteogenesis imperfecta. Cytotherapy. (2018) 1:62–73. doi: 10.1016/j.jcyt.2017.09.012
73. Lanzillotti C, De Mattei M, Mazziotta C, Taraballi F, Rotondo JC, Tognon M, et al. Long non-coding RNAs and MicroRNAs interplay in osteogenic differentiation of mesenchymal stem cells. Front Cell Dev Biol. (2021) 9:646032. doi: 10.3389/fcell.2021.646032
74. Sun W, Zhao C, Li Y, Wang L, Nie G, Peng J, et al. Osteoclast-derived microRNA-containing exosomes selectively inhibit osteoblast activity. Cell Discov. (2016) 1:16015. doi: 10.1038/celldisc.2016.15
75. Silva AM, Almeida MI, Teixeira JH, Maia AF, Calin GA, Barbosa MA, et al. Dendritic cell-derived extracellular vesicles mediate mesenchymal stem/stromal cell recruitment. Sci Rep. (2017) 1:1667. doi: 10.1038/s41598-017-01809-x
76. Ekström K, Omar O, Granéli C, Wang X, Vazirisani F, Thomsen P. Monocyte exosomes stimulate the osteogenic gene expression of mesenchymal stem cells. PLoS ONE. (2013) 9:e75227. doi: 10.1371/journal.pone.0075227
77. Teixeira FG, Carvalho MM, Sousa N, Salgado AJ. Mesenchymal stem cells secretome: a new paradigm for central nervous system regeneration? Cell Mol Life Sci. (2013) 20:3871–82. doi: 10.1007/s00018-013-1290-8
78. Chen L, Tredget EE, Wu PYG, Wu Y. Paracrine factors of mesenchymal stem cells recruit macrophages and endothelial lineage cells and enhance wound healing. PLoS ONE. (2008) 4:e1886. doi: 10.1371/journal.pone.0001886
79. Gnecchi M, He H, Liang OD, Melo LG, Morello F, Mu H, et al. Paracrine action accounts for marked protection of ischemic heart by Akt-modified mesenchymal stem cells. Nat Med. (2005) 4:367–8. doi: 10.1038/nm0405-367
80. Meyerrose T, Olson S, Pontow S, Kalomoiris S, Jung Y, Annett G, et al. Mesenchymal stem cells for the sustained in vivo delivery of bioactive factors. Adv Drug Deliv Rev. (2010) 12:1167–74. doi: 10.1016/j.addr.2010.09.013
81. Bronckaers A, Hilkens P, Fanton Y, Struys T, Gervois P, Politis C, et al. Angiogenic properties of human dental pulp stem cells. PLoS ONE. (2013) 8:e71104. doi: 10.1371/journal.pone.0071104
82. Osugi M, Katagiri W, Yoshimi R, Inukai T, Hibi H, Ueda M. Conditioned media from mesenchymal stem cells enhanced bone regeneration in rat calvarial bone defects. Tissue Eng Part A. (2012) 13-14:1479–89. doi: 10.1089/ten.tea.2011.0325
83. Katagiri W, Kawai T, Osugi M, Sugimura-Wakayama Y, Sakaguchi K, Kojima T, et al. Angiogenesis in newly regenerated bone by secretomes of human mesenchymal stem cells. Maxillofac Plast Reconstr Surg. (2017) 1:8. doi: 10.1186/s40902-017-0106-4
84. Théry C, Ostrowski M, Segura E. Membrane vesicles as conveyors of immune responses. Nat Rev Immunol. (2009) 8:581–93. doi: 10.1038/nri2567
85. Herrmann IK, Wood MJA, Fuhrmann G. Extracellular vesicles as a next-generation drug delivery platform. Nat Nanotechnol. (2021) 7:748–59. doi: 10.1038/s41565-021-00931-2
86. Théry C, Witwer KW, Aikawa E, Alcaraz MJ, Anderson JD, Andriantsitohaina R, et al. Minimal information for studies of extracellular vesicles 2018 (MISEV2018) : a position statement of the International Society for Extracellular Vesicles and update of the MISEV2014 guidelines. J Extracell Vesicles. (2018) 1:1535750. doi: 10.1080/20013078.2018.1535750
87. Lener T, Gimona M, Aigner L, Börger V, Buzas E, Camussi G, et al. Applying extracellular vesicles based therapeutics in clinical trials - an ISEV position paper. J Extracell Vesicles. (2015) 4:30087. doi: 10.3402/jev.v4.30087
88. Sagaradze G, Grigorieva O, Nimiritsky P, Basalova N, Kalinina N, Akopyan Z, et al. Conditioned medium from human mesenchymal stromal cells: towards the clinical translation. Int J Mol Sci. (2019) 7:1656. doi: 10.3390/ijms20071656
89. Gwam C, Mohammed N, Ma X. Stem cell secretome, regeneration, and clinical translation: a narrative review. Ann Transl Med. (2021) 1:70. doi: 10.21037/atm-20-5030
90. Gstraunthaler G, Lindl T, van der Valk J. A plea to reduce or replace fetal bovine serum in cell culture media. Cytotechnology. (2013) 5:791–3. doi: 10.1007/s10616-013-9633-8
91. Gruber R, Kandler B, Holzmann P, Vogele-Kadletz M, Losert U, Fischer MB, et al. Bone marrow stromal cells can provide a local environment that favors migration and formation of tubular structures of endothelial cells. Tissue Eng. (2005) 5-6:896–903. doi: 10.1089/ten.2005.11.896
92. Lehrich BM, Liang Y, Fiandaca MS. Foetal bovine serum influence on in vitro extracellular vesicle analyses. J Extracell Vesicles. (2021) 10:e12061. doi: 10.1002/jev2.12061
93. Théry C, Amigorena S, Raposo G, Clayton A. Isolation and characterization of exosomes from cell culture supernatants and biological fluids. Curr Protoc Cell Biol. (2006) 1:3. doi: 10.1002/0471143030.cb0322s30
94. Ochieng J, Pratap S, Khatua AK, Sakwe AM. Anchorage-independent growth of breast carcinoma cells is mediated by serum exosomes. Exp Cell Res. (2009) 11:1875–88. doi: 10.1016/j.yexcr.2009.03.010
95. Angelini F, Ionta V, Rossi F, Miraldi F, Messina E, Giacomello A. Foetal bovine serum-derived exosomes affect yield and phenotype of human cardiac progenitor cell culture. BioImpacts. (2016) 1:15–24. doi: 10.15171/bi.2016.03
96. Aswad H, Jalabert A, Rome S. Depleting extracellular vesicles from fetal bovine serum alters proliferation and differentiation of skeletal muscle cells in vitro. BMC Biotechnol. (2016) 16. doi: 10.1186/s12896-016-0262-0
97. Kornilov R, Puhka M, Mannerström B, Hiidenmaa H, Peltoniemi H, Siljander P, et al. Efficient ultrafiltration-based protocol to deplete extracellular vesicles from fetal bovine serum. J Extracell Vesicles. (2018) 1:1422674. doi: 10.1080/20013078.2017.1422674
98. Eitan E, Zhang S, Witwer KW, Mattson MP. Extracellular vesicle–depleted fetal bovine and human sera have reduced capacity to support cell growth. J Extracell Vesicles. (2015) 1:26373. doi: 10.3402/jev.v4.26373
99. Li J, Lee Y, Johansson HJ, Mäger I, Vader P, Nordin JZ, et al. Serum-free culture alters the quantity and protein composition of neuroblastoma-derived extracellular vesicles. J Extracell Vesicles. (2015) 4:26883. doi: 10.3402/jev.v4.26883
100. Gudbergsson JM, Johnsen KB, Skov MN, Duroux M. Systematic review of factors influencing extracellular vesicle yield from cell cultures. Cytotechnology. (2016) 4:579–92. doi: 10.1007/s10616-015-9913-6
101. Lehrich B, Liang Y, Khosravi P, Federoff H, Fiandaca M. Fetal bovine serum-derived extracellular vesicles persist within vesicle-depleted culture media. Int J Mol Sci. (2018) 11:3538. doi: 10.3390/ijms19113538
102. Lehrich BM, Liang Y, Fiandaca MS. Response to “Technical approaches to reduce interference of Fetal calf serum derived RNA in the analysis of extracellular vesicle RNA from cultured cells”. J Extracell Vesicles. (2019) 1:1599681. doi: 10.1080/20013078.2019.1599681
103. Wang H, Jiang D, Li W, Xiang X, Zhao J, Yu B, et al. Evaluation of serum extracellular vesicles as noninvasive diagnostic markers of glioma. Theranostics. (2019) 18:5347–58. doi: 10.7150/thno.33114
104. Clabaut A, Grare C, Léger T, Hardouin P, Broux O. Variations of secretome profiles according to conditioned medium preparation: the example of human mesenchymal stem cell-derived adipocytes. Electrophoresis. (2015) 20:2587–93. doi: 10.1002/elps.201500086
105. Sun L, Wang H-X, Zhu X-J, Wu P-H, Chen W-Q, Zou P, et al. Serum deprivation elevates the levels of microvesicles with different size distributions and selectively enriched proteins in human myeloma cells in vitro. Acta Pharmacologica Sinica. (2014) 3:381–93. doi: 10.1038/aps.2013.166
106. Rauch C, Feifel E, Amann EM, Spötl HP, Schennach H, Pfaller W, et al. Alternatives to the use of fetal bovine serum: human platelet lysates as a serum substitute in cell culture media. Altex. (2011) 4:305–16. doi: 10.14573/altex.2011.4.305
107. Pachler K, Lener T, Streif D, Dunai ZA, Desgeorges A, Feichtner M, et al. A Good manufacturing practice–grade standard protocol for exclusively human mesenchymal stromal cell–derived extracellular vesicles. Cytotherapy. (2017) 4:458–72. doi: 10.1016/j.jcyt.2017.01.001
108. Watson DC, Bayik D, Srivatsan A, Bergamaschi C, Valentin A, Niu G, et al. Efficient production and enhanced tumor delivery of engineered extracellular vesicles. Biomaterials. (2016) 105:195–205. doi: 10.1016/j.biomaterials.2016.07.003
109. Patel DB, Santoro M, Born LJ, Fisher JP, Jay SM. Towards rationally designed biomanufacturing of therapeutic extracellular vesicles: impact of the bioproduction microenvironment. Biotechnol Adv. (2018) 8:2051–9. doi: 10.1016/j.biotechadv.2018.09.001
110. Geeurickx E, Lippens L, Rappu P, De Geest BG, De Wever O, Hendrix A. Recombinant extracellular vesicles as biological reference material for method development, data normalization and assessment of (pre-) analytical variables. Nat Protoc. (2021) 2:603–33. doi: 10.1038/s41596-020-00446-5
111. Lieberman MA, Glaser L. Density-dependent regulation of cell growth: an example of a cell-cell recognition phenomenon. J Membr Biol. (1981) 1-2:1–11. doi: 10.1007/BF01969440
112. Patel DB, Gray KM, Santharam Y, Lamichhane TN, Stroka KM, Jay SM. Impact of cell culture parameters on production and vascularization bioactivity of mesenchymal stem cell-derived extracellular vesicles. Bioeng Transl Med. (2017) 2:170–9. doi: 10.1002/btm2.10065
113. Ren J, Wang H, Tran K, Civini S, Jin P, Castiello L, et al. Human bone marrow stromal cell confluence: effects on cell characteristics and methods of assessment. Cytotherapy. (2015) 7:897–911. doi: 10.1016/j.jcyt.2015.03.607
114. Ruijtenberg S, van den Heuvel S. Coordinating cell proliferation and differentiation: antagonism between cell cycle regulators and cell type-specific gene expression. Cell Cycle. (2016) 2:196–212. doi: 10.1080/15384101.2015.1120925
115. Abo-Aziza Zaki. The impact of confluence on bone marrow mesenchymal stem (BMMSC) proliferation and osteogenic differentiation. Int J Hematol Oncol Stem Cell Res. (2017) 2:121–32.
116. Bitar M, Brown RA, Salih V, Kidane AG, Knowles JC, Nazhat SN. Effect of cell density on osteoblastic differentiation and matrix degradation of biomimetic dense collagen scaffolds. Biomacromolecules. (2008) 1:129–35. doi: 10.1021/bm701112w
117. Xue R, Li JY-S, Yeh Y, Yang L, Chien S. Effects of matrix elasticity and cell density on human mesenchymal stem cells differentiation. J Orthop Res. (2013) 9:1360–5. doi: 10.1002/jor.22374
118. Fedarko NS, Bianco P, Vetter U, Robey PG. Human bone cell enzyme expression and cellular heterogeneity: correlation of alkaline phosphatase enzyme activity with cell cycle. J Cell Physiol. (1990) 1:115–21. doi: 10.1002/jcp.1041440115
119. Izadpanah R, Kaushal D, Kriedt C, Tsien F, Patel B, Dufour J, et al. Long-term in vitro expansion alters the biology of adult mesenchymal stem cells. Cancer Res. (2008) 11:4229–38. doi: 10.1158/0008-5472.CAN-07-5272
120. Gu Y, Li T, Ding Y, Sun L, Tu T, Zhu W, et al. Changes in mesenchymal stem cells following long-term culture in vitro. Mol Med Rep. (2016) 6:5207–15. doi: 10.3892/mmr.2016.5169
121. Gardiner C, Vizio DD, Sahoo S, Théry C, Witwer KW, Wauben M, et al. Techniques used for the isolation and characterization of extracellular vesicles: results of a worldwide survey. J Extracell Vesicles. (2016) 1:32945. doi: 10.3402/jev.v5.32945
122. Brennan K, Martin K, FitzGerald SP, O'Sullivan J, Wu Y, Blanco A, et al. A comparison of methods for the isolation and separation of extracellular vesicles from protein and lipid particles in human serum. Sci Rep. (2020) 1:1039. doi: 10.1038/s41598-020-57497-7
123. Carnino JM, Lee H, Jin Y. Isolation and characterization of extracellular vesicles from Broncho-alveolar lavage fluid: a review and comparison of different methods. Respir Res. (2019) 1:240. doi: 10.1186/s12931-019-1210-z
124. Webber J, Clayton A. How pure are your vesicles? J Extracell Vesicles. (2013) 1:19861. doi: 10.3402/jev.v2i0.19861
125. Lamparski HG, Metha-Damani A, Yao JY, Patel S, Hsu DH, Ruegg C, et al. Production and characterization of clinical grade exosomes derived from dendritic cells. J Immunol Methods. (2002) 2:211–26. doi: 10.1016/S0022-1759(02)00330-7
126. Lucchetti D, Fattorossi A, Sgambato A. Extracellular vesicles in oncology: progress and pitfalls in the methods of isolation and analysis. Biotechnol J. (2019) 1:e1700716. doi: 10.1002/biot.201700716
127. Shin H, Han C, Labuz JM, Kim J, Kim J, Cho S, et al. High-yield isolation of extracellular vesicles using aqueous two-phase system. Sci Rep. (2015) 1:13103. doi: 10.1038/srep13103
128. Konoshenko MY, Lekchnov EA, Vlassov AV, Laktionov PP. Isolation of extracellular vesicles: general methodologies and latest trends. BioMed Research International. (2018) 2018:1–27. doi: 10.1155/2018/8545347
129. Van Deun J, Mestdagh P, Sormunen R, Cocquyt V, Vermaelen K, Vandesompele J, et al. The impact of disparate isolation methods for extracellular vesicles on downstream RNA profiling. J Extracell Vesicles. (2014) 1:24858. doi: 10.3402/jev.v3.24858
130. Taylor D, Shah S. Methods of isolating extracellular vesicles impact down-stream analyses of their cargoes. Methods. (2015) 87:3–10. doi: 10.1016/j.ymeth.2015.02.019
131. Alraies A, Waddington RJ, Sloan AJ, Moseley R. Evaluation of dental pulp stem cell heterogeneity and behaviour in 3D Type I collagen gels. BioMed Research International. (2020) 2020:1–12. doi: 10.1155/2020/3034727
132. Sloan AJ, Waddington RJ. Dental pulp stem cells: what, where, how? Int J Paediatr Dent. (2009) 1:61–70. doi: 10.1111/j.1365-263X.2008.00964.x
133. Gronthos S, Brahim J, Li W, Fisher LW, Cherman N, Boyde A, et al. Stem cell properties of human dental pulp stem cells. J Dent Res. (2002) 8:531–5. doi: 10.1177/154405910208100806
134. Waddington RJ, Sloan AJ. Research advances in tissue regeneration by dental pulp stem cells. In: Waddington RJ, Sloan AJ, editor. Tissue Engineering and Regeneration in Dentistry: Current Strategies. Chichester: Wiley Blackwell (2016) p. 50–68.
135. Li Z, Liu C, Xie Z, Song P, Zhao RCH, Guo L, et al. Epigenetic dysregulation in mesenchymal stem cell aging and spontaneous differentiation. PLoS ONE. (2011) 6:e20526. doi: 10.1371/journal.pone.0020526
136. Sonomoto K, Yamaoka K, Kaneko H, Yamagata K, Sakata K, Zhang X, et al. Spontaneous differentiation of human mesenchymal stem cells on poly-lactic-co-glycolic acid nano-fiber scaffold. PLoS ONE. (2016) 4:e0153231. doi: 10.1371/journal.pone.0153231
137. Tseng PY, Chen CJ, Sheu CC, Yu CW, Huang YS. Spontaneous differentiation of adult rat marrow stromal cells in a long-term culture. J Vet Med Sci. (2007) 2:95–102. doi: 10.1292/jvms.69.95
138. Alraies A, Alaidaroos NY, Waddington RJ, Moseley R, Sloan AJ. Variation in human dental pulp stem cell ageing profiles reflect contrasting proliferative and regenerative capabilities. BMC Cell Biol. (2017) 1:12. doi: 10.1186/s12860-017-0128-x
139. Lee CP, Colombo JS, Ayre WN, Sloan AJ, Waddington RJ. Elucidating the cellular actions of demineralised dentine matrix extract on a clonal dental pulp stem cell population in orchestrating dental tissue repair. J Tissue Eng. (2015) 0:204173141558631. doi: 10.1177/2041731415586318
140. Avery S, Sadaghiani L, Sloan A, Waddington R. Analysing the bioactive makeup of demineralised dentine matrix on bone marrow mesenchymal stem cells for enhanced bone repair. Eur Cells Mater. (2017) 34:1–14. doi: 10.22203/eCM.v034a01
141. Téclès O, Laurent P, Zygouritsas S, Burger AS, Camps J, Dejou J, et al. Activation of human dental pulp progenitor/stem cells in response to odontoblast injury. Arch Oral Biol. (2005) 2:103–8. doi: 10.1016/j.archoralbio.2004.11.009
142. Yusop N, Battersby P, Alraies A, Sloan AJ, Moseley R, Waddington RJ. Isolation and characterisation of mesenchymal stem cells from rat bone marrow and the endosteal niche: a comparative study. Stem Cells Int. (2018) 2018:6869128. doi: 10.1155/2018/6869128
143. Wang X, Omar O, Vazirisani F, Thomsen P, Ekström K. Mesenchymal stem cell-derived exosomes have altered microRNA profiles and induce osteogenic differentiation depending on the stage of differentiation. PLoS ONE. (2018) 2:e0193059. doi: 10.1371/journal.pone.0193059
144. Pishavar E, Copus JS, Atala A, Lee SJ. Comparison study of stem cell-derived extracellular vesicles for enhanced osteogenic differentiation. Tissue Eng Part A. (2020) 15-16:1044–54. doi: 10.1089/ten.tea.2020.0194
145. Gimona M, Brizzi MF, Choo ABH, Dominici M, Davidson SM, Grillari J, et al. Critical considerations for the development of potency tests for therapeutic applications of mesenchymal stromal cell-derived small extracellular vesicles. Cytotherapy. (2021) 5:373–80. doi: 10.1016/j.jcyt.2021.01.001
146. Witwer KW, Van Balkom BWM, Bruno S, Choo A, Dominici M, Gimona M, et al. Defining mesenchymal stromal cell (MSC) -derived small extracellular vesicles for therapeutic applications. J Extracell Vesicles. (2019) 1:1609206. doi: 10.1080/20013078.2019.1609206
147. Reiner AT, Witwer KW, van Balkom BWM, de Beer J, Brodie C, Corteling RL, et al. Concise review: developing best-practice models for the therapeutic use of extracellular vesicles. Stem Cells Transl Med. (2017) 8:1730–9. doi: 10.1002/sctm.17-0055
148. Paolini L, Federici S, Consoli G, Arceri D, Radeghieri A, Alessandri I, et al. Fourier-transform Infrared (FT-IR) spectroscopy fingerprints subpopulations of extracellular vesicles of different sizes and cellular origin. J Extracell Vesicles. (2020) 1:1741174. doi: 10.1080/20013078.2020.1741174
149. Takahashi Y, Nishikawa M, Shinotsuka H, Matsui Y, Ohara S, Imai T, et al. Visualization and in vivo tracking of the exosomes of murine melanoma B16-BL6 cells in mice after intravenous injection. J Biotechnol. (2013) 2:77–84. doi: 10.1016/j.jbiotec.2013.03.013
150. Zhang Y, Hao Z, Wang P, Xia Y, Wu J, Xia D, et al. Exosomes from human umbilical cord mesenchymal stem cells enhance fracture healing through HIF-1α-mediated promotion of angiogenesis in a rat model of stabilized fracture. Cell Prolif. (2019) 2:e12570. doi: 10.1111/cpr.12570
151. Golub EE. Biomineralization and matrix vesicles in biology and pathology. Semin Immunopathol. (2011) 5:409–17. doi: 10.1007/s00281-010-0230-z
152. Murali VP, Holmes CA. Mesenchymal stromal cell-derived extracellular vesicles for bone regeneration therapy. Bone Rep. (2021) 14:101093. doi: 10.1016/j.bonr.2021.101093
153. Furuta T, Miyaki S, Ishitobi H, Ogura T, Kato Y, Kamei N, et al. Mesenchymal stem cell-derived exosomes promote fracture healing in a mouse model. Stem Cells Transl Med. (2016) 12:1620–30. doi: 10.5966/sctm.2015-0285
154. Zhang L, Jiao G, Ren S, Zhang X, Li C, Wu W, et al. Exosomes from bone marrow mesenchymal stem cells enhance fracture healing through the promotion of osteogenesis and angiogenesis in a rat model of nonunion. Stem Cell Res Ther. (2020) 1:38. doi: 10.1186/s13287-020-1562-9
155. Xie H, Wang Z, Zhang L, Lei Q, Zhao A, Wang H, et al. Extracellular vesicle-functionalized decalcified bone matrix scaffolds with enhanced pro-angiogenic and pro-bone regeneration activities. Sci Rep. (2017) 1:45622. doi: 10.1038/srep45622
156. Liu X, Li Q, Niu X, Hu B, Chen S, Song W, et al. Exosomes secreted from human-induced pluripotent stem cell-derived mesenchymal stem cells prevent osteonecrosis of the femoral head by promoting angiogenesis. Int J Biol Sci. (2017) 2:232–44. doi: 10.7150/ijbs.16951
157. Zhou J, Liu HX, Li SH, Gong YS, Zhou MW, Zhang JH, et al. Effects of human umbilical cord mesenchymal stem cells-derived exosomes on fracture healing in rats through the Wnt signaling pathway. Eur Rev Med Pharmacol Sci. (2019) 11:4954–60. doi: 10.26355/eurrev_201906_18086
158. Zhai M, Zhu Y, Yang M, Mao C. Human mesenchymal stem cell derived exosomes enhance cell-free bone regeneration by altering their miRNAs profiles. Adv Sci. (2020) 19:2001334. doi: 10.1002/advs.202001334
159. Li W, Liu Y, Zhang P, Tang Y, Zhou M, Jiang W, et al. Tissue-engineered bone immobilized with human adipose stem cells-derived exosomes promotes bone regeneration. ACS Appl Mater Interfaces. (2018) 6:5240–54. doi: 10.1021/acsami.7b17620
160. Chen L, Mou S, Li F, Zeng Y, Sun Y, Horch RE, et al. Self-assembled human adipose-derived stem cell-derived extracellular vesicle-functionalized biotin-doped polypyrrole titanium with long-term stability and potential osteoinductive ability. ACS Appl Mater Interfaces. (2019) 49:46183–96. doi: 10.1021/acsami.9b17015
161. Akdeniz-Dogan Z, Sendur S, Karademir-Yilmaz B, Bugdayci O, Cilingir-Kaya OT, Yilmaz-Goler AM, et al. The role of extracellular vesicles secreted from thermal stress-induced adipose-derived stem cells on bone regeneration. J Craniofac Surg. (2021) 6:2245–50. doi: 10.1097/SCS.0000000000007901
162. Gandolfi MG, Gardin C, Zamparini F, Ferroni L, Esposti MD, Parchi G, et al. Mineral-Doped Poly(L-lactide) acid scaffolds enriched with exosomes improve osteogenic commitment of human adipose-derived mesenchymal stem cells. Nanomaterials. (2020) 3. doi: 10.3390/nano10030432
163. Todorova D, Simoncini S, Lacroix R, Sabatier F, Dignat-George F. Extracellular vesicles in angiogenesis. Circulation Res. (2017) 10:1658–73. doi: 10.1161/CIRCRESAHA.117.309681
164. Narayanan R, Huang C-C, Ravindran S. Hijacking the cellular mail: exosome mediated differentiation of mesenchymal stem cells. Stem Cells Int. (2016) 2016. doi: 10.1155/2016/3808674
165. Takeuchi R, Katagiri W, Endo S, Kobayashi T. Exosomes from conditioned media of bone marrow-derived mesenchymal stem cells promote bone regeneration by enhancing angiogenesis. PLoS ONE. (2019) 11:e0225472. doi: 10.1371/journal.pone.0225472
166. James AW, Péault B. Perivascular mesenchymal progenitors for bone regeneration. J Orthop Res. (2019) 6:1221–8. doi: 10.1002/jor.24284
167. Xu J, Wang Y, Hsu C-Y, Gao Y, Meyers CA, Chang L, et al. Human perivascular stem cell-derived extracellular vesicles mediate bone repair. eLife. (2019) 8:e48191 doi: 10.7554/eLife.48191
168. Huang C-C, Kang M, Lu Y, Shirazi S, Diaz JI, Cooper LF, et al. Functionally engineered extracellular vesicles improve bone regeneration. Acta Biomaterialia. (2020) 109:182–94. doi: 10.1016/j.actbio.2020.04.017
169. Rachner TD, Khosla S, Hofbauer LC. Osteoporosis: now and the future. Lancet. (2011) 9773:1276–87. doi: 10.1016/S0140-6736(10)62349-5
170. Sözen T, Özişik L, Başaran NÇ. An overview and management of osteoporosis. Eur J Rheumatol. (2016) 1:46–56. doi: 10.5152/eurjrheum.2016.048
171. Hu Y, Xu R, Chen C-Y, Rao S-S, Xia K, Huang J, et al. Extracellular vesicles from human umbilical cord blood ameliorate bone loss in senile osteoporotic mice. Metab Clin Exp. (2019) 13:93–101. doi: 10.1016/j.metabol.2019.01.009
172. Hu R, Liu W, Li H, Yang L, Chen C, Xia Z-Y, et al. A Runx2/miR-3960/miR-2861 regulatory feedback loop during mouse osteoblast differentiation. J Biol Chem. (2011) 14:12328–39. doi: 10.1074/jbc.M110.176099
173. Xia Z, Hu Y, Xie P, Tang S, Luo X, Liao E, et al. Runx2/miR-3960/miR-2861 Positive FeedbackLoop is responsible for osteogenic transdifferentiation ofvascular smooth muscle cells. BioMed Research International. (2015) 2015:624037. doi: 10.1155/2015/624037
174. Lee KS, Lee J, Kim HK, Yeom SH, Woo CH, Jung YJ, et al. Extracellular vesicles from adipose tissue-derived stem cells alleviate osteoporosis through osteoprotegerin and miR-21-5p. J Extracell Vesicles. (2021) 12:e12052. doi: 10.1002/jev2.12152
175. Chen C-Y, Rao S-S, Tan Y-J, Luo M-J, Hu X-K, Yin H, et al. Extracellular vesicles from human urine-derived stem cells prevent osteoporosis by transferring CTHRC1 and OPG. Bone Rep. (2019) 7. doi: 10.1038/s41413-019-0056-9
176. Wang Y, Yao J, Cai L, Liu T, Wang X, Zhang Y, et al. Bone-targeted extracellular vesicles from mesenchymal stem cells for osteoporosis therapy. Int J Nanomedicine. (2020) 15:7967–77. doi: 10.2147/IJN.S263756
Keywords: extracellular vesicles, bone repair, osteogenesis, mesenchymal stromal cells, angiogenesis, cell-derived therapies
Citation: Brown SV, Dewitt S, Clayton A and Waddington RJ (2022) Identifying the Efficacy of Extracellular Vesicles in Osteogenic Differentiation: An EV-Lution in Regenerative Medicine. Front. Dent. Med. 3:849724. doi: 10.3389/fdmed.2022.849724
Received: 06 January 2022; Accepted: 14 February 2022;
Published: 17 March 2022.
Edited by:
Han Sung Jung, Yonsei University, South KoreaReviewed by:
Wei Seong Toh, National University of Singapore, SingaporeNan Jiang, Peking University Hospital of Stomatology, China
Copyright © 2022 Brown, Dewitt, Clayton and Waddington. This is an open-access article distributed under the terms of the Creative Commons Attribution License (CC BY). The use, distribution or reproduction in other forums is permitted, provided the original author(s) and the copyright owner(s) are credited and that the original publication in this journal is cited, in accordance with accepted academic practice. No use, distribution or reproduction is permitted which does not comply with these terms.
*Correspondence: Rachel J. Waddington, d2FkZGluZ3RvbnJqJiN4MDAwNDA7Y2FyZGlmZi5hYy51aw==