- 1National Institute of Dental and Craniofacial Research, National Institutes of Health, Bethesda, MD, United States
- 2Department of Periodontics, College of Dentistry, University of Iowa, Iowa City, IA, United States
The oral microbiome (OM) is a diverse and dynamic collection of species, separated from the alveolar bone by the oral mucosa. Pathogenic shifts in the OM (dysbiosis) during periodontitis are associated with an inflammatory response in the oral mucosa that drives alveolar bone resorption. The alveolar bone is also affected by metabolic disorders such as osteoporosis. Accumulating evidence has linked another microbial community, the gut microbiome (GM), to systemic bone metabolism and osteoporosis. Underlying this connection is the biological activity of metabolites, byproducts of host and bacterial activity. Limited evidence also suggests that metabolites in the oral cavity signal between the OM and immune system, influencing both alveolar bone homeostasis and pathologic bone destruction in periodontitis. While the oral cavity and gut are connected through the gastrointestinal tract, dissimilar roles for known metabolites between these two niches exemplify the difficulty in translating knowledge on gut-derived metabolites and bone metabolism to the alveolar bone. Integrated metabolomic, transcriptomic, and metagenomic approaches hold promise for resolving these challenges and identifying novel metabolites that impact alveolar bone health. Further interrogation through mechanistic testing in pre-clinical models and carefully controlled clinical studies have the potential to lead to the translation of these discoveries into meaningful therapies.
Introduction
The human body is colonized by trillions of microbes (1). Recent advances, including the Human Microbiome Project and the development of next-generation sequencing technologies, have convincingly demonstrated that distinct microbial communities colonize different body sites and interact with host cells to modulate health and disease (2, 3). It is further established that maintenance of health requires a state of homeostasis between the microbiome and immune system across different body sites, also known as niches (4, 5). Two distinct niches, the gut and oral cavity, are characterized by a complex relationship between the host and gut microbiome (GM) and oral microbiome (OM), respectively (6, 7). Disturbances in these homeostatic interactions drive dysbiosis and inflammation and are associated with several chronic diseases, including inflammatory bowel disease (IBD), type 2 diabetes (T2D), obesity, metabolic syndrome, osteoporosis, rheumatoid arthritis, Alzheimer's disease, periodontal disease, dental caries, and various cancers (8, 9). The role of the OM in driving alveolar bone destruction is well established (10), and the role for the GM in regulating systemic bone health has become increasingly appreciated (11, 12). Accordingly, the nature of the microbial-host interrelationships that regulate bone metabolism in health and disease are active areas of investigation.
The alveolar bone is the specialized portion of the mandible and maxilla which houses, supports, and protects the root structures of teeth (13). Formation and remodeling of alveolar bone are shaped by local factors, such as the eruption of teeth into the oral cavity and ongoing masticatory forces and systemic regulation through hormonal and metabolic signaling (14, 15). Distinct from other skeletal structures, alveolar bone lies in close proximity to OM biofilms and undergoes resorption during the course of periodontitis, a chronic and widespread disease (16). The periodontitis-associated OM is characterized by dysbiotic biofilms on tooth and root surfaces containing several pathogenic species such as P. gingivalis, Treponema denticola, Tannerella forsythia, and A. actinomycetemcomitans (17). Concurrently, a heavy immune cell infiltration is present in the gingiva, the oral mucosal tissue surrounding the teeth, which drives osteoclast activity in the underlying alveolar bone (18, 19). Diseases that affect bone metabolism, such as osteoporosis, also affect alveolar bone health (20). Thus, an interplay between OM, the associated immune response, and local and systemic factors affecting bone shape the pathogenesis of alveolar bone loss.
Metabolites, the currency of bacterial-host crosstalk
Metabolites are the byproducts of microbial or host metabolism specific to the environment, modulating health by signaling to host cells and influencing bacterial community interactions (21). Host amino acids (22) and byproducts from glucose-related pathways (e.g., glycolysis and gluconeogenesis) (23) and mitochondrial metabolism (e.g., tricarboxylic acid cycle metabolites succinate, fumarate, and aconitate) (24) have well-known roles in signaling within and between immune and bone cell populations. Microbial metabolite production, best characterized in the gut environment, is heavily driven by dietary intake, with fermentation of complex carbohydrates and proteins leading to production of short- and branched-chain fatty acids, and metabolism of proteins and peptides producing amines, phenols, and indoles from amino acids (25, 26).
Interactions between microbially-derived metabolites and host cells are increasingly recognized as drivers of human health and disease (21, 27). Extensive research in the gut has identified roles for microbially derived metabolites, including secondary bile acids, short-chain fatty acids (SCFAs), trimethylamine-N-oxide (TMAO), polysaccharide A, 4-ethyl phenyl sulfate, and catecholamines, in systemic diseases affecting bone (28–31). In contrast, there is currently a narrower understanding of the scope and nature of OM-derived metabolites and their role in alveolar bone health (Figure 1).
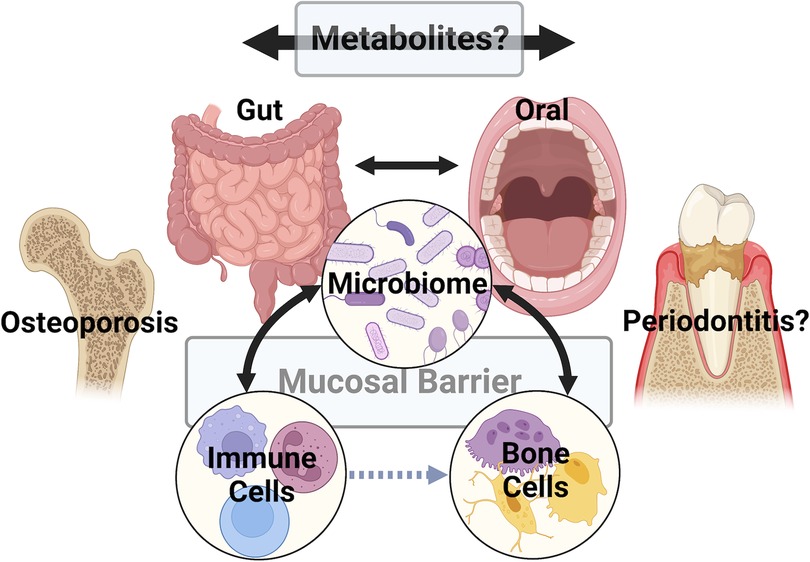
Figure 1 A multitude of studies have explored the connections between the gut and/or oral microbiomes, the host immune system, and bone cells (i.e., osteoblasts, osteocytes, and osteoclasts). Recent work suggests that metabolites are key signaling factors in these pathways (represented by black bidirectional arrows), acting directly or indirectly (i.e. via the immune system – gray dashed arrow) to influence pathologic bone disorders like osteoporosis. A significant challenge is translating knowledge gained from studies of the gut and osteoporosis to the oral cavity to understand if metabolites play similar or distinct roles in the metabolism of alveolar bone. Underlying this challenge are differences in mucosal barrier structures, microbiome populations, and immune cells between the gut and oral mucosa. Figure created with BioRender.com.
The emerging field of metabolomics has enabled cataloging of both well-known and novel metabolites using an array of platforms and techniques (32). The number of metabolite entries in the Human Metabolome Database, the most comprehensive collection of human metabolites, has burgeoned from 2,180 entries in 2007 to 217,920 annotated metabolite entries and 1,581,537 unannotated entries (33). These technological advances in unbiased metabolomics have significant potential to (1) uncover the net biological activity in the oral cavity, (2) expand our knowledge of the pathogenesis of alveolar bone destruction beyond identifying specific bacterial species, and (3) identify novel targets for disease diagnosis, prognosis, and treatment.
Lessons learned from the gut microbiome
The microbiome colonizing the human intestine, known as the gut microbiome (GM), is the largest microbial niche in the human body and comprises a complex ecosystem with established roles in human health and disease (34). Initially formed in utero or at birth, the GM rapidly develops between ages 1–4 and continues to evolve in response to intrinsic and environmental factors such as geographic location, gender, diet, and antibiotic use (35). The intestinal mucosal epithelium serves as the interface between the host and the microbiome, controlling interactions through the coordinated activities of mucus, epithelial cell junctions, immunoglobulin A, antimicrobial peptides, and immune cells (36, 37). Nutrients and metabolites also pass through this barrier to interact with local cells or enter the circulation (38).
Bone remodeling and homeostasis are regulated by a network of systemic hormones, including parathyroid hormone (PTH), calcitonin, FGF23, 1,25-dihydroxyvitamin D3 (Vitamin D), and estrogen. The GM is considered an endocrine organ (39) and animal models show that altering or preventing GM development influences skeletal bone mass and osteoclast activity (40–42). Gut microbes synthesize vitamin K2 which stimulates osteoblast activity and is a cofactor for post-translational modification of osteocalcin (12). Disruption of the ecosystem with antibiotics inhibits vitamin K2 synthesis and reduces bone quality (43). Enzymes secreted by gut microbiota can metabolize or re-activate estrogen, altering circulating or excreted levels (44). GM dysbiosis can also mediate estrogen deficiency-related bone loss through increases in mucosal permeability, immune cell numbers, and inflammatory cytokines (45, 46).
The GM can enable serotonin production by enterochromaffin cells (47) and deconjugate bile acid compounds and further metabolize them to secondary bile acids such as lithocholic and deoxycholic acid (48). Gut-derived serotonin may inhibit bone formation (49) and lithocholic acid can bind vitamin D receptor (VDR), leading to inactivation of vitamin D and decreased intestinal calcium absorption (50). Bile acids can also signal enteroendocrine cells to release GLP-1 which promotes bone formation and inhibits bone resorption (51). Hydrogen sulfide (H2S) is produced by gastrointestinal cells and the GM (52). Loss of H2S results in osteopenia in mice (53) and administration of an H2S donating compound in ovariectomy-treated mice improves bone formation (54).
Emerging evidence points to gut-derived short-chain fatty acids (SCFAs) as modulators of systemic health and bone maintenance [see reviews (11, 12, 55, 56)]. In brief, SCFAs, including butyrate, propionate, and acetate, are primarily produced by microbial fermentation of non-digestible polysaccharides and are rapidly absorbed through the intestinal mucosa, acting as a source of energy for both host and microbiota (56). While SCFAs can directly suppress osteoclast activity and promote osteoblast differentiation (57, 58), signaling between SCFA and endocrine organs or immune cells may underlie the connection between GM and bone. Gut microbial colonization or SCFA supplementation is associated with the production of insulin-like growth factor 1 (IFG-1), an important hormone for skeletal growth and bone mass maintenance (59). SCFAs, including butyrate, promote proliferation and differentiation of regulatory T cells (Treg) (60) which may reduce bone absorption by interfering with osteoclast development and activity (61). Butyrate can increase Treg numbers in the intestine and bone marrow which signals to CD8+ T cells to produce WNT10b, a bone anabolic signaling factor (62). Butyrate produced by GM may also regulate PTH-mediated bone formation through signaling in dendritic cells and Tregs (63).
Probiotics have been widely studied as a means to target osteoporosis via manipulation of the GM (64). A clinical study showed Lactobaciillus reuteri probiotics increased BMD and elevated butyrylcarnitine, which can act as a pool and transporter of butyrate (65). Prebiotics are non-digestible oligosaccharides that are selectively fermented in the colon and support the growth of specific bacterial species. Positive results for prebiotics in animal models, including increased calcium absorption and improved BMD and bone strength, have been primarily attributed to fermentation of prebiotics to SCFAs by GM (66). Clinical trials have further indicated that prebiotics can increase intestinal calcium absorption (67).
Altogether, the GM plays a critical role in regulating systemic bone metabolism, in part, through production of metabolites. GM-derived metabolites act both locally and systemically on host cells to drive immune responses that shape bone metabolism. Improved understanding of GM metabolites and their role in shaping bone health have led to development of therapeutic interventions, including probiotics and prebiotics, suggesting that probing the connection between the oral cavity and gut and identifying similar pathways in the oral cavity has promise for improving alveolar bone health.
The oral-gut-bone connection
Ingested saliva, food, and drink directly connect the OM and GM (68, 69). Patients with conditions characterized by GM inflammation and dysbiosis, such as inflammatory bowel disease, have an altered OM, increased numbers of OM-derived species in the gut, and higher rates of periodontitis (70, 71). Studies in mice suggest that ingested OM bacteria can reach the gut and induce an inflammatory immune response (72), and immune cells exposed to OM can reach the gut to interact with OM-derived gut microbes (73). Gut colonization with specific bacterial species can also influence T cell development in alveolar bone marrow and increase alveolar bone osteoclast activity, further illustrating the potential bidirectional mechanisms whereby microbial populations in both gut and oral cavity can help disrupt or maintain bone homeostasis (74).
Studies probing the oral-gut connection have shown that administering oral P. gingivalis modifies the GM and alters serum and gut metabolite profiles (75, 76), including increasing gut lactic acid and reducing succinic acid and butyrate levels (77). Additional evidence connecting GM, metabolites, and alveolar bone has been provided by animal studies of probiotic administration or diet alterations. In ovariectomized rats, probiotics increased levels of butyrate-producing GM and reduced osteoclast and Th17 cell numbers while increasing Treg cells and minimizing maxillary bone loss during ligature-induced periodontitis (78). Transplantation of fecal contents from high fat diet (HFD) obese mice altered host GM and gut and serum metabolite compositions with little change in the OM while increasing Th17 cells in submandibular and mesenteric lymph nodes and aggravating alveolar bone loss in experimental periodontitis (79). One metabolite of purine degradation, uric acid, was increased in serum with HFD fecal transplant and induction of periodontitis, and administration of allopurinol suppressed alveolar bone destruction in uremic mice (79).
Overall, these findings lend support to the concept that oral health is connected to systemic health and highlight distinct molecular pathways connecting the gut and oral microbiomes and the immune system through metabolites. Whether such mechanisms identified in mouse models can be translated to meaningful interventions in humans is still unknown. Nevertheless, such studies provide further motivation for studying the role of metabolites in bone health and, in particular, within the oral niche.
Oral metabolites and alveolar bone
The oral cavity is rich in byproducts of host and OM metabolism (80). Saliva and gingival crevicular fluid (GCF) show distinct profiles of metabolite compositions between health and periodontitis (81–83) with clinical studies showing specific associations between periodontitis and increased levels of arachidonic acid, purine, pyrimidine, glutathione, and amino acid metabolites (84–87). Accordingly, various metabolites have been explored as predictors of gingival inflammation or periodontitis (88) or as factors that regulate the disruption or maintenance of the gingival epithelial barrier (junctional epithelium) (89). However, clear evidence is lacking for how specific metabolites or metabolic pathways act to help maintain alveolar bone in oral health or aggravate bone destruction during periodontitis.
Existing studies on oral metabolites and alveolar bone have focused on butyrate, and contrary to the gut, have ascribed it a pathogenic role in periodontitis (Figure 2). This distinction may be due to several factors, including differences in butyrate concentrations, mucosal tissue structure, and microbial populations between GM and OM environments (90, 91). Periodontitis-associated oral bacteria, P. gingivalis and F. nucleatum, produce butyrate (92). Further, butyrate can stimulate heme production which supports the growth of periodontal pathogens like P. gingivalis (90). Butyrate concentrations in periodontal pockets can reach up to 14 mM (93) with levels correlating to periodontal disease severity (94) and decreasing in GCF after periodontal treatment (95). While butyrate levels may be similar or higher in the colon compared to the oral cavity, a much lower concentration may actually reach colonic epithelial cells after penetrating through the thick colon mucous layer (96). A recent animal study found that butyrate could disrupt the periodontal junctional epithelial barrier (97). This finding, coupled with in vitro studies showing a negative effect of butyrate on different oral cell types (90, 91), and in particular, epithelial cells (98), suggests that differences in the mucosal barrier anatomy between the gut and periodontal tissues could account for some of the opposing effects of butyrate on alveolar vs. other bone sites.
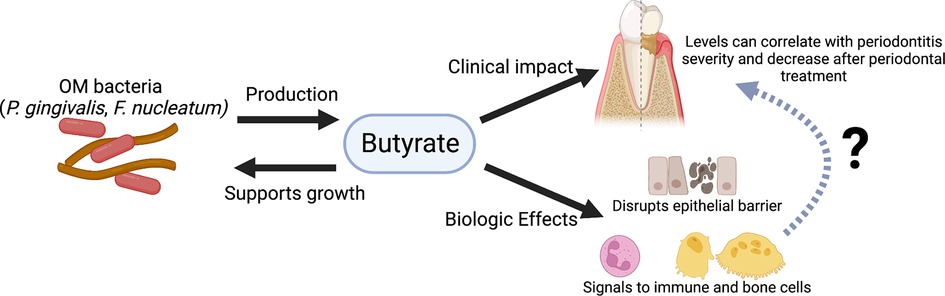
Figure 2 Graphical summary of evidence for butyrate's role in periodontitis. Periodontitis-associated bacteria found within the OM (e.g., P. gingivalis and F. nucleatum) produce butyrate, which in turn can support their growth. Clinical studies have shown an association between butyrate levels and periodontitis and found that butyrate levels decrease after periodontal treatment. Experimental studies indicate butyrate may disrupt the junctional epithelial barrier and can signal to immune and bone cells. However, the exact mechanisms connecting butyrate and its possible biologic effects to periodontitis and alveolar bone are still unknown. Figure created with BioRender.com.
Conceivably, OM-derived butyrate and other SCFAs signal to immune, epithelial, and stromal cells in periodontal tissues which could then interact with osteoblasts and osteoclasts. SCFAs appear to affect the ability of neutrophils to respond to the periodontal pathogen A. actinomycetemcomitans (99). Mice deficient in the SCFA receptor FFAR2 showed increased alveolar bone loss and decreased maxillary bone density, with the latter partially rescued by a high fiber diet (100). While osteoclasts derived from FFAR2-deficient mice showed increased in vitro differentiation, the only SCFA that could inhibit this activity was butyrate, indicating that butyrate acted independently of the FFAR2 receptor.
Clearly, further work is needed to identify how metabolites beyond SCFAs affect alveolar bone and to better understand how butyrate and other metabolites modulate alveolar bone metabolism through the oral mucosal immune response to OM biofilms. Additional questions inspired by the role of GM metabolites in bone health may provide insight. Do metabolites produced in the oral cavity act on the oral mucosal immune system similar to how the GM indirectly influences bone health? Do differences or similarities between the oral and gut niches underly the impact of oral metabolites on the alveolar bone? Answers to these and other questions, aided by advances in scientific techniques, may provide new options for diagnosing, treating, or preventing periodontitis and the associated loss of alveolar bone.
The path forward
The bulk of studies on periodontitis and alveolar bone thus far continue to focus on OM characterization through either 16S or whole genome shotgun sequencing approaches and interrogating the host immune response. Work investigating the biologically active small compounds that determine the net functional activity in the oral environment remains scarce. However, such investigations are beginning to emerge, enabled by technological advances in metabolomics. Indeed, recent studies have demonstrated that combining metabolomics with transcriptomics, 16S DNA genomics, and other unbiased techniques has potential for identifying new molecular pathways and therapeutic targets for periodontitis and alveolar bone loss (101–103).
In parallel, rigorous studies are required for determining the mechanisms behind oral metabolites and alveolar bone. The majority of existing studies on oral metabolites utilize in vitro models of homogeneous cells and/or bacterial populations. Such approaches have significant limitations in their ability to recapitulate the complex environment of subgingival biofilms, oral mucosal tissues, and underlying alveolar bone. Thus, carefully controlled animal studies should be designed to investigate the mechanisms behind host and bacterial metabolites and alveolar bone health.
The translation of findings on known or novel oral metabolites to effective therapies for maintaining alveolar bone face specific challenges in study design and analysis. Characterization and validation of possible targets for therapy will entail clinical studies with rigorous study design, careful cohort stratification, and inclusion and exclusion criteria to ensure application and reproducibility. Data integration and analysis with multi-omics approaches is challenging due to heterogeneity in the data format from each -omics technologies, discrepancies in annotation, and non-uniform missing data from different data. Additionally, the computational complexity and lack of standardization for analytical and bioinformatic pipelines may hinder reproducibility across studies. Thus, the introduction of standardized protocols for clinical studies and computational approaches, along with techniques to accommodate for data heterogeneity and missing data, are critical for the success of future work. With these tools in hand, an integrative multi-omics approach combining metabolomics, metagenomics, transcriptomics, and other -omics techniques may be able to resolve the interconnected roles of the OM and immune response in alveolar bone health and disease.
Data availability statement
The original contributions presented in the study are included in the article, further inquiries can be directed to the corresponding author.
Author contributions
DF and SMG: conceptualization, writing – original draft, writing – review and editing. SMG: funding acquisition. All authors contributed to the article and approved the submitted version.
Funding
DF was supported by intramural programs of the NIH/NIDCR (DIR OCD Clinical Research Fellowship grant no. DE000727). SMG was supported by an extramural grant NIH/NIDCR grant no. R03DE030527.
Conflict of interest
The authors declare that the research was conducted in the absence of any commercial or financial relationships that could be construed as a potential conflict of interest.
Publisher's note
All claims expressed in this article are solely those of the authors and do not necessarily represent those of their affiliated organizations, or those of the publisher, the editors and the reviewers. Any product that may be evaluated in this article, or claim that may be made by its manufacturer, is not guaranteed or endorsed by the publisher.
References
1. Sender R, Fuchs S, Milo R. Revised estimates for the number of human and Bacteria cells in the body. PLoS Biol. (2016) 14(8):e1002533. doi: 10.1371/journal.pbio.1002533
2. Turnbaugh PJ, Ley RE, Hamady M, Fraser-Liggett CM, Knight R, Gordon JI. The human microbiome project. Nat. (2007) 449(7164):804–10. doi: 10.1038/nature06244
3. Wensel CR, Pluznick JL, Salzberg SL, Sears CL. Next-generation sequencing: insights to advance clinical investigations of the microbiome. J Clin Invest. (2022) 132(7):e154944. doi: 10.1172/JCI154944
4. Runge S, Rosshart SP. The mammalian metaorganism: a holistic view on how microbes of all kingdoms and niches shape local and systemic immunity. Front Immunol. (2021) 12. doi: 10.3389/fimmu.2021.702378
5. Louca S, Polz MF, Mazel F, Albright MBN, Huber JA, O’Connor MI, et al. Function and functional redundancy in microbial systems. Nat Ecol Evol. (2018) 2(6):936–43. doi: 10.1038/s41559-018-0519-1
6. Suárez LJ, Arboleda S, Angelov N, Arce RM. Oral versus gastrointestinal mucosal immune niches in homeostasis and allostasis. Front Immunol. (2021) 12. doi: 10.3389/fimmu.2021.705206
7. Radaic A, Kapila YL. The oralome and its dysbiosis: new insights into oral microbiome-host interactions. Comput Struct Biotechnol J. (2021) 19:1335–60. doi: 10.1016/j.csbj.2021.02.010
8. Carding S, Verbeke K, Vipond DT, Corfe BM, Owen LJ. Dysbiosis of the gut microbiota in disease. Microb Ecol Health Dis. (2015) 26(1):26191. doi: 10.3402/mehd.v26.26191
9. Kumar PS. From focal sepsis to periodontal medicine: a century of exploring the role of the oral microbiome in systemic disease. J Physiol. (2017) 595(2):465–76. doi: 10.1113/JP272427
10. Scannapieco FA, Dongari-Bagtzoglou A. Dysbiosis revisited: understanding the role of the oral microbiome in the pathogenesis of gingivitis and periodontitis: a critical assessment. J Periodontol. (2021) 92(8):1071–8. doi: 10.1002/JPER.21-0120
11. Behera J, Ison J, Tyagi SC, Tyagi N. The role of gut microbiota in bone homeostasis. Bone. (2020) 135:115317. doi: 10.1016/j.bone.2020.115317
12. Cooney OD, Nagareddy PR, Murphy AJ, Lee MKS. Healthy gut, healthy bones: targeting the gut microbiome to promote bone health. Front Endocrinol. (2021) 11. doi: 10.3389/fendo.2020.620466
13. Nanci A, Bosshardt DD. Structure of periodontal tissues in health and disease. Periodontol. (2006) 40:11–28. doi: 10.1111/j.1600-0757.2005.00141.x
14. Jonasson G, Skoglund I, Rythén M. The rise and fall of the alveolar process: dependency of teeth and metabolic aspects. Arch Oral Biol. (2018) 96:195–200. doi: 10.1016/j.archoralbio.2018.09.016
15. Sodek J, McKee MD. Molecular and cellular biology of alveolar bone. Periodontol. (2000) 24(1):99–126. doi: 10.1034/j.1600-0757.2000.2240106.x
16. Bartold PM, Cantley MD, Haynes DR. Mechanisms and control of pathologic bone loss in periodontitis. Periodontol. (2010) 53(1):55–69. doi: 10.1111/j.1600-0757.2010.00347.x
17. Socransky SS, Haffajee AD, Cugini MA, Smith C, Kent RL Jr. Microbial complexes in subgingival plaque. J Clin Periodontol. (1998) 25(2):134–44. doi: 10.1111/j.1600-051X.1998.tb02419.x
18. Moutsopoulos NM, Konkel JE. Tissue-specific immunity at the oral mucosal barrier. Trends Immunol. (2018) 39(4):276–87. doi: 10.1016/j.it.2017.08.005
19. Ikeuchi T, Moutsopoulos NM. Osteoimmunology in periodontitis; a paradigm for Th17/IL-17 inflammatory bone loss. Bone. (2022) 167:116500. doi: 10.1016/j.bone.2022.116500
20. Yu B, Wang C-Y. Osteoporosis and periodontal diseases – an update on their association and mechanistic links. Periodontol. (2022) 89(1):99–113. doi: 10.1111/prd.12422
21. Blacher E, Levy M, Tatirovsky E, Elinav E. Microbiome-modulated metabolites at the interface of host immunity. J Immun. (2017) 198(2):572–80. doi: 10.4049/jimmunol.1601247
22. Kelly B, Pearce EL. Amino assets: how amino acids support immunity. Cell Metab. (2020) 32(2):154–75. doi: 10.1016/j.cmet.2020.06.010
23. Karner CM, Long F. Glucose metabolism in bone. Bone. (2018) 115:2–7. doi: 10.1016/j.bone.2017.08.008
24. Martínez-Reyes I, Chandel NS. Mitochondrial TCA cycle metabolites control physiology and disease. Nat Commun. (2020) 11(1):102. doi: 10.1038/s41467-019-13668-3
25. Oliphant K, Allen-Vercoe E. Macronutrient metabolism by the human gut microbiome: major fermentation by-products and their impact on host health. Microbiome. (2019) 7(1):91. doi: 10.1186/s40168-019-0704-8
26. Krautkramer KA, Fan J, Bäckhed F. Gut microbial metabolites as multi-kingdom intermediates. Nat Rev Microbiol. (2021) 19(2):77–94. doi: 10.1038/s41579-020-0438-4
27. Kim CH. Immune regulation by microbiome metabolites. Immunol. (2018) 154(2):220–9. doi: 10.1111/imm.12930
28. Brown JM, Hazen SL. The gut microbial endocrine organ: bacterially derived signals driving cardiometabolic diseases. Annu Rev Med. (2015) 66(1):343–59. doi: 10.1146/annurev-med-060513-093205
29. Dasgupta S, Erturk-Hasdemir D, Ochoa-Reparaz J, Reinecker H-C, Kasper Dennis L. Plasmacytoid dendritic cells mediate anti-inflammatory responses to a gut commensal molecule via both innate and adaptive mechanisms. Cell Host Microbe. (2014) 15(4):413–23. doi: 10.1016/j.chom.2014.03.006
30. Asano Y, Hiramoto T, Nishino R, Aiba Y, Kimura T, Yoshihara K, et al. Critical role of gut microbiota in the production of biologically active, free catecholamines in the gut lumen of mice. Am J Physiol Gastrointest Liver Physiol. (2012) 303(11):G1288–95. doi: 10.1152/ajpgi.00341.2012
31. Zhou T, Heianza Y, Chen Y, Li X, Sun D, DiDonato JA, et al. Circulating gut microbiota metabolite trimethylamine N-oxide (TMAO) and changes in bone density in response to weight loss diets: the POUNDS lost trial. Diabetes Care. (2019) 42(8):1365–71. doi: 10.2337/dc19-0134
32. Bauermeister A, Mannochio-Russo H, Costa-Lotufo LV, Jarmusch AK, Dorrestein PC. Mass spectrometry-based metabolomics in microbiome investigations. Nat Rev Microbiol. (2022) 20(3):143–60. doi: 10.1038/s41579-021-00621-9
33. Wishart DS, Guo A, Oler E, Wang F, Anjum A, Peters H, et al. HMDB 5.0: the human metabolome database for 2022. Nucleic Acids Res. (2022) 50(D1):D622–31. doi: 10.1093/nar/gkab1062
34. Heintz-Buschart A, Wilmes P. Human gut microbiome: function matters. Trends Microbiol. (2018) 26(7):563–74. doi: 10.1016/j.tim.2017.11.002
35. Greenhalgh K, Meyer KM, Aagaard KM, Wilmes P. The human gut microbiome in health: establishment and resilience of microbiota over a lifetime. Environ Microbiol. (2016) 18(7):2103–16. doi: 10.1111/1462-2920.13318
36. Turner JR. Intestinal mucosal barrier function in health and disease. Nat Rev Immunol. (2009) 9(11):799–809. doi: 10.1038/nri2653
37. Martens EC, Neumann M, Desai MS. Interactions of commensal and pathogenic microorganisms with the intestinal mucosal barrier. Nat Rev Microbiol. (2018) 16(8):457–70. doi: 10.1038/s41579-018-0036-x
38. Belkaid Y, Harrison OJ. Homeostatic immunity and the microbiota. Immunity. (2017) 46(4):562–76. doi: 10.1016/j.immuni.2017.04.008
39. Evans JM, Morris LS, Marchesi JR. The gut microbiome: the role of a virtual organ in the endocrinology of the host. J Endocrinol. (2013) 218(3):R37–47. doi: 10.1530/JOE-13-0131
40. Ohlsson C, Nigro G, Boneca IG, Bäckhed F, Sansonetti P, Sjögren K. Regulation of bone mass by the gut microbiota is dependent on NOD1 and NOD2 signaling. Cell Immunol. (2017) 317:55–8. doi: 10.1016/j.cellimm.2017.05.003
41. Sjögren K, Engdahl C, Henning P, Lerner UH, Tremaroli V, Lagerquist MK, et al. The gut microbiota regulates bone mass in mice. J Bone Miner Res. (2012) 27(6):1357–67. doi: 10.1002/jbmr.1588
42. Guss JD, Horsfield MW, Fontenele FF, Sandoval TN, Luna M, Apoorva F, et al. Alterations to the gut microbiome impair bone strength and tissue material properties. J Bone Miner Res. (2017) 32(6):1343–53. doi: 10.1002/jbmr.3114
43. Guss JD, Taylor E, Rouse Z, Roubert S, Higgins CH, Thomas CJ, et al. The microbial metagenome and bone tissue composition in mice with microbiome-induced reductions in bone strength. Bone. (2019) 127:146–54. doi: 10.1016/j.bone.2019.06.010
44. Ervin SM, Li H, Lim L, Roberts LR, Liang X, Mani S, et al. Gut microbial β-glucuronidases reactivate estrogens as components of the estrobolome that reactivate estrogens. J Biol Chem. (2019) 294(49):18586–99. doi: 10.1074/jbc.RA119.010950
45. Li JY, Chassaing B, Tyagi AM, Vaccaro C, Luo T, Adams J, et al. Sex steroid deficiency-associated bone loss is microbiota dependent and prevented by probiotics. J Clin Invest. (2016) 126(6):2049–63. doi: 10.1172/JCI86062
46. Yu M, Pal S, Paterson CW, Li J-Y, Tyagi AM, Adams J, et al. Ovariectomy induces bone loss via microbial-dependent trafficking of intestinal TNF+ T cells and Th17 cells. J Clin Invest. (2021) 131(4):e143137. doi: 10.1172/JCI143137
47. Yano JM, Yu K, Donaldson GP, Shastri GG, Ann P, Ma L, et al. Indigenous bacteria from the gut microbiota regulate host serotonin biosynthesis. Cell. (2015) 161(2):264–76. doi: 10.1016/j.cell.2015.02.047
48. Ridlon JM, Kang D-J, Hylemon PB. Bile salt biotransformations by human intestinal bacteria. J Lipid Res. (2006) 47(2):241–59. doi: 10.1194/jlr.R500013-JLR200
49. Yadav VK, Balaji S, Suresh PS, Liu XS, Lu X, Li Z, et al. Pharmacological inhibition of gut-derived serotonin synthesis is a potential bone anabolic treatment for osteoporosis. Nat Med. (2010) 16(3):308–12. doi: 10.1038/nm.2098
50. Ishizawa M, Akagi D, Makishima M. Lithocholic acid is a vitamin D receptor ligand that acts preferentially in the ileum. Int J Mol Sci. (2018) 19(7):1975. doi: 10.3390/ijms19071975
51. Brighton CA, Rievaj J, Kuhre RE, Glass LL, Schoonjans K, Holst JJ, et al. Bile acids trigger GLP-1 release predominantly by accessing basolaterally located G protein-coupled bile acid receptors. Endocrinol. (2015) 156(11):3961–70. doi: 10.1210/en.2015-1321
52. Wallace JL, Motta J-P, Buret AG. Hydrogen sulfide: an agent of stability at the microbiome-mucosa interface. Am J Physiol Gastrointest Liver Physiol. (2018) 314(2):G143–9. doi: 10.1152/ajpgi.00249.2017
53. Liu Y, Yang R, Liu X, Zhou Y, Qu C, Kikuiri T, et al. Hydrogen sulfide maintains mesenchymal stem cell function and bone homeostasis via regulation of Ca2+ channel sulfhydration. Cell Stem Cell. (2014) 15(1):66–78. doi: 10.1016/j.stem.2014.03.005
54. Grassi F, Tyagi AM, Calvert JW, Gambari L, Walker LD, Yu M, et al. Hydrogen sulfide is a novel regulator of bone formation implicated in the bone loss induced by estrogen deficiency. J Bone Miner Res. (2016) 31(5):949–63. doi: 10.1002/jbmr.2757
55. Li L, Rao S, Cheng Y, Zhuo X, Deng C, Xu N, et al. Microbial osteoporosis: the interplay between the gut microbiota and bones via host metabolism and immunity. Microbiologyopen. (2019) 8(8):e00810. doi: 10.1002/mbo3.810
56. Zaiss MM, Jones RM, Schett G, Pacifici R. The gut-bone axis: how bacterial metabolites bridge the distance. J Clin Invest. (2019) 129(8):3018–28. doi: 10.1172/JCI128521
57. Chen T-H, Chen W-M, Hsu K-H, Kuo C-D, Hung S-C. Sodium butyrate activates ERK to regulate differentiation of mesenchymal stem cells. Biochem Biophys Res Commun. (2007) 355(4):913–8. doi: 10.1016/j.bbrc.2007.02.057
58. Lucas S, Omata Y, Hofmann J, Böttcher M, Iljazovic A, Sarter K, et al. Short-chain fatty acids regulate systemic bone mass and protect from pathological bone loss. Nat Commun. (2018) 9(1):55. doi: 10.1038/s41467-017-02490-4
59. Yan J, Herzog Jeremy W, Tsang K, Brennan Caitlin A, Bower Maureen A, Garrett Wendy S, et al. Gut microbiota induce IGF-1 and promote bone formation and growth. Proc Natl Acad Sci USA. (2016) 113(47):E7554–63. doi: 10.1073/pnas.1607235113
60. Smith PM, Howitt MR, Panikov N, Michaud M, Gallini CA, Bohlooly-y M, et al. The microbial metabolites, short-chain fatty acids, regulate colonic treg cell homeostasis. Science. (2013) 341(6145):569–73. doi: 10.1126/science.1241165
61. Fischer L, Herkner C, Kitte R, Dohnke S, Riewaldt J, Kretschmer K, et al. Foxp3(+) regulatory T cells in bone and hematopoietic homeostasis. Front Endocrinol. (2019) 10:578. doi: 10.3389/fendo.2019.00578
62. Tyagi AM, Yu M, Darby TM, Vaccaro C, Li JY, Owens JA, et al. The microbial metabolite butyrate stimulates bone formation via T regulatory cell-mediated regulation of WNT10B expression. Immunity. (2018) 49(6):1116–31.e7. doi: 10.1016/j.immuni.2018.10.013
63. Li JY, Yu M, Pal S, Tyagi AM, Dar H, Adams J, et al. Parathyroid hormone-dependent bone formation requires butyrate production by intestinal microbiota. J Clin Invest. (2020) 130(4):1767–81. doi: 10.1172/JCI133473
64. Collins FL, Rios-Arce ND, Schepper JD, Parameswaran N, McCabe LR, Britton RA, et al. The potential of probiotics as a therapy for osteoporosis. Microbiol Spectr. (2017) 5(4):5.4.20. doi: 10.1128/microbiolspec.BAD-0015-2016
65. Li P, Sundh D, Ji B, Lappa D, Ye L, Nielsen J, et al. Metabolic alterations in older women with low bone mineral density supplemented with lactobacillus reuteri. JBMR Plus. (2021) 5(4):e10478. doi: 10.1002/jbm4.10478
66. Whisner CM, Weaver CM. Prebiotics and bone. In: McCabe LR, Parameswaran N, editors. Understanding the gut-bone signaling axis: mechanisms and therapeutic implications. Cham: Springer International Publishing (2017). p. 201–24.
67. Sanders ME, Merenstein DJ, Reid G, Gibson GR, Rastall RA. Probiotics and prebiotics in intestinal health and disease: from biology to the clinic. Nat Rev Gastroenterol Hepatol. (2019) 16(10):605–16. doi: 10.1038/s41575-019-0173-3
68. Olsen I, Yamazaki K. Can oral bacteria affect the microbiome of the gut? J Oral Microbiol. (2019) 11(1):1586422. doi: 10.1080/20002297.2019.1586422
69. Kitamoto S, Nagao-Kitamoto H, Hein R, Schmidt TM, Kamada N. The bacterial connection between the oral cavity and the gut diseases. J Dent Res. (2020) 99(9):1021–9. doi: 10.1177/0022034520924633
70. Kitamoto S, Kamada N. Periodontal connection with intestinal inflammation: microbiological and immunological mechanisms. Periodontol. (2022) 89(1):142–53. doi: 10.1111/prd.12424
71. Said HS, Suda W, Nakagome S, Chinen H, Oshima K, Kim S, et al. Dysbiosis of salivary microbiota in inflammatory bowel disease and its association with oral immunological biomarkers. DNA Res. (2014) 21(1):15–25. doi: 10.1093/dnares/dst037
72. Atarashi K, Suda W, Luo C, Kawaguchi T, Motoo I, Narushima S, et al. Ectopic colonization of oral bacteria in the intestine drives TH1 cell induction and inflammation. Science. (2017) 358(6361):359–65. doi: 10.1126/science.aan4526
73. Kitamoto S, Nagao-Kitamoto H, Jiao Y, Gillilland MG, Hayashi A, Imai J, et al. The intermucosal connection between the mouth and gut in commensal pathobiont-driven colitis. Cell. (2020) 182(2):447–62.e14. doi: 10.1016/j.cell.2020.05.048
74. Hathaway-Schrader JD, Carson MD, Gerasco JE, Warner AJ, Swanson BA, Aguirre JI, et al. Commensal gut bacterium critically regulates alveolar bone homeostasis. Lab Invest. (2022) 102(4):363–75. doi: 10.1038/s41374-021-00697-0
75. Kato T, Yamazaki K, Nakajima M, Date Y, Kikuchi J, Hase K, et al. Oral administration of porphyromonas gingivalis alters the gut microbiome and Serum metabolome. mSphere. (2018) 3(5):e00460–18. doi: 10.1128/mSphere.00460-18
76. Simas AM, Kramer CD, Weinberg EO, Genco CA. Oral infection with a periodontal pathogen alters oral and gut microbiomes. Anaerobe. (2021) 71:102399. doi: 10.1016/j.anaerobe.2021.102399
77. Kobayashi R, Ogawa Y, Hashizume-Takizawa T, Kurita-Ochiai T. Oral bacteria affect the gut microbiome and intestinal immunity. Pathog Dis. (2020) 78(3). doi: 10.1093/femspd/ftaa024
78. Jia L, Tu Y, Jia X, Du Q, Zheng X, Yuan Q, et al. Probiotics ameliorate alveolar bone loss by regulating gut microbiota. Cell Prolif. (2021) 54(7):e13075. doi: 10.1111/cpr.13075
79. Sato K, Yamazaki K, Kato T, Nakanishi Y, Tsuzuno T, Yokoji-Takeuchi M, et al. Obesity-related gut microbiota aggravates alveolar bone destruction in experimental periodontitis through elevation of uric acid. mBio. (2021) 12(3):e00771–21. doi: 10.1128/mBio.00771-21
80. Barbour A, Elebyary O, Fine N, Oveisi M, Glogauer M. Metabolites of the oral microbiome: important mediators of multikingdom interactions. FEMS Microbiol Rev. (2022) 46(1). doi: 10.1093/femsre/fuab039
82. Baima G, Iaderosa G, Citterio F, Grossi S, Romano F, Berta GN, et al. Salivary metabolomics for the diagnosis of periodontal diseases: a systematic review with methodological quality assessment. Metabolomics. (2021) 17(1):1. doi: 10.1007/s11306-020-01754-3
83. Baima G, Corana M, Iaderosa G, Romano F, Citterio F, Meoni G, et al. Metabolomics of gingival crevicular fluid to identify biomarkers for periodontitis: a systematic review with meta-analysis. J Periodontal Res. (2021) 56(4):633–45. doi: 10.1111/jre.12872
84. Liebsch C, Pitchika V, Pink C, Samietz S, Kastenmüller G, Artati A, et al. The saliva metabolome in association to oral health Status. J Dent Res. (2019) 98(6):642–51. doi: 10.1177/0022034519842853
85. Sakanaka A, Kuboniwa M, Hashino E, Bamba T, Fukusaki E, Amano A. Distinct signatures of dental plaque metabolic byproducts dictated by periodontal inflammatory status. Sci Rep. (2017) 7(1):42818. doi: 10.1038/srep42818
86. Pei J, Li F, Xie Y, Liu J, Yu T, Feng X. Microbial and metabolomic analysis of gingival crevicular fluid in general chronic periodontitis patients: lessons for a predictive, preventive, and personalized medical approach. EPMA Journal. (2020) 11(2):197–215. doi: 10.1007/s13167-020-00202-5
87. Barnes VM, Kennedy AD, Panagakos F, Devizio W, Trivedi HM, Jönsson T, et al. Global metabolomic analysis of human Saliva and plasma from healthy and diabetic subjects, with and without periodontal disease. PLoS One. (2014) 9(8):e105181. doi: 10.1371/journal.pone.0105181
88. Lohinai Z, Keremi B, Szoko E, Tabi T, Szabo C, Tulassay Z, et al. Bacterial lysine decarboxylase influences human dental biofilm lysine content, biofilm accumulation, and subclinical gingival inflammation. J Periodontol. (2012) 83(8):1048–56. doi: 10.1902/jop.2011.110474
89. Yamada M, Takahashi N, Matsuda Y, Sato K, Yokoji M, Sulijaya B, et al. A bacterial metabolite ameliorates periodontal pathogen-induced gingival epithelial barrier disruption via GPR40 signaling. Sci Rep. (2018) 8(1):9008. doi: 10.1038/s41598-018-27408-y
90. Cueno ME, Ochiai K. Re-discovering periodontal butyric acid: new insights on an old metabolite. Microb Pathog. (2016) 94:48–53. doi: 10.1016/j.micpath.2015.10.006
91. Guan X, Li W, Meng H. A double-edged sword: role of butyrate in the oral cavity and the gut. Mol Oral Microbiol. (2021) 36(2):121–31. doi: 10.1111/omi.12322
92. Kurita-Ochiai T, Fukushima K, Ochiai K. Volatile fatty acids, metabolic by-products of periodontopathic bacteria, inhibit lymphocyte proliferation and cytokine production. J Dent Res. (1995) 74(7):1367–73. doi: 10.1177/00220345950740070801
93. Pöllänen MT, Overman DO, Salonen JI. Bacterial metabolites sodium butyrate and propionate inhibit epithelial cell growth in vitro. J Periodontal Res. (1997) 32(3):326–34. doi: 10.1111/j.1600-0765.1997.tb00541.x
94. Niederman R, Buyle-Bodin Y, Lu BY, Robinson P, Naleway C. Short-chain carboxylic acid concentration in human gingival crevicular fluid. J Dent Res. (1997) 76(1):575–9. doi: 10.1177/00220345970760010801
95. Lu R, Meng H, Gao X, Xu L, Feng X. Effect of non-surgical periodontal treatment on short chain fatty acid levels in gingival crevicular fluid of patients with generalized aggressive periodontitis. J Periodontal Res. (2014) 49(5):574–83. doi: 10.1111/jre.12137
96. Dallas R, Leonard B, Wali A, Bigler R, Sun W, Bultman Scott J. The warburg effect dictates the mechanism of butyrate-mediated histone acetylation and cell proliferation. Mol Cell. (2012) 48(4):612–26. doi: 10.1016/j.molcel.2012.08.033
97. Liu J, Wang Y, Meng H, Yu J, Lu H, Li W, et al. Butyrate rather than LPS subverts gingival epithelial homeostasis by downregulation of intercellular junctions and triggering pyroptosis. J Clin Periodontol. (2019) 46(9):894–907. doi: 10.1111/jcpe.13162
98. Magrin GL, Strauss FJ, Benfatti CA, Maia LC, Gruber R. Effects of short-chain fatty acids on human oral epithelial cells and the potential impact on periodontal disease: a systematic review of in vitro studies. Int J Mol Sci. (2020) 21(14):4895. doi: 10.3390/ijms21144895
99. Corrêa RO, Vieira A, Sernaglia EM, Lancellotti M, Vieira AT, Avila-Campos MJ, et al. Bacterial short-chain fatty acid metabolites modulate the inflammatory response against infectious bacteria. Cell Microbiol. (2017) 19(7):e12720. doi: 10.1111/cmi.12720
100. Montalvany-Antonucci CC, Duffles LF, de Arruda JAA, Zicker MC, de Oliveira S, Macari S, et al. Short-chain fatty acids and FFAR2 as suppressors of bone resorption. Bone. (2019) 125:112–21. doi: 10.1016/j.bone.2019.05.016
101. Overmyer KA, Rhoads TW, Merrill AE, Ye Z, Westphall MS, Acharya A, et al. Proteomics, lipidomics, metabolomics, and 16S DNA sequencing of dental plaque from patients with diabetes and periodontal disease. Mol Cell Proteomics. (2021) 20:100126. doi: 10.1016/j.mcpro.2021.100126
102. Califf KJ, Schwarzberg-Lipson K, Garg N, Gibbons Sean M, Caporaso JG, Slots J, et al. Multi-omics analysis of periodontal pocket microbial communities pre- and posttreatment. mSystems. (2017) 2(3):e00016–17. doi: 10.1128/mSystems.00016-17
Keywords: alveolar bone, oral microbiome, gut microbiome, metabolites, periodontitis, osteoporosis
Citation: Fraser D and Ganesan SM (2023) Microbiome, alveolar bone, and metabolites: Connecting the dots. Front. Dent. Med 3: 1074339. doi: 10.3389/fdmed.2022.1074339
Received: 19 October 2022; Accepted: 20 December 2022;
Published: 10 January 2023.
Edited by:
Francisco Nociti, Universidade Estadual de Campinas, BrazilReviewed by:
Marcelo Henrique Napimoga, São Leopoldo Mandic School, BrazilRenato Correa Viana Casarin, Universidade Estadual de Campinas, Brazil
© 2023 Fraser and Ganesan. This is an open-access article distributed under the terms of the Creative Commons Attribution License (CC BY). The use, distribution or reproduction in other forums is permitted, provided the original author(s) and the copyright owner(s) are credited and that the original publication in this journal is cited, in accordance with accepted academic practice. No use, distribution or reproduction is permitted which does not comply with these terms.
*Correspondence: Sukirth M. Ganesan, sukirth-ganesan@uiowa.edu
Specialty Section: This article was submitted to Systems Integration, a section of the journal Frontiers in Dental Medicine