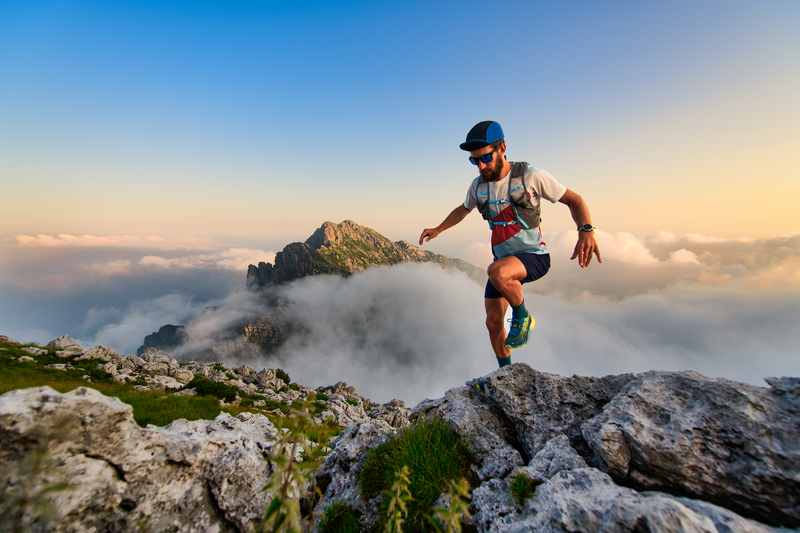
95% of researchers rate our articles as excellent or good
Learn more about the work of our research integrity team to safeguard the quality of each article we publish.
Find out more
ORIGINAL RESEARCH article
Front. Dement. , 06 September 2023
Sec. Translational Research in Dementia
Volume 2 - 2023 | https://doi.org/10.3389/frdem.2023.1198006
This article is part of the Research Topic Lifestyle and Healthy Aging to Prevent Cognitive Decline and Dementia View all 28 articles
Background: Modulation of physical activity represents an important intervention that may delay, slow, or prevent mild cognitive impairment (MCI) or dementia due to Alzheimer's disease (AD). One mechanism proposed to underlie the beneficial effect of physical exercise (PE) involves the apparent stimulation of adult hippocampal neurogenesis (AHN). BCI-838 is a pro-drug whose active metabolite BCI-632 is a negative allosteric modulator at group II metabotropic glutamate receptors (mGluR2/3). We previously demonstrated that administration of BCI-838 to a mouse model of brain accumulation of oligomeric AβE22Q (APPE693Q = “Dutch APP”) reduced learning behavior impairment and anxiety, both of which are associated with the phenotype of Dutch APP mice.
Methods: 3-month-old mice were administered BCI-838 and/or physical exercise for 1 month and then tested in novel object recognition, neurogenesis, and RNAseq.
Results: Here we show that (i) administration of BCI-838 and a combination of BCI-838 and PE enhanced AHN in a 4-month old mouse model of AD amyloid pathology (APPKM670/671NL/PSEN1Δexon9= APP/PS1), (ii) administration of BCI-838 alone or with PE led to stimulation of AHN and improvement in recognition memory, (iii) the hippocampal dentate gyrus transcriptome of APP/PS1 mice following BCI-838 treatment showed up-regulation of brain-derived neurotrophic factor (BDNF), PIK3C2A of the PI3K-mTOR pathway, and metabotropic glutamate receptors, and down-regulation of EIF5A involved in modulation of mTOR activity by ketamine, and (iv) validation by qPCR of an association between increased BDNF levels and BCI-838 treatment.
Conclusion: Our study points to BCI-838 as a safe and orally active compound capable of mimicking the beneficial effect of PE on AHN and recognition memory in a mouse model of AD amyloid pathology.
Physical exercise (PE) has important neuroprotective and pro-cognitive benefits (Buchman et al., 2019; Mortimer and Stern, 2019). One long-suggested mechanism underlying the beneficial effects of PE on central nervous system (CNS) function is its effect on neurogenesis in the adult hippocampal dentate gyrus (DG) and the subventricular zone (SVZ) of the lateral ventricles (Obernier and Alvarez-Buylla, 2019; Toda et al., 2019). In these regions, new neurons are generated and incorporated into existing neuronal circuits where they promote structural and functional plasticity. Stimulation of adult hippocampal neurogenesis (AHN) has been proposed as a central mechanism of adult brain plasticity and a potential therapeutic target in a variety of psychiatric and neurodegenerative conditions (Toda et al., 2019). Drugs such as antidepressants that affect monoaminergic systems consistently increase AHN (Hanson et al., 2011). The DG is critical for learning and memory, and altered AHN has been implicated in the pathogenesis of neurodegenerative diseases such as Alzheimer's disease (AD) (Lazarov and Marr, 2010; Llorens-Martin, 2018). A recent review of AHN in neurological diseases (Gage, 2021) highlighted a key study of the DG from postmortem samples of patients who died from neurodegenerative disorders. The study demonstrated that functions of the neurogenic niche shifted and the cells produced were abnormal in shape and differentiation, further emphasizing the plasticity characteristic of the hippocampus and its potential as a therapeutic target (Terreros-Roncal et al., 2021). Furthermore, a recent study demonstrated that greater physical activity was associated with higher levels of presynaptic proteins, suggesting maintenance or building brain resilience (Casaletto et al., 2022).
Exercise-induced AHN has been extensively studied in rodents, and voluntary PE (e.g., running wheels) has been shown to enhance AHN in the DG (Ma et al., 2017). Studies utilizing cell fate tracers [e.g., bromodeoxyuridine (BrdU)], have shown that exercise increases proliferation of progenitor cells in the subgranular zone and promotes their survival and differentiation into mature neurons (Van Praag et al., 1999; Buchman et al., 2019). Increased expression of brain-derived neurotrophic factor (BDNF) may be one mechanism underlying the proneurogenic effect of PE (Liu and Nusslock, 2018).
Altered AHN has been reported in postmortem brain from humans suffering from AD (Lazarov and Marr, 2010; Llorens-Martin, 2018). In humans, long-term exercise improves blood flow while also increasing hippocampal volume and neurogenesis in subjects with AD (Karssemeijer et al., 2017; Meng et al., 2020). AHN is also altered in transgenic mouse models of AD (Jin et al., 2004; Wen et al., 2004; Kuhn et al., 2007; Taniuchi et al., 2007; Verret et al., 2007; Niidome et al., 2008; Yu et al., 2009; Chuang, 2010; Demars et al., 2010; Elder et al., 2010; Krezymon et al., 2013; Unger et al., 2016), and exercise has been reported to improve learning behavior in some of these models. For example, in APPKM670/671NL/PSEN1Δexon9(APP/PS1) transgenic mice, 2 days of treadmill running for 30 min was associated with increased brain activity, as shown by increased theta rhythm on electroencephalography (Borchelt et al., 1996). In the same APP/PS1 model, 20 weeks of treadmill training improved learning behavior and reduced brain levels of the 42-aminoacid form of amyloid beta peptide (Aβ) (Bo et al., 2014). In the 5xFAD mouse model of AD, 4 months of voluntary PE in a running wheel improved learning behavior and increased levels of BDNF and synaptic markers in the hippocampus (Choi et al., 2018).
BCI-838 (MGS0210) is a pro-drug that is metabolized to generate a negative allosteric modulator that targets group II metabotropic glutamate receptors (mGluR2/3) (Kim et al., 2014; Perez-Garcia et al., 2018). BCI-838 is metabolized in the liver into BCI-632, which is the active compound delivered to the brain. As a class, mGluR2/3 receptor antagonists are proneurogenic, as evidenced by their stimulation of AHN while also enhancing learning behavior and exerting broad anxiolytic and antidepressant properties (Chaki and Fukumoto, 2018). Previously, BCI-838 administration was observed to improve learning behavior and reduce anxiety in a transgenic mouse model of cerebral amyloidosis (Kim et al., 2014) and in a rat model of blast-related traumatic brain injury (Perez-Garcia et al., 2018).
Since both BCI-838 and PE are associated with enhanced AHN, we sought to determine whether BCI-838 might mimic the effects of PE, and whether a combined treatment with both drug and PE might be additive or synergistic. Our findings demonstrate that BCI-838 stimulates AHN, improves recognition memory, and upregulates BDNF and PIK3C2A levels of the mammalian target of rapamycin (mTOR) pathway, as well as metabotropic glutamate receptors in APP/PS1 transgenic mice, thereby mimicking some of the effects of PE.
Experimental procedures were conducted in accordance with and approved by the Institutional Animal Care and Use Committee (IACUC) of the James J. Peters VA Medical Center. Studies were conducted in compliance with the US Public Health Service policy on the humane care and use of laboratory animals, the NIH Guide for the Care and Use of Laboratory Animals, and all applicable Federal regulations governing the protection of animals in research. APPKM670/671NL/PSEN1Δexon9 (APP/PS1) transgenic mice (Cat# 34832-JAX; RRID: MMRRC_034832-JAX) were obtained from Jackson Laboratories (JAX). Mice used for this study were generated by breeding APP/PS1 mice with C57BL/6 wild-type (WT) mice (also obtained from JAX; Cat#000664 RRID: IMSR_JAX: 000664). Non-transgenic littermates were used as wild type controls and all mice were on a C57BL/6 background. All studies used male mice and included three cohorts. Figure 1 outlines the studies and how the mice were distributed across the different assays. The first cohort was perfusion-fixed with 4% paraformaldehyde for neurogenesis studies (five animals per group). The second cohort was processed for biochemical studies. These mice were euthanized by CO2 narcosis, and the brain was divided into 2 hemispheres with the left dentate gyrus (DG) used for RNAseq and the right DG for ELISA to measure BDNF levels. The third cohort used 10 to 12 mice per group for behavioral testing. To avoid the confounding effects of behavioral testing, tissue from mice that underwent behavioral testing was not used for any of the RNA or neurogenesis studies. The total number of mice used for all studies was 105.
Figure 1. Experimental design and spontaneous activity. (A) APP/PS1 mice were divided into four groups: Group 1 no PE/no drug; Group 2 PE/no drug; Group 3, no PE/drug; and Group 4, PE + drug. Group 5 consisted of WT mice (C57BL/6). (B) Groups receiving PE were given ad libitum access to a running wheel and treatment with drug or vehicle was continued for 30 days. All studies used male mice and included three cohorts. The first cohort was processed for neurogenesis studies, following perfusion-fixation with PFA 4% (five animals per group). The second cohort was processed for RNA and biochemical studies. We sacrificed animals by CO2 narcosis and the DG was removed for RNAseq and determination of BDNF by qPCR. We processed five animals per group. The third cohort was used for behavior testing (10-12 mice per group).
Cages were fitted with running wheels obtained from Columbus Instruments and equipped with Activity Wheel Monitoring Software. Mice were singly housed with one running wheel per cage and were allowed access to running wheels ad libitum. Running wheel (Keiser et al., 2009) data (number of wheel revolutions) were recorded. Cages that housed mice in the non-exercise groups were fitted with dummy wheels that the mice could enter but could not run.
BCI-838 was prepared as previously described (Perez-Garcia et al., 2018). BCI-838 was dissolved in a solution of 5% carboxymethylcellulose (CMC; Sigma Aldrich) and 0.3% 2N hydrochloric acid solution (Sigma Aldrich). Animals were divided into five experimental groups: (1) APP/PS1 mice treated with vehicle (APP/PS1 control); (2) APP/PS1 mice treated with vehicle and exposed to running wheels (APP/PS1 + PE); (3) APP/PS1 mice treated with 5 mg/kg BCI-838 (APP/PS1 + BCI-838); and (4) APP/PS1 mice treated with 5 mg/kg BCI-838 and exposed to running wheels (APP/PS1 + BCI-838 + PE combination) and (5) WT mice treated with vehicle (Figures 1A, B). BCI-838 was administered daily for 30 days by oral gavage using a 5-cm straight stainless-steel gavage needle with a 2-mm ball tip (Fischer Scientific).
All animals received a single intraperitoneal injection of BrdU (50 mg/kg of body weight) 24 h before sacrifice at the end of drug treatment. BrdU (Sigma) was dissolved in saline solution (0.9% NaCl in sterile H2O) warmed to 40oC and gently vortexed. The solution was allowed to cool to room temperature (25oC) and injected 24 h before sacrifice.
Behavioral tests began at the end of the 30 days of drug administration for the third cohort (Figure 1). Each group included 10 to 12 mice. All behavior sessions and trials were recorded by video camera (Sentech) and analyzed with ANYMAZE software (San Diego Instruments).
Mice were habituated to the circular arena (30 cm in diameter length × 30 cm height) for 10 min, 24 h before training. On the training day, two identical objects were placed in opposite ends of the empty arena, and the mouse was allowed to freely explore the objects (Ob1 and Ob2) for 5 min. After 24 h, during which the mouse was held in its home cage, one of the two now familiar objects (FO) was replaced with a novel object. The mouse was allowed to freely explore the FO and NO for 5 min to assess long-term memory (LTM). Raw exploration times for each object were expressed in seconds. Object exploration was defined as sniffing or touching the object with the nose placed at < 2 cm. Offline analysis by an investigator blind to the treatment status of the animals was performed. During the training session, two identical objects made of LEGO plastic material (≈2.5 cm diameter and height) were used. In the long-term memory (LTM) testing, one of the LEGOs was replaced with a ceramic cup (≈2.5 cm maximum diameter and height). No animals were observed to climb the objects. All objects were wiped with 70% ethanol between trials.
Animals were sacrificed at the conclusion of the drug treatment, PE exposure, and behavioral testing. We induced deep anesthesia with a solution of 100 mg/kg ketamine and 20 mg/kg xylazine, and mice were euthanized by transcardial perfusion with cold PBS (20 ml) followed by perfusion with 4% paraformaldehyde (PFA) in PBS. After perfusion, brains were removed and postfixed in PFA 4% for 48 h, transferred to PBS, and stored at 4oC until sectioning. Coronal sections (50 μm) were cut through the entire extent of the hippocampus using a Leica VT1000 S Vibratome (Leica). The sections were stored at −20oC in a cryoprotectant solution (25% ethylene glycol and 25% glycerine in 0.05 M PBS) until processing for immunofluorescence. Stereology-based counting was performed as described (Perez-Garcia et al., 2016). Every sixth section in a series through the hippocampus was processed for immunohistochemistry so that the interval between sections within a given series was 300 μm. BrdU and doublecortin (DCX) stainings were performed as previously described in Perez-Garcia et al. (2016, 2018). 4-5 animals per group were included in the analyses.
DG samples were homogenized in QIAzol Lysis Reagent (Qiagen), and total RNA purification was performed with the miRNeasy Micro kit (Qiagen). BDNF mRNA levels were determined by real-time quantitative PCR (qPCR). 200 ng of total RNAs were reverse transcribed using the High-Capacity RNA-to-cDNA Kit (Applied Biosystem). cDNAs were subjected to real-time qPCR in a StepOne Plus system (Applied Biosystem) using the TaqMan Gene Expression Master Mix (Applied Biosystem). qPCR consisted of 40 cycles, 10 s at 95oC, 20 s at 60oC, and 15 s at 70oC each. Ct values were normalized to the expression level of GAPDH. Five animals per group were included in the analyses. Sample sizes were selected based on previous studies (Readhead et al., 2018).
Values are expressed as mean ± SEM. For behavioral analysis, stereology, spontaneous activity analysis, biochemical analysis, and stereological analysis, statistical tests were performed using unpaired t-tests, one-way ANOVA, repeated measures ANOVA, and the Kuskall–Wallis non-parametric test following by an uncorrected Dunn test. When more than two groups were compared, a one-way ANOVA was follow by Sidak's post-test if only selected groups were compared or Tukey's multiple comparison's test if all groups were compared. When repeated-measures ANOVA was used, sphericity was assessed using Mauchly's test. If the assumption of sphericity was violated (p < 0.05, Mauchly's test), significance was determined using the Greenhouse-Geisser correction. Pearson correlation coefficients were calculated. Statistical analysis was performed using Prism 9.4.1 (GraphPad Software) and SPSS v27.0.1.0 (IBM).
Total RNA from DG of all mice was subjected to RNA sequencing. The sequencing library was prepared using the NEB library prep kit (Novogene). Five animals per group were included in the analyses based on the experience of the Gandy/Ehrlich lab in previous studies (Readhead et al., 2016).
Computational analysis was performed using R version 4.0.2 (r-project.org). Paired-end RNA-Seq fastq files for 24 samples were aligned to the Mouse Reference genome (mm10) using the STAR (Dobin et al., 2013) read aligner. As part of quality control and to allow discrimination between human APP and PSEN1 transgenes and mouse APP and PSEN1 genes, all samples were also aligned to Homo sapiens reference genome (Grch38), and corresponding gene expressions were checked for sanity. Mapped reads were summarized to gene counts using the subread function of featureCounts (Liao et al., 2014).
Variance partitioning performed using variancePartition (Hoffman and Schadt, 2016) R package showed a high fraction of unexplained variance (“residuals,” see Supplementary Figure 1). We addressed this by applying surrogate variable analysis (SVA) using the sva (Leek et al., 2012) R package, to estimate unwanted sources of variation. The svaseq function estimated five surrogate variables (n.sv = 5), which were then included in our differential expression analysis as covariates to be accounted.
Gene count matrices were generated separately for five groups of 24 samples in total: WT (n = 5), APP/PS1 control (n = 5), APP/PS1 + BCI-838 (n = 5), APP/PS1 + PE (n = 4), APP/PS1 combination (n = 5); and six primary comparisons: APP/PS1 + BCI-838 vs. APP/PS1 control, APP/PS1 + PE vs. APP/PS1 control, APP/PS1 combination vs. APP/PS1 control, APP/PS1 combination vs. APP/PS1 + BCI-838, APP/PS1 combination vs. APP/PS1 + PE, APP/PS1 + BCI-838 vs. APP/PS1 + PE, and APP/PS1 control vs. WT. The overall gene count matrix was corrected for library size, normalized, and the resulting 18,357 genes were statistically analyzed for differentially expressed genes (DEGs) using DESeq2 (Love et al., 2014). p-values were adjusted using the Benjamini-Hochberg method, where DEGs were defined at a false discovery rate (FDR) of 0.05. DEGs sorted by adjusted p-values are provided in Supplementary Table 1.
DEG sets per primary comparison were tested for statistical enrichment using the EnrichR (Kuleshov et al., 2016) R package for transcription factors, pathways, and gene ontology against 11 relevant public databases. Relevant matches with an FDR of 0.05 were identified. Enrichments are provided in Supplementary Table 2.
All scripts used for analysis are publicly available via our GitHub pages: https://github.com/MesudeBicak/AlzheimersDiseaseResearch/tree/master/BCI838vsExerciseAPPPS1Mice.
Drug-induced gene expression fold-changes were obtained from the Connectivity Map database (Lamb et al., 2006). Individual expression profiles (6,100) were merged into a single representative signature for 1,309 unique compounds, according to the prototype-ranked list method (Iorio et al., 2010). Each compound was scored according to the transcriptomic similarity with BCI-838 transcriptomics (DEGs from APP/PS1 + BCI-838 vs. APP/PS1). Compounds were ranked in order of descending connectivity score. For each compound in the drug signature library, referenced drug–target associations (Law et al., 2014), predicted off-targeting (Keiser et al., 2009), and side effects were collected. For each of these features, we calculated a running sum enrichment score, reflecting whether that feature was over-represented among the compounds with transcriptomic similarity to BCI-838. Two-tailed p-values were based on comparison with 10,000 permuted null scores, generated from randomized drug target sets that contain an equivalent number of compounds to the true set under evaluation, and adjusted using the Benjamin–Hochberg method. Computational screening and chemogenomic enrichment analysis were performed using R. Drug repurposing results are provided in Supplementary Table 3.
Mouse groups received treatment with BCI-838 or vehicle for 1 month with or without PE (Figure 1). PE consisted of ad libitum access to a running wheel. Three-month-old mice were divided into five experimental groups: (1) APP/PS1 mice treated with vehicle (APP/PS1 control); (2) APP/PS1 mice treated with vehicle and exposed to running wheels (APP/PS1 + PE); (3) APP/PS1 mice treated with 5 mg/kg BCI-838 (APP/PS1 + BCI-838); and (4) APP/PS1 mice treated with 5 mg/kg BCI-838 and exposed to running wheels (APP/PS1 + BCI-838 + PE combination) and (5) WT mice treated with vehicle (Figures 1A, B). In this design, comparison of the single group of untreated wild type mice (Group 5) to untreated APP/PS1 mice (Group 1) served as a positive control for appearance of the transgene-related behavioral phenotype while comparison of the treated groups (2, 3, and 4) to group 1 provided a measure of the effects of the various treatments. Three cohorts of mice were studied. One cohort was used for behavioral analysis, the second for neurogenesis assays and the third for transcriptomic analysis. Spontaneous running wheel activity was recorded in the two cohorts exposed to active running wheels. In these two cohorts, when compared to APP/PS1 control, APP/PS1 + BCI-838 showed decreased spontaneous activity during the last 2 weeks of the treatment protocol, as evidenced by reduced counts of running wheel activity (Figure 2A). Weights of mice before and after treatment are shown in Figure 2B. All groups gained weight during treatment (Figure 2B) with no differences between APP/PS1 mice that received PE alone vs. PE + BCI-838 (Figure 2C).
Figure 2. BCI-838 decreased spontaneous activity after 2 weeks of treatment. (A) Number of running wheel turns (# counts) are shown for the two groups that received running wheel access. APP/PS1 mice treated with BCI-838 decreased running wheel use during the last 2 weeks of drug treatment and running wheel exposure. Repeated measures ANOVA over the entire 30 days of treatment revealed a significant difference in running wheel activity within groups (F3.8,61.1 = 3.695, p = 0.011) but no day*condition interaction effect (p = 0.052). A test of between subject effects over the 30 days revealed no significant effect of condition (F1,16 = 2.399, p = 0.14). Tests of within subject effects revealed significant effects of running wheel activity if analyzed over 1–14 days (F3.5,59.5 = 3.579, p = 0.014 for activity, F3.5,59.5 = 2.062, p = 0.105 for day*condition) and 14–28 days (F2.9,47.0 = 4.414, p = 0.008 for activity, F2.9,47.0 = 4.414, p = 0.366 for day*condition). There was no difference in running wheel activity between groups over days 1–14 (F1, 17 = 0.799) whereas activity was reduced in mice that received PE + drug between days 14–28 (F1,16 = 6.052, p = 0.026). Values significantly different between groups at individual time points are indicated by asterisks (*p < 0.05, **p < 0.01, unpaired t-tests). Values are expressed as mean ± SEM. 12 mice per group for each cohort were used. (B) Weights of mice before and after treatment with PE and/or BCI-838. There were no differences between groups analyzed pre-treatment (one way ANOVA, F4,42 = 0.9107, p = 0.4666), but differences between WT and BCI-838 and PE + BCI-838 group were found post-treatment (F4,42 = 5.588, p = 0.0011). However, a repeated measures ANOVA comparing pre-treatment to post-treatment revealed that mice gained weight after treatment (F2.575,21.17 = 8.547, p = 0.0010). Asterisks indicate significant between group differences when selected groups were directly compared using Sidak's test (*p < 0.05, **p < 0.01, ***p < 0.001). (C) Running wheel activity in APP/PS1mice treated with PE or PE + BCI-838 is shown pre-treatment, after 15 days of treatment and post-treatment. A one-way ANOVA revealed significant between group differences when all 6 groups were compared F(5,57) = 3.589, p = 0.0069. Asterisks indicate significant between group differences when indicated groups were directly compared using Sidak's test (*p < 0.05, **p < 0.01, ns, not significant).
We probed the effect of PE, BCI-838, or the two combined, on recognition memory in APP/PS1 mice (Figure 3). Novel object recognition (NOR) is a standard behavioral test used to evaluate hippocampal- and perirhinal-dependent memory. NOR is widely used to assess the progression of behavioral deficits in mouse models of AD. During training, no differences in object preference were observed among groups (Figure 3A). During LTM testing (Figure 3B), WT mice spent more time exploring the NO than the FO (p = 0.028). APP/PS1 mice explored the FO and NO for the same amount of time (p = 0.989), consistent with the conclusion that APP/PS1 mice at 4 months of age have impaired recognition memory. However, APP/PS1 mice exposed to 1 month of PE spent more time exploring the NO when tested 24 h after training (p = 0.0013). APP/PS1 mice treated with BCI-838 for 1 month also spent more time exploring the NO (p = 0.0001). APP/PS1 mice treated with the combination of BCI-838 and PE for 1 month also showed a preference for the NO compared to FO (p = 0.0006). Total exploration times between groups during training differed only in that APP/PS1 mice treated with PE and BCI-838 spent more time exploring the objects than wild type mice administered vehicle (Figure 3C) while in LTM testing APP/PS1 mice treated with BCI-838 alone were more exploratory than APP/PS1 mice treated with vehicle (Figure 3D). A discrimination index calculated for the training session showed no differences in object preference between the groups (Figure 3E) and one calculated for the LTM (Figure 3F), confirmed conclusions drawn from the raw object exploration data (Figure 3B) suggesting that recognition memory had been rescued in all treated groups compared to APP/PS1 mice administered vehicle alone. These results suggest that 1-month of either PE or BCI-838 drug administration, as well as the combination of both, can reverse impaired recognition memory in APP/PS1 mice.
Figure 3. Effect of BCI-838 treatment and PE on novel object recognition (NOR). (A) Training. No differences were found among groups during the training when the mice explored the identical objects, Object 1 (Ob1) and Object 2 (Ob2). (B) Long-term memory session (LTM). During the LTM testing conducted at 24 h after training, wild type mice spent more time exploring the novel object (NO) as compared to the familiar object (FO). APP/PS1 mice treated with vehicle explored the FO and the NO similar amounts of time whereas APP/PS1 mice exposed to PE, treated with BCI-838 or the combination of BCI-838 + PE for 1 month showed a preference for the NO compared to the FO. Values significantly different are indicated by asterisks (*p < 0.05, **p < 0.01, ***p < 0.001, ****p < 0.0001, ANOVA followed by Sidak's test, comparing selected pairs of columns). (C) Total time spent exploring the objects during training is shown. The combination of BCI-838 + PE mice explored the objects more compared to WT mice (one-way ANOVA: F4,48 = 3.286, p = 0.0185; *p < 0.05, Tukey's multiple comparisons test). (D) During LTM testing, APP/PS1 mice treated with BCI-838 alone were more exploratory than APP/PS1 mice treated with vehicle (F2,47 = 3.308, p = 0.0181; *p < 0.05, Tukey's multiple comparisons test). (E) A discrimination index calculated for the training session showed no difference in object preference between groups (F4, 47 = 1.226, p = 0.3124). (F) A discrimination index calculated for LTM (F4,47 = 6.131, p = 0.0005) found differences between APP/PS1 treated with vehicle and all other groups but no differences between treatments (** p < 0.01, ***p < 0.0001, Tukey's multiple comparisons test). Values are expressed as mean ± SEM). Ten to 12 mice per group were used for each cohort.
We next probed the effect of PE, BCI-838 administration, or the combination of both on AHN in APP/PS1 mice (Figure 4). Using immunofluorescence staining, we quantified newly generated neurons (doublecortin-labeled; DCX) cells (Figures 4a–e) and newly generated cells (BrdU-labeled; Figure 4f) in APP/PS1 mice treated with vehicle or PE, BCI-838, or both. While the number of DCX-labeled neurons was unchanged in APP/PS1 mice treated with vehicle vs. WT mice, this number was increased in APP/PS1 mice treated with BCI-838 or with BCI-838 plus PE, when either was compared to APP/PS1 treated with vehicle (F4,16 = 1.367, p = 0.0025; p < 0.05, ANOVA Tukey's multiple comparisons Figures 4a–e, g). Moreover, we observed that the total number of BrdU-labeled cells was increased in APP/PS1 mice treated with BCI-838 or BCI-838 plus PE as compared to APP/PS1 mice treated with vehicle (F4,14 = 1.734; p = 0.0148, p < 0.05, ANOVA Tukey's multiple comparisons Figures 4f, h). By contrast, PE alone did not increase the number of DCX or BrdU-labeled cells compared to vehicle treated WT or APP/PS1 mice.
Figure 4. Quantification of neurogenesis in WT, APP/PS1 treated with vehicle, PE, BCI-838 or the combination. (a–e) Images of DCX staining in WT, APP/PS1, APP/PS1 + PE, APP/PS1 + BCI-838, and APP/PS1 combination. (f) Representative image of BrdU staining in a WT mouse. The hilus and granule cell layer (GCL) are indicated. Arrows mark BrdU-labeled cells in the subgranular zone. Scale bar 50 μm. (g) Total number of doublecortin-labeled cells was increased in the APP/PS1 + BCI-838 and APP/PS1 combination as compared to APP/PS1 control. Values are expressed as mean ± SEM and differences among groups are indicated by asterisks (*p < 0.05, **p < 0.01, one-way ANOVA followed by Tukey's test). (h) Total number of BrdU-labeled cells was increased in APP/PS1 + BCI-838 and APP/PS1 combination compared to APP/PS1 control. Values significantly different among groups are indicated by asterisks as in panel (g). 4–5 mice per group for each cohort were used.
We generated transcriptomic profiles from all four groups of four-month-old APP/PS1 mice and one group of WT mice and performed RNA sequencing on 24 DG samples (Figure 5). When we performed variance partitioning analysis as part of quality control, we noticed a high fraction of unexplained variance in our RNA-Seq dataset and used surrogate variable analysis to address this issue (see Methods and Supplementary Figure 1). We then performed DEG analysis to compare all mice groups with each other, from APP/PS1 mice exposed to either BCI-838, PE or a combination, as well as WT mice. DEGs were identified at a false discovery rate (FDR) of 0.05 (see Supplementary Table 1 for a list of all DEGs for all comparisons). To investigate biological pathways that might be differentially dysregulated by administration of BCI-838 or exposure to exercise, we performed gene set enrichment analysis (GSEA) on the identified DEGs, resulting in enriched biological pathways, gene ontology sets, and transcription factor binding sites at an adjusted p-value < 0.05 (see Supplementary Table 2 for GSEA results). Top DEGs and GSEA results sorted by their adjusted p-values are summarized in Figure 5.
Figure 5. Differential gene expression and enrichment analysis summary in dentate gyrus (DG) of APP/PS1 mice treated with BCI-838, PE or a combination of both BCI-838 + PE. RNA sequencing was performed on dentate gyrus for five groups of four-month-old APP/PS1 and WT mice, comprising a total of 24 samples. (A) Schematic overview of mouse AD transcriptome analysis. (B) Top DEGs in DG of APP/PS1 mice treated with BCI-838 vs. APP/PS1 treated with vehicle. (C) Top DEGs in DG of APP/PS1 mice treated with PE vs. APP/PS1 treated with vehicle. (D) Top DEGs in DG of APP/PS1 mice treated with a combination of both BCI-838 and PE vs. APP/PS1 treated with vehicle. (E) Top DEGs in DG of APP/PS1 mice treated with a combination of both BCI-838 and PE vs. APP/PS1 treated with PE. (F) Venn Diagram of DEGs and selected pathway enrichments of known or suspected relevance to AD pathophysiology, shared across different comparisons of APP/PS1 mice groups (transcription factor enrichments shown in red).
The most striking changes across all groups were the transcriptomic changes induced by one month of BCI-838 administration in the DG of APP/PS1 (APP/PS1 + BCI-838), which identified 319 DEGs. One hundred ninety-one up-regulated DEGs from this analysis included BDNF, as well as PIK3C2A of the PI3K-mTOR pathway, whereas 128 down-regulated DEGs included IL11RA1, the interleukin 11 receptor of the AKT signaling pathway, as well as EIF5A, the eukaryotic translation initiation factor. Up-regulated DEGs also included ITGB8 of the integrin family, an extracellular matrix (ECM) receptor, known to regulate neurogenesis and neurovascular homeostasis in adult brain (Mobley et al., 2009). Of further relevance, dysregulation of several GABA (inhibitory) receptors, GABRB1, GABRB2, and GABRD, and up-regulation of two metabotropic glutamate receptor subunits, GRM1 and GRM5, and the ATP- binding AD risk gene ABCA1 (Lupton et al., 2014) were identified among DEGs. GSEA pointed to SP1 as the top transcription factor binding site for down-regulated DEGs; other differentially regulated pathways included neurodegenerative diseases, such as AD and Huntington disease, whereas up-regulated DEGs were found enriched for glutamate receptor activity and AKT1 knockdown pathways as expected.
After 1 month of PE, the transcriptomes of the hippocampal DG of APP/PS1 mice (APP/PS1 + PE) were compared to those of APP/PS1 control mice, leading to the identification of seven DEGs (two up-regulated, five down-regulated) that were found to be enriched for transcripts associated with “response to mTOR inhibitor,” “extracellular matrix receptor reaction,” “trigeminal nerve development,” “peripheral nervous system neuron development,” and “polycomb repressive complex component SUZ12,” which plays a critical role in regulating neurogenic potential and differentiation of embryonic stem cells (Pasini et al., 2007). Meanwhile, compared with untreated APP/PS1 mice, in APP/PS1 mice treated with the combination of PE and BCI-838 (APP/PS1 combination) for 1 month, 17 DEGs were identified (seven up-regulated, 10 down-regulated), which were enriched for SUZ12, as well as glycogen metabolism and exercise-induced myalgia pathways. When APP/PS1 combination was compared with APP/PS1 + BCI-838, we did not observe any DEGs. However, when APP/PS1 combination was compared with APP/PS1 + PE, we identified 772 DEGs, which were found to be enriched in EIF3K and REST transcription factors, both shown to regulate the mTOR signaling pathway in adipocyte yeast (Harris et al., 2006) and oral cancer cells (Cho et al., 2015), respectively. Cadherin signaling, axon guidance mediated by netrin, epidermal growth factor (EGF) receptor signaling, SYK inhibition and knockdown were also among differential pathways of relevance with strong associations to mTOR activity and signaling, suggesting neurogenesis and PE, thereby demonstrating the validity of our RNA-Seq data and experiment.
Finally, a comparison of the transcriptomes of the hippocampal DG of APP/PS1 control mice against those of WT mice showed 60 DEGs (41 upregulated, 19 down-regulated) found to be enriched in calcium cation antiporter activity and ATP-dependent microtubule motor activity pathways, in line with highlighted findings from our reported transcriptomic analysis of APP/PS1 vs. WT (Readhead et al., 2016).
Increased levels of BDNF have been implicated in the beneficial effects of PE in 5xFAD transgenic mice (Choi et al., 2018). Figure 6 shows BDNF RNA levels in mice following treatment with PE and BCI-838. A one-way ANOVA did not indicate between group differences in BDNF RNA levels. Only if a Kuskall–Wallis non-parametric test, followed by an uncorrected Dunn test, were used were BDNF mRNA levels found to be significantly increased in APP/PS1 mice treated with BCI-838, but not in APP/PS1 mice treated with PE (Figure 6, p = 0.0255, Kuskall–Wallis).
Figure 6. BDNF analysis in APP/PS1 mice treated with BCI-838 or PE. qPCR for BDNF mRNA. Asterisk indicates p = 0.025 (Kuskall–Wallis, uncorrected Dunn test). Five mice per group were used for the analysis.
We performed computational drug repurposing to identify compounds that induce a transcriptomically similar profile to BCI-838 using a modified Connectivity Mapping (Lamb et al., 2006) approach (Figure 7). We then performed a chemogenomic enrichment analysis (Readhead et al., 2018) on these compounds to identify drug targets and side effects that were enriched among compounds that were transcriptomically similar to BCI-838 (see Supplementary Table 3 for drug repurposing results). We observed enrichment for protein targets that included several glutamate receptor targets, including GRL1A, NMDE3, GRM2, GRM3, and NMD3B. We also found transcriptomic similarity with other drug targets, such as KDM1A [whose inhibition has been shown to restore adult neurogenesis and improve hippocampal dependent memory (Zhang et al., 2021)] and NLRP3 [which plays a role in regulation of inflammation, immune response, and apoptosis (Hirota et al., 2011)]. We also observed that drugs that were transcriptomically similar to BCI-838 were enriched for the side effect of “increasing blood ketones” as well as associated with “pain relief” (Figure 8). A ketogenic diet has been shown to inhibit the mTOR pathway (McDaniel et al., 2011). Glutamatergic neurotransmission plays a major role in pain sensation and transmission from peripheral tissues into the CNS (Wozniak et al., 2012). These observations comport with findings from DEG and pathway analysis and may suggest follow-up experiments to look for ketogenesis among BCI-838-treated mice.
Figure 8. Drug targets and side effects enriched among compounds with transcriptomic similarity to BCI-838.
Metabotropic glutamate receptors (mGluRs) play important roles in regulating glutamatergic neurotransmission. Agents that modulate mGluR2/3 activity have gained attention as possible therapeutic agents for a range of mental health-related disorders (Chaki, 2019). BCI-838 is an mGluR2/3 antagonist pro-drug that is metabolized in the liver into the active metabolite BCI-632. We previously reported that BCI-838 administration reduced anxiety and improved learning behavior in a mouse model of accumulation of oligomeric AβE22Q (Kim et al., 2014). More recently, we showed that BCI-838 treatment reversed anxiety- and post-traumatic stress disorder-related traits in a rat model of mild blast injury (Perez-Garcia et al., 2018). In both studies, BCI-838 treatment increased brain levels of markers of neurogenesis (BrdU, DCX, PCNA), reduced anxiety-related behaviors, and reversed learning behavior deficits.
Here we examined the effects of BCI-838, PE, or the combination, in an additional AD mouse model (APP/PS1) that exhibits both oligomeric and fibrillar amyloid pathology. We show that administration of BCI-838 alone or in combination with PE led to stimulation of AHN and improvement in recognition memory. Furthermore, transcriptomic analysis of the hippocampal dentate gyrus following BCI-838 treatment showed up-regulation of BDNF, metabotropic glutamate receptors, and effects on modulators of the PI3K-mTOR pathway. The only general effect of BCI-838 on behavior was that the drug reduced running activity after 2 weeks of treatment, an effect that does not seem to have been previously described for BCI-838 or other compounds in this drug class. It is unlikely however that reduced motor activity in BCI-838 treated mice impacted behavioral test results since exploratory behavior in NOR testing increased in BCI-838 treated mice.
Like BCI-838, PE stimulates neurogenesis in the hippocampal DG (Liu and Nusslock, 2018). Another purpose of the current study was to investigate whether BCI-838 treatment combined with PE could have synergistic effects on recognition memory and AHN in APP/PS1 transgenic mice. Both BCI-838 and PE were associated with restored recognition memory in APP/PS1 mice in a NOR test which is dependent on hippocampal and perirhinal function.
While it is tempting to draw direct comparisons between AD mouse models and human AD, significant differences seem to exist. APP/PS1 mice develop age related amyloid plaque deposition (Borchelt et al., 1996). However, unlike humans, transgenic mice exhibit cognitive findings before amyloid deposition is present (as shown by the NOR testing in this study) and learning behavior impairments tend to progress in parallel with amyloid plaque deposition (Howlett et al., 2004; Morgan, 2006). Transgenic mice also typically lack the tau pathology and synaptic loss characteristic of human AD (Ashe and Zahs, 2010). Yet despite the imperfect correlation between mouse models and human AD, the current study suggests that BCI-838 and exercise might be beneficial in the pre-symptomatic and/or MCI phases of AD. Interestingly, BCI-838 also reduces Aβ monomers and oligomers (Kim et al., 2014). Thus, BCI-838 could be acting through multiple mechanisms, some amyloid dependent and others not. Future studies in APP/PS1 mice at older ages (e.g., 5 or 6 months) when levels of oligomeric Aβ and amyloid deposition are increasing rapidly will be of interest. More extended treatment regimens (e.g., 2–3 months) beginning at 3 months of age (or earlier) could provide support for the notion that BCI-838 might be useful for some form of prophylaxis in humans.
Alone or in combination, BCI-838 administration and PE enhanced hippocampal neurogenesis. This conclusion was supported both by BrdU injections given 24 h before sacrifice (which provided a measure of neural progenitor proliferation) and by counts of DCX-labeled cells, which provided a measure of the steady-state population of young neurons. Using either measure, BrdU- and DCX-labeled cells were increased following drug treatment or the combination of exercise and drug treatment when compared to APP/PS1 mice treated with vehicle. However, comparing BCI-838 alone to the combination group did not lead to any obvious additional benefit of adding BCI-838 to PE. We interpret this as suggesting that BCI-838 acts to mimic aspects of exercise's effects rather than to act synergistically to enhance it. The fact that no DEGs were observed when comparing the combination group with BCI-838 alone further supports the notion that the BCI-838 effect is to mimic rather than synergistically enhance PE.
Of note, in our studies, neurogenesis was not impaired in APP/PS1 mice treated with vehicle when compared to WT mice. Prior studies in AD transgenic mice have produced inconsistent results concerning the effect of familial AD mutations on AHN (Jin et al., 2004; Wen et al., 2004; Kuhn et al., 2007; Taniuchi et al., 2007; Verret et al., 2007; Niidome et al., 2008; Yu et al., 2009; Chuang, 2010; Demars et al., 2010; Elder et al., 2010; Krezymon et al., 2013; Unger et al., 2016), including in the APP/PS1 mice studied here (Taniuchi et al., 2007; Verret et al., 2007; Niidome et al., 2008; Yu et al., 2009; Chuang, 2010; Unger et al., 2016). The absence of impaired AHN in APP/PS1 mice in this study, compared to WT mice suggests that the effect of BCI-838 and exercise on the neurogenesis may be an enhancement of baseline function rather than restoration of disease-related deficiency. This point may be important in determining whether PE is most effective administered as prophylaxis vs. retardation of symptom progression.
Most studies suggest that voluntary running alone increases adult neural progenitor proliferation, while other manipulations such as environmental enrichment favor survival of adult-born dentate granule neurons and increased integration of immature neurons into neuronal circuits (Van Praag et al., 2005; Ramirez-Amaya et al., 2006; Toda et al., 2019). Learning itself also promotes functional integration of new neurons into behaviorally relevant networks, in particular recruiting higher proportions of younger rather than older born cells into hippocampal assemblies (Ramirez-Amaya et al., 2006). Additional studies will be required to determine the fate of newly generated cells and whether BCI-838 and/or PE stimulate the production of new neurons that become integrated into functional neuronal networks as well as whether enhancement of neurogenesis is required for the effects of BCI-838 with or without PE.
Why an mGluR2/3 receptor antagonist should mimic some effects of PE is unclear. PE exerts a variety of beneficial effects on neuroplasticity, spatial learning, and memory (Caselli and Reiman, 2013). Neurogenesis in adult brain is likely regulated through a combination of factors (Obernier and Alvarez-Buylla, 2019), and PE also acts on multiple pathways (Buchman et al., 2019). Mechanistically, many studies have emphasized PE-related effects on trophic factor production, especially BDNF, insulin-like growth factor-1 (IGF-1), and vascular endothelial growth factor (VEGF) (Cassilhas et al., 2016). A recent study in AD transgenic 5xFAD mice further demonstrated that exercise-induced AHN improved cognition along with increased levels of BDNF (Choi et al., 2018).
More recent work has emphasized how exercise affects secreted factors in the periphery (Cooper et al., 2018). Myokines secreted by muscle during exercise improve learning and memory by regulating hippocampal function (Lourenco et al., 2019; Pedersen, 2019). Cathepsin B, a PE-related myokine, crosses the blood-brain barrier and enhances BDNF production (Lourenco et al., 2019). Furthermore, irisin, the cleaved and circulating form of the exercise-induced fibronectin type III domain-containing membrane protein (FNDC5), stimulates BDNF production (Rabiee et al., 2020; Islam et al., 2021); its genetic deletion was shown to impair cognitive function in exercise, aging and AD; and its peripheral application was observed to be sufficient to rescue the learning behavior decline in mouse models of AD (Islam et al., 2021). We did not detect FNDC5 as a DEG in the DG of the APP/PSEN1 either following BCI-838 administration or after PE vs. sedentary, which may be due to the relatively young age of the APP/PS1 mice used and the absence of neuronal and synaptic loss at this age in this model. The length of voluntary PE may also have been a factor.
Indeed, one of the most important recent clues to the molecular basis for PE may be the discovery of irisin by Lourenco et al. (2019). This was recently confirmed and extended by Islam et al. (2021). Moreover, while our paper was under review, Kam et al. (2022), reported that irisin can ameliorate synuclein pathology in mouse models of Parkinson's disease. Integrins are receptors for irisin in bone and fat cells (Kim et al., 2018). Single-cell RNA-seq data from the murine DG found ITGAV expressed in neuronal and non-neuronal cells and ITGB5 expressed in astrocytes and microglia, raising the possibility that irisin might mediate glia activation through integrin receptor complexes (Islam et al., 2021). When we scrutinized our data for possible evidence of transcriptomic changes among integrin family members, we observed upregulation of ITGB8, an ECM receptor that regulates neurogenesis and neurovascular homeostasis in the adult brain (Mobley et al., 2009), following BCI-838 administration to APP/PS1 mice, at an FDR of 5%, as well as ITGB6 and ITGB7 at an FDR of 1%. Future studies will be needed to determine whether the effects of BCI-838 may be through irisin-related signaling (Rabiee et al., 2020).
mGluR2/3 receptors function primarily as presynaptic autoreceptors that, when stimulated, inhibit glutamate release (Koike et al., 2011; Chaki, 2019). The antidepressant effects of agents acting on the mGlu2/3 receptor have been widely studied in animal models. mGlu2/3 receptor blockade appears to act at least in part through mTOR signaling, which may contribute to the sustained antidepressant-like effects of mGlu2/3 receptor antagonists (Chaki and Fukumoto, 2018). However, the full synaptic and neural mechanisms of their action (including how mGluR2/3 antagonists stimulate AHN) remain to be clarified (Koike et al., 2011; Kim et al., 2019).
In this study, PE did not significantly influence AHN. The study did not include a group of WT mice treated with PE alone. Therefore, there was no internal control for the effect of PE on AHN in WT mice. The effect of PE on AHN in transgenic AD models has not been consistent. In 5x FAD mice, 4 months of voluntary PE was found to increase AHN (Choi et al., 2018). Two studies found increased AHN in APP/PS1 mice (Falkenhain et al., 2020; Yu et al., 2021). However, another study found that PE did not alter the number of BrdU positive cells in the dentate gyrus of APP/PS1 mice (Zhang et al., 2022). In our own studies, we saw a trend toward PE increasing anti-doublecortin (anti-DCX) labeled cells in the DG of APP/PS1 mice treated with PE alone (Figure 4), but this trend only reached statistical significance if no correction for multiple comparisons was made. By contrast, BCI-838 or BCI-838 + PE clearly increased the number of DCX and BrdU labeled cells (Figure 4). Differences between our studies and other studies may reflect differences in the model(s) used (e.g., 5x FAD vs. APP/PS1) as well as differences in ages of the animals studied, the PE interval, and whether PE was voluntary or forced.
Work in transgenic 5xFAD mice (Choi et al., 2018) revealed that chronic voluntary PE in running wheels for 4 months, beginning at 2 months of age, improved learning behavior in 5xFAD mice; our study revealed improvement in 1 month of PE. PE-mediated enhancement of neurogenesis in hippocampus is suggested to be mediated through BDNF signaling (Cooper et al., 2018). In our study, while a short treatment with BCI-838 or PE was associated with increased neurogenesis in the DG, the trend toward increased levels of BDNF did not reach statistical significance. However, APP/PS1 mice treated with BCI-838 and PE displayed indistinguishable levels of BDNF when compared with PE-treated mice, even though they spent less time on running wheels. This effect shows the potential of BCI-838 to mimic the effects of PE on BDNF expression in the DG. Future studies with larger sample sizes will be needed to better establish the effects of BCI-838 on BDNF levels in relationship to PE.
While attractive as a contributing factor, modulation of hippocampal levels of BDNF is unlikely to provide a complete explanation for the effects we observed. Unlike treatment with P7C3 studied by Choi et al. (2018), BCI-838 stimulated AHN and improved recognition memory without addition of PE. Thus BCI-838 may provide a pharmacological mimic of some aspects of PE, in its effects on behavior.
Our findings further demonstrate that administration of BCI-838 alone induced transcriptomic changes that resulted in up-regulation of BDNF and PIK3C2A of mTOR signaling, down-regulation of IL11RA1 interleukin receptor of AKT signaling, and enriched SP1 and further relevant pathways, implicating neurogenesis and effects of PE. These findings are especially notable as the mTOR pathway is involved in many aspects of neurogenesis as a regulator of cellular energy metabolism, a nutrient sensor and growth factor inducer via insulin, IGF-1 and BDNF (LiCausi and Hartman, 2018; Querfurth and Lee, 2021). The mTORC1 protein complex controls protein synthesis by phosphorylating downstream targets essential for mRNA translation, 4E-BP1 (eIF-4E binding protein) and ribosome biogenesis (Swiech et al., 2008). Neuronal growth factors known to support learning and memory such as BDNF and EGF do so through mTOR activation. Furthermore, in relation to the AD brain being an insulin-resistant organ, mTORC1 has been shown to be involved with down-regulating insulin/Akt signaling through an inactivating phosphorylation of IRS-1 (Tzatsos and Kandror, 2006).
Mechanistically, BCI-838 is considered an orally active ketamine-mimetic, and recent evidence indicates that ketamine modulates mTOR activity and the actions of the physiological effectors of elongation inhibitory factor 4E (eIF4E) and its protein partners, eIF4E binding proteins (eIF4E-BPs). This is also consistent with our findings of dysregulation of a related eukaryotic translation initiation factor, EIF5A. mTOR is known to modulate autophagy (Dossou and Basu, 2019). Given the behavioral benefits of BCI-838 in multiple types of proteopathies (Perez-Garcia et al., 2023) its apparent similarity to ketamine regarding mTOR modulation, and the role for mTOR in autophagy, we propose that, like ketamine, BCI-838 exerts its actions through protein translation and autophagy.
A drug repurposing analysis identified several known compounds transcriptomically similar to BCI-838. Of these compounds some have been suggested as having a potential role in AD pathophysiology or therapy. In particular, meptazinol derivatives are dual inhibitors of cholinesterases and amyloid-beta aggregation with multiple studies showing effectiveness of these derivatives in APP/PS1 mice (Liu et al., 2013; Shao et al., 2014; Shi et al., 2018; Wang et al., 2019). Several studies have identified a puromycin-sensitive aminopeptidase as a potential inhibitor of tau (Karsten et al., 2006; Sengupta et al., 2006; Hui, 2007; Kudo et al., 2011) and Aβ related neurodegeneration (Kruppa et al., 2013). Why puromycin which inhibits this aminopeptidase (Constam et al., 1995) should have transcriptional effects similar to BCI-838 is unclear. Gramine derivatives have been suggested to potentially exert neuroprotective effects in neurodegenerative disorders including AD through their ability to ameliorate calcium overload and modify serine/threonine proteases (Lajarin-Cuesta et al., 2016; Gonzalez et al., 2018). MG-132 is a proteosome inhibitor commonly used to experimentally induce proteinopathy related neurodegeneration (Posimo et al., 2015; Heinemann et al., 2016) although in one study MG-132 restored impaired activity-dependent synaptic plasticity as well as associative long-term memory in APP/PS1 mice, an effect possibly mediated through stimulation of the mTOR pathway (Krishna et al., 2020). Future studies will be needed to understand the complex transcriptional effects of BCI-838 and these related compounds.
Previous studies explored the effects of PE or mGluR2/3 modulation alone on AD-related pathology (Kim et al., 2014; Tapia-Rojas et al., 2016). However, none explored the effect of the combination of mGluR2/3 modulation and PE in the early stages of AD development in a mouse model. Here we show that BCI-838 improved behavior in APP/PS1 mice and stimulated AHN. Combined with our previous studies in another AD mouse model (Kim et al., 2014), a rat model of blast-related traumatic brain injury (Perez-Garcia et al., 2018) and the PS19 MAPTP301S mouse model of tauopathy (Perez-Garcia et al., 2023), four preclinical studies now support the effectiveness of BCI-838 for relief of a range of models of neurocognitive, neurobehavioral, and neurodegenerative traits. The ability of BCI-838 to recapitulate many of the effects of PE suggests that this and/or other mGluR2/3 antagonists may represent safe and novel pharmacological mimics of PE. If this notion regarding pharmacological mimicry of PE proves to be true, it will be worth evaluating whether these drugs can prevent, delay, and/or treat neurodegenerative and/or post-traumatic disorders of cognition, anxiety, and/or behavior.
Several study limitations should be mentioned. The experimental design did not include non-transgenic wild type mice treated with BCI-838. The rationale for this exclusion was to limit animal usage to the minimum needed to achieve the experimental goal of determining the effects of BCI-838 on behavior in APP/PS1 mice. In the design utilized (Figure 1), we reasoned that comparison of the single group of vehicle treated WT mice (group 5) to vehicle treated APP/PS1 mice (group 1) served as a positive control for appearance of the transgene-related behavioral phenotype while comparison of BCI-838 +/– PE treated APP/PS1 mice (groups 2–4) to vehicle treated APP/PS1 mice (group 1) measured effectiveness of BCI-838 treatment.
The BCI-838 effect is unlikely to be transgene-specific in that as noted above BCI-838 has been shown to rescue behavioral deficits in a transgenic model using a different AD transgene (Kim et al., 2014). BCI-838 also rescued behavioral deficits in wild type rats subjected to blast exposure (Perez-Garcia et al., 2018) and recently proved effective in reversing impaired recognition memory in the PS19 MAPTP301S mouse model of tauopathy (Perez-Garcia et al., 2023). In addition, as a class, mGluR2/3 receptor antagonists show anti-depressant like action in a variety of stress induced models using wild type mice or rats (Chaki, 2019). We note that in the previous study utilizing Dutch APP (APPE693Q) transgenic mice, while BCI-838 improved learning in BCI-838 treated Dutch APP mice, the drug had no effect on NOR or cued fear learning in non-transgenic wild type mice (Kim et al., 2014).
In addition, we do not know how behavior may affect drug, exercise and transgene interactions on RNA transcriptomics. In this study, a conscious decision was made not to perform transcriptional analysis on mice that had undergone behavioral testing. This decision was made to focus attention on the static state changes induced by the drug, exercise, and drug/exercise interactions alone without the added complication of behavioral interactions. The transcriptional studies provide a correlation with behavior in that they represent the state of the animals before they underwent behavioral testing. However, in humans, there are always effects of behavioral interactions. It therefore will be of interest to perform additional transcriptomics studies after selected behavioral testing. To understand BCI-838's potentially complex effects, the study of additional BCI-838 treated WT mice will be essential. The choice of these studies will be facilitated by results of the present work.
The conclusions of this study are also limited by the restricted number of behavioral tests that were performed. In particular, anxiety and depression are now understood to be core clinical features of AD, and both could affect performance in cognitive tasks. Future studies including an expanded range of behavioral tests will be needed to more fully understand BCI-838's effects. A final limitation of the current studies is that we only included male mice, which reduces the generalizability of the findings. There indeed may be sex-specific differences in response to BCI-838, and there are sex differences in amyloid deposition in APP/PS1 mice (Wang et al., 2003). Future studies in female mice will be important.
Lifestyle modification is being widely studied as a disease-modifying intervention for AD. PE was a major component of the FINGER trial (Ngandu et al., 2015; Wimo et al., 2022). Thus understanding the action of compounds like BCI-838, which apparently comprise a combination of PE-related and PE-independent effects, offers new opportunities to better illuminate these mechanisms, perhaps extend them and inform the development of new therapeutic strategies for this devastating disease.
While we do not mean to imply that BCI-838 could or should replace PE in otherwise healthy individuals, there are many individuals with chronic medical or physical conditions that limit their full participation in regular PE programs. For such individuals, a drug like BCI-838 might be a reasonable way to supplement an inability to fully participate in a formal PE program. In humans, the application of PE may well need to be individualized in relationship to diet, lifestyle, genetic background, and education, all goals of modern personalized medicine, making it perhaps even more important to understand the effects of PE alone in an animal model where these effects can be isolated.
The pharmacokinetics, safety and tolerability of BCI-838 have been evaluated through phase 1 human studies, which found BCI-838 well tolerated in healthy subjects without serious adverse effects (Clinicaltrials.gov1, 2012; Clinicaltrials.gov2, 2012). Thus, data from both experimental animals and humans suggest that BCI-838 is safe and ready to be advanced into human clinical trials.
PE has beneficial neuroprotective and pro-cognitive effects including stimulating AHN. PE has been widely studied as an intervention that may delay the development of AD. Here we show that BCI-838 improved recognition memory and enhanced AHN in an APP/PS1 mouse AD model. Transcriptional analysis of the hippocampal dentate gyrus following BCI-838 treatment showed up-regulation of brain BDNF, PIK3C2A of the PI3K-mTOR pathway and down-regulation of EIF5A a modulator mTOR activity. BCI-838 is thus a safe orally active compound capable of mimicking some of the beneficial effects of PE.
The original contributions presented in the study are publicly available. This data can be found here: https://www.ncbi.nlm.nih.gov/, GSE203554.
The animal study was reviewed and approved by Institutional Animal Care and Use Committee (IACUC) of the James J. Peters VA Medical Center.
CB and FHG identified the key pro-neurogenic action of BCI-838 and supplied the drug for these studies. ME, SG, GPG, JD, and GE designed the study. GPG, J-VH-M, GP, and AO-P performed the experiments. GPG, BR, MB, and GE analyzed data. GPG, MB, SG, ME, JD, J-VH-M, BG, BR, MS, and GE wrote the paper. All authors have read and approved the final manuscript.
This work was supported by the Department of Veterans Affairs, Veterans Health Administration, Rehabilitation Research and Development Service Awards 1I01RX000684 (SG), 1I01RX002333 (SG), 101RX002660 (GE), 1I01RX003846 (GE), 1I21RX003459-01 (MG), 1I21RX002069-01 (MG), and 1I21RX002876-01 (MG), the Department of Veterans Affairs Office of Research and Development Basic Medical Research Service awards 1I01BX004067 (GE) and 1I01BX005882-01 (GE), AHA-Allen Initiative in Brain Health and Cognitive Impairment award (FHG), the American Heart Association (FHG), The Paul G. Allen Frontiers Group: 19PABH134610000 (FHG), the Milky Way Research Foundation (FHG), NIH grants: R37 AG072502-02, P30 AG068635-03, P01 AG051449-07, and RF1 AG056306-06 (all to FHG).
JB was employed by Generable, Inc. CB was employed by E-Scape Bio. MS has served as a consultant for Bayer Schering Pharma, Bristol-Meyers Squibb, Elan Corporation, Genentech, Medivation, Medpace, Pfizer, Janssen, Takeda Pharmaceutical Company Limited, and United Biosource Corporation. She receives research support from the NIH. FHG was a founder and member of the SAB for BCI, but he no longer serves as a consultant or has stock because BCI no longer exists as a company. He has received funding from NIA, HIH, and NIMH on projects related to adult neurogenesis but not related to this or related compounds. CB is a former employee of BrainCells, Inc. BrainCells, Inc. provided drug and advice. JD has served as a consultant for Janssen and is an equity holder in Thorne HealthTech. BG is a consultant for Anthem AI and a scientific advisor and consultant for Prometheus Biosciences. He has received consulting fees from GLG Research and honoraria from Virtual EP Connect. ME receives research support from the NIH, the XDP Foundation, and the Cure Alzheimer's Fund. SG is a co-founder of Recuerdo Pharmaceuticals. He has served as a consultant in the past for J&J, Diagenic, and Pfizer, and he currently consults for Cognito Therapeutics, GLG Group, SVB Securities, Guidepoint, Third Bridge, Leerink, MEDACORP, Altpep, Vigil Neurosciences, and Eisai. He has received research support from Warner-Lambert, Pfizer, Baxter Healthcare, Amicus, Avid, and ADDF. He served on the DSMB for an amyloid vaccine trial by Elan Pharmaceuticals. He receives research support from the VA, NIH, and the Cure Alzheimer's Fund. SG and ME have received compensation for chart review in the areas of cognitive neurology and pediatric neurology, respectively.
The remaining authors declare that the research was conducted in the absence of any commercial or financial relationships that could be construed as a potential conflict of interest.
The author(s) SG, BG, FHG, MG, and J-VH-M declare that they were editors board member of Frontiers, at the time of submission. This had no impact on the peer review process and the final decision.
All claims expressed in this article are solely those of the authors and do not necessarily represent those of their affiliated organizations, or those of the publisher, the editors and the reviewers. Any product that may be evaluated in this article, or claim that may be made by its manufacturer, is not guaranteed or endorsed by the publisher.
The Supplementary Material for this article can be found online at: https://www.frontiersin.org/articles/10.3389/frdem.2023.1198006/full#supplementary-material
AD, Alzheimer's disease; MCI, mild cognitive impairment; AHN, adult hippocampal neurogenesis; APP/PS1 mice, APPKM670/671NL/PSEN1Δexon9; BDNF, brain-derived neurotrophic factor; BrdU, bromodeoxyuridine; CNS, central nervous system; DG, dentate gyrus; DCX, doublecortin; FNDC5, fibronectin type III domain-containing membrane protein; FO, familiar object; eIF4E-BPs, eIF4E binding proteins; IGF-1, insulin-like growth factor-1; LTM, long term memory; mGluR2/3, metabotropic glutamate receptors type II; mTOR, mammalian target of rapamycin; NO, novel object; NOR, novel object recognition; Ob1,2, object 1 or object 2; VEGF, vascular endothelial growth factor; PE, physical exercise; PI3K, phosphoinositide 3-kinases; PIK3C2A, phosphatidylinositol-4-phosphate 3-kinase catalytic subunit type 2 alpha; qPCR, quantitative real-time PCR; SVZ, Subventricular zone; WT, wild-type.
Ashe, K. H., and Zahs, K. R. (2010). Probing the biology of Alzheimer's disease in mice. Neuron 66, 631–645. doi: 10.1016/j.neuron.2010.04.031
Bo, H., Kang, W., Jiang, N., Wang, X., Zhang, Y., Ji, L. L., et al. (2014). Exercise-induced neuroprotection of hippocampus in APP/PS1 transgenic mice via upregulation of mitochondrial 8-oxoguanine DNA glycosylase. Oxid. Med. Cell. Longev. 2014, 834502. doi: 10.1155/2014/834502
Borchelt, D. R., Thinakaran, G., Eckman, C. B., Lee, M. K., Davenport, F., Ratovitsky, T., et al. (1996). Familial Alzheimer's disease-linked presenilin 1 variants elevate Abeta1-42/1-40 ratio in vitro and in vivo. Neuron 17, 1005–1013. doi: 10.1016/S0896-6273(00)80230-5
Buchman, A. S., Yu, L., Wilson, R. S., Lim, A., Dawe, R. J., Gaiteri, C., et al. (2019). Physical activity, common brain pathologies, and cognition in community-dwelling older adults. Neurology 92, e811–e822. doi: 10.1212/WNL.0000000000006954
Casaletto, K., Ramos-Miguel, A., Vandebunte, A., Memel, M., Buchman, A., Bennett, D., et al. (2022). Late-life physical activity relates to brain tissue synaptic integrity markers in older adults. Alzheimers Dement. 8, 2023–2035. doi: 10.1002/alz.12530
Caselli, R. J., and Reiman, E. M. (2013). Characterizing the preclinical stages of Alzheimer's disease and the prospect of presymptomatic intervention. J. Alzheimers. Dis. 33(Suppl 1), S405–S416. doi: 10.3233/JAD-2012-129026
Cassilhas, R. C., Tufik, S., and Mello, D. e. M. T. (2016). Physical exercise, neuroplasticity, spatial learning and memory. Cell. Mol. Life Sci. 73, 975–983. doi: 10.1007/s00018-015-2102-0
Chaki, S. (2019). mGlu2/3 receptor antagonists. Adv. Pharmacol. 86, 97–120. doi: 10.1016/bs.apha.2019.03.004
Chaki, S., and Fukumoto, K. (2018). mGlu receptors as potential targets for novel antidepressants. Curr. Opin. Pharmacol. 38, 24–30. doi: 10.1016/j.coph.2018.02.001
Cho, E., Moon, S. M., Park, B. R., Kim, D. K., Lee, B. K., Kim, C. S., et al. (2015). NRSF/REST regulates the mTOR signaling pathway in oral cancer cells. Oncol. Rep. 33, 1459–1464. doi: 10.3892/or.2014.3675
Choi, S. H., Bylykbashi, E., Chatila, Z. K., Lee, S. W., Pulli, B., Clemenson, G. D., et al. (2018). Combined adult neurogenesis and BDNF mimic exercise effects on cognition in an Alzheimer's mouse model. Science 361, eaan8821. doi: 10.1126/science.aan8821
Chuang, T. T. (2010). Neurogenesis in mouse models of Alzheimer's disease. Biochim. Biophys. Acta 1802, 872–880. doi: 10.1016/j.bbadis.2009.12.008
Clinicaltrials.gov1 (2012). “A study of BCI-838 and several BCI-632 prodrugs in healthy volunteers,” in National Library of Medicine, B. M., 2000 (ed.).
Clinicaltrials.gov2 (2012). “A randomized, double-blind, placebo-controlled, multiple ascending dose, safety, tolerability, pharmacokinetic and pharmacodynamic study of BCI 838 in healthy adult subjects,” in National Library of Medicine, B. M., 2000 (ed.).
Constam, D. B., Tobler, A. R., Rensing-Ehl, A., Kemler, I., Hersh, L. B., Fontana, A., et al. (1995). Puromycin-sensitive aminopeptidase. Sequence analysis, expression, and functional characterization. J. Biol. Chem. 270, 26931–26939. doi: 10.1074/jbc.270.45.26931
Cooper, C., Moon, H. Y., and Van Praag, H. (2018). On the run for hippocampal plasticity. Cold Spring Harb. Perspect. Med. 8, a029736. doi: 10.1101/cshperspect.a029736
Demars, M., Hu, Y. S., Gadadhar, A., and Lazarov, O. (2010). Impaired neurogenesis is an early event in the etiology of familial Alzheimer's disease in transgenic mice. J. Neurosci. Res. 88, 2103–2117. doi: 10.1002/jnr.22387
Dobin, A., Davis, C. A., Schlesinger, F., Drenkow, J., Zaleski, C., Jha, S., et al. (2013). STAR: ultrafast universal RNA-seq aligner. Bioinformatics 29, 15–21. doi: 10.1093/bioinformatics/bts635
Dossou, A. S., and Basu, A. (2019). The emerging roles of mTORC1 in macromanaging autophagy. Cancers 11, 1422. doi: 10.3390/cancers11101422
Elder, G. A., Gama Sosa, M. A., De Gasperi, R., Dickstein, D. L., and Hof, P. R. (2010). Presenilin transgenic mice as models of Alzheimer's disease. Brain Struct. Funct. 214, 127–143. doi: 10.1007/s00429-009-0227-3
Falkenhain, K., Ruiz-Uribe, N. E., Haft-Javaherian, M., Ali, M., Stall, C., Michelucci, P. E., et al. (2020). A pilot study investigating the effects of voluntary exercise on capillary stalling and cerebral blood flow in the APP/PS1 mouse model of Alzheimer's disease. PLoS ONE 15, e0235691. doi: 10.1371/journal.pone.0235691
Gage, F. H. (2021). Adult neurogenesis in neurological diseases. Science 374, 1049–1050. doi: 10.1126/science.abm7468
Gonzalez, D., Arribas, R. L., Viejo, L., Lajarin-Cuesta, R., and de Los Rios, C. (2018). Substituent effect of N-benzylated gramine derivatives that prevent the PP2A inhibition and dissipate the neuronal Ca(2+) overload, as a multitarget strategy for the treatment of Alzheimer's disease. Bioorg. Med. Chem. 26, 2551–2560. doi: 10.1016/j.bmc.2018.04.019
Hanson, N. D., Owens, M. J., and Nemeroff, C. B. (2011). Depression, antidepressants, and neurogenesis: a critical reappraisal. Neuropsychopharmacology 36, 2589–2602. doi: 10.1038/npp.2011.220
Harris, T. E., Chi, A., Shabanowitz, J., Hunt, D. F., Rhoads, R. E., and Lawrence, J. C. Jr. (2006). mTOR-dependent stimulation of the association of eIF4G and eIF3 by insulin. EMBO J. 25, 1659–1668. doi: 10.1038/sj.emboj.7601047
Heinemann, S. D., Posimo, J. M., Mason, D. M., Hutchison, D. F., and Leak, R. K. (2016). Synergistic stress exacerbation in hippocampal neurons: evidence favoring the dual-hit hypothesis of neurodegeneration. Hippocampus 26, 980–994. doi: 10.1002/hipo.22580
Hirota, S. A., Ng, J., Lueng, A., Khajah, M., Parhar, K., Li, Y., et al. (2011). NLRP3 inflammasome plays a key role in the regulation of intestinal homeostasis. Inflamm. Bowel Dis. 17, 1359–1372. doi: 10.1002/ibd.21478
Hoffman, G. E., and Schadt, E. E. (2016). variancePartition: interpreting drivers of variation in complex gene expression studies. BMC Bioinformatics 17, 483. doi: 10.1186/s12859-016-1323-z
Howlett, D. R., Richardson, J. C., Austin, A., Parsons, A. A., Bate, S. T., Davies, D. C., et al. (2004). Cognitive correlates of Abeta deposition in male and female mice bearing amyloid precursor protein and presenilin-1 mutant transgenes. Brain Res. 1017, 130–136. doi: 10.1016/j.brainres.2004.05.029
Hui, K. S. (2007). Brain-specific aminopeptidase: from enkephalinase to protector against neurodegeneration. Neurochem. Res. 32, 2062–2071. doi: 10.1007/s11064-007-9356-3
Iorio, F., Bosotti, R., Scacheri, E., Belcastro, V., Mithbaokar, P., Ferriero, R., et al. (2010). Discovery of drug mode of action and drug repositioning from transcriptional responses. Proc. Natl. Acad. Sci. USA. 107, 14621–14626. doi: 10.1073/pnas.1000138107
Islam, M. R., Valaris, S., Young, M. F., Haley, E. B., Luo, R., Bond, S. F., et al. (2021). Exercise hormone irisin is a critical regulator of cognitive function. Nat. Metab. 3, 1058–1070. doi: 10.1038/s42255-021-00438-z
Jin, K., Peel, A. L., Mao, X. O., Xie, L., Cottrell, B. A., Henshall, D. C., et al. (2004). Increased hippocampal neurogenesis in Alzheimer's disease. Proc. Natl. Acad. Sci. USA. 101, 343–347. doi: 10.1073/pnas.2634794100
Kam, T. I., Park, H., Chou, S. C., Van Vranken, J. G., Mittenbuhler, M. J., Kim, H., et al. (2022). Amelioration of pathologic alpha-synuclein-induced Parkinson's disease by irisin. Proc. Natl. Acad. Sci. USA. 119, e2204835119. doi: 10.1073/pnas.2204835119
Karssemeijer, E. G. A., Aaronson, J. A., Bossers, W. J., Smits, T., Olde Rikkert, M. G. M., Kessels, R. P. C., et al. (2017). Positive effects of combined cognitive and physical exercise training on cognitive function in older adults with mild cognitive impairment or dementia: a meta-analysis. Ageing Res. Rev. 40, 75–83. doi: 10.1016/j.arr.2017.09.003
Karsten, S. L., Sang, T. K., Gehman, L. T., Chatterjee, S., Liu, J., Lawless, G. M., et al. (2006). A genomic screen for modifiers of tauopathy identifies puromycin-sensitive aminopeptidase as an inhibitor of tau-induced neurodegeneration. Neuron 51, 549–560. doi: 10.1016/j.neuron.2006.07.019
Keiser, M. J., Setola, V., Irwin, J. J., Laggner, C., Abbas, A. I., Hufeisen, S. J., et al. (2009). Predicting new molecular targets for known drugs. Nature 462, 175–181. doi: 10.1038/nature08506
Kim, D., Cho, J., and Kang, H. (2019). Protective effect of exercise training against the progression of Alzheimer's disease in 3xTg-AD mice. Behav. Brain Res. 374, 112105. doi: 10.1016/j.bbr.2019.112105
Kim, H., Wrann, C. D., Jedrychowski, M., Vidoni, S., Kitase, Y., Nagano, K., et al. (2018). Irisin mediates effects on bone and fat via alphaV integrin receptors. Cell 175, 1756–1768. e17. doi: 10.1016/j.cell.2018.10.025
Kim, S. H., Steele, J. W., Lee, S. W., Clemenson, G. D., Carter, T. A., Treuner, K., et al. (2014). Proneurogenic group II mGluR antagonist improves learning and reduces anxiety in Alzheimer Abeta oligomer mouse. Mol. Psychiatry 19, 1235–1242. doi: 10.1038/mp.2014.87
Koike, H., Iijima, M., and Chaki, S. (2011). Involvement of the mammalian target of rapamycin signaling in the antidepressant-like effect of group II metabotropic glutamate receptor antagonists. Neuropharmacology 61, 1419–1423. doi: 10.1016/j.neuropharm.2011.08.034
Krezymon, A., Richetin, K., Halley, H., Roybon, L., Lassalle, J. M., Frances, B., et al. (2013). Modifications of hippocampal circuits and early disruption of adult neurogenesis in the tg2576 mouse model of Alzheimer's disease. PLoS ONE 8, e76497. doi: 10.1371/journal.pone.0076497
Krishna, K. K., Baby, N., Raghuraman, R., Navakkode, S., Behnisch, T., Sajikumar, S., et al. (2020). Regulation of aberrant proteasome activity re-establishes plasticity and long-term memory in an animal model of Alzheimer's disease. FASEB J. 34, 9466–9479. doi: 10.1096/fj.201902844RR
Kruppa, A. J., Ott, S., Chandraratna, D. S., Irving, J. A., Page, R. M., Speretta, E., et al. (2013). Suppression of abeta toxicity by puromycin-sensitive aminopeptidase is independent of its proteolytic activity. Biochim. Biophys. Acta 1832, 2115–2126. doi: 10.1016/j.bbadis.2013.07.019
Kudo, L. C., Parfenova, L., Ren, G., Vi, N., Hui, M., Ma, Z., et al. (2011). Puromycin-sensitive aminopeptidase (PSA/NPEPPS) impedes development of neuropathology in hPSA/TAU(P301L) double-transgenic mice. Hum. Mol. Genet. 20, 1820–1833. doi: 10.1093/hmg/ddr065
Kuhn, H. G., Cooper-Kuhn, C. M., Boekhoorn, K., and Lucassen, P. J. (2007). Changes in neurogenesis in dementia and Alzheimer mouse models: are they functionally relevant? Eur. Arch. Psychiatry Clin. Neurosci. 257, 281–289. doi: 10.1007/s00406-007-0732-4
Kuleshov, M. V., Jones, M. R., Rouillard, A. D., Fernandez, N. F., Duan, Q., Wang, Z., et al. (2016). Enrichr: a comprehensive gene set enrichment analysis web server 2016 update. Nucleic Acids Res. 44, W90–W97. doi: 10.1093/nar/gkw377
Lajarin-Cuesta, R., Nanclares, C., Arranz-Tagarro, J. A., Gonzalez-Lafuente, L., Arribas, R. L., Araujo de Brito, M., et al. (2016). Gramine derivatives targeting Ca(2+) channels and Ser/Thr phosphatases: a new dual strategy for the treatment of neurodegenerative diseases. J. Med. Chem. 59, 6265–6280. doi: 10.1021/acs.jmedchem.6b00478
Lamb, J., Crawford, E. D., Peck, D., Modell, J. W., Blat, I. C., Wrobel, M. J., et al. (2006). The Connectivity Map: using gene-expression signatures to connect small molecules, genes, and disease. Science 313, 1929–1935. doi: 10.1126/science.1132939
Law, V., Knox, C., Djoumbou, Y., Jewison, T., Guo, A. C., Liu, Y., et al. (2014). DrugBank 4.0: shedding new light on drug metabolism. Nucleic Acids Res. 42, D1091–D1097. doi: 10.1093/nar/gkt1068
Lazarov, O., and Marr, R. A. (2010). Neurogenesis and Alzheimer's disease: at the crossroads. Exp. Neurol. 223, 267–281. doi: 10.1016/j.expneurol.2009.08.009
Leek, J. T., Johnson, W. E., Parker, H. S., Jaffe, A. E., and Storey, J. D. (2012). The sva package for removing batch effects and other unwanted variation in high-throughput experiments. Bioinformatics 28, 882–883. doi: 10.1093/bioinformatics/bts034
Liao, Y., Smyth, G. K., and Shi, W. (2014). featureCounts: an efficient general purpose program for assigning sequence reads to genomic features. Bioinformatics 30, 923–930. doi: 10.1093/bioinformatics/btt656
LiCausi, F., and Hartman, N. W. (2018). Role of mTOR complexes in neurogenesis. Int. J. Mol. Sci. 19, 1544. doi: 10.3390/ijms19051544
Liu, P. Z., and Nusslock, R. (2018). Exercise-mediated neurogenesis in the hippocampus via BDNF. Front. Neurosci. 12, 52. doi: 10.3389/fnins.2018.00052
Liu, T., Xia, Z., Zhang, W. W., Xu, J. R., Ge, X. X., Li, J., et al. (2013). Bis(9)-(-)-nor-meptazinol as a novel dual-binding AChEI potently ameliorates scopolamine-induced cognitive deficits in mice. Pharmacol. Biochem. Behav. 104, 138–143. doi: 10.1016/j.pbb.2012.11.009
Llorens-Martin, M. (2018). Exercising new neurons to vanquish Alzheimer disease. Brain Plast. 4, 111–126. doi: 10.3233/BPL-180065
Lourenco, M. V., Frozza, R. L., De Freitas, G. B., Zhang, H., Kincheski, G. C., Ribeiro, F. C., et al. (2019). Exercise-linked FNDC5/irisin rescues synaptic plasticity and memory defects in Alzheimer's models. Nat. Med. 25, 165–175. doi: 10.1038/s41591-018-0275-4
Love, M. I., Huber, W., and Anders, S. (2014). Moderated estimation of fold change and dispersion for RNA-seq data with DESeq2. Genome Biol. 15, 550. doi: 10.1186/s13059-014-0550-8
Lupton, M. K., Proitsi, P., Lin, K., Hamilton, G., Daniilidou, M., Tsolaki, M., et al. (2014). The role of ABCA1 gene sequence variants on risk of Alzheimer's disease. J. Alzheimers. Dis. 38, 897–906. doi: 10.3233/JAD-131121
Ma, C. L., Ma, X. T., Wang, J. J., Liu, H., Chen, Y. F., Yang, Y., et al. (2017). Physical exercise induces hippocampal neurogenesis and prevents cognitive decline. Behav. Brain Res. 317, 332–339. doi: 10.1016/j.bbr.2016.09.067
McDaniel, S. S., Rensing, N. R., Thio, L. L., Yamada, K. A., and Wong, M. (2011). The ketogenic diet inhibits the mammalian target of rapamycin (mTOR) pathway. Epilepsia 52, e7–e11. doi: 10.1111/j.1528-1167.2011.02981.x
Meng, Q., Lin, M. S., and Tzeng, I. S. (2020). Relationship between exercise and Alzheimer's disease: a narrative literature review. Front. Neurosci. 14, 131. doi: 10.3389/fnins.2020.00131
Mobley, A. K., Tchaicha, J. H., Shin, J., Hossain, M. G., and Mccarty, J. H. (2009). Beta8 integrin regulates neurogenesis and neurovascular homeostasis in the adult brain. J. Cell Sci. 122, 1842–1851. doi: 10.1242/jcs.043257
Morgan, D. (2006). “Cognitive impairment in transgenic mouse models of amyloid deposition,” in Animal Models of Cognitive Impairment, eds E. D. Levin, and J. J. Buccafusco (Boca Raton, FL: CRC Press), 183–198. doi: 10.1201/9781420004335.sec3
Mortimer, J. A., and Stern, Y. (2019). Physical exercise and activity may be important in reducing dementia risk at any age. Neurology 92, 362–363. doi: 10.1212/WNL.0000000000006935
Ngandu, T., Lehtisalo, J., Solomon, A., Levalahti, E., Ahtiluoto, S., Antikainen, R., et al. (2015). A 2 year multidomain intervention of diet, exercise, cognitive training, and vascular risk monitoring versus control to prevent cognitive decline in at-risk elderly people (FINGER): a randomised controlled trial. Lancet 385, 2255–2263. doi: 10.1016/S0140-6736(15)60461-5
Niidome, T., Taniuchi, N., Akaike, A., Kihara, T., and Sugimoto, H. (2008). Differential regulation of neurogenesis in two neurogenic regions of APPswe/PS1dE9 transgenic mice. Neuroreport 19, 1361–1364. doi: 10.1097/WNR.0b013e32830e6dd6
Obernier, K., and Alvarez-Buylla, A. (2019). Neural stem cells: origin, heterogeneity and regulation in the adult mammalian brain. Development 146, dev156059. doi: 10.1242/dev.156059
Pasini, D., Bracken, A. P., Hansen, J. B., Capillo, M., and Helin, K. (2007). The polycomb group protein Suz12 is required for embryonic stem cell differentiation. Mol. Cell. Biol. 27, 3769–3779. doi: 10.1128/MCB.01432-06
Pedersen, B. K. (2019). Physical activity and muscle-brain crosstalk. Nat. Rev. Endocrinol. 15, 383–392. doi: 10.1038/s41574-019-0174-x
Perez-Garcia, G., Bicak, M., Haure-Mirande, J. V., Perez, G. M., Otero-Pagan, A., Gama Sosa, M. A., et al. (2023). BCI-838, an orally active mGluR2/3 receptor antagonist pro-drug, rescues learning behavior deficits in the PS19 MAPT(P301S) mouse model of tauopathy. Neurosci. Lett. 797, 137080. doi: 10.1016/j.neulet.2023.137080
Perez-Garcia, G., De Gasperi, R., Gama Sosa, M. A., Perez, G. M., Otero-Pagan, A., and Tschiffely, S. (2018). PTSD-related behavioral traits in a rat model of blast-induced mTBI are reversed by the mGluR2/3 receptor antagonist BCI-838. eNeuro 5. doi: 10.1523/ENEURO.0357-17.2018
Perez-Garcia, G., Guzman-Quevedo, O., Da Silva Aragao, R., and Bolanos-Jimenez, F. (2016). Early malnutrition results in long-lasting impairments in pattern-separation for overlapping novel object and novel location memories and reduced hippocampal neurogenesis. Sci. Rep. 6, 21275. doi: 10.1038/srep21275
Posimo, J. M., Weilnau, J. N., Gleixner, A. M., Broeren, M. T., Weiland, N. L., Brodsky, J. L., et al. (2015). Heat shock protein defenses in the neocortex and allocortex of the telencephalon. Neurobiol. Aging 36, 1924–1937. doi: 10.1016/j.neurobiolaging.2015.02.011
Querfurth, H., and Lee, H. K. (2021). Mammalian/mechanistic target of rapamycin (mTOR) complexes in neurodegeneration. Mol. Neurodegener. 16, 44. doi: 10.1186/s13024-021-00428-5
Rabiee, F., Lachinani, L., Ghaedi, S., Nasr-Esfahani, M. H., Megraw, T. L., Ghaedi, K., et al. (2020). New insights into the cellular activities of Fndc5/Irisin and its signaling pathways. Cell Biosci. 10, 51. doi: 10.1186/s13578-020-00413-3
Ramirez-Amaya, V., Marrone, D. F., Gage, F. H., Worley, P. F., and Barnes, C. A. (2006). Integration of New Neurons into Functional Neural Networks. J Neurosci. 26, 12237–12241. doi: 10.1523/JNEUROSCI.2195-06.2006
Readhead, B., Hartley, B. J., Eastwood, B. J., Collier, D. A., Evans, D., Farias, R., et al. (2018). Expression-based drug screening of neural progenitor cells from individuals with schizophrenia. Nat. Commun. 9, 4412. doi: 10.1038/s41467-018-06515-4
Readhead, B., Haure-Mirande, J. V., Zhang, B., Haroutunian, V., Gandy, S., Schadt, E. E., et al. (2016). Molecular systems evaluation of oligomerogenic APP(E693Q) and fibrillogenic APP(KM670/671NL)/PSEN1(Deltaexon9) mouse models identifies shared features with human Alzheimer's brain molecular pathology. Mol. Psychiatry 21, 1153–1154. doi: 10.1038/mp.2015.215
Sengupta, S., Horowitz, P. M., Karsten, S. L., Jackson, G. R., Geschwind, D. H., Fu, Y., et al. (2006). Degradation of tau protein by puromycin-sensitive aminopeptidase in vitro. Biochemistry 45, 15111–15119. doi: 10.1021/bi061830d
Shao, B. Y., Xia, Z., Xie, Q., Ge, X. X., Zhang, W. W., Sun, J., et al. (2014). Meserine, a novel carbamate AChE inhibitor, ameliorates scopolamine-induced dementia and alleviates amyloidogenesis of APP/PS1 transgenic mice. CNS Neurosci. Ther. 20, 165–171. doi: 10.1111/cns.12183
Shi, Y., Huang, W., Wang, Y., Zhang, R., Hou, L., Xu, J., et al. (2018). Bis(9)-(-)-Meptazinol, a novel dual-binding AChE inhibitor, rescues cognitive deficits and pathological changes in APP/PS1 transgenic mice. Transl. Neurodegener. 7, 21. doi: 10.1186/s40035-018-0126-8
Swiech, L., Perycz, M., Malik, A., and Jaworski, J. (2008). Role of mTOR in physiology and pathology of the nervous system. Biochim. Biophys. Acta 1784, 116–132. doi: 10.1016/j.bbapap.2007.08.015
Taniuchi, N., Niidome, T., Goto, Y., Akaike, A., Kihara, T., Sugimoto, H., et al. (2007). Decreased proliferation of hippocampal progenitor cells in APPswe/PS1dE9 transgenic mice. Neuroreport 18, 1801–1805. doi: 10.1097/WNR.0b013e3282f1c9e9
Tapia-Rojas, C., Aranguiz, F., Varela-Nallar, L., and Inestrosa, N. C. (2016). Voluntary running attenuates memory loss, decreases neuropathological changes and induces neurogenesis in a mouse model of Alzheimer's disease. Brain Pathol. 26, 62–74. doi: 10.1111/bpa.12255
Terreros-Roncal, J., Moreno-Jimenez, E. P., Flor-Garcia, M., Rodriguez-Moreno, C. B., Trinchero, M. F., Cafini, F., et al. (2021). Impact of neurodegenerative diseases on human adult hippocampal neurogenesis. Science 374, 1106–1113. doi: 10.1126/science.abl5163
Toda, T., Parylak, S. L., Linker, S. B., and Gage, F. H. (2019). The role of adult hippocampal neurogenesis in brain health and disease. Mol. Psychiatry 24, 67–87. doi: 10.1038/s41380-018-0036-2
Tzatsos, A., and Kandror, K. V. (2006). Nutrients suppress phosphatidylinositol 3-kinase/Akt signaling via raptor-dependent mTOR-mediated insulin receptor substrate 1 phosphorylation. Mol. Cell. Biol. 26, 63–76. doi: 10.1128/MCB.26.1.63-76.2006
Unger, M. S., Marschallinger, J., Kaindl, J., Hofling, C., Rossner, S., Heneka, M. T., et al. (2016). Early changes in hippocampal neurogenesis in transgenic mouse models for Alzheimer's disease. Mol. Neurobiol. 53, 5796–5806. doi: 10.1007/s12035-016-0018-9
Van Praag, H., Kempermann, G., and Gage, F. H. (1999). Running increases cell proliferation and neurogenesis in the adult mouse dentate gyrus. Nat. Neurosci. 2, 266–270. doi: 10.1038/6368
Van Praag, H., Shubert, T., Zhao, C., and Gage, F. H. (2005). Exercise enhances learning and hippocampal neurogenesis in aged mice list. J. Neurosci. 25, 8680–8685. doi: 10.1523/JNEUROSCI.1731-05.2005
Verret, L., Jankowsky, J. L., Xu, G. M., Borchelt, D. R., and Rampon, C. (2007). Alzheimer's-type amyloidosis in transgenic mice impairs survival of newborn neurons derived from adult hippocampal neurogenesis. J. Neurosci. 27, 6771–6780. doi: 10.1523/JNEUROSCI.5564-06.2007
Wang, J., Tanila, H., Puolivali, J., Kadish, I., and Van Groen, T. (2003). Gender differences in the amount and deposition of amyloidbeta in APPswe and PS1 double transgenic mice. Neurobiol. Dis. 14, 318–327. doi: 10.1016/j.nbd.2003.08.009
Wang, Y., Yang, Y., Hong, K. H., Ning, Y., Yu, P., Ren, J., et al. (2019). Design, synthesis and evaluation of a novel metal chelator as multifunctional agents for the treatment of Alzheimer's disease. Bioorg. Chem. 87, 720–727. doi: 10.1016/j.bioorg.2019.03.064
Wen, P. H., Hof, P. R., Chen, X., Gluck, K., Austin, G., Younkin, S. G., et al. (2004). The presenilin-1 familial Alzheimer disease mutant P117L impairs neurogenesis in the hippocampus of adult mice. Exp. Neurol. 188, 224–237. doi: 10.1016/j.expneurol.2004.04.002
Wimo, A., Handels, R., Antikainen, R., Eriksdotter, M., Jonsson, L., Knapp, M., et al. (2022). Dementia prevention: the potential long-term cost-effectiveness of the FINGER prevention program. Alzheimers Dement. doi: 10.1002/alz.13044
Wozniak, K. M., Rojas, C., Wu, Y., and Slusher, B. S. (2012). The role of glutamate signaling in pain processes and its regulation by GCP II inhibition. Curr. Med. Chem. 19, 1323–1334. doi: 10.2174/092986712799462630
Yu, H., Zhang, C., Xia, J., and Xu, B. (2021). Treadmill exercise ameliorates adult hippocampal neurogenesis possibly by adjusting the APP proteolytic pathway in APP/PS1 transgenic mice. Int. J. Mol. Sci. 22, 9570. doi: 10.3390/ijms22179570
Yu, Y., He, J., Zhang, Y., Luo, H., Zhu, S., Yang, Y., et al. (2009). Increased hippocampal neurogenesis in the progressive stage of Alzheimer's disease phenotype in an APP/PS1 double transgenic mouse model. Hippocampus 19, 1247–1253. doi: 10.1002/hipo.20587
Zhang, L., Pilarowski, G., Pich, E. M., Nakatani, A., Dunlop, J., Baba, R., et al. (2021). Inhibition of KDM1A activity restores adult neurogenesis and improves hippocampal memory in a mouse model of Kabuki syndrome. Mol. Ther. Methods Clin. Dev. 20, 779–791. doi: 10.1016/j.omtm.2021.02.011
Zhang, S. S., Zhu, L., Peng, Y., Zhang, L., Chao, F. L., Jiang, L., et al. (2022). Long-term running exercise improves cognitive function and promotes microglial glucose metabolism and morphological plasticity in the hippocampus of APP/PS1 mice. J. Neuroinflammation 19, 34. doi: 10.1186/s12974-022-02401-5
Keywords: APP/PS1 mice, physical exercise, mGlu2/3 antagonist, neurogenesis, BDNF, AD amyloid pathology
Citation: Perez Garcia G, Bicak M, Buros J, Haure-Mirande J-V, Perez GM, Otero-Pagan A, Gama Sosa MA, De Gasperi R, Sano M, Gage FH, Barlow C, Dudley JT, Glicksberg BS, Wang Y, Readhead B, Ehrlich ME, Elder GA and Gandy S (2023) Beneficial effects of physical exercise and an orally active mGluR2/3 antagonist pro-drug on neurogenesis and behavior in an Alzheimer's amyloidosis model. Front. Dement. 2:1198006. doi: 10.3389/frdem.2023.1198006
Received: 31 March 2023; Accepted: 31 July 2023;
Published: 06 September 2023.
Edited by:
Adrian Preda, University of California, Irvine, United StatesReviewed by:
Federico Emanuele Pozzi, IRCCS Fondazione San Gerardo, ItalyCopyright © 2023 Perez Garcia, Bicak, Buros, Haure-Mirande, Perez, Otero-Pagan, Gama Sosa, De Gasperi, Sano, Gage, Barlow, Dudley, Glicksberg, Wang, Readhead, Ehrlich, Elder and Gandy. This is an open-access article distributed under the terms of the Creative Commons Attribution License (CC BY). The use, distribution or reproduction in other forums is permitted, provided the original author(s) and the copyright owner(s) are credited and that the original publication in this journal is cited, in accordance with accepted academic practice. No use, distribution or reproduction is permitted which does not comply with these terms.
*Correspondence: Sam Gandy, c2FtdWVsLmdhbmR5QHZhLmdvdg==; c2FtdWVsLmdhbmR5QG1zc20uZWR1
†Present addresses: Jacqueline Buros, Generable, Inc., New York, NY, United States
Joel T. Dudley, Innovation Endeavors, Louisville, CO, United States
‡These authors share first authorship
Disclaimer: All claims expressed in this article are solely those of the authors and do not necessarily represent those of their affiliated organizations, or those of the publisher, the editors and the reviewers. Any product that may be evaluated in this article or claim that may be made by its manufacturer is not guaranteed or endorsed by the publisher.
Research integrity at Frontiers
Learn more about the work of our research integrity team to safeguard the quality of each article we publish.