- 1Division of Cariology and Endodontology, CUMD—University Clinics of Dental Medicine, Faculty of Medicine, University of Geneva, Geneva, Switzerland
- 2Department of Political Sciences, University of Genoa, Genoa, Italy
- 3Department of Dental Material Sciences, Academic Center for Dentistry Amsterdam (ACTA), University of Amsterdam and Vrije Universiteit Amsterdam, Amsterdam, Netherlands
Introduction: The aim of the present study was to assess the generation of laser-activated irrigation (LAI) with a 9,300-nm CO2 laser, a 455- and 970-nm diode laser, as well as an 810- and 980-nm diode laser with and without carbon-coated “hot tips” for improved irrigation in endodontics. Therefore, the formation of cavitation bubbles at the laser tip ends in different liquids was evaluated and compared with the traditionally applied Er:YAG laser.
Methods: The CO2 laser was applied at different power settings at a repetition rate of 14 Hz, SIROLASE for each wavelength at 10% Duty Cycle, and WISER with both wavelengths at different power settings and wavelengths. The LAI was videotaped with a high-speed camera. To measure the streaming velocity due to cavitation, all lasers were loosely coated with carbon particles and activated in pure water. Then, due to LAI, moving particles were videotaped with the same high-speed camera setup. To determine the streaming velocities, the movement of one carbon particle was tracked with the help of a measuring tool in ImageJP software and streaming velocities were calculated.
Results: With both diode laser devices at four wavelengths, it was only possible to introduce clear cavitation bubbles with low-power parameters (180 mW for the SIROLASE and 200 mW average power for the WISER laser), with the black coating of the laser tips. The 9,300-nm CO2 laser produced clear cavitation bubbles with all tested parameters. Consequently, all tested lasers produced streaming of the liquid, with decreasing velocities at increasing distances from the laser tip: Er:YAG laser 6.4 m/s, CO2 laser up to 5 m/s, and diode lasers in the range of 0.01–0.09 m/s. LIA with the Er:YAG and 9,300-nm CO2 lasers generated high and similar streaming velocities. The diode lasers tested generated significantly lower streaming velocities.
Introduction
A successful root canal treatment depends on the creation and maintenance of a root free from micro-organisms and debris. Achieving this goal is complex, as the root canal wall is made up of millions of dentinal tubules that are, in case a root canal treatment is required, often contaminated with micro-organisms and the remnants of dead cells (1). Besides widening the root canal with files and reamers, the chemical and mechanical cleaning of the tubules is a quality-determining factor for the success of this treatment (2, 3). To date, several methods have been developed and investigated to improve the chemical and mechanical streaming actions of irrigants via ultrasonic or laser-activated irrigation (LAI) (4).
The effect of LAI is based on cavitation, a physical phenomenon in which the static pressure of a liquid reduces to below the liquid's vapor pressure, leading to the formation of small vapor-filled cavities in the liquid. The laser-emitted light is absorbed in a thin superficial layer (∼1 μm thick) of the irrigant around the tip. The irrigant is then superheated over its boiling point, resulting in the formation of vapor and bubbles. The bubbles expand until the pressure in the bubble reaches that of the surrounding liquid, finally leading to a collapse of the bubble that causes shock waves, which, in turn, enhance the cleaning effect of the irrigant (5–9). The implosion of the vapor bubble creates a pressure wave, leading to streaming of the liquid with velocities up to 20 m/s (5, 6). The resulting forces and shear stress on the dentinal walls due to the streaming of the irrigant are sufficient to pump the tissue debris out of the canal and remove the smear layer and biofilm from the surfaces (5, 8). These effects are directly dependent on the energy and pulse duration parameters of the laser system, which modulate the time of vapor formation, as well as the size and life cycle of the vapor bubbles (5, 7).
For LAI, the wavelength on which a laser operates and its applied power is of importance as the absorption coefficient of the irrigating liquid is wavelength dependent, while the power influences the amount of cavitation. Until now, wavelengths of 1,000–3,000 nm have been used (6, 10, 11). The Er:YAG laser has been of particular interest due to its wavelength (2,094 nm), which allows a very powerful interaction with water (absorption coefficient 1.2 × 104/cm) (12).
Recently, CO2 lasers with new wavelengths have been successfully developed with an absorption coefficient in the range of 6 × 102/cm. The 9,300-nm wavelength has gained attention for its possibility to ablate dental soft and hard tissues (13). As this wavelength is strongly absorbed in hydroxyapatite and water, it might also be suitable for the LAI technique. To the authors' best knowledge, there is no existing literature on cavitation capacities and possible applications in LAI with the 9,300-nm CO2 laser.
Another group of lasers, diode lasers with visible and near-IR wavelengths, has already been well described in endodontics (10). Their classic indication in the endodontic treatment process is photothermal disinfection and activation of the chemical action of irrigants, such as sodium hypochlorite (NaOCl) and ethylenediaminetetraacetic acid (EDTA) (14, 15). To date, there is only limited literature on the application of near-IR diode lasers in the mechanical activation of liquids, mainly concentrating on the 940- and 980-nm wavelengths (absorption coefficient of approximately 9 × 10−1/cm), as they are much more absorbed in water than other available near-IR diode wavelengths (16), which act more as heaters of the irrigant than creating direct cavitation. Comparatively high-power settings and very long pulses must be applied for the formation of vapor bubbles (17). They are likely to aversively interact with the dentinal tissues of the root and possibly even with the surrounding periodontium (18, 19).
In their aim to make the commonly used diode laser with wavelengths in the range of 400–1,000 nm suitable for LAI with low-power settings, the authors intended to improve their function as heaters in the present study by coating the laser tip black. This “hot-tip” method has already been applied in laser surgery, where different wavelengths of the diode lasers are applied to tissues with attributes that do not compulsorily coincide with the laser wavelength (20, 21). The literature explains this as the “hot-tip phenomena.” In this context, the laser does not cut optically with photons but with its heated fiber as an electrocautery. For this aim, the transparent glass tip must be charred so that the laser light is absorbed in the black color of the char and heats the tip that can now burn and cut the tissue in contact (22).
The aim of this study was to evaluate the mechanism of laser-activated irrigation (LAI) for a 9,300-nm CO2 laser and for diode lasers with and without carbon-coated hot tips at different laser wavelengths. Hereby diode lasers (455, 810, 980, and 970 nm) and a CO2 laser (9,300 nm) were compared with the traditionally applied Er:YAG laser with regard to the formation of vapor bubbles at the laser tip ends in different liquids and the ability of liquid streaming due to cavitation.
The first hypothesis was that the 9,300 nm CO2 laser and low-power diode lasers would produce cavitation in water-based irrigants, and much more so with increasing laser power settings (energy and pulse duration), and that the hot-tip method will allow all diode lasers on cavitation independently on their wavelengths and the irrigant’s color.
The second null hypothesis was that concerning the irrigant streaming due to cavitation; both the CO2 laser and the diode lasers lead to the streaming of water at higher velocities with increasing laser power settings for both types of lasers.
The third null hypothesis concerned a similar performance of the tested diode laser wavelengths, but in all applications inferior to the CO2 laser. We supposed that the CO2 laser would be similar to the Er:YAG laser in terms of cavitation and the resulting streaming velocities in water with distinct laser parameters.
Materials and methods
Evaluation of the potential for cavitation development with CO2 and diode lasers
Bubble development as a measure of cavitation potential
The potential for cavitation development in a water-based liquid with a 9,300-nm CO2 laser (Solea; Convergent Dental, Inc., Natick, MA, United States) and diode lasers (Wiser Doctor Smile 808 and 980 nm; Lambda SpA, Brendola, Italy; and SIROLASE 455 and 970 nm; Dentsply Sirona Deutschland GmbH, Bensheim, Germany) was evaluated in terms of the development of vapor bubbles. For the diode lasers, wavelengths of 455, 808, 970, and 980 nm were applied with the original transparent laser tip (without coating) in pure water. For the diode lasers working at 455 and 808 nm (visible range), we tested the development of bubbles with dyed water, dependent on their absorption capacities: the SIROLASE laser (455 nm) in an orange dye (E110 food coloring; Trawosa, St. Gallen, Switzerland) and the WISER laser (808 nm) in a green dye (ICG-Pulsion; Pulsion Medical Systems, Munich, Germany).
To study the effect of a hot tip, all laser tips of the diode lasers were black coated with carbon particles (Carbon black, acetylene 100%, compressed, 99.9+%, Alfa Aesar, Kandel, Germany) that were glued to the tip end with a transparent glue spray (Toolcraft, Conrad Electronic AG, Wollerau, Switzerland) and activated again in pure water. Four wavelengths (455, 808, 970, and 980 nm) were tested with a laser tip inserted 5 mm into a glass cartridge (1.7 ml Ubistesin, 3M ESPE, Seefeld, Germany) filled with the corresponding liquid. The development of bubbles was evaluated by light microscopy (16X, A 60; Leica, Heerbrugg, Switzerland) and consequently photographed (VHX-S550E microscope; Keyence Corporation, Osaka, Japan).
Assessing the effects of power settings on the cavity quality (bubble morphology and size)
The second part of this study concentrated on the bubble morphology and size at various wavelengths and tip coatings of diode lasers, the Er:YAG and 9,300-nm CO2 lasers, in pure water. The influence of different power settings with the diode lasers (with black-coated tips) and 9,300-nm CO2 laser on bubble morphology and size was compared with the Er:YAG laser cavitation with uncoated tips. The Er:YAG and 9,300-nm CO2 lasers (with uncoated tips) created bubbles that appeared and disappeared in very short time ranges, so that imaging of the primary bubbles required a high-speed capturing device, while streaming and secondary bubbles were visible under a light microscope. The laser tips were immersed 5 mm in pure water in a plexiglass cuboid (10 mm × 10 mm × 23 mm) and activated. The evaluation was performed using a high-speed camera (MotionXtra HG-100 K; Redlake, San Diego, CA, United States; Videal, Serial No. 150176), applying a frame rate of 5,175 Hz and an exposure time of 190 µs. For an adequate illumination of the setup, we used two lights mounted on arms (Intralux 5100, 200 W; Volpiag, Schlieren, Switzerland). A summary of all laser parameters is presented in Table 1.
Evaluation of the effectiveness of cavitation of CO2 and diode lasers
Particle velocity (streaming) was measured to assess the effectiveness of LAI and consequent cavitation effects. To visualize liquid movements during laser activation, we used the above-described setting of high-speed imaging to track carbon particles in pure water that was activated with lasers with the parameters described above (see Table 1). The same transparent plexiglass cuboid was filled with a 4% surfactant distilled water solution (TritonTM X-100, nonionic, aqueous solution; Sigma-Aldrich, Buch, Switzerland), allowing the immersion of carbon powder particles in water. Each laser tip was loosely coated in carbon powder and was then introduced into the liquid-filled cuboid. The activation was repeated five times per experimental group. The image sequences of one activation (and nine frames) were imported to “Image J 1.53e” (Wayne Rasband and contributors, National Institutes of Health, United States) and one carbon particle traced for each activation. The distances of the position of this special particle between each frame (nine frames per activation) were calculated with a measuring tool in ImageJP software (ImageJ 1.52 k; Wayne Rasband, National Institutes of Health, United States). In groups 1–5, the frame length was set to a duration of 193 µs. To determine the velocity of the movements of carbon powder particles due to laser activation, we considered the next eight frames for the analysis, resulting in a time lapse of 1,544 µs. Due to slower particle movements with diode lasers (groups 6–17), we considered every 20th frame, resulting in a time lapse of 30,460 µs.
Statistics
The statistical analysis was performed using repeated measures ANOVA and with speed as the repeated dependent variable and the lasers as factors. The usual assumption required to run ANOVA was checked using the Kolmogorov–Smirnov normality test and Levene's test for homogeneity of variances. A ranking of lasers was obtained using Fisher's LSD post-hoc test to evaluate the pairwise group. The level of significance was set at 0.05. The analyses were run in Statistica 13 software (Tibco Co).
Results
Potential for cavitation development with diode and CO2 lasers
In pure water, with a non-modified original laser tip, no diode lasers produced any clear vapor bubble up to the maximal possible power parameters. With the 810-nm wavelength, no effects on the water were visible, while with the 455-nm wavelength, a slight movement of the liquid was detected, but there was no bubble formation even with higher energy inputs starting with 3 W (Figure 1, middle). With the 970- and 980-nm wavelengths, there was no formation of vapor bubbles with the transparent tip in the water. However, a slight movement of liquid was observed in front of the tip with 1 W for the 980-nm laser and 200 mW for the 970-nm laser, which increased with increasing power.
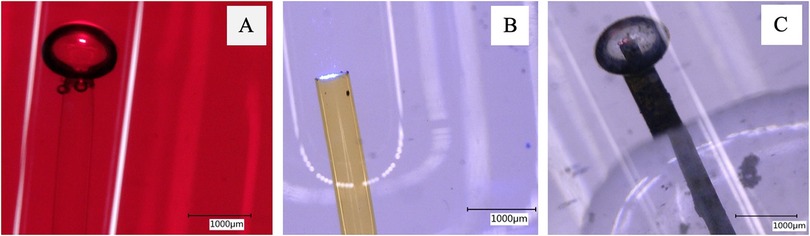
Figure 1 LAI with a diode laser dependent on tip coating and different colors of liquids. Left to right image, absorption of 455 nm SIROLASE @ 3 W, 90%, 1 Hz avg power 2.7 W in an orange dye (A) and water (B) without coating as well as with black carbon coating in water (C). LAI, laser-activated irrigation.
When coloring the water with the laser wavelength’s corresponding dyes (455 and 808 nm), the non-modified original laser tip produced clear vapor bubbles for both wavelengths equally. The 455-nm laser produced vapor bubbles in an orange dye starting with low-energy inputs and manifesting a clear vapor bubble with just 200 mW (average power 180 mW) and a comparatively large bubble with 3 W (average 2.7 W) (Figure 1, left). We observed similar phenomena with the 808-nm laser. We started seeing the movement of liquid and bubbles with an average power of 200 mW in Indocyanine green.
With black-coated laser tips for all four wavelengths we observed clear vapor bubbles. With the 455- and 808-nm wavelengths, similar vapor bubbles were produced without tip coating in dye and with hot-tip coating in pure water with 200 mW (see Figure 1, right and left). The same applied for the 970- and 980-nm wavelengths.
The vapor bubbles introduced by the diode lasers (tip diameters: 0.2 mm; surface: 0.0314 mm2) were round in shape and much smaller than those produced by the ER:YAG and CO2 lasers (Figure 2). Their size continuously grew over a period of several frames (10–12 ×193 μs) and then stayed static until the end of the laser pulse.
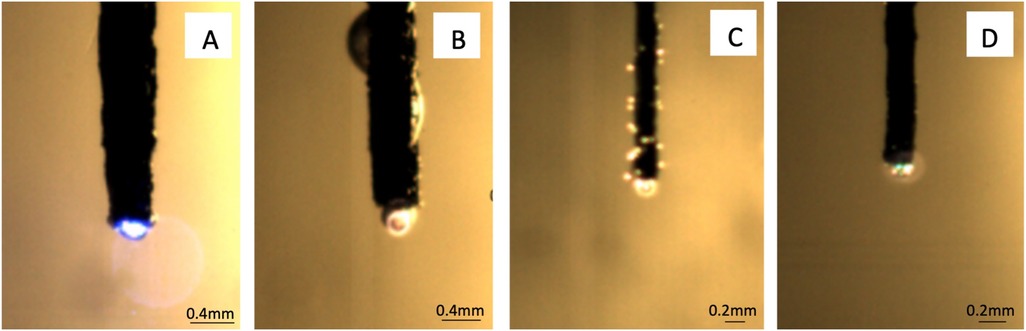
Figure 2 Activated laser tips submerged in deionized water; the frame length is 3,860 μs for diode lasers. (A) SIROLASE 455 nm with 67% DC, 0.8 W, 15 Hz; (B) SIROLASE 970 nm with 67% DC, 0.8 W, 15 Hz; (C) WISER 808 nm with 1 W, 15 Hz, 67%; (D) WISER 980 nm with 1 W, 15 Hz, 67%.
The Er:YAG laser, with a flat tip with a diameter of 1.3 mm (surface: 1.33 mm2) and energy of 50 mJ and 15 Hz, produced large and clearly visible bubbles in pure water in the range of two frames (386 μs) (see Figure 3A). The 9,300 nm CO2 laser, at the 40% and 60% power settings and with a laser tip diameter of 1.25 mm (surface: 1.227 mm2 and flat tip), produced bubbles of comparable sizes to the Er:YAG laser that were in the same time range (Figures 3D,E). With the 10% and 20% power settings (Figures 3B,C), we accordingly observed smaller vapor bubbles that disappeared within one frame (193 μs). Comparing the shape of these bubbles, we observed that the Er:YAG bubbles were relatively flat and larger in width than in height. The CO2 laser-introduced bubbles were more like a droplet with a larger height.
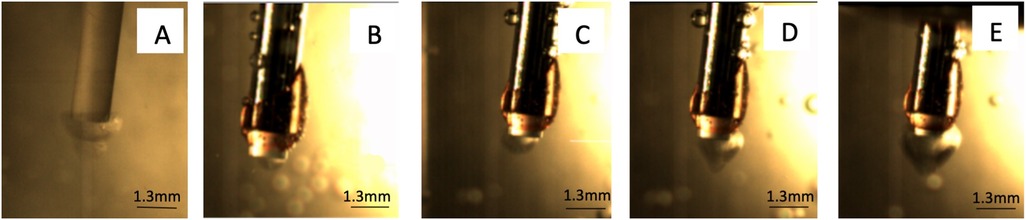
Figure 3 Activated laser tips submerged in deionized water; the frame length is 193 μs for (A) Er:YAG; (B) CO2 10%; (C) CO2 20%; (D) CO2 40%; (E) CO2 60%.
Effectiveness of cavitation development (streaming) with diode and CO2 lasers
We clearly observed differences in the movement of carbon particles between the Er:YAG laser, the 9,300-nm CO2 laser, and the diode lasers. The total path traveled by a particle during eight frames (1,544 μs) was significantly higher for the Er:YAG and 9,300-nm CO2 lasers with power parameters ≥20% than for the diode lasers. The particles activated with the Er:YAG and CO2 lasers traveled >1 mm in the liquid and <0.7 mm with the diode LAI in a period 20 times longer (30,460 μs). Further, the carbon particles, after activation with the 9,300-nm CO2 laser (10%) and all the diodes, led to an upward movement of the particles contrarily to the straight direction downward with the Er:YAG laser and the 9,300-nm CO2 laser with ≥20% power.
With regard to the distances and velocities, the movements of the particles slowed down the further they moved away from the laser tip (Figure 4). The velocities of all lasers significantly decreased with each frame but dropped after the second frame. This is why we used the statistical analysis on the first two frames.
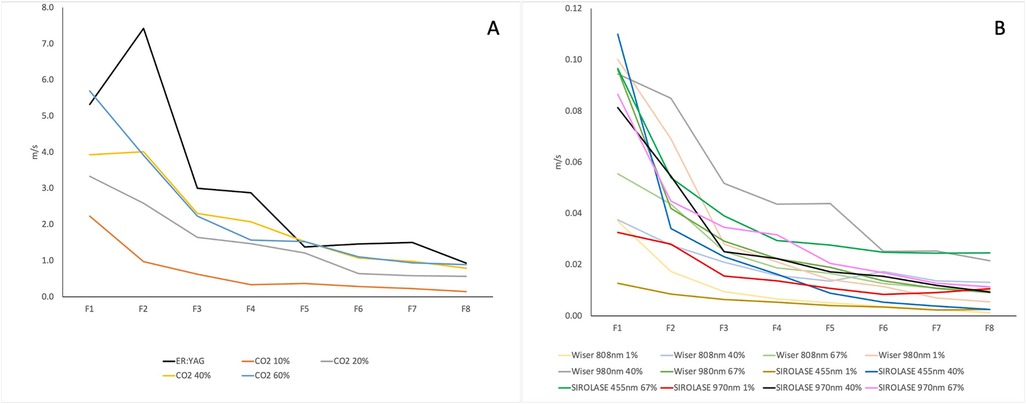
Figure 4 Velocities of carbon particles over nine frames in liquid activated by (A) Er:YAG and CO2 lasers; (B) diode lasers.
With regard to the average values of the first two frames, the velocities of the particle movements are statistically different between the Er:YAG and the different parameters of the 9,300-nm CO2 and diode lasers, but not within the group of diode lasers (Table 2, ranking capital letters). The activation of the Er:YAG laser produced the highest velocities, followed by the 9,300-nm CO2 laser with 60% power. The streaming velocities with the 9,300-nm CO2 laser were modulated by the power input (see Figure 5A and Table 2). The diode laser WISER (compare Figure 5C) reached significantly higher streaming velocities with 980 nm as with 808 nm. The values of the SIROLASE with both wavelengths were similar for the higher power parameters (see Figure 5B and Table 2). However, there was a significant difference between the different wavelengths of the diode lasers (compare Table 2, small letters).
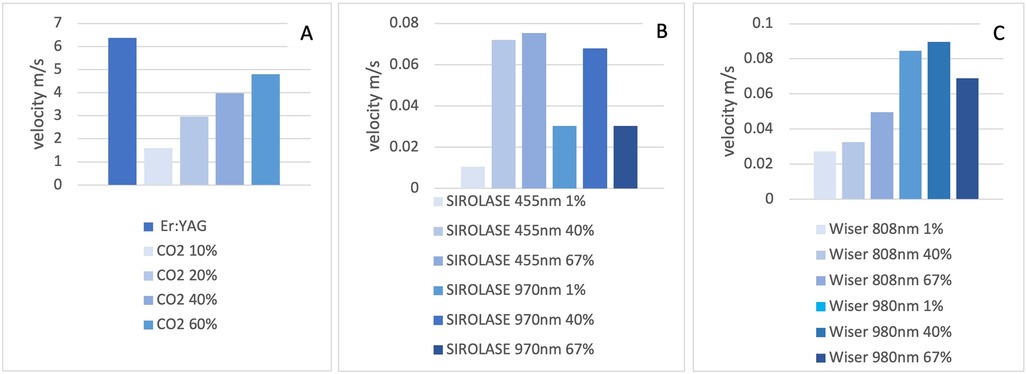
Figure 5 Velocity of first two frames in m/s for (A) Er:YAG and CO2 lasers; (B) diode laser SIROLASE; (C) diode laser WISER.
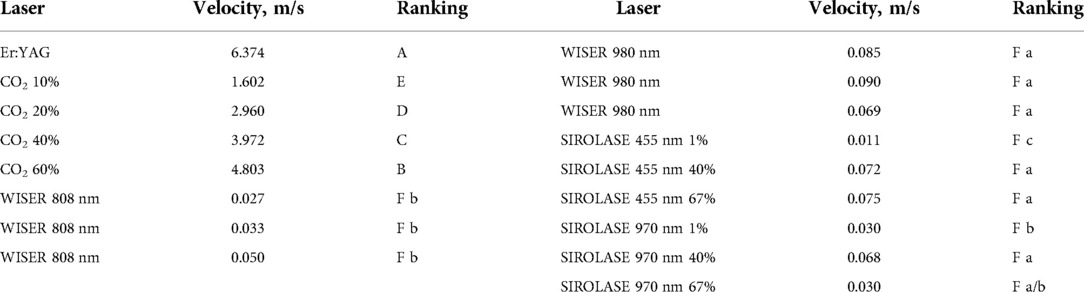
Table 2 Velocity of carbon particle movement after laser activation; the capital letters describe a ranking of all included lasers together and the small letters consider exclusively a ranking of the diode lasers (WISER and SIROLASE).
Discussion
With the aim of improving endodontic success rates with possibilities of enhanced cleaning and disinfection of root canal systems, this study evaluated the suitability of new laser wavelengths for LAI. Laser light absorption in the irrigant leads to cavitation, and consequent streaming of the liquid might enhance the penetration of irrigants into the root canal system as well as its disinfection potential on the tissues in deeper layers. LAI is therefore a promising approach to be included in clinical protocols.
In the present study, we investigated the basic mechanism of cavitation and its influence on the irrigant in terms of liquid streaming with a 9,300-nm CO2 laser and low-power diode lasers with 455, 810, 980, and 970 nm wavelengths.
With both tested diode laser devices and their four wavelengths, it was not possible to introduce clear cavitation bubbles in water. However, it cannot be concluded that the 980/970-nm wavelengths are not able to produce cavitation in water, as the literature reports on the development of vapor bubbles with higher laser power parameters (17, 23, 24). Blue (455 nm) and red diode lasers (808 nm) produce powerful cavitation in water, colored with orange and green dyes, which can be explained by their direct absorption in the corresponding liquids (25).
A black coating, however, enabled all tested wavelengths to produce vapor bubbles in pure water with just 180 mW for the SIROLASE laser and 200 mW average power for the WISER laser. Therefore, we can conclude that, theoretically, every diode laser—independent of its wavelengths—can produce vapor bubbles and consequent streaming in water-based solutions when its tip is adequately blackened. Furthermore, it can be confirmed that with this technique, the energy required to produce vapor bubbles is significantly lower than the values described in the literature without the blackening of the tips (17). Still, it is possible to introduce more powerful cavitation with increasing laser average power, meaning increasing peak powers and/or increasing the pulse length (duty cycles: time laser on vs. laser off within one period). The mechanism of the hot tip is based on the transformation of the laser energy in thermal energy through the coating. The laser light absorbs the black coating and heats it up constantly. The heat from the coating is transferred to the surrounding liquid and, after a certain time, leads to boiling and therefore to the formation of vapor bubbles (22).
Hereby, the life cycle of laser-induced bubbles is directly dependent on the pulse duration as the latter determines the beginning and end of the bubble cycle and its size (8, 9). Further, they need more time to be formed and last over a longer period of time. The literature explains this with relatively long thermal relaxation times and a temperature gradient that stays confined over a long period (26).
The difference between the bubbles for both lasers might be due to differences in the diameters of the tips (0.4 and 0.2 mm) and in the laser systems themselves, with each modulating its energy output and therefore the capacity of heating.
The application of the hot tip for LAI might be a very promising feature of diode lasers in the field of endodontic treatments. Clinicians might use the same diode laser device for photodisinfection via direct laser irradiation of the root canals and for enhanced irrigation and cleaning via LAI.
In contrast to the diode laser wavelengths, the Er:YAG and 9,300-nm CO2 lasers showed a powerful absorption in water. Their emitted light led to an explosive vaporization of a water layer adjacent to the fiber tip, generating the vapor bubble that appeared a few microseconds after the laser pulse (26).
The results of these experiments confirm the hypothesis that the shape and size of the bubbles with the Er:YAG and 9,300-nm CO2 lasers in pure water are similar with specific power parameters. The laser effects on the irrigant depend on the laser pulse energy applied: increasing power parameters increase the LAI effects (7). Preliminary unpublished tests also confirm the suitability of all tested laser devices for the activation of NaOCl.
The powerful absorption of the Er:YAG and the 9,300-nm CO2 laser lights in water explains the differences emanating from the diode lasers concerning the production of vapor bubbles. The process of transformation of laser light energy to heat energy with the diode lasers is less effective as the bubbles that are produced by the direct absorption with the other two lasers in the water. More output energy seems to be necessary to heat up the coating (enough) to introduce the boiling of water and production of vapor. Furthermore, the bubbles introduced with the diode lasers are smaller as with the Er:YAG and 9,300-nm CO2 lasers, which can be explained by the difference in absorption as well as the diameter of the tips and coating.
In contrast to the basic concepts in physics, the literature describes larger bubbles for larger tip diameters at the same laser energy settings (27). This might further play a role in the differences in the size of the bubbles between the larger tips of the Er:YAG (diameter of 1.3 mm) and 9,300-nm CO2 (diameter of 1.25 mm) lasers compared with the diode lasers (diameters of 0.4 and 0.2 mm). In the context of cavitation bubbles, the influence of the tip's geometry and size might be an important factor that was not investigated in the present study. This is why the values for bubble size and streaming velocity might not be identical with the application of the same laser and its parameters with different tip diameters. We applied flat cylindrical tips for all laser devices, so that the laser radiation exits the optical fiber in a forward direction with a relatively small divergence (9), basically resulting in channel-like bubbles with an oval, long, and almost elliptical shape (5, 9).
With modified tip morphologies, the literature describes an advantageous three-dimensional energy distribution on root canal walls and improved cleaning abilities (8, 9, 28, 29). The differences between the laser devices themselves as well as the different tip morphologies and sizes make a direct comparison with the literature difficult. In addition, the diameter of the model (root canal, tube) determines the size of the bubbles and therefore influences the results (30).
Effectiveness of cavitation development (streaming) with diode and CO2 lasers
To assess the effectiveness of laser-induced cavitation, we analyzed the resulting streaming velocities in the liquid with the visualization of particle movements.
First, we have to emphasize that the anatomic form and diameter of the investigated tube (artificial root canal system, single tube) and the overall liquid volume directly determine the streaming velocities within the liquids as well as the size of the laser tip. Differences in the dynamics of bubble growth between root canal models and free-standing water situations are important. In narrow root canal models, the expansion of the bubbles is limited sideways by the lateral canal walls and backward by the fiber tip itself, leading to long-lasting high pressure inside the bubbles, which is more difficult to be overtaken by the surrounding water pressure. The literature reports that these aspects delay the dynamics of expansion and implosion by three times compared with the free-water situation (6, 8, 31).
Due to the principle “the higher the diameter, the lower the velocity,” velocities in tubes with smaller diameters are higher as in free running water, which also explains the differences between different root canal models and different root parts (7). It is therefore important to consider that the streaming properties and streaming velocities of the present study cannot be transferred directly to root canal systems and clinical situations. These experiments can only prove the principle of laser activation with the applied lasers.
As shown in the results section, the velocities clearly decrease with longer distances from the laser tip for all lasers. Due to the water resistance, the particle's velocity tends toward zero with increasing distances (8).
With our experimental setup, we saw that diode lasers are only able to introduce liquid movements in the direct surrounding of the tip. We observed that the laser power and consequent velocities of carbon particles might determine the direction of the movements. The higher the velocities, the straighter the particles' way downward into the liquid and the further the particle movement have been. All diode laser settings and the 9,300-nm CO2 laser with 10% setting show particle movements oriented in an upward direction, which is shorter than that of other CO2 laser parameters, and Er:YAG laser activation, which is oriented straight downward into the liquid. The upward-moving trend might be explained by the specific properties of carbon particles (mass and density) and the effect of Triton X on water surface tension (decreasing), which are more important than the downward trend due to laser activation.
With regard to the velocity measurements, we have to reject the null hypothesis that the Er:YAG and 9,300-nm CO2 lasers can produce similar streaming, but accept that diode lasers will be on a par with or inferior to both the Er:YAG and the 9,300-nm CO2 lasers.
As reported in the literature, all diode lasers with black-coated tips and low-power laser parameters induce higher velocities than a 980-nm diode laser without coating, which emphasizes the positive effect of the black coating on LAI. However, the described velocities for the Er:YAG laser in the literature vary widely (1–30 m/s), which accentuates the importance of the factors described above, such as laser power, liquid volumes, and the tube's geometry (5, 7, 28, 30).
LAI with the Er:YAG and CO2 lasers seems to be very promising when comparing the introduced streaming velocities with traditional syringe irrigation. The literature reports on velocities in the order of 1 m/s at needle orifices (32). The tested diode lasers introduce significantly slower streaming velocities with the present setup, which could result in less important effects on the cleaning abilities of root canal systems and dentin, which should be investigated further.
Future research should concentrate on the effectivity and safety of CO2 and diode lasers to clean root canal systems filled with debris and the smear layer. In addition, further possibilities and improvements regarding tip morphology and coating possibilities should be investigated.
Conclusion
It could be shown that a 9,300-nm CO2 laser produces cavitation in water-based irrigants. With the low-power diode lasers, the activation of water was only possible with a hot-tip method, which allowed cavitation of all diode lasers independent of their wavelengths and the irrigant's color. The cavitation and streaming velocities in water were therefore stronger with increasing laser power settings (energy and pulse duration).
Compared with the Er:YAG laser, the CO2 laser induced similar bubbles with 40% and 60% power, but streaming velocities of the liquid were slower. The diode lasers’ wavelengths performed similarly but were inferior to those of the Er:YAG and CO2 lasers. The streaming velocities of all tested lasers decreased with a larger distance from the laser tip. This effect was most prominent for the diode laser, indicating that its action is concentrated only on the direct surrounding of the laser tip.
Data availability statement
The raw data supporting the conclusions of this article will be made available by the authors, without undue reservation.
Ethics statement
Ethical review and approval was not required for this study in accordance with the local legislation and institutional requirements.
Author contributions
Conceptualization: CIAyO and IK. Methodology: CIAyO and IK. Investigation: CIAyO and LM. Data analysis: EMB. Writing, original draft preparation: CIAyO. Writing, review and editing: LM, AF, and IK. Supervision: AF and IK. Project administration: CIAyO. All authors contributed to the article and approved the submitted version.
Funding
Open access funding provided by the University of Geneva.
Acknowledgments
We would like to thank Bastian Rheingans, Laboratory for Joining Technologies and Corrosion, Empa, Swiss Federal Laboratories for Materials Science and Technology, and Dübendorf, Switzerland, for providing access to the high-speed camera equipment and support for its application, as well as acknowledge Orcos Medical AG and Convergent Dental for providing the laser device. Orcos Medical and Convergent Dental were not involved in the study design, collection, analysis, interpretation of data, the writing of this article, or the decision to submit it for publication.
Conflict of interest
The authors declare that the research was conducted in the absence of any commercial or financial relationships that could be construed as a potential conflict of interest.
Publisher's note
All claims expressed in this article are solely those of the authors and do not necessarily represent those of their affiliated organizations or those of the publisher, the editors, and the reviewers. Any product that may be evaluated in this article, or claim that may be made by its manufacturer, is not guaranteed or endorsed by the publisher.
References
1. Haapasalo M, Shen YA, Ricucci D. Reasons for persistent and emerging post-treatment endodontic disease. Endod Topics. (2008) 18:31–50. doi: 10.1111/j.1601-1546.2011.00256.x
2. Schilder H. Cleaning and shaping the root canal. Dent Clin North Am. (1974) 18(2):269–96. doi: 10.1016/S0011-8532(22)00677-2
3. Haapasalo M, Orstavik D. In vitro infection and disinfection of dentinal tubules. J Dent Res. (1987) 66(8):1375–9. doi: 10.1177/00220345870660081801
4. Dioguardi M, Di Gioia G, Illuzzi G, Laneve E, Cocco A, Troiano G. Endodontic irrigants: different methods to improve efficacy and related problems. Eur J Dent. (2018) 12(3):459–66. doi: 10.4103/ejd.ejd_56_18
5. Blanken J, De Moor RJ, Meire M, Verdaasdonk R. Laser induced explosive vapor and cavitation resulting in effective irrigation of the root canal. Part 1: a visualization study. Lasers Surg Med. (2009) 41(7):514–9. doi: 10.1002/lsm.20798
6. Blankena JW, Rudolf M B. Verdaasdonkb, cavitation as a working mechanism of the Er,Cr:ySGG laser in endodontics: a visualization study. J Oral Laser Appl. (2007) 7:97–106.
7. de Groot SD, Verhaagen B, Versluis M, Wu MK, Wesselink PR, van der Sluis LW. Laser-activated irrigation within root canals: cleaning efficacy and flow visualization. Int Endod J. (2009) 42(12):1077–83. doi: 10.1111/j.1365-2591.2009.01634.x
8. Matsumoto H, Yoshimine Y, Akamine A. Visualization of irrigant flow and cavitation induced by Er:YAG laser within a root canal model. J Endod. (2011) 37(6):839–43. doi: 10.1016/j.joen.2011.02.035
9. Gregorcic P, Jezersek M, Mozina J. Optodynamic energy-conversion efficiency during an Er:YAG-laser-pulse delivery into a liquid through different fiber-tip geometries. J Biomed Opt. (2012) 17(7):075006. doi: 10.1117/1.JBO.17.7.075006
10. Anagnostaki E, Mylona V, Parker S, Lynch E, Grootveld M. Systematic review on the role of lasers in endodontic therapy: valuable adjunct treatment? Dent J. (2020) 8(3). doi: 10.3390/dj8030063
11. Lukac N, Zadravec J, Gregorcic P, Lukac M, Jezersek M. Wavelength dependence of photon-induced photoacoustic streaming technique for root canal irrigation. J Biomed Opt. (2016) 21(7):75007. doi: 10.1117/1.JBO.21.7.075007
12. Do QL, Gaudin A. The efficiency of the Er:YAG laser and photon-induced photoacoustic streaming (PIPS) as an activation method in endodontic irrigation: a literature review. J Lasers Med Sci. (2020) 11(3):316–34. doi: 10.34172/jlms.2020.53
13. Nguyen D, Chang K, Hedayatollahnajafi S, Staninec M, Chan K, Lee R, et al. High-speed scanning ablation of dental hard tissues with a lambda=9.3 mum CO2 laser: adhesion, mechanical strength, heat accumulation, and peripheral thermal damage. J Biomed Opt. (2011) 16(7):071410. doi: 10.1117/1.3603996
14. Benedicenti S, Cassanelli C, Signore A, Ravera G, Angiero F. Decontamination of root canals with the gallium-aluminum-arsenide laser: an in vitro study. Photomed Laser Surg. (2008) 26(4):367–70. doi: 10.1089/pho.2008.2158
15. Alfredo E, Souza-Gabriel AE, Silva SR, Sousa-Neto MD, Brugnera-Junior A, Silva-Sousa YT. Morphological alterations of radicular dentine pretreated with different irrigating solutions and irradiated with 980-nm diode laser. Microsc Res Tech. (2009) 72(1):22–7. doi: 10.1002/jemt.20638
16. Gutknecht N, Franzen R, Schippers M, Lampert F. Bactericidal effect of a 980-nm diode laser in the root canal wall dentin of bovine teeth. J Clin Laser Med Surg. (2004) 22(1):9–13. doi: 10.1089/104454704773660912
17. Hmud R, Kahler WA, George R, Walsh LJ. Cavitational effects in aqueous endodontic irrigants generated by near-infrared lasers. J Endod. (2010) 36(2):275–8. doi: 10.1016/j.joen.2009.08.012
18. Hmud R, Kahler WA, Walsh LJ. Temperature changes accompanying near infrared diode laser endodontic treatment of wet canals. J Endod. (2010) 36(5):908–11. doi: 10.1016/j.joen.2010.01.007
19. Alfredo E, Marchesan MA, Sousa-Neto MD, Brugnera-Junior A, Silva-Sousa YT. Temperature variation at the external root surface during 980-nm diode laser irradiation in the root canal. J Dent. (2008) 36(7):529–34. doi: 10.1016/j.jdent.2008.03.009
20. Levine R, Vitruk P. Laser-assisted operculectomy. Compend Contin Educ Dent. (2015) 36(8):561–7, quiz 568. PMID: 26355439.26355439
21. Romanos GE. Diode laser soft-tissue surgery: advancements aimed at consistent cutting, improved clinical outcomes. Compend Contin Educ Dent. (2013) 34(10):752–7, quiz 758. PMID: 24571504.24571504
22. Rosenthal E, Foussas S, Montarello JK, Boyd EG, Curry PV. Laser thermal angioplasty probe (“hot tip”) temperature: effects of flow. Lasers Surg Med. (1990) 10(2):124–32. doi: 10.1002/lsm.1900100204
23. Lagemann M, George R, Chai L, Walsh LJ. Activation of ethylenediaminetetraacetic acid by a 940 nm diode laser for enhanced removal of smear layer. Aust Endod J. (2014) 40(2):72–5. doi: 10.1111/aej.12039
24. Walsh LJ, George R. Activation of alkaline irrigation fluids in endodontics. Materials. (2017) 10(10). doi: 10.3390/ma10101214
25. Beltes C, Economides N, Sakkas H, Papadopoulou C, Lambrianidis T. Evaluation of antimicrobial photodynamic therapy using indocyanine green and near-infrared diode laser against Enterococcus faecalis in infected human root canals. Photomed Laser Surg. (2017) 35(5):264–9. doi: 10.1089/pho.2016.4100
26. Paltauf G, Schmidt-Kloiber H, Frenz M. Photoacoustic waves excited in liquids by fiber-transmitted laser pulses. Acoust Soc Am. (1998) 104(890):3616–27. doi: 10.1121/1.423334
27. Kouno A, Watanabe S, Hongo T, Yao K, Satake K, Okiji T. Effect of pulse energy, pulse frequency, and tip diameter on intracanal vaporized bubble kinetics and apical pressure during laser-activated irrigation using Er:YAG laser. Photobiomodul Photomed Laser Surg. (2020) 38(7):431–7. doi: 10.1089/photob.2019.4739
28. George R, Chan K, Walsh LJ. Laser-induced agitation and cavitation from proprietary honeycomb tips for endodontic applications. Lasers Med Sci. (2015) 30(4):1203–8. doi: 10.1007/s10103-014-1539-y
29. George R, Meyers IA, Walsh LJ. Laser activation of endodontic irrigants with improved conical laser fiber tips for removing smear layer in the apical third of the root canal. J Endod. (2008) 34(12):1524–7. doi: 10.1016/j.joen.2008.08.029
30. Koch JD, Jaramillo DE, DiVito E, Peters OA. Irrigant flow during photon-induced photoacoustic streaming (PIPS) using particle image velocimetry (PIV). Clin Oral Investig. (2016) 20(2):381–6. doi: 10.1007/s00784-015-1562-9
31. De Moor R, Meire M, Verdaasdonk R. The power of the bubble: comparing ultrasonic and laser activated irrigation. Proc. SPIE (2013) 8925:892504. doi: 10.1117/12.2042357
Keywords: laser-activated irrigation, endodontics and laser therapy (MeSH), streaming, laser, cavitation, Er:YAG and erbium-based solid state lasers, CO2 laser, diode laser
Citation: Anton y Otero CI, Marger L, Di Bella E, Feilzer A and Krejci I (2023) Laser-activated irrigation: Cavitation and streaming effects from dental lasers. Front. Dent. Med 3:1010916. doi: 10.3389/fdmed.2022.1010916
Received: 3 August 2022; Accepted: 29 November 2022;
Published: 24 February 2023.
Edited by:
Ashraf F. Fouad, University of Alabama at Birmingham, United StatesReviewed by:
Vasudev Ballal, Manipal Academy of Higher Education, IndiaNasim Chiniforush, Tehran University of Medical Sciences, Iran
© 2023 Anton y Otero, Marger, Di Bella, Feilzer and Krejci. This is an open-access article distributed under the terms of the Creative Commons Attribution License (CC BY). The use, distribution or reproduction in other forums is permitted, provided the original author(s) and the copyright owner(s) are credited and that the original publication in this journal is cited, in accordance with accepted academic practice. No use, distribution or reproduction is permitted which does not comply with these terms.
*Correspondence: Clara Isabel Anton y Otero, Y2xhcmEuYW50b255b3Rlcm9AdW5pZ2UuY2g=
Specialty Section: This article was submitted to Endodontics, a section of the journal Frontiers in Dental Medicine