- Department of Periodontology, Osaka University Graduate School of Dentistry, Suita, Osaka, Japan
Energy metabolism is crucial in stem cells as they harbor various metabolic pathways depending on their developmental stages. Moreover, understanding the control of their self-renewal or differentiation via manipulation of their metabolic state may yield novel regenerative therapies. Periodontal ligament (PDL) cells existing between the tooth and alveolar bone are crucial for maintaining homeostasis in the periodontal tissue. In addition, they play a pivotal role in periodontal regeneration, as they possess the properties of mesenchymal stem cells and are capable of differentiating into osteogenic cells. Despite these abilities, the treatment outcome of periodontal regenerative therapy remains unpredictable because the biological aspects of PDL cells and the mechanisms of their differentiation remain unclear. Recent studies have revealed that metabolism and factors affecting metabolic pathways are involved in the differentiation of PDL cells. Furthermore, understanding the metabolic profile of PDL cells could be crucial in manipulating the differentiation of PDL cells. In this review, first we discuss the energy metabolism in osteoblasts and stem cells to understand the metabolism of PDL cells. Next, we summarize the metabolic preferences of PDL cells during their maintenance and cytodifferentiation. The perspectives discussed have potential applicability for creating a platform for reliable regenerative therapies for periodontal tissue.
Introduction
Periodontitis is an inflammatory disease of the tooth-supporting tissue that affects 20–50 percent of the world population (1). Numerous studies have shown its relation to a variety of systemic diseases, including metabolic syndrome, a group of risk factors linked to coronary heart disease and type 2 diabetes mellitus (1–3). It has been found that periodontitis and diabetes are interlinked (4). In addition, it has been reported that periodontal therapy improves metabolic outcomes, including blood sugar (glucose) levels in type 2 diabetes patients (5–7). However, further investigations are needed to elucidate the relationship between periodontitis and metabolism.
The periodontal ligament (PDL) plays a pivotal role in periodontal therapy, which is widely used for regenerating healthy periodontium destroyed by periodontal disease. The PDL is located between the cementum and alveolar bone and maintains the stability of the teeth in the alveolar bone (8). It is comprised of heterogeneous cell populations, comprehensively known as PDL cells, and acts as a reservoir of mesenchymal stem cells (MSCs) that can differentiate into multiple cell types, such as osteoblasts, adipocytes, and chondrocytes (9, 10). The cellular characteristics of PDL cells and their differentiation mechanisms are crucial in understanding homeostasis of periodontal tissue and the induction of effective periodontal regeneration. To date, numerous efforts have been made to identify the specific gene markers and the location of PDL cells with stemness (11–13); however, no direct evidence distinguishes the heterogeneous population of PDL cells.
Metabolism is a complicated balance between catabolism and anabolism, which are deeply involved in the fundamental cellular functions such as proliferation and differentiation (14). Recent studies have shed a light on energy metabolic profiles, characterizing the cellular status during the differentiation process (15). Moreover, the effects of stem cell energy metabolism on osteogenic differentiation have gained immense interest as the alterations in metabolism occur depending on their differentiation stages (16–18). PDL cells comprise a heterogeneous mixture of several cell types, including PDL stem cells and osteoprogenitor cells at different stages (19, 20). Although energy metabolism is involved in the osteogenic differentiation of PDL cells, insufficient evidence is available in this regard. This current review summarizes recent research highlighting the major metabolic pathways in osteoblasts and stem cells and further describes the role of energy metabolism in PDL cells for the future strategies of periodontal regeneration.
Glucose Metabolism During Osteoblast Differentiation
Glucose is the primary energy source for differentiating and maturing osteoblasts. Glucose is transported to cells via the glucose transporter family. To generate adenosine triphosphate (ATP) for energy, glucose molecules are converted to pyruvate for the tricarboxylic acid (TCA) cycle-driven oxidative phosphorylation (OXPHOS) and lactate-producing glycolysis in the presence and absence of oxygen, respectively (18, 21). Despite oxygen availability, glucose is utilized via lactate-producing glycolysis (aerobic glycolysis), a process known as the Warburg effect, which commonly occurs in cancer cells (22). Although glycolysis generates less ATP compared to OXPHOS, ATP production by aerobic glycolysis is faster and sufficient to support osteoblast differentiation; moreover, it is involved in citrate production, which is crucial for apatite nanocrystal formation (18, 21) (Figure 1). Recently, several studies have reported that disruption of aerobic glycolysis (such as via inhibition of lactate dehydrogenase A, LDHA), which is mainly involved in the conversion of pyruvate to lactate, negatively affects osteoblast differentiation, i.e., acceleration and stabilization of aerobic glycolysis upregulates the differentiation in osteoblasts (23–25). Hong et al. (23) revealed that overexpression of miR-34a targeting 3'UTR of LDHA suppresses late osteoblast differentiation in human mesenchymal stem cells by inhibiting cellular glycolysis. In addition, Wu et al. (24) showed that addition of exogenous lactic acid (LA) to MC3T3-E1 cells significantly increases alkaline phosphatase (ALP) activity, whereas siRNA treatment of lactate dehydrogenase B (LDHB), an enzyme converting LA to pyruvate, which is a fuel for OXPHOS, decreases ALP activity in MC3T3-E1 cells, indicating that lactate is important for osteoblast differentiation via aerobic glycolysis. Yang et al. (25) reported that a lack of leucine-rich repeat-containing G-protein-coupled receptor 4 (Lgr4) in preosteoblasts inhibits osteogenic function and decreases the glucose metabolism under aerobic conditions. Also, it causes reduced bone formation and impaired glycolysis in the osteoblast-specific Lgr4 conditional knockout mice (25). For another example, the overexpression of malic enzyme helps to uncouple pyruvate from OXPHOS and consequently enhances glycolysis and osteoblast differentiation (26).
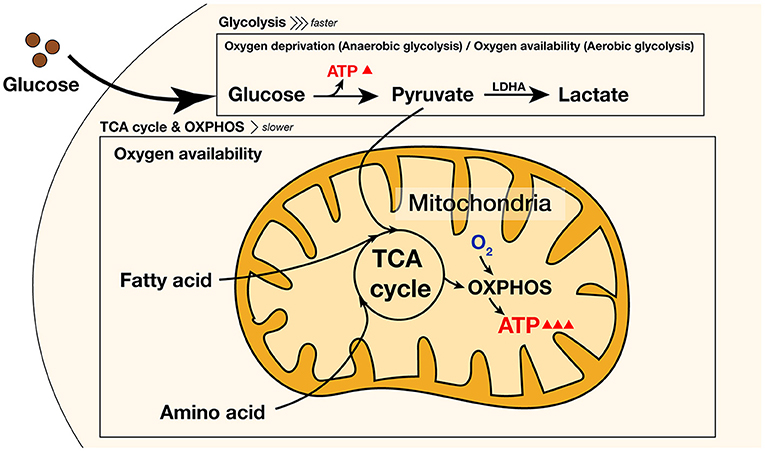
Figure 1. Pathways of intracellular energy metabolism during differentiation and reprogramming. In glucose metabolism, ATP is generated via aerobic/anaerobic glycolysis or OXPHOS that requires O2. Glycolysis generates ATP faster than OXPHOS, whereas OXPHOS slowly generates much more ATP than glycolysis. Amino acids and fatty acids fuel the TCA cycle and enhance the OXPHOS pathway to generate additional ATP. ATP, adenosine triphosphate; LDHA, lactate dehydrogenase A; TCA, tricarboxylic acid; OXPHOS, oxygen phosphorylation; O2, oxygen.
Nevertheless, the metabolic preference for glucose during osteoblast differentiation remains controversial. Some studies have reported that differentiating cells dominantly utilize glucose via OXPHOS during osteogenic induction (27, 28). A recent study has reported that inhibition of glycolysis by an LDHA inhibitor enhances OXPHOS and osteoblast differentiation (29). Moreover, the discrepancy in the energy metabolism is presumably caused by differences in cell types, their differentiation stage, energy substrate, aging, oxygen availability, and other experimental conditions (18, 28, 30). For example, there has been a discrepancy in which high glucose (HG) affects osteoblast differentiation positively or negatively; the effects of HG on osteoblast differentiation are altered depending on the stages of their differentiation (31). Wu et al. (24) have proposed that a mediator such as lactate enables the maintenance of homeostasis between glycolysis and OXPHOS, depending on the heterogeneous preferences of each cell. Therefore, it is worthy to note that glycolysis and OXPHOS pathways may orchestrate to keep a balance during various differentiation stages of osteogenic induction.
Metabolic Reprogramming of Stem Cells: A Way to Regeneration
Osteoprogenitor cells are committed from mesenchymal stem cells (MSCs), which are multipotent adult stem cells capable of differentiating into mesoderm-derived cells to maintain homeostasis and regenerative functions (32). Several studies reported that undifferentiated MSCs primarily rely on glycolysis. In contrast, after osteogenic induction, OXPHOS is upregulated during osteoblast differentiation, whereas glycolysis is maintained or gradually declines (16, 17). The transition from MSCs to mature osteoblasts employs dynamic metabolic preferences. Moreover, the specific environment of each MSC may be diversified by substrate and oxygen availability, leading to different metabolic states (33–37). Understanding energy metabolism in stem cells may guide the involvement of metabolic reprogramming during osteoblast differentiation.
Furthermore, metabolic reprogramming can alter the fate of stem cells, leading to epigenetic modifications (36). Several years ago, to reprogram human somatic cells, the induced pluripotent stem cells (iPSCs) were identified by external expression of Yamanaka factors (Octamer-binding transcription factor 4 (OCT4), SRY-box transcription factor 2 (SOX2), Kruppel-like factor 4 (KLF4) and MYC proto-oncogene, bHLH transcription factor (c-MYC)) (38). The upregulation of glycolysis and inhibition of OXPHOS by transfection of the Yamanaka factors strongly enhanced cellular pluripotency and nuclear reprogramming (39). Several studies have reported that the enhancement of glycolysis is crucial for metabolic reprogramming. Honkoop et al. (40) revealed that metabolic reprogramming by promoting glycolysis is involved in the heart regeneration of zebrafish. Pieknell et al. (41) reported that the overexpression of Lin-28 homolog A (LIN28A) promotes the regenerative ability of human somatic stem cells by enhancing glycolysis. Additionally, LIN28A supports mitochondrial health in pluripotency, leading to efficient differentiation when the metabolic preference shifts to OXPHOS (41). Hypoxia-inducible factor 1 subunit alpha (HIF1α) is a transcription factor that allows energy metabolism to shift toward glycolysis during the reprogramming process (42). Consequently, metabolic reprogramming has increasingly drawn attention to regenerative strategies in various fields (34, 43). For example, the enhancement of glycolysis mitigates cell death, resulting in improved efficacy of heart repair (34). Pre-treatment with lactate stabilizes HIF1α and upregulates metabolic regulators to promote metabolic reprogramming in human diploid fibroblasts (44).
Understanding Energy Metabolism in PDL Cells: A Possible Strategy for Periodontal Regeneration
It is more apparent that energy metabolism is involved in the osteogenic differentiation of PDL cells, although the studies in this regard are still insufficient and inconclusive. For example, several studies have reported controversial results for the effects of HG on the differentiation of PDL cells (45–47). The overexpression of LIN28A enhances the osteogenic process and calcified nodule formation by increasing lactate and ATP production and decreasing reactive oxygen species production (30); furthermore, proteomic analysis of human PDL cells has identified OXPHOS as the most significantly upregulated pathway during their osteogenic differentiation (48). Elucidation of the dynamic metabolic preference of PDL cells during differentiation will presumably explain the discrepancy among several studies on the energy metabolism of PDL cells.
Moreover, the importance of energy metabolism in PDL cells has shed a light on cellular reprogramming induced by the metabolic shifts. Reprogramming of human periodontal fibroblasts has been widely used in generating iPSCs by transfecting with the Yamanaka factors (49). Recently, Mao et al. (50) reported that the supplementation of succinate leads to a metabolic shift from OXPHOS to glycolysis by inducing pseudohypoxia via HIF1α in human PDL cells, even in the presence of oxygen. This pseudohypoxia condition promotes osteoblast differentiation (50).
Metabolic reprogramming is a new field of periodontal research. Thus, to date, the studies on these aspects are limited. Further understanding of metabolic reprogramming may pave the way for periodontal tissue regeneration.
Discussion
In the current review, we summarize the two principal pathways in cellular energy metabolism, namely glycolysis and mitochondrial OXPHOS, with respect to osteoblast differentiation and stem cell biology. Osteoblasts use glycolysis and OXPHOS depending on their differentiation stage (24, 31), whereas stem cells are highly dependent on glycolysis to maintain stemness (40, 41). As osteogenic differentiation requires tremendous energy, OXPHOS is generally used, particularly at the early stage of their differentiation (26). Additionally, osteoblasts have the ability to differentiate by glycolysis even under hypoxia, such as in the bone marrow cavity (51, 52). Lactate drives glycolysis through a positive feedback loop in hypoxic osteoblasts (24). It is presumed that by enhancing glycolysis, osteoblasts might obtain a certain amount of energy for differentiation in a short time, as glycolysis can produce energy rapidly. In contrast, the characteristics of the metabolism in PDL cells remain inconsistent because the roles of cellular metabolism during differentiation of PDL cells remain to be elucidated (45–47). The findings in the energy metabolism of osteogenic and stem cell biology suggest that undifferentiated PDL cells alter their metabolic profiles from glycolysis to OXPHOS toward their differentiation; however, the metabolism may be affected by stages of differentiation, aging, and oxygen availability, among other factors (Figure 2). Owing to the unique microenvironment of PDL cells, compared with the other osteogenic cells, and their heterogeneity, the bioenergetic phenotypes in PDL cells need to be investigated by exploring their characteristics in different osteogenic phenotypes derived from multiple donors.
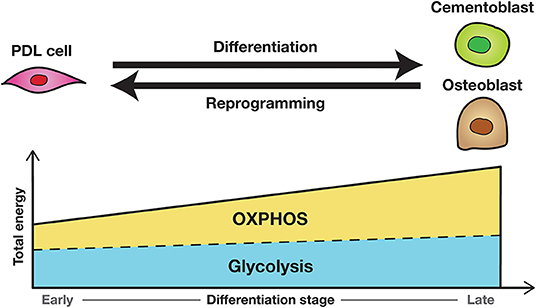
Figure 2. The hypothesis of dynamic metabolic preferences during osteogenic differentiation and reprogramming of PDL cells. OXPHOS is largely used at the early stage of differentiation because osteogenic differentiation requires tremendous energy. Undifferentiated PDL cells may alter their metabolic profiles from glycolysis to OXPHOS toward their differentiation; the osteogenic cells may do vice versa toward their reprogramming. PDL, periodontal ligament; OXPHOS, oxygen phosphorylation.
Moreover, further studies are required regarding the energy metabolism of PDL cells, with respect to the type of additional substrate that supports principal metabolic pathways used in PDL cells. Although glucose is the main energy source, other energy substrates are essential for osteoblast differentiation, including fatty acids and amino acids, as they play important roles in homeostasis and osteoblast differentiation as well as glucose (53, 54). For instance, fatty acids are essential for appropriate differentiation and serve as a substrate for ATP production, whereas glutamine is involved in the commitment to the osteoblast lineage. Various amino acids, including, but not limited to, glutamine, are essential for the production of cellular structures as well as the stability and fate of pluripotent stem cells by supplying functional groups for chromatin modification. Furthermore, some amino acids can promote osteogenic differentiation (18, 33, 55). Multiple studies have revealed that altered metabolism of these substrates affects osteoblast differentiation in both negative and positive ways. For example, by using the glutaminase (Gls) -floxed mice crossed with the Paired-related homeobox 1 (Prx1) Cre mice expressing Cre recombinase specifically throughout the limb bud mesenchyme, the deletion of Gls in the mesenchymal progenitor cells of limb bud impairs the osteogenic phenotype and osteoblast differentiation (54). In addition, the inhibition of fatty acid utilization negatively affects osteoblast differentiation (53). Although several studies have reported the roles of amino acids and lipids in differentiation of osteoblasts and stem cells, little attention has been paid to their roles in differentiation of PDL cells. Further studies in this aspect will be essential to successfully stimulate periodontal regeneration because amino acids or lipids can induce PDL cells to differentiate into osteogenic cells by activating the TCA cycle and enhancing the OXPHOS pathway (Figure 1).
Recently, new technologies have enabled us to monitor the real-time alteration of cellular metabolism and analyze the large-scale profiles of metabolites simultaneously in vitro or ex vivo (56, 57). Ex vivo monitoring of stem cell metabolism can be useful for selecting cells with stemness for periodontal regenerative therapy using MSC transplantation. The application of metabolic regulators shifting to glycolysis might encourage the reprogramming of differentiated cells to the undifferentiated stage, leading to potent periodontal regeneration. In the near future, we will understand the entire scenario of energy metabolic pathways at the single-cell level as well as monitor cellular metabolic shifts in vivo. By applying these technologies to periodontal regenerative therapies, we could presumably examine the metabolism of PDL cells at the cellular level before applying periodontal regenerative therapy. Based on their metabolic preference, we could assess whether PDL cells remaining at the operation sites have potential for the success of regenerative therapies. This will allow us to predict the efficacy of regeneration at a chairside. Furthermore, by utilizing adjunctive substrates triggering the OXPHOS pathway, we could potentially enhance neogenesis of cementum and alveolar bone. Eventually, targeting the energy metabolism of PDL cells may be a potential strategy for predicting and increasing the efficacy of periodontal regeneration.
In conclusion, osteogenic progenitors, including PDL cells, use glucose as the main source for energy production, and energy metabolism is important for their osteogenic differentiation and reprogramming. Metabolic preference depends on cell types, substrate, and the oxygen environment. Thus, elucidation of a holistic view of the metabolic profile of PDL cells at different stages and manipulation of the differentiation of PDL cells will help design an effective strategy for reliable regenerative therapies for periodontal tissue.
Author Contributions
CF and TN contributed to the conception, drafted the manuscript, and revised the manuscript. SM contributed to critical revision of the manuscript. All authors approved the current and any revised version to be submitted.
Funding
This study was supported by the Japan Society for the Promotion of Science (JSPS) KAKENHI Grant Numbers T21K195950, A20H038640, and A19H010690 and the Frontiers Fee Support program.
Conflict of Interest
The authors declare that the research was conducted in the absence of any commercial or financial relationships that could be construed as a potential conflict of interest.
Publisher's Note
All claims expressed in this article are solely those of the author and do not necessarily represent those of their affiliated organizations or those of the publisher, the editors, and the reviewers. Any product that may be evaluated in this article, or claim that may be made by its manufacturer, is not guaranteed or endorsed by the publisher.
References
1. Nazir MA. Prevalence of periodontal disease, its association with systemic diseases and prevention. Int J Health Sci. (2017) 11:72–80.
2. Ngoude JXE, Moor VJA, Nadia-Flore TT, Agoons BB, Marcelle GGC, MacBrain EE, et al. Relationship between periodontal diseases and newly-diagnosed metabolic syndrome components in a sub-Saharan population: a cross sectional study. BMC Oral Health. (2021) 21:326. doi: 10.1186/s12903-021-01661-6
3. Gobin R, Tian D, Liu Q, Wang J. Periodontal diseases and the risk of metabolic syndrome: an updated systematic review and meta-analysis. Front Endocrinol. (2020) 11:336. doi: 10.3389/fendo.2020.00336
4. Stohr J, Barbaresko J, Neuenschwander M, Schlesinger S. Bidirectional association between periodontal disease and diabetes mellitus: a systematic review and meta-analysis of cohort studies. Sci Rep. (2021) 11:13686. doi: 10.1038/s41598-021-93062-6
5. Teshome A, Yitayeh A. The effect of periodontal therapy on glycemic control and fasting plasma glucose level in type 2 diabetic patients: systematic review and meta-analysis. BMC Oral Health. (2016) 17:31. doi: 10.1186/s12903-016-0249-1
6. Baeza M, Morales A, Cisterna C, Cavalla F, Jara G, Isamitt Y, et al. Effect of periodontal treatment in patients with periodontitis and diabetes: systematic review and meta-analysis. J Appl Oral Sci. (2020) 28:e20190248. doi: 10.1590/1678-7757-2019-0248
7. Cao R, Li Q, Wu Q, Yao M, Chen Y, Zhou H. Effect of non-surgical periodontal therapy on glycemic control of type 2 diabetes mellitus: a systematic review and Bayesian network meta-analysis. BMC Oral Health. (2019) 19:176. doi: 10.1186/s12903-019-0829-y
8. Beertsen W, McCulloch CA, Sodek J. The periodontal ligament: a unique, multifunctional connective tissue. Periodontol 2000. (1997) 13:20–40. doi: 10.1111/j.1600-0757.1997.tb00094.x
9. McCulloch CA, Bordin S. Role of fibroblast subpopulations in periodontal physiology and pathology. J Periodontal Res. (1991) 26:144–54. doi: 10.1111/j.1600-0765.1991.tb01638.x
10. Isaka J, Ohazama A, Kobayashi M, Nagashima C, Takiguchi T, Kawasaki H, et al. Participation of periodontal ligament cells with regeneration of alveolar bone. J Periodontol. (2001) 72:314–23. doi: 10.1902/jop.2001.72.3.314
11. Huang GT, Gronthos S, Shi S. Mesenchymal stem cells derived from dental tissues vs. those from other sources: their biology and role in regenerative medicine. J Dent Res. (2009) 88:792–806. doi: 10.1177/0022034509340867
12. Bosshardt DD, Lang NP. The junctional epithelium: from health to disease. J Dent Res. (2005) 84:9–20. doi: 10.1177/154405910508400102
13. Nagata M, Chu AKY, Ono N, Welch JD, Ono W. Single-cell transcriptomic analysis reveals developmental relationships and specific markers of mouse periodontium cellular subsets. Front Dent Med. (2021) 2:679937. doi: 10.3389/fdmed.2021.679937
14. Agathocleous M, Harris WA. Metabolism in physiological cell proliferation and differentiation. Trends Cell Biol. (2013) 23:484–92. doi: 10.1016/j.tcb.2013.05.004
15. Folmes CD, Dzeja PP, Nelson TJ, Terzic A. Metabolic plasticity in stem cell homeostasis and differentiation. Cell Stem Cell. (2012) 11:596–606. doi: 10.1016/j.stem.2012.10.002
16. Chen CT, Shih YR, Kuo TK, Lee OK, Wei YH. Coordinated changes of mitochondrial biogenesis and antioxidant enzymes during osteogenic differentiation of human mesenchymal stem cells. Stem Cells. (2008) 26:960–8. doi: 10.1634/stemcells.2007-0509
17. Shum LC, White NS, Mills BN, Bentley KL, Eliseev RA. Energy metabolism in mesenchymal stem cells during osteogenic differentiation. Stem Cells Dev. (2016) 25:114–22. doi: 10.1089/scd.2015.0193
18. Lee WC, Guntur AR, Long F, Rosen CJ. Energy metabolism of the osteoblast: implications for osteoporosis. Endocr Rev. (2017) 38:255–66. doi: 10.1210/er.2017-00064
19. Zhu W, Liang M. Periodontal ligament stem cells: current status, concerns, and future prospects. Stem Cells Int. (2015) 2015:972313. doi: 10.1155/2015/972313
20. Seo BM, Miura M, Gronthos S, Bartold PM, Batouli S, Brahim J, et al. Investigation of multipotent postnatal stem cells from human periodontal ligament. Lancet. (2004) 364:149–55. doi: 10.1016/S0140-6736(04)16627-0
21. Donat A, Knapstein PR, Jiang S, Baranowsky A, Ballhause TM, Frosch KH, et al. Glucose metabolism in osteoblasts in healthy and pathophysiological conditions. Int J Mol Sci. (2021) 22:4120. doi: 10.3390/ijms22084120
22. Warburg O. On the origin of cancer cells. Science. (1956) 123:309–14. doi: 10.1126/science.123.3191.309
23. Hong M, Zhang XB, Xiang F, Fei X, Ouyang XL, Peng XC. MiR-34a suppresses osteoblast differentiation through glycolysis inhibition by targeting lactate dehydrogenase-A (LDHA). In Vitro Cell Dev Biol Anim. (2020) 56:480–7. doi: 10.1007/s11626-020-00467-0
24. Wu Y, Wang M, Feng H, Peng Y, Sun J, Qu X, et al. Lactate induces osteoblast differentiation by stabilization of HIF1alpha. Mol Cell Endocrinol. (2017) 452:84–92. doi: 10.1016/j.mce.2017.05.017
25. Yang YY, Zhou YM, Xu JZ, Sun LH, Tao B, Wang WQ, et al. Lgr4 promotes aerobic glycolysis and differentiation in osteoblasts via the canonical Wnt/beta-catenin pathway. J Bone Miner Res. (2021) 36:1605–20. doi: 10.1002/jbmr.4321
26. Lee WC, Ji X, Nissim I, Long F. Malic enzyme couples mitochondria with aerobic glycolysis in osteoblasts. Cell Rep. (2020) 32:108108. doi: 10.1016/j.celrep.2020.108108
27. Muller DIH, Stoll C, Palumbo-Zerr K, Bohm C, Krishnacoumar B, Ipseiz N, et al. PPARdelta-mediated mitochondrial rewiring of osteoblasts determines bone mass. Sci Rep. (2020) 10:8428. doi: 10.1038/s41598-020-65305-5
28. Smith CO, Eliseev RA. Energy metabolism during osteogenic differentiation: the role of akt. Stem Cells Dev. (2021) 30:149–62. doi: 10.1089/scd.2020.0141
29. Hollenberg AM, Smith CO, Shum LC, Awad H, Eliseev RA. Lactate dehydrogenase inhibition with oxamate exerts bone anabolic effect. J Bone Miner Res. (2020) 35:2432–43. doi: 10.1002/jbmr.4142
30. Park JH, Park BW, Kang YH, Byun SH, Hwang SC, Kim DR, et al. Lin28a enhances in vitro osteoblastic differentiation of human periosteum-derived cells. Cell Biochem Funct. (2017) 35:497–509. doi: 10.1002/cbf.3305
31. Takeno A, Kanazawa I, Tanaka KI, Notsu M, Kanasaki K, Oono T, et al. High glucose promotes mineralization via bone morphogenetic protein 4-Smad signals in early stage of osteoblast differentiation. Diabetol Int. (2021) 12:171–80. doi: 10.1007/s13340-020-00463-5
32. Ullah I, Subbarao RB, Rho GJ. Human mesenchymal stem cells - current trends and future prospective. Biosci Rep. (2015) 35:e00191. doi: 10.1042/BSR20150025
33. Tsogtbaatar E, Landin C, Minter-Dykhouse K, Folmes CDL. Energy metabolism regulates stem cell pluripotency. Front Cell Dev Biol. (2020) 8:87. doi: 10.3389/fcell.2020.00087
34. Rigaud VOC, Hoy R, Mohsin S, Khan M. Stem cell metabolism: powering cell-based therapeutics. Cells. (2020) 9:2490. doi: 10.3390/cells9112490
35. Rafalski VA, Mancini E, Brunet A. Energy metabolism and energy-sensing pathways in mammalian embryonic and adult stem cell fate. J Cell Sci. (2012) 125:5597–608. doi: 10.1242/jcs.114827
36. Ryall JG, Cliff T, Dalton S, Sartorelli V. Metabolic reprogramming of stem cell epigenetics. Cell Stem Cell. (2015) 17:651–62. doi: 10.1016/j.stem.2015.11.012
37. Shyh-Chang N, Ng HH. The metabolic programming of stem cells. Genes Dev. (2017) 31:336–46. doi: 10.1101/gad.293167.116
38. Takahashi K, Tanabe K, Ohnuki M, Narita M, Ichisaka T, Tomoda K, et al. Induction of pluripotent stem cells from adult human fibroblasts by defined factors. Cell. (2007) 131:861–72. doi: 10.1016/j.cell.2007.11.019
39. Nishimura K, Fukuda A, Hisatake K. Mechanisms of the metabolic shift during somatic cell reprogramming. Int J Mol Sci. (2019) 20:2254. doi: 10.3390/ijms20092254
40. Honkoop H, de Bakker DE, Aharonov A, Kruse F, Shakked A, Nguyen PD, et al. Single-cell analysis uncovers that metabolic reprogramming by ErbB2 signaling is essential for cardiomyocyte proliferation in the regenerating heart. Elife. (2019) 8:e50163. doi: 10.7554/eLife.50163
41. Pieknell K, Sulistio YA, Wulansari N, Darsono WHW, Chang MY, Ko JY, et al. LIN28A enhances regenerative capacity of human somatic tissue stem cells via metabolic and mitochondrial reprogramming. Cell Death Differ. (2021). doi: 10.1038/s41418-021-00873-1
42. Ishida T, Nakao S, Ueyama T, Harada Y, Kawamura T. Metabolic remodeling during somatic cell reprogramming to induced pluripotent stem cells: involvement of hypoxia-inducible factor 1. Inflamm Regen. (2020) 40:8. doi: 10.1186/s41232-020-00117-8
43. Walden EL, Li S. Metabolic reprogramming of glial cells as a new target for central nervous system axon regeneration. Neural Regen Res. (2022) 17:997–8. doi: 10.4103/1673-5374.324833
44. Kozlov AM, Lone A, Betts DH, Cumming RC. Lactate preconditioning promotes a HIF-1alpha-mediated metabolic shift from OXPHOS to glycolysis in normal human diploid fibroblasts. Sci Rep. (2020) 10:8388. doi: 10.1038/s41598-020-65193-9
45. Seubbuk S, Sritanaudomchai H, Kasetsuwan J, Surarit R. High glucose promotes the osteogenic differentiation capability of human periodontal ligament fibroblasts. Mol Med Rep. (2017) 15:2788–94. doi: 10.3892/mmr.2017.6333
46. Liu Z, Chen T, Sun W, Yuan Z, Yu M, Chen G, et al. DNA demethylation rescues the impaired osteogenic differentiation ability of human periodontal ligament stem cells in high glucose. Sci Rep. (2016) 6:27447. doi: 10.1038/srep27447
47. Zhen L, Jiang X, Chen Y, Fan D. MiR-31 is involved in the high glucose-suppressed osteogenic differentiation of human periodontal ligament stem cells by targeting Satb2. Am J Transl Res. (2017) 9:2384–93.
48. Li J, Wang Z, Huang X, Wang Z, Chen Z, Wang R, et al. Dynamic proteomic profiling of human periodontal ligament stem cells during osteogenic differentiation. Stem Cell Res Ther. (2021) 12:98. doi: 10.1186/s13287-020-02123-6
49. Wada N, Wang B, Lin NH, Laslett AL, Gronthos S, Bartold PM. Induced pluripotent stem cell lines derived from human gingival fibroblasts and periodontal ligament fibroblasts. J Periodontal Res. (2011) 46:438–47. doi: 10.1111/j.1600-0765.2011.01358.x
50. Mao H, Yang A, Zhao Y, Lei L, Li H. Succinate supplement elicited “pseudohypoxia” condition to promote proliferation, migration, and osteogenesis of periodontal ligament cells. Stem Cells Int. (2020) 2020:2016809. doi: 10.1155/2020/2016809
51. Munoz N, Kim J, Liu Y, Logan TM, Ma T. Gas chromatography-mass spectrometry analysis of human mesenchymal stem cell metabolism during proliferation and osteogenic differentiation under different oxygen tensions. J Biotechnol. (2014) 169:95–102. doi: 10.1016/j.jbiotec.2013.11.010
52. Watanuki S, Kobayashi H, Sorimachi Y, Yamamoto M, Okamoto S, Takubo K, et al. turnover and glucose dependency in hematopoietic stem/progenitor cells are increased by proliferation and differentiation. Biochem Biophys Res Commun. (2019) 514:287–94. doi: 10.1016/j.bbrc.2019.04.123
53. Rendina-Ruedy E, Guntur AR, Rosen CJ. Intracellular lipid droplets support osteoblast function. Adipocyte. (2017) 6:250–8. doi: 10.1080/21623945.2017.1356505
54. Yu Y, Newman H, Shen L, Sharma D, Hu G, Mirando AJ, et al. Glutamine metabolism regulates proliferation and lineage allocation in skeletal stem cells. Cell Metab. (2019) 29:966–78. doi: 10.1016/j.cmet.2019.01.016
55. Liu K, Cao J, Shi X, Wang L, Zhao T. Cellular metabolism and homeostasis in pluripotency regulation. Protein Cell. (2020) 11:630–40. doi: 10.1007/s13238-020-00755-1
56. Zhang J, Chen H, Kornreich R, Yu C. Prenatal diagnosis of tay-sachs disease. Methods Mol Biol. (2019) 1885:233–50. doi: 10.1007/978-1-4939-8889-1_16
Keywords: energy metabolism, osteogenic differentiation, metabolic reprogramming, periodontal regeneration, periodontal ligament cells, osteoblasts
Citation: Fujihara C, Nantakeeratipat T and Murakami S (2022) Energy Metabolism in Osteogenic Differentiation and Reprogramming: A Possible Future Strategy for Periodontal Regeneration. Front. Dent. Med. 3:815140. doi: 10.3389/fdmed.2022.815140
Received: 15 November 2021; Accepted: 06 January 2022;
Published: 28 January 2022.
Edited by:
Han Sung Jung, Yonsei University, South KoreaReviewed by:
Jinhua Yu, Nanjing Medical University, ChinaMaisa Hanna-Maija Seppala, King's College London, United Kingdom
Copyright © 2022 Fujihara, Nantakeeratipat and Murakami. This is an open-access article distributed under the terms of the Creative Commons Attribution License (CC BY). The use, distribution or reproduction in other forums is permitted, provided the original author(s) and the copyright owner(s) are credited and that the original publication in this journal is cited, in accordance with accepted academic practice. No use, distribution or reproduction is permitted which does not comply with these terms.
*Correspondence: Chiharu Fujihara, ZnVqaWhhcmEuY2hpaGFydS5kZW50QG9zYWthLXUuYWMuanA=
†These authors have contributed equally to this work and share first authorship