- 1The Johns Hopkins University School of Medicine, Department of Molecular and Comparative Pathobiology, Baltimore, MD, United States
- 2Agencia de Regulación y Control de la Bioseguridad y Cuarentena para Galápagos (Agency for the Regulation and Control of Biosecurity and Quarantine for Galápagos; ABG), Puerto Ayora, Santa Cruz Island, Galápagos, Ecuador
- 3Independent Researcher, Quito, Ecuador
- 4Section of Zoological Medicine, Department of Clinical Sciences, Cornell University College of Veterinary Medicine, Ithaca, NY, United States
The Galápagos archipelago is a vast reservoir of terrestrial and marine biodiversity, owing in large part to its relatively recent volcanic genesis and colonization by humans. This unique ecological system is particularly susceptible to human, animal, and environmental impacts. Climate change, globalization, and the blurring of human-domestic animal-wildlife interfaces are poised to bring new threats and challenges to the region. A One Health perspective that simultaneously considers human, animal, and environmental health is imperative in assessing and mitigating the challenges facing the Galápagos Islands. In Part I of this review, we provide the historical context for biodiversity in the archipelago; discuss the role of invasive species in habitat destruction, fragmentation, and competition with endemic species; and summarize the established and emerging infectious disease threats. We also discuss the imperative to implement research, surveillance, and preventative measures to identify and manage future threats from a One Health perspective, with a specific emphasis on implications for wildlife health. In Part II of this review, we outline the socioeconomic context of life in the Galápagos Islands, evaluate the current and predicted effects of climate change, and discuss direct anthropogenic factors affecting Galápagos biodiversity, such as tourism, fishing, pollution, and the illegal wildlife trade. We also examine the impact of the COVID-19 pandemic in the region. We build a cohesive picture of One Health in the Galápagos Islands by integrating past work, current needs, and emerging threats. We also consider overarching goals for conservation, ecosystem management, and socioeconomic sustainability that have been previously defined by both governmental and non-governmental stakeholders, and we identify discrete, implementable, and interdisciplinary recommendations that will facilitate achievement of those goals.
1 Introduction
1.1 Historical context of Galápagos biodiversity
Located 960 kilometers west of Ecuador, the Galápagos Islands harbor unique terrestrial and marine biodiversity, comprising over 6,000 species, with 1,870 being endemic (CDF, 2023b). “Endemic” species are those limited to a small geographic region: in layman’s terms, “found nowhere else.” The Galápagos Islands have one of the highest rates of endemism in the world, including 22% of birds, 72% of reptiles, 38% of terrestrial mammals, 7% of marine mammals, 12% of fish (CDF, 2023b), 37% of vascular plants (Tye and Francisco-Ortega, 2011), 47% of insects (Parent et al., 2008), 29% of shallow-water corals (Hickman, 2009), and 20% of mollusks (Finet, 1994). We have summarized the conservation status of Galápagos vertebrates in Table 1.
The archipelago’s relatively recent volcanic genesis (Tye et al., 2002), has influenced the composition of its flora and fauna, whose ancestors arrived by flying, swimming, or floating. Isolated habitats and diverse ecosystems also provided selective pressures for species diversification. The striking morphological differences between species of mockingbirds in the Galápagos Islands, for example, are thought to have inspired Charles Darwin’s theory of evolution (Arbogast et al., 2006). The Galápagos Islands are also unique, even among oceanic islands, in that there has been relatively little time for humans to impart change. The archipelago was first discovered in 1535, but apart from serving as an occasional mooring for pirates and whalers, was not colonized until 1832 (Smith, 1979). As a result of this relatively recent history of human settlement, the archipelago can be considered “a rare remnant of a prehistorical pattern of global biological diversity where great proportions of the world’s distinctive and often bizarre species occurred on islands,” as wrote Charles Darwin Foundation (CDF) researchers in 2002. “Man has destroyed much of that pattern. The biological diversity of the Galápagos is one of the best examples of that pattern because it remains,” (Tye et al., 2002). The value in preserving the archipelago’s unique endemic species, therefore, cannot be understated.
Nonetheless, human exploitation of endemic wildlife and extractive use of natural resources have long been a part of the archipelago’s history. Whalers touted the Galápagos giant tortoise as a ready source of fresh meat and hydration (Nicholls, 2021; Conrad & Gibbs, 2021), killing at least 100,000 Galápagos giant tortoises between 1800-1870 (Townsend, 1925; Conrad & Gibbs, 2021). These population decimations led to the extinction of tortoises on Santa Fe and Floreana Islands (Conrad & Gibbs, 2021; MacFarland et al., 1974) and enormous pressures on tortoises on multiple other islands (Conrad & Gibbs, 2021), from which modern populations have never fully recovered. Mariners introduced goats, pigs, and donkeys intentionally, while black rats and house mice arrived as stowaways on ships. In 1832, Ecuador claimed the Galápagos Islands from Spain and a prison colony was established on Floreana Island (Smith, 1979). Between 1860 and 1930, further colonies were established for sugarcane farming, coffee crops, and salt mining. By that time, horses, cattle, dogs, and cats had been introduced; these invasive species destroyed vegetation, predated nests and hatchlings, and competed with endemic species for resources (MacFarland et al., 1974). remaining a principal threat today.
In considering the historical narrative of the Galápagos Islands, the long and complicated relationship with foreign scientists cannot be ignored. In 1835, Charles Darwin documented biodiversity in the region through 1,500 biologic specimens. For the next century, foreign researchers were fascinated by the novel flora and fauna and even began to recognize threats imposed by whalers and invasive species (Dumbacher and West, 2010). However, their solution was preservation of endemic species not in their natural habitat, but in the form of museum specimens. Between 1897 and 1905, for instance, American scientist Rollo Beck collected tens of thousands of insects, birds, plants, and reptiles (Gifford, 1908; Dumbacher and West, 2010). G. T. Corley Smith of the CDF wrote in 1979: “Conservation was a concept virtually unknown to their generation. Scientists simply accepted that the Galápagos fauna was doomed to extinction and that their duty to posterity was to preserve as much as they could in museums.” Despite the research value of these specimens (Dumbacher and West, 2010; Tonnis et al., 2005), their method of acquisition – collecting nesting individuals and eggs, or in some cases, capture of every single individual seen – doubtlessly contributed to population declines still faced by these species today. The Galápagos National Park Directorate (GNPD) now requires scientists to acquire research permits prior to collection, with stringent review of scientific justification and research methodology. These reviews ensure both that the type and number of specimens collected will not negatively impact endemic populations or ecosystems, and that their use is likely to result in meaningful research outcomes.
The Galápagos Islands are still faced with the challenge of “helicopter research,” in which foreign scientists with considerable resources enter developing regions, collect samples, publish data without inclusion of local scientists, and then leave (Adame, 2021; Minasny et al., 2020; Chin et al., 2019). Too often, research methods and results are not applicable or reproducible for local stakeholders, hindering long-term benefits. Conversely, there still exist barriers to dissemination of results of locally performed research. For instance, Barnett and Rudd first documented canine heartworm (Dirofilaria immitis) microfilariae in Galápagos sea lions (Zalophus wollebaeki) on Floreana Island in the 1980s (Barnett and Rudd, 1983; Barnett, 1985a). This publication, written in Spanish and held in print at the Charles Darwin Research Station, is largely inaccessible to the larger scientific community, and thus, a 2023 publication claims to be the first report of D. immitis infection in Galápagos sea lions (Gregory et al., 2023). Mitigating such barriers to information-sharing and maximizing the local impacts of research is essential, as is the integration of input from various sectors and stakeholders in human, animal, and environmental health. Otherwise, we risk continuing to perform research in silos, which can result in duplication of research efforts and limitation of the downstream benefits of research results.
1.2 Recognition of threats to Galápagos ecosystems
The Galápagos National Park (GNP) was established in 1959, comprising 97% of the archipelago land mass; the remaining 3% was designated as human-inhabited areas across Santa Cruz, San Cristóbal, Floreana, and Isabela Islands. The role of the GNP is to protect sensitive wildlife and ecological resources in the archipelago and to invest in conservation research. Under the 1998 Special Law for Galápagos, enacted by the Ecuadorian government, 133,000 square-kilometers of marine, coastal, and inland waters were incorporated into the Galápagos Marine Reserve (GMR). These protected areas were intended to serve as refuges for native species and offset habitat degradation.
In 1978, UNESCO recognized the vast biodiversity and ecological importance of the Galápagos Islands by declaring the archipelago a UNESCO World Heritage site, with the surrounding waters added to this designation in 2001. UNESCO participates in the conservation of World Heritage sites through conservation funding, emergency aid, and training and development, as well as through periodic assessments of data submitted by State Parties and development of conservation recommendations, as discussed in Section 3.1.
Prompted by ongoing and emerging threats to biodiversity, in 1999, the CDF and the World Wildlife Fund (WWF) conducted a joint biological analysis of the region. The resulting Biodiversity Vision for Galápagos summarized the ecological status of the islands, identified species most threatened by anthropogenic factors, and outlined a vision for conservation – including recommendations to mitigate biodiversity threats, and benchmarks by which to measure progress (Bensted-Smith, 2002). At that time, the archipelago retained 95% of its terrestrial biodiversity as compared to pre-human colonization. However, pressures from immigration, tourism, and industrial fishing were mounting, alongside the prospect of ecological degradation and consequent decline in biodiversity. Pressures were summarized by Snell et al. (2002) as reproduced in Table 2. Biologists feared that Galápagos flora and fauna might be at a precipice, and that failing to intervene could be disastrous, so much so that the workshop goals were summarized as: “Back to Eden – one last chance.”
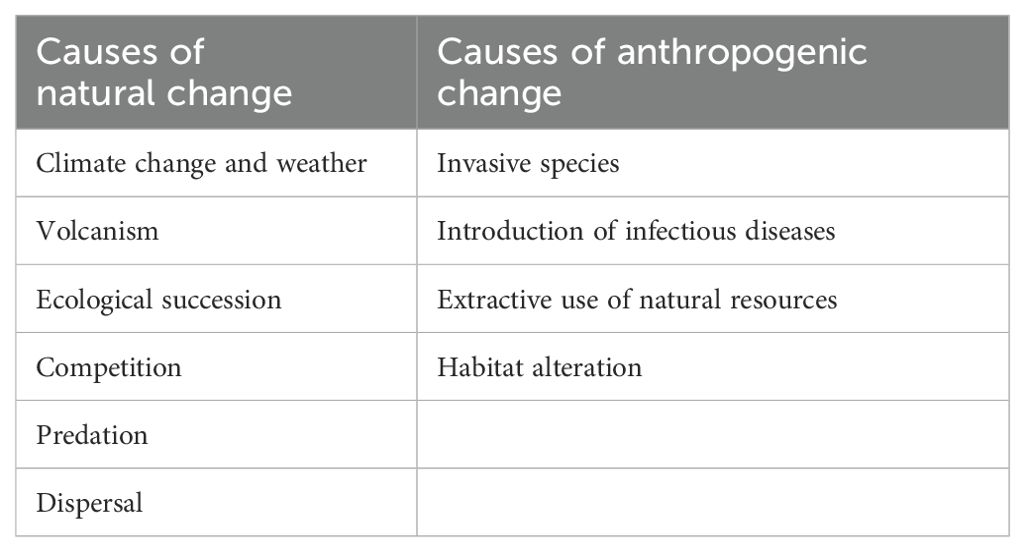
Table 2. Historical causes of natural and anthropogenic changes in the Galápagos Islands (Snell et al., 2002).
1.3 Impetus for the current review
Over the past 25 years, the Galápagos Islands has faced rising tourism, immigration, introduction of invasive species, and overfishing, as well as insufficient resources for local agencies to effectively manage these issues, leading to lack of implementation of quarantine, inspection, and biosecurity regulations (UNESCO, 2006). The GNPD has also documented tremendous pressures on ecosystem services and increasing demand for resources, making current conditions unsustainable (DPNG, 2014). Factors currently affecting ecosystem services include changes in land use, invasive species, loss of biodiversity, habitat fragmentation and degradation, overfishing, and stone materials overexploitation (DNPG, 2014; UNESCO, 2006). Water resource degradation and pollution are compounding factors, alongside the demand for infrastructure for fishing, tourism, transportation, and maritime activities (DPNG, 2014). These concerns led to the inclusion of the Galápagos Islands on the UNESCO List of World Heritage in Danger from 2007-2010 (UNESCO, 2006, 2007).
In October 2012, the Agency for the Regulation and Control of Biosecurity and Quarantine for Galápagos (ABG) was established to prevent phyto/zoo-sanitary threats and control and reduce the risk of invasive species. ABG oversees prevention and early detection of threats through various surveillance mechanisms, and where necessary, develops control and eradication strategies. Previously, these responsibilities were distributed among various national and regional institutions (Bensted-Smith et al., 2002); centralizing responsibilities under ABG improved the efficiency of management, surveillance, and research. However, optimal implementation and maintenance of these systems is still hindered by periodic lapses in funding, personnel, and equipment (Rogg et al., 2005).
Only one extinction has been documented in the Galápagos Islands in the past 30 years: the Pinta Island tortoise (Chelonoidis niger abingdonii), of which the famous final member, Lonesome George, passed away in 2012. The most recent prior extinctions were the San Cristóbal Vermilion Flycatcher (Pyrocephalus dubius), last sighted in 1987, and the Floreana Island tortoise (C. n. niger), hunted to extinction around 1850. In total, 16 formerly endemic Galápagos species are extinct (Table 3), five of which are known only from the fossil record (Steadman et al., 1991; Jiménez-Uzcátegui et al., 2007). Several species previously considered extinct are no longer recognized as such, and these were not included in the table. For example, recent genetic evidence suggests that there remain extant individuals of the Fernandina giant tortoise, Chelonoidis niger phantasticus (formerly Geochelone phantastica) (Jensen et al., 2022), and this species is currently considered Critically Endangered by the IUCN. Older literature references the Rábida giant tortoise (Chelonoidis niger wallacei); however, this description originated from a single specimen and is suspected to have been a stray individual from another island (MacFarland et al., 1974) and is thus no longer considered a subspecies (Frazier, 2021). Finally, we did not include species that are locally extinct on some of the islands but are still present within the archipelago, such as the Galápagos hawk (Buteo galapagoensis), unless official island-specific subspecies have been designated. For instance, we included Aegialomys galapagoensis galapagoensis, the extinct subspecies of rice rat once endemic to San Cristóbal Island, although Aegialomys galapagoensis bauri is still present on Santa Fé Island.
Despite this seemingly small number of species extinctions, population dynamics of many endemic Galápagos species have changed profoundly over the past decades. Declines in multiple finch species on Floreana Island have been documented since 1979 (Grant et al., 2005; O’Connor et al., 2010; Dvorak et al., 2017, 2011). Per a 2015-16 survey of endemic birds on Floreana Island, the Galápagos rail (Laterallus spilonota), vegetarian finch (Platyspiza crassirostris), and gray warbler finch (Certhidea fusca) were extirpated (locally extinct), and the Galápagos dove (Zenaida galapagoensis) was at risk (Dvorak et al., 2017). Populations of the endangered Galápagos sea lion have declined approximately 50% in the past 30 years (Riofrío-Lazo et al., 2017) due to human activities (Denkinger et al., 2014, 2015). Taken together, these changes suggest that endemic flora and fauna are struggling to contend with pressures of climate change, invasive species, and resource limitation, and that we can expect to see further shifts in the relative composition of endemic species. It is therefore necessary to reassess the status of previously identified threats facing the region, and to highlight the emergence of new pressures.
Both the International Union for Conservation of Nature (IUCN) and the CDF monitor the population status of native and endemic species in the Galápagos archipelago. Overall, CDF recognizes 82 species as Critically Endangered (CR), 63 as Endangered (EN), 193 as Vulnerable (VU), and 87 as Near-Threatened (NT), while the IUCN Red List assesses only 61 Galápagos species as CR, 32 as EN, 95 as VU, and 25 as NT (IUCN, 2022). The differences these classifications will be discussed in section 3.1. The at-risk Galápagos vertebrate species and their IUCN Red List statuses from 1994-2020 are summarized in Figure 1; the species included are listed in the Supplementary Tables.
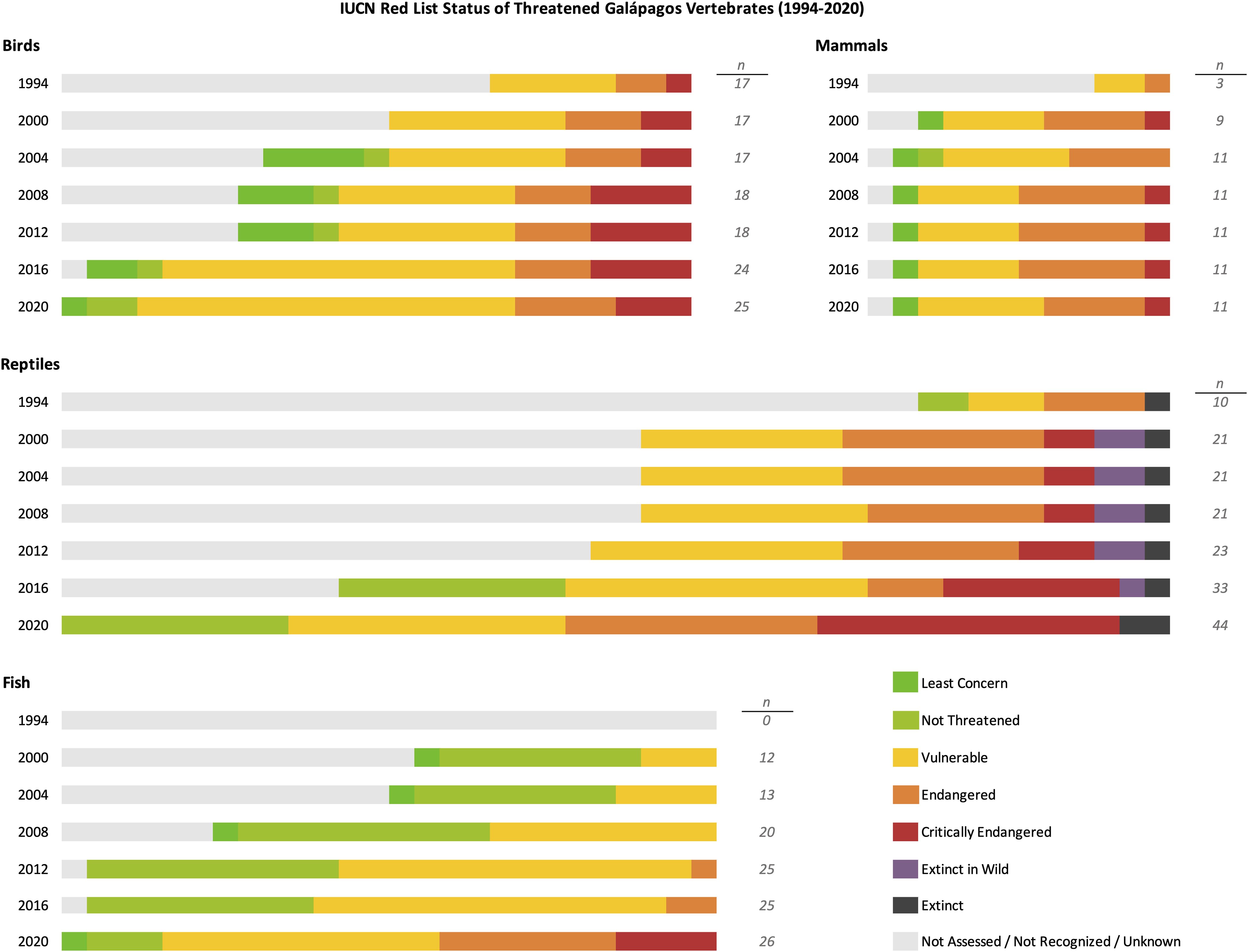
Figure 1. IUCN Red List status of at-risk vertebrate species of the Galápagos Islands, 1994-2020. Dark green indicates Least Concern status; light green indicates Not Threatened status; yellow indicates Vulnerable status; orange indicates Endangered status; red indicates Critically Endangered status; purple indicates species that are Extinct in the Wild; black indicates species that are Extinct; and light gray indicates species that were not assessed, not recognized as species, or with unknown status.
The essentiality of the link between human, domestic animal, wildlife, and ecosystem health must be the lens through which we approach biodiversity preservation in the Galápagos Islands. As defined by the One Health High-Level Expert Panel, One Health was conceptualized as a mindset that “recognizes the health of humans, domestic and wild animals, plants, and the wider environment (including ecosystems) are closely linked and interdependent” (OHHLEP et al., 2022). However, in practice, One Health has often been approached through an anthropocentric lens, aiming to improve animal health to mitigate the effects of poor animal health on human populations, such as emergence of zoonotic diseases (Fauci, 2006; Machalaba et al., 2015) or compromised food security (Kappes et al., 2023; Espinosa et al., 2020). Such an application of One Health leaves out key components: the impacts of anthropogenic activities on ecological stability and downstream effects, and the importance of safeguarding animal and environmental health (Stephen et al., 2023).
“Planetary Health” is an interdisciplinary field, founded by The Rockefeller Foundation-Lancet Commission, that focuses on how human activities can compromise environmental health and how these effects are then reflected in public health consequences (Whitmee et al., 2015; Planetary Health Alliance, 2021). Only by understanding and measuring these impacts can we develop appropriate policy approaches to challenges in public health and environmental management. Addressing interactions across these sectors, such as infectious disease, food and water sustainability, clean air and energy, and climate change, requires a sustainable balance between human, animal, and ecosystem health (Whitmee et al., 2015). To achieve this balance, integration of input from stakeholders, including policymakers and scientists, across multiple sectors is imperative (Whitmee et al., 2015). We find the concepts of One Health and Planetary Health key to the discussion of pressures facing the Galápagos Islands and to the development of management strategies that maximally benefit humans, animals, and the environment. The timeliness of this conversation could not be more apt in the wake of both the COVID-19 pandemic (Tounta et al., 2022) and the emergence of Highly Pathogenic Avian Influenza (HPAI) strain H5N1.
Here, we discuss the complex historical and socioeconomic contexts surrounding Galápagos biodiversity, revisit the pressures previously identified by Snell et al. (2002), and describe threats to biodiversity that have emerged over the past twenty-five years. Despite the large amount of work that has been accomplished in Galápagos conservation, we hold that there remains an imperative for further change which, to maximize impact, necessitates a One Health perspective.
2 Threats to Galápagos biodiversity
Part I of this review describes the impacts of introduced plants, invertebrates, and vertebrates on native Galápagos species, and reviews established, emerging, and future infectious disease threats. Part II of this review will address climate change, ocean acidification, and direct human activities such as tourism, fishing, pollution, agriculture, and human-wildlife conflict.
2.1 Introduced and invasive species
Introduced species are non-native species that have been introduced, often by anthropogenic events, to a given environment (National Invasive Species Information Center, 1999; Iannone et al., 2020). Invasive species are those introduced species that have or may have detrimental effects on human, animal, or environmental health (National Invasive Species Information Center, 1999; Iannone et al., 2020). Invasive species often spread rapidly beyond the area of introduction (Guo et al., 2024). An introduced species can subsequently become naturalized (integrated into the ecosystem and capable of maintaining their populations) (Guo et al., 2024). Invasive species have major consequences for endemic flora and fauna. Invasive plants can alter soil composition, water and light availability, nutrient cycling, and pollinator populations, depleting resources and impacting native plants, as well as affecting animals that rely on the ecosystem for food and shelter. Invasive animals can alter food chain and ecosystem dynamics, as well as participate in habitat degradation. Plant and animal extinctions exceed continental extinctions worldwide (Sax and Gaines, 2008), suggesting that islands are particularly susceptible to these impacts. In the Galápagos Islands, invasive species including mammals, invertebrates, and plants have resulted in tens of millions of dollars of economic losses (Ballesteros-Mejia et al., 2021).
In general, the importation of non-native plants and animals to the Galápagos Islands has been prohibited since 1999 (ABG, 2013; Toral-Granda et al., 2017; Zapata, 2008), and surveillance measures at ports of entry aim to identify and prevent both accidental and purposeful introductions, whether via passenger luggage or cargo shipping. Case-by-case exemptions to this rule are granted by the board of ABG; for example, in 2014, trained dogs were imported to detect invasive giant African snails (Lissachatina fulica), which destroy crops and native vegetation and threaten critically endangered endemic Galápagos land snails (Bulimulus ochsneri) (Galápagos Conservancy Annual Report, 2014). Similarly, until recent restrictions associated with HPAI surveillance, unvaccinated chicks could be imported to supply the local poultry industry (Puente-Rodriguez et al., 2019). However, despite these restrictions, introduced species still represent a major pressure on Galápagos ecosystems due to ongoing presence of historically-introduced species (such as now-feral domestic animals and plants no longer confined to agriculture) and the risk of new introductions of species that evade detection. Between 1990 and 2007, the documented introduced species in the archipelago increased ten-fold (Watkins and Cruz, 2007). As of 2017, 1,579 species were estimated to have been introduced to the Galápagos Islands, with 93% having become naturalized (Toral-Granda et al., 2017). Pizzitutti et al. (2016) reported that in 2012, 20% of species were introduced, and predicted that by 2033, under a model of continued high rates of growth, that proportion could rise to 50% (Pizzitutti et al., 2016).
2.1.1 Terrestrial invasive plants
Nearly half of the species introduced to the Galápagos Islands were intentional introductions of plants, primarily for agricultural purposes; non-native plants now outnumber endemic flora (Toral-Granda et al., 2017; Guézou et al., 2010; Tye, 2006). In a review of IUCN Extinct species, alien plants were implicated as a cause for 25% of plant and 33% of animal extinctions (Blackburn et al., 2019), demonstrating the major impact of invasive species on native flora and fauna. Sax and colleagues also proposed the possibility of a lag time between the introduction of an invasive species and extinction of native species, leading to an “extinction debt” (Sax et al., 2002; Sax and Gaines, 2008) that may need to be paid, even in the absence of new introductions of invasive species.
The blackberry shrub (Rubus niveus) is one of the most widespread invasive plants in the Galápagos Islands and serves as a prime example of the vast economic and biodiversity impacts of introduced flora (Rentería et al., 2012). Originally introduced for agriculture on Santa Cruz and San Cristóbal Islands in the 1960s-70s, R. niveus has since spread to other islands and overgrown into vast thickets, displacing native flora and diminishing arable land (Rentería et al., 2012, 2021). R. niveus has been associated with diminished richness of native plants and implicated in the altered structure of the Scalesia pedunculata forest, a key Galápagos ecosystem (Rentería et al., 2012; Riegl et al., 2023). Jäger et al. (2024) found that land invaded by R. niveus showed a 71% decrease in S. pedunculata and suggested that extinction of S. pedunculata on Santa Cruz Island was likely within 20 years if R. niveus growth is not curtailed (Jäger et al., 2024).
Currently, the most common strategies for invasive plant control and eradication in the Galápagos Islands are mechanical removal followed by chemical application (Jager et al., 2024). However, these methods have limitations. Mechanical removal is labor-intensive, requires removal of the roots to prevent regrowth, and does not account for recurrence due to residual seeds or wind dispersal. Chemicals can deteriorate soil quality and have off-target effects on insects and vertebrates. In addition, control methods must be differentiated from eradication strategies. Control methods suppress populations of invasive species but by virtue of being incomplete, incur a cumulative financial, time, and personnel cost (Gardener et al., 2013). Eradication strategies often require a much larger input of funding at the onset, but over time, may be comparable in cost to long-term maintenance of control strategies (Gardener et al., 2013). However, eradication plans, by virtue of the goal of complete removal of the target species, require even more intensive planning and complete implementation to ensure success. Gardener et al. (2010) reported that only four of 30 plant eradication projects carried out in the Galápagos Islands between 1996 and 2010 were successful; the remaining projects were discontinued due to insufficient time, lack of funding, or lack of permission to access privately owned land. In addition, management strategies geared toward the control of individual invasive species must still consider that the removal of one invasive species could result in replacement by, and downstream consequences of, other invasive species (Gardener et al., 2013).
More recently, biological control methods have also been investigated to target invasive plants in the Galápagos Islands. Biological control of invasive species involves the release of a living organism – typically bacteria, fungi, or insects – leading to selective depopulation of the invasive species. Ideal biological control methods will have minimal off-target effects on endemic plants and animals, including invertebrates which may serve as important pollinators, as well as on plants grown for agriculture. The Australian ladybird beetle (Novius cardinalis), for instance, was introduced to the Galápagos Islands in 2002 to control the invasive cotton scale insect (Icerya purchasi), successfully reducing their populations (Calderón Alvaréz et al., 2012). In March 2023, 100,000 sterile Aedes aegypti mosquitoes were released on Santa Cruz Island as part of a collaborative campaign to eradicate the mosquito, spearheaded by ABG and the National Institute for Public Health Research (INSPI) and supported by the Galápagos Conservancy. Similar biological control methods have been successful elsewhere, such as the eradication of the American screwworm (Cochliomyia hominivorax) from several countries in the Americas (Wyss, 2000). The fungus Puccinia lantanae has been suggested as a potential biocontrol agent of Lantana camara, an invasive perennial shrub in the Galápagos Islands, with no documented effects on the related endemic Lantana pedicularis (Rentería and Ellison, 2004; Thomas et al., 2021).
In March of 2022, the CDF and GNPD co-hosted a workshop aimed at developing biological control methods for R. niveus and fostering multisectoral collaborations, including a project with the Centre for Agriculture and Bioscience International (CABI) to identify predators of R. niveus in its natural range (Pollard et al., 2019). Given its devastating impact on native ecosystems and the time- and financial-intensity of current control methods, biological control is an attractive proposition for the eradication of this invasive species. In addition, since R. niveus is the only species in the Rubus genus in Galápagos Islands, off-target effects on other plants may be easier to avoid (Galapagos Conservancy, 2022).
The Charles Darwin Foundation has also successfully implemented drones and satellites to monitor the landscape for changes in plant biodiversity, allowing conservation efforts to specifically target at-risk areas. Restorative efforts include removal of invasive plants followed by replanting of endemic flora, alongside education of local farmers on the importance of allowing their land to be repopulated (CDF, 2023c).
2.1.2 Terrestrial invasive invertebrates
As of 2006, almost 500 insects and arthropods had been introduced to the archipelago (Causton et al., 2006). Unintentional introduction of invertebrates occurs primarily via hitchhiking on imported plants or produce, or on transport vehicles (e.g. boats or planes) (Toral-Granda et al., 2017). Preventing the introduction of invertebrates is essential for several reasons: invertebrates may destroy native vegetation, serve as predators or parasites of endemic species and thereby alter population dynamics and ecosystem stability, and/or serve as vectors of infectious diseases that could affect both humans and animals.
The now-naturalized southern house mosquito (Culex quinquefasciatus) and the yellow fever mosquito (A. aegypti), for instance, were introduced to the Galápagos Islands via airplanes (Bataille et al., 2009a, b; Whiteman et al., 2005; Sinclair, 2017). These mosquitoes are competent vectors of human and animal diseases, including West Nile Virus (WNV) (Kilpatrick et al., 2006; Sardelis et al., 2001), canine heartworm (Barnett, 1985a, b; Hendrix et al., 1986), and avian malaria (Plasmodium spp.) (van Riper et al., 1986; Harvey-Samuel et al., 2021) and thus pose risks for infectious disease introduction and establishment (Causton et al., 2006; Nishida and Evenhuis, 2000). In 1996, shortly after C. quinquefasciatus is thought to have been introduced, a survey of Galápagos penguins (Spheniscus mendiculus) identified no positive cases of avian malaria (Miller et al., 2001). Conversely, in 2013, avian malaria was identified in Galápagos penguins (Palmer et al., 2013; Levin et al., 2013) and yellow warblers (Setophaga petechia) (Levin et al., 2013), proposed to have been transmitted from migratory birds to mosquitoes in the archipelago, and subsequently transmitted to endemic birds. These findings suggest that the naturalization of C. quinquefasciatus was sufficient to allow avian malaria to gain a foothold in the Galápagos Islands. Currently, control of C. quinquefasciatus in the Galápagos Islands is primarily via fumigation of airplanes with insecticides and reduction of mosquito-attracting light sources in tourist areas (Harvey-Samuel et al., 2021).
The invasive bot fly, Philornis downsi, is another major threat to birds in the archipelago. Introduced to the Galápagos Islands in 1964 (Causton et al., 2006), P. downsi has since become widespread (Fessl et al., 2018). P. downsi has been associated with severe population declines in at least 16 species of Galápagos land birds, including the critically endangered mangrove finch (Fessl et al., 2018). The parasitic P. downsi larvae feed on hatchlings and cause severe morbidity and mortality (Causton et al., 2013). The CDF and GNPD have ongoing multi-center collaborations to continue researching the biology and impacts of P. downsi and implement appropriate control strategies. However, control of this parasite is challenging because diagnosis and treatment require access to nests and the use of methods that are both safe for birds, and do not result in environmental contamination with potential off-target effects on other species, including native insects. Boulton et al. (2019) recently demonstrated that insect traps placed below the canopy were most effective at trapping P. downsi adults and excluding bycatch of other insects, a finding that will augment the capture of this species for research and could contribute to eradication strategies while minimizing the impact on endemic insects. While chemical control with pesticides such as permethrin and cyromazine is effective against P. downsi, application methods must reduce off-target effects on native species. Current methods include injection, spray, and self-fumigation (Bueno et al. (2021); injection and spraying require direct nest access, which is physically challenging, requires more time and personnel, and has the potential to cause stress in nesting birds. Self-fumigation involves supplying permethrin-treated cotton to native finches as nest material and has been shown to be a successful method of pesticide dispersion effective against P. downsi (Knutie et al., 2014). Recently, biological control of P. downsi has also been investigated, with researchers concluding that the parasitoid wasp Conura annulifera had minimal impacts on non-target hosts and thus merits further study as a potential method to control P. downsi (Boulton et al., 2019).
Two species of fire ants are invasive in the Galápagos Islands - the little red fire ant (Wasmannia auropunctata) and the tropical fire ant (Solenopsis geminata) (Herrera & Causton, 2008; Wauters et al., 2015), both thought to have been introduced in the early 1900s (Wetterer and Porter, 2003; Clark et al., 1982; Wheeler, 1919). Given their small size and predilection for nesting at the base of trees, W. auropunctata may have been transported via imported plants or soil (Roque-Albelo and Causton, 1999). W. auropunctata is particularly successful as an invasive pest because it can reproduce both clonally and sexually, thus increasing their populations rapidly (Foucaud et al., 2009) and further complicating eradication efforts. Both species of ants have been linked to declines in diversity of other invertebrates (Wauters et al., 2015, 2014; Roque-Albelo and Causton, 1999; Williams & Whelan, 1991; Lubin, 1984; Armbrecht and Ulloa-Chacón, 2003; Kastdalen, 1982; Silberglied, 1972), including other ant species, due to predation (Holway et al., 2002) and resources/territory competition (Jourdan, 1997). W. auropunctata is also involved in transporting immature life stages of I. purchasi (Wetterer and Porter, 2003) with which it has a symbiotic relationship. While W. auropunctata has not been reported to impact vertebrates in the archipelago, it has been documented to sting or bite reptiles, domestic animals, and humans in other regions (Rosselli and Wetterer, 2017; Jourdan et al., 2001, 2022), suggesting that this ant may also have the potential to threaten Galápagos vertebrates. S. geminata is responsible for hatchling mortality in native reptiles and birds, including tortoises and penguins, and also attacks older animals (Williams & Whelan, 1991; Marquez et al., 2004; Roque-Albelo and Causton, 1999; Tapia, 1997).
Historically, control of invasive fire ants was primarily through vegetation clearance and pesticide application. While the use of methoprene, an insect growth regulator, was largely ineffective at achieving population reduction in W. auropunctata (Ulloa-Chacon and Cherix, 1994), serial application of hydramethylnon (Amdro) achieved presumptive eradication of W. auropunctata from Santa Fé Island in 1990, with subsequent surveys failing to identify any individuals over the course of several years (Abedrabbo, 1994). Similar efforts were successful at eradicating W. auropunctata from a large portion of Marchena Island in 2001 (Causton et al., 2005). Based on these historical efforts, Wetterer and Porter (2003) suggested that eradication of W. auropunctata could be accomplished with 4-5 treatments of insecticide bait over two years, with intensive post-treatment surveillance using food bait, if the area treated did not exceed several hectares. Large infestations, however, would be more challenging, particularly without access to extensive financial resources. We also note that care should be taken when interpreting a lack of sightings as indicative of true eradication. In addition, reintroduction of ants through interisland transport is a possibility, and has been implicated as one of the reasons for failure of eradication of S. geminata thus far (CDF, 2024). As of December 2023, the Galápagos Conservancy reported a collaboration with ABG to enhance biosecurity screening for insects at ports of entry, waste disposal sites, and marketplaces, as well as collaborations with farmers to minimize the impacts of invasive ants on agriculture (Galápagos Conservancy, 2023).
Given the off-target effects of pesticide application on native invertebrates, alternatives to pesticides should continue to be investigated. Orasema minutissima, a wasp that naturally parasitizes W. auropunctata, has been suggested as a potential agent for biological control (Heraty et al., 2021; Wetterer and Porter, 2003; Heraty, 1994). O. costaricensis, a related introduced wasp, is already present in the Galápagos Islands without reported negative environmental effects (Heraty, 1994; Peck et al., 1998), which may support the potential of O. minutissima as a biological control agent (Heraty, 1994).
ABG currently utilizes food bait to capture insects at Galápagos ports of entry, as well as in airports in Quito and Guayaquil on mainland Ecuador (the only two airports with flights to the Galápagos) to identify potentially invasive species (Guerrero et al., 2019). Airplanes bound for Galápagos must undergo disinfection procedures before departure. Since 2017, cargo traveling to Galápagos are also treated with pesticides. Reference collections are available at ABG checkpoints to aid personnel in rapid detection of invasive species. ABG also hosts educational workshops to engage farmers in active surveillance for invasive pests (Guerrero et al., 2019).
2.1.3 Terrestrial invasive vertebrates
Without natural predators, domestic animals and rodents introduced to the Galápagos Islands by mariners and early settlers established large feral colonies, with major downstream effects. Carnivorous and omnivorous invasive vertebrates, such as dogs, swine, and rodents, are responsible for predation of endemic species. In an analysis of extinctions documented in the IUCN database, Sax and Gaines (2008) reported that predation has been involved in nearly 80% of all terrestrial vertebrate extinctions worldwide. Grazing species are a major source of habitat alteration, decline in vegetation diversity, and resource competition. For example, feral goats are responsible for significant habitat destruction, incurring an estimated cost of 20 million US dollars between 1983 and 2017 (Ballesteros-Meija et al., 2021). Cattle and horses have caused extirpation of native plant species (Bush et al., 2022), a factor which also contributes to takeover by invasive plants.
Following recognition of these impacts, conservation programs were implemented with the goal of eradicating invasive animals. In 1974, Santiago Island had an estimated 100,000 feral goats and 20,000 feral pigs (deVries and Black, 1983). Through a combination of land and aerial depopulation methods, goats and pigs were completely eradicated from Santiago Island by 2005 (Cruz et al., 2009, 2005). Similarly, Project Isabela was responsible for the depopulation of over 140,000 goats from Isabela Island through hunting, with the aid of “Judas goats” wearing radio collars, and “Mata Hari” goats - sterilized female goats induced into estrus and then released on the island to lead hunters to the remaining goats. A small population of goats remains on Isabela Island and is maintained in check via aerial surveillance (Carrion et al., 2011). Intensive conservation efforts also resulted in presumptive eradication of feral donkeys from Santiago Island and the Alcedo Volcano area of Isabela Island by 2005 (Carrion et al., 2007); pigeons from Santa Cruz, San Cristóbal, and Isabela Islands by 2005 (Phillips et al., 2012a); and cats from Baltra Island in 2003 (Phillips et al., 2012b). In each case, negative surveillance for 1-2 years following the last known sighting led to conclusions that eradication was successful.
Eradication of rodents has also been a major goal for the Galápagos Islands. Rodenticides such as brodifacoum have been utilized to successfully control rat populations on various islands. In 2012, black rats were eradicated from Pinzón Island with widespread aerial application of bait containing brodifacoum rodenticide, leading to enhanced survival of Pinzón giant tortoise hatchlings (Rueda et al., 2019). In 2019, the GNPD partnered with non-profit organization Island Conservation to deploy drones carrying rodenticide on North Seymour Island and Mosquera Islet; both locations were declared free of rats by the GNPD in 2021 (Uribe, 2021). Eradication efforts for rats and feral cats on Floreana Island were also implemented in October of 2023 (Galapagos Conservation Trust, 2023a), with the goal of subsequently introducing 15 locally extinct species from neighboring islands (Galapagos Conservation Trust, 2023b).
Despite the benefits of widespread bait distribution systems for future rodent eradication programs, care must be taken to minimize off-target effects on other species. For example, ingestion of rodenticide by lava lizards was documented on Pinzón Island, and while the lizards themselves suffered no documented effects, rodenticide relay toxicosis led to morbidity and mortality in endemic Galápagos hawks (Rueda et al., 2016; Rueda et al., 2019).
While depopulation efforts have mitigated the destruction caused by invasive vertebrates, much work remains. In particular, canine overpopulation is a significant problem for endemic Galápagos species. Domestic dogs were first introduced to the archipelago in 1832 (Barnett, 1986), with large feral dog colonies present through the 1980s (Barnett and Rudd, 1983; Reponen et al., 2014). Canine nest predation of giant tortoises and land iguanas resulted in population declines that ultimately necessitated the development of nest conservation programs (Barnett, 1986). Canine predation upon the endemic marine iguana, Galápagos penguin, Galápagos sea lion, Galápagos fur seal, blue pelican, blue-footed booby, and Audubon shearwater have also been reported (Barnett and Rudd, 1983; Kruuk and Snell, 1981). Canine depopulation efforts by the GNPD and CDF, principally via bait laced with toxic sodium monofluoroacetate (Compound 1080), led to drastic reductions in feral dog populations in the 1980s (Barnett and Rudd, 1983; Barnett, 1986). While feral dog colonies are no longer prevalent in the archipelago, pet dogs are still commonly free-roaming (Jimenez et al., 2020; Diaz et al., 2016; Gingrich et al., 2010) and may thereby encounter wildlife, leading to predation, injury, or disease transmission (Barnett, 1985b). Ectoparasites, such as ticks, may transmit disease or cause hematologic abnormalities in domestic dogs, humans or wildlife. Free-roaming domestic dogs may also be the victims of vehicular trauma or other injuries. Controlling dog populations thus remains a key focus. Spay and neuter campaigns have been ongoing over the past decade; however, as of 2018, the human:dog ratio was estimated to be 4:1 on Santa Cruz Island, a 55% increase in the dog population since 2014 (Hernandez et al., 2020), demonstrating that current campaigns are still insufficient to mitigate population growth (Hernandez et al., 2020). Re-establishment of truly feral canine colonies in remote regions is a low but present risk (Reponen et al., 2014).
Another prominent example of the persistent impacts of introduced vertebrates is that of the smooth-billed ani (Crotophaga ani), intentionally introduced in the 1960s as a form of biological control to predate upon cattle ticks (Cooke et al., 2019). The smooth-billed ani is now considered the most damaging invasive bird in the Galápagos Islands, owing to its rapid population growth and predation of native plants and animals, particularly endemic birds (Connett et al., 2016), snakes (Cooke et al., 2020), and the endemic Galápagos carpenter bee (Xylocopa darwinii), a major pollinator. The bird also propagates invasive plants by ingesting and spreading their seeds (Cooke et al., 2019). The widespread negative impacts of the smooth-billed ani illustrate the danger of implementing biological control efforts without appropriate risk management considerations. Since 1980, the CDF has studied the impacts of this species and evaluated eradication techniques, but no large-scale control plan has yet been implemented, and the bird remains widespread (Cooke et al., 2019). A major challenge in developing an efficient eradication strategy for this invasive bird is the lack of data on its typical behavior and life history. By studying the size of their ecological range, breeding strategies, nest-building, migration, and diet, researchers may prioritize areas to target for intervention.
2.1.4 Invasive marine species
Overall, marine invasive species, particularly invertebrates, have been understudied (Keith et al., 2016; Baert, 1994; Carlton et al., 2019), complicating an accurate assessment of current numbers and ecosystem-level impacts. Marine traffic from tourism, fishing, and cargo shipping is likely the major route by which marine invasive species enter the GMR (Keith et al., 2016; Carlton et al., 2019). Carlton et al. (2019) hypothesized that shoreline structures such as docks and buoys also facilitate colonization by marine species introduced via the hulls of ships. According to the CDF, at least 59 invasive marine species have been documented in the archipelago, including the green algae Caulerpa chemnitzia (CDF, 2023a). Fast-growing algae can outcompete corals, particularly in already compromised areas; C. chemnitzia is thus a threat to Darwin Island’s Wellington Reef (CDF, 2023a). Recent work by Keith et al. (2022) reported that C. chemnitzia populations grow and contract in response to climate change, calling for an early detection rapid response system to monitor marine invasive species. A baseline of the species in the GMR and modes of entry for invasive species must be defined to inform prevention and eradication strategies.
2.2 Infectious diseases
Non-native hosts and vectors contribute to the introduction and establishment of infectious diseases in susceptible endemic populations. Introduced pathogens also have the potential to establish wildlife reservoirs, enabling them to circulate within wildlife populations and subsequently to be transmitted back to humans or domestic animals (Haydon et al., 2002). Many of the pathogens described in this section remain under-researched in the Galápagos Islands; therefore, in many cases, the presence of wildlife reservoirs has yet to be confirmed. Nonetheless, it is important to identify pathogens for which potential local reservoir hosts exist. Prevention and early detection are key, as once wildlife reservoirs are established, disease eradication becomes increasingly difficult and may become impossible (Dowdle and Hopkins, 1998; Guertin, 2019). Strategies used to target domestic animal reservoir hosts, such as mass vaccination or depopulation, can be more logistically challenging, expensive, labor-intensive, and/or ethically complex to implement in wildlife species (Barnett and Civitello, 2020; Miguel et al., 2020; Sharma and Hinds, 2012).
Domestic dogs are one of the most prominent potential reservoirs of infectious diseases that could affect Galápagos wildlife. Canine vaccination was prohibited in the Galápagos Islands until 2017 (Levy et al., 2008; Vega-Mariño et al., 2023); the dog population thus remains under-vaccinated and susceptible to outbreaks. While ABG has since promoted vaccination campaigns, efforts have not yet resulted in adequate herd immunity against common canine pathogens, such as Canine Distemper Virus (CDV) (Vega-Mariño et al., 2023) and canine parvovirus. For instance, an outbreak of CDV in 2001 resulted in the death of over 600 dogs and documented exposure of Galápagos sea lions (Levy et al., 2008). Due to lack of education and poor product availability, the use of adequate ectoparasite preventatives is also uncommon, leading domestic dogs to carry a high tick burden (Jimenez et al., 2020), which further promotes disease transmission in dogs, wildlife, and humans.
The food animal industry also presents a risk to wildlife, particularly birds. Food security and economic sustainability for humans in oceanic islands require stable sources of locally-produced food. As of 2014, approximately 100,000 chickens and over 3,500 pigs were present in the archipelago (Puente-Rodríguez et al., 2019). Until recently, importation of unvaccinated day-old chicks was common and represented a key exemption to the prohibition on animal importation; this practice was seen as necessary to supplement the poultry industry but could serve as a route of disease introduction (Puente-Rodríguez et al., 2019). Pathogens such as Newcastle Disease Virus (NDV), Marek’s Disease Virus (MDV), Infectious Bronchitis Virus (IBV) and Mycoplasma spp. have been detected in poultry in the Galápagos Islands, and could spread to wildlife, particularly from backyard flocks with contact with wild birds (Gottdenker et al., 2005; Whitehead et al., 2018; Wikelski et al., 2004).
In Table 4, we report selected bacterial, viral, fungal, and parasitic pathogens of One Health importance to the Galápagos Islands, the risks of which are summarized in the following sections. We focus on pathogens that have been documented in domestic animal reservoirs and/or in endemic wildlife, and/or that have zoonotic potential. For those pathogens without confirmed cases in Galápagos wildlife, such as Coxiella burnetii, we assess risk based on the competency of these pathogens in related wildlife species in other geographic regions. In addition, we include several pathogens that have not been identified in the Galápagos Islands, such as WNV, but which are still considered high risk. We also assign each pathogen a Risk Level, based on a consideration of the potential for introduction, global status of the disease, susceptibility of Galápagos species, presence of wildlife reservoirs and vectors, and zoonotic potential. It should be noted that this list is not comprehensive, but represents our assessment of pathogens for which monitoring should be prioritized.
2.2.1 Viral pathogens of importance to the Galápagos Islands
CDV is a paramyxovirus that affects wild and domestic carnivores (Martinez-Gutierrez and Ruiz-Saenz, 2016; Beineke et al., 2015). CDV is globally distributed and remains one of the leading causes of death in domestic dogs worldwide, including in the Galápagos Islands (Vega-Mariño et al., 2023). Transmission of CDV from domestic dogs to wildlife has been documented in other regions, posing a major threat to conservation (Williams et al., 1988; Gilbert et al., 2020; van de Bildt et al., 2002). While an outbreak of clinical disease caused by CDV has not occurred in Galápagos sea lions to date, positive pups and adults having been identified (Levy et al., 2008; Denkinger et al., 2017) and a recent study reported increasing seroprevalence (Ruiz-Saenz et al., 2023), suggesting that CDV presents a threat to this endemic species. Morbidity and mortality associated with CDV have been documented in other pinnipeds (Kennedy et al., 2019), suggesting that CDV may similarly pose a risk to the Galápagos fur seal. CDV also has the potential to become established in wild seal populations (Bengtson et al., 1991), posing a barrier to disease eradication.
Phocine distemper virus (PDV) is a paramyxovirus that emerged in 1988, likely derived from CDV following contact between domestic dogs and seals. Several outbreaks of PDV decimated seal populations in Arctic and North Atlantic waters between 1980 and 2006 (Duignan et al., 2014; Härkönen et al., 2006; Earle et al., 2006; Kennedy et al., 2019). In 2004, PDV was first identified in previously naïve marine mammals in the Northern Pacific Ocean, in association with sea ice reduction (Goldstein et al., 2009; Kennedy et al., 2019; VanWormer et al., 2019). While PDV has yet to be identified in tropical or subtropical climates, including the Galápagos Islands, its recent introductions into previously naïve populations and high mortality rate are concerning for continued spread of this pathogen, particularly in the context of climate change. Antibody cross-reactivity between CDV and PDV has been documented in seals, therefore real-time PCR (RT-PCR) is the gold standard for differentiating these related viruses (Stanton et al., 2004).
Viral pathogens of domestic poultry can affect economic stability and threaten endemic birds. MDV, NDV, IBV, and Infectious Bursal Disease Virus (IBDV) are highly infectious viruses, for which seropositive poultry have been identified on multiple islands in the archipelago (Soos et al., 2008; Whitehead et al., 2018; Deem et al., 2012a; Gottdenker et al., 2005; Wikelski et al., 2004). In the Galápagos Islands, wild birds of several species have tested seropositive for NDV, avian poxvirus, and avian adenovirus II (AAV-II) (Deem et al., 2012a). Conversely, several studies in other wild bird species have shown wild birds to be largely seronegative (Soos et al., 2008; Travis et al., 2006a, b; Padilla et al., 2003; Deem et al., 2011). These pathogens thus appear to be currently contained within the poultry industry. These studies also demonstrate that there are differences in susceptibility between endemic species. However, according to a 2008 survey on Santa Cruz Island (Soos et al., 2008), backyard poultry flocks were more likely to show clinical disease compared to broiler chickens, and had higher rates of seropositivity for infectious laryngotracheitis virus, IBR, avian reovirus, and MDV. This is a significant concern, given that backyard poultry are more likely to encounter wildlife, directly or through a shared environment, and are thus poised to facilitate spillover (Ayala et al., 2020). Ongoing surveillance is therefore necessary, in combination with enhanced biosecurity measures, particularly with regards to backyard poultry.
Avian poxvirus is another avian virus of major concern in the region. Avian poxvirus was anthropogenically introduced to the Galápagos Islands over a century ago (Parker et al., 2011) and has been associated with morbidity and mortality in Darwin’s finches and waved albatross (Phoebastria irrorata) (Tompkins et al., 2017; McNew et al., 2022; Kleindorfer and Dudaniec, 2008). Different finch species may have varying levels of susceptibility to this virus (Kleindorfer and Dudaniec, 2008; McNew et al., 2022). In addition, Zylberberg et al. (2013) reported that proximity to agricultural areas was a risk factor for avian poxvirus prevalence and suggested that agricultural land use may influence immunologic susceptibility. Further research is currently underway to assess the impacts of seasonality on avian poxvirus transmission in Galápagos passerines. Given the potential for stress and poor health status as a risk factor for the development of clinical disease associated with avian poxvirus, we may see increases in the prevalence of this virus in birds coinfected with other pathogens, or stressed by climate change, ecosystem disturbances, predation, or parasitism.
2.2.2 Bacterial pathogens of importance to the Galápagos Islands
Leptospirosis is a re-emerging zoonotic bacterial disease caused by the spirochete Leptospira interrogans. Rodents, particularly rats, serve as reservoirs. Leptospira is shed in the urine of infected individuals and then contaminates water and soil, remaining infective for months. Leptospirosis has been documented in Galápagos sea lions on San Cristóbal Island (Denkinger et al., 2017) as well as in the California sea lion (Zalophus californianus) (Lloyd-Smith et al., 2007), South American fur seal (Arctocephalus australis) and South American sea lion (Otaria flavescens) (Sepúlveda et al., 2015; Katz et al., 2022). Canine leptospirosis is endemic in South America (Rodriguez et al., 2004; Blazius et al., 2005; Calvopiña et al., 2023). Surveillance for leptospirosis in the Galápagos Islands has been minimal; however, one study in dogs in the archipelago identified no positive cases (Levy et al., 2008) and no human cases of leptospirosis were identified between 2000 and 2020 (Calvopiña et al., 2022). The patterns of transmission of Leptospira to endemic pinnipeds are therefore not yet known, and thus the risk factors for exposure, or potential routes of cross-species transmission, have yet to be elucidated. Barragan et al. (2016) suggested that livestock may be an understudied reservoir of leptospirosis in Ecuador; this possibility has not been evaluated in the Galápagos Islands.
Mycoplasma gallisepticum is a poultry pathogen with significant implications for wild birds. M. gallisepticum has been documented in backyard poultry and broilers on Santa Cruz (Soos et al., 2008) and Floreana Island (Deem et al., 2012a) and causes severe conjunctivitis, affecting sight, flight, and resource acquisition. Although M. gallisepticum has not yet been identified in surveyed wild birds (Soos et al., 2008; Deem et al., 2011), the potential remains for transmission from poultry. For instance, in North America, M. gallisepticum established reservoirs in house finches before becoming endemic in wild songbirds (Ley et al., 2016; Delaney et al., 2012; Sawicka et al., 2020), demonstrating the potential for establishment in wildlife.
Other bacterial pathogens of poultry, such as Mycoplasma synoviae, Bordetella avium, Pasteurella multocida, and Chlamydia psittaci, also pose threats to wild birds. For example, C. psittaci, a zoonotic pathogen that causes avian chlamydiosis and human psittacosis, has been identified in flightless cormorant (Nannopterum harrisi) (Travis et al., 2006b) and Galápagos dove (Zenaida galapagoensis) (Padilla et al., 2004). Conversely, Padilla et al. (2003) reported no waved albatross seropositive for C. psittaci. Variations in prevalence of C. psittaci in different bird species may be secondary to exposure patterns depending on season, location or diet, or differences in susceptibility due to genetic factors, immune status, or stress from concurrent underlying disease or other pressures.
In a survey of several vulnerable species of endemic Galápagos birds, Aaziz et al. (2023) identified Chlamydia abortus in 35.6% of waved albatross from Española Island. C. abortus is a causative agent of abortion in ruminants, but its potential as an avian pathogen is unknown (Szymanska-Czerwinska et al., 2017). To the authors’ knowledge, no studies have surveilled cattle in the Galápagos Islands for the presence of C. abortus. The role of livestock in the transmission of C. abortus to birds, or vice versa, is unknown and should be further investigated.
2.2.3 Parasitic diseases of importance to the Galápagos Islands
Dirofilaria immitis is the causative agent of canine heartworm disease and a zoonotic pathogen. This filarial nematode is transmitted by the bite of infected mosquitoes, after which adults develop and reside in the pulmonary arteries and can be recovered from these vessels as well from within the heart. In the 1980s, D. immitis microfilariae were first documented in the archipelago in dogs (Barnett and Rudd, 1983), demonstrating a domestic animal reservoir, and Galápagos sea lions (Barnett, 1985a) presenting a direct risk to this iconic endemic pinniped. D. immitis has since been identified in dogs on Isabela Island (Levy et al., 2008) and Santa Cruz Island, more commonly around brackish water lagoons that serve as a mosquito breeding site (Jimenez et al., 2020). A newer report also confirmed the presence of intracardiac adult heartworms in a Galápagos sea lion (Gregory et al., 2023). In addition, Barnett (1985a) surveyed humans on Floreana Island and documented 84% seropositivity for antibodies against D. immitis. Taken together, these reports illustrate concurrent risks to humans and wildlife secondary to a parasite with a reservoir in dogs. Additionally, the presence of both adult heartworms and circulating microfilariae in Galápagos sea lions suggests that this parasite can also circulate within a sylvatic cycle between mosquitoes and sea lions, posing an additional barrier to eradication. D. immitis has also been reported in South African fur seals (Arctocephalus pusillus pusillus) and common seals (Phoca vitulina) (Alho et al., 2017), as well as a Humboldt penguin (S. humboldti) (Sano et al., 2005), in other regions, suggesting that the Galápagos fur seal and Galápagos penguin are also at risk.
2.2.4 Emerging pathogens of One Health importance for the Galápagos Islands
Emerging pathogens discussed in this section include those recently documented among humans, domestic animals, and/or wildlife in the Galápagos Islands. This section also includes pathogens that have been documented in humans or domestic animals in the archipelago but have either not yet been documented in Galápagos wildlife or are in the early stages of diagnosis in wildlife.
2.2.4.1 Avian influenza
Avian influenza is an emerging pathogen in the Galápagos Islands. This virus is a zoonotic respiratory and gastrointestinal pathogen, with both Low Pathogenic (LPAI) and Highly Pathogenic (HPAI) forms. LPAI strains circulate naturally in wild birds, particularly waterfowl, and can spread to domestic birds via fecal contamination. LPAI typically causes mild or subclinical disease in poultry and is not considered a major public health threat. Wild waterfowl can carry multiple LPAI strains and remain subclinical. The emergence of HPAI is intrinsically linked to anthropogenic activities through the maintenance of poultry at high stocking densities in intensified agricultural conditions. Circulation of LPAI strains in poultry promotes the development of HPAI strains that then spill back over into wild birds; migrating birds can then spread HPAI along migration routes. HPAI strains cause severe disease, including respiratory, gastrointestinal, and neurologic signs, and high mortality, with outbreaks in poultry leading to severe economic losses. Because control measures of positive flocks involve depopulation, an HPAI outbreak can decimate the poultry industry.
While outbreaks of HPAI have periodically cycled through Eurasia and North America, South America has historically remained geographically insulated from this pathogen. However, in 2021, a new strain of HPAI, H5N1, emerged in Eurasia and rapidly spread to North America before spreading to Peru, presumably through wild bird migration. This strain is both highly transmissible and carries high mortality for poultry and wild birds, as well as mammals. In 2022, H5N1 was linked to mortality in harbor seal (Phoca vitulina) and gray seal (Halichoerus grypus) in Maine (Puryear et al., 2023) and dolphins (Delphinus delphis) and South American sea lions (Otaria flavescens) in Peru (Leguia et al., 2023). H5N1 has also spilled over into dairy cattle, causing clinical respiratory disease and reduced feed intake, and efficient transmission directly between cattle (Caserta et al., 2024). The virus appeared to have a tropism for mammary epithelium, and viral particles were identified in milk (Caserta et al., 2024).
The first outbreak of HPAI in Ecuador occurred in November of 2022, with high mortality in poultry (Bruno et al., 2023). In response, ABG issued an emergency resolution to prohibit importation of day-old chicks and poultry products, including meat and eggs, and to suspend interisland movement of poultry. ABG also initiated active surveillance for HPAI in poultry farms, for which all samples to date have been negative. Over the prior two decades, several studies had surveilled endemic Galápagos birds for HPAI, with no positive samples identified (Travis et al., 2006a, b; Padilla et al., 2003, Deem et al., 2012). Unfortunately, coinciding with the emergence of H5N1, HPAI was identified in the Galápagos Islands for the first time in September of 2023, following reports of mortality and clinical signs in wild birds on Wolf, Genovesa, and Darwin Islands (Gobierno del Ecuador, 2023; Cruz, 2024). Two dead frigate birds and one red-footed booby were confirmed positive for HPAI (Stokstad, 2023). Since the initial detection, ABG has rapidly established the capability to perform on-site molecular testing and intensive surveillance is ongoing. In December 2023, the GNPD reported that all samples collected from Galápagos penguins and flightless cormorants were negative (Primicias, 2023). ABG also plans to conduct genomic testing on any HPAI strains identified to assess origin, virulence, and transmissibility, informing risk assessment, management and containment strategies. As a precautionary measure, several visitor sites across the GNP have been closed pending further investigation. Education of farmers, tour guides, and GNPD staff is also necessary to increase the capacity for visual surveillance of birds and mammals, and to warn tourists to report, but not to approach, any animals with concerning signs. To aid in the response against HPAI in the Galápagos Islands, the UNESCO Rapid Response Facility (RRF) provided 40,000 USD toward seabird surveillance (UNESCO, 2024).
The potential impacts of H5N1 emergence in the Galápagos Islands cannot be understated, and thus ongoing efforts to identify and isolate cases are critical. H5N1 has the potential to cause large-scale mortality in poultry, threatening food security and economic stability, alongside the newly emerging threat of H5N1 to livestock. Given the prevalence of backyard poultry in the archipelago, an outbreak of HPAI in poultry also carries the risk of spillover into native birds. H5N1 could result in high morbidity and mortality if an outbreak occurred in Galápagos wild birds or mammals. Notably, several mutations concerning for mammalian host adaptation have been identified in samples from the recent Peruvian H5N1 outbreak, with direct mammal-to-mammal transmission suspected to play a role in sea lion die-offs (Leguia et al., 2023). These findings have dire implications for the Galápagos sea lion and fur seal in the event of an outbreak in these vulnerable species. Furthermore, Galápagos endemic wildlife are already under stress associated with anthropogenic pressures and El Niño weather patterns that affect temperature, humidity, and resource availability, which may render them more susceptible to infectious diseases.
Historically, HPAI infections in humans were primarily a risk for poultry workers or veterinarians, secondary to zoonotic transmission from infected poultry (Kaplan and Webby, 2013). The role of wildlife in the transmission of the current strain of H5N1 to humans is not yet clear; however, increased evidence for mammal-to-mammal transmission suggests that a larger-scale outbreak in humans may be possible. Given the evolving global status of the H5N1 outbreak, HPAI remains a transboundary disease with high risk and dire potential consequences for both humans and animals in the Galápagos Islands.
2.2.4.2 Severe acute respiratory syndrome related coronavirus 2 (SARS-CoV-2)
Severe acute respiratory syndrome related coronavirus 2 (SARS-CoV-2) is the causative agent of the Coronavirus disease 2019 (COVID-19) pandemic. The response to and impact of the COVID-19 pandemic in the Galápagos Islands was markedly different from that of mainland Ecuador, and will be covered in Part II of this review.
SARS-CoV-2 is thought to have emerged during a zoonotic spillover event from wildlife (Crits-Christoph et al., 2023), although the intermediate host is not definitively known. Birds, reptiles, and invertebrates do not appear to be susceptible to SARS-CoV-2 infection, but mammals may become infected. While no cases of SARS-CoV-2 have been documented in animals in the Galápagos Islands, there is some evidence that this pathogen could pose a threat to endemic marine mammals. SARS-CoV-2 utilizes the angiotensin-converting enzyme 2 (ACE2) receptor to enter host cells; ACE2 receptor structure has thus been used to predict the risk of SARS-CoV-2 infection in different mammals (Damas et al., 2020; Mathavarajah et al., 2021), with evidence suggesting that cetaceans, seals, and otters may be highly susceptible (Luan et al., 2020; Mathavarajah et al., 2021). Interestingly, ACE2 receptors in California sea lions had multiple mutations that led to reducing binding affinity of SARS-CoV-2, suggesting this species may be less susceptible to infection (Mathavarajah et al., 2021). If the same holds true for the Galápagos sea lion, this could confer a protective effect; however, until such data is available, the Galápagos sea lion and Galápagos fur seal should be considered potentially susceptible to SARS-CoV-2. Mathavarajah et al. (2021) suggested that marine mammals may be exposed to virus shed by infected humans into untreated wastewater. Ecuador is one of many countries in which untreated wastewater is discharged into natural waters (Guerrero-Latorre et al., 2020). Wastewater management in the Galápagos Islands will be further discussed in Part II of this review. While it is possible that exposure of marine mammals could occur in association with human contact – zooanthroponosis of SARS-CoV-2 has been documented in farmed American mink (Neovison vison) (Oude Munnink et al., 2021) – the long-standing prohibitions of close contact between humans and wildlife in the Galápagos Islands likely limit this possible route of exposure.
Despite the theoretical risk conferred by ACE2 receptor morphology, further research is necessary to fully assess the susceptibility of marine mammals to SARS-CoV-2. The anatomical distribution of ACE2 receptors varies between species; for instance, ACE2 receptors were present in the bronchiolar epithelium but not in the lungs of harbor seals (Lean et al., 2023) while juvenile bottlenose dolphins, but not adults, exhibited ACE2 receptors in the lungs (Audino et al., 2022). The distribution of receptors thus may affect the susceptibility of marine mammals exposed via inhalation. To date, no cases of SARS-CoV-2 natural infection in marine mammals have been reported worldwide. Johnstone and Baez (2021) suggested that enhanced investigation of coronaviruses in marine mammals should be pursued as a matter of both animal and public health.
2.2.4.3 Toxoplasma gondii and intestinal parasites
Toxoplasma gondii is a zoonotic parasite for which felids are the definitive host, shedding environment-contaminating oocysts (VanWormer et al., 2013) in the feces, which subsequently contaminate groundwater (VanWormer et al., 2014; Shapiro et al., 2019; Dubey et al., 2003). Ingestion of oocysts by mammals or birds results in formation of cysts within muscle or migration to the brain, causing neurologic signs.
T. gondii has been identified in domestic cats in the Galápagos Islands (Levy et al., 2008). Seropositivity for T. gondii has been reported in the Galápagos hawk (Deem et al., 2012b), Galápagos penguin, and flightless cormorant (Deem et al., 2010). More recently, T. gondii seropositivity in marine and terrestrial birds in the Galápagos Islands was reported to range from 13% in Nazca boobies (Sula granti) to 100% in Galápagos mockingbirds (Mimus parvulus), with diet being a risk factor for infection (Mosquera et al., 2023). T. gondii has also been reported in the California sea lion (Migaki et al., 1977; Carlson-Bremer et al., 2015) and other pinnipeds (Michael et al., 2016; Sepúlveda et al., 2015; Alvarado-Esquivel et al., 2012). Taken together, these results highlight the wide variety of species that can be affected by T. gondii, and illustrate the importance of considering risk factors for susceptibility in different species. Ongoing ABG research efforts include characterization of T. gondii prevalence in cattle and dogs from the four populated islands of the archipelago. ABG has also established a protocol for circumstantial monitoring of the Galápagos sea lion and Galápagos fur seal for this parasite, with preliminary results identifying positive individuals of both species (unpublished data). The prevalence of T. gondii in humans in the Galápagos Islands is not known.
The canine hookworm, Ancylostoma caninum, and the canine roundworm, Toxocara canis, have been identified in dogs in the Galápagos Islands (Diaz et al., 2016; Gingrich et al., 2010). These parasites can cause cutaneous and visceral larva migrans in humans, respectively. Neither species has been reported to affect pinnipeds; however, another hookworm species, Uncinaria spp., has been identified in otariids (Seguel and Gottdenker, 2017), including the Galápagos sea lion (Herbert, 2014). The potential thus remains for marine mammals to serve as aberrant hosts of canine intestinal parasites. In atypical hosts, nematodes tend migrate and cause extra-intestinal signs, which can include neurologic sequelae.
2.2.4.4 Vector-borne pathogens
As introduced in section 2.1.2, invasive arthropods are a major concern for transmission of vector-borne diseases. The naturalized yellow fever mosquito (A. aegypti) vectors dengue virus, chikungunya virus, and Zika virus, all of which are emerging pathogens in the Galápagos Islands, with the first human cases identified in 2002, 2015, and 2016, respectively (Ryan et al., 2019). During epidemics of these viruses, humans are the primary host and source of infection for new mosquitoes. In other regions, these viruses are also enzootically maintained between non-human primates and mosquitoes (Vasilakis et al., 2011; Gutierrez-Bugallo et al., 2019; Evans et al., 2022; Weaver et al., 2012). Seropositivity has also been documented in rodents and birds; however, there is scant evidence that these species develop clinical disease or are capable of infecting new mosquitoes (Silva and Dermody, 2017; Bueno et al., 2016; Gwee et al., 2021; Bosco-Lauth et al., 2016). Similarly, serologic and genetic evidence of dengue, Zika, chikungunya, and other arboviruses have also been identified in bats, although the epidemiological significance remains controversial (Fagre and Kading, 2019; Gwee et al., 2021). To our knowledge, no studies have evaluated the endemic Galápagos red bat (Laciurus borealis brachyotis) or the native hoary bat (Lasiurus cinereus spp. villosissimus) (Key and Sangoquiza, 2008; McCracken et al., 1997) in the context of infectious disease. It remains unknown whether there are any Galápagos wildlife capable of maintaining sylvatic cycles of arboviruses.
Ectoparasites, such as ticks, fleas, and lice, transmit disease and could transfer between animals and humans through close contact. The brown dog tick, Rhipicephalus sanguineus, commonly infests dogs in the Galápagos Islands (Jimenez et al., 2020) and will also rarely bite humans. R. sanguineus is a vector for several zoonotic diseases previously identified in Galápagos dogs, including Ehrlichia canis, E. ewingii, and Anaplasma phagocytophilum (Diaz et al., 2016; Jimenez et al., 2020). Ectoparasite preventatives are infrequently used in the region and free-roaming dogs may encounter parasites and come into contact with other potential hosts (Diaz et al., 2016; Jimenez et al., 2020). Thus, the risk of exposure to these canine vector-borne diseases is multifactorial.
Bartonella spp., a genus of zoonotic bacteria, has been identified in cats on Isabela Island (Levy et al., 2008). Bartonellosis has also been documented in seals in other regions, transmitted by lice (Morick et al., 2009), although no studies have identified Bartonella spp. in pinnipeds in the Galápagos Islands. Leishmania donovani, another zoonotic pathogen, has also been reported in dogs on Isabela Island (Levy et al., 2008), although its New World vector, the phlebotomine sand fly (Lutzomyia spp.), has yet to be identified in the archipelago. The identification of these pathogens in the Galápagos Islands in the absence of previously documented invertebrate vectors illustrates the importance of further research to identify the means by which these pathogens are vectored. Elucidating the regional life cycle of these pathogens will aid in surveillance and management.
2.2.4.5 Novel reptile adenoviruses and herpesviruses
In 2021, researchers identified novel adenoviruses and herpesviruses among five species of giant tortoises on Santa Cruz, Isabela, and Española Islands (Nieto-Claudin et al., 2021), with unknown pathogenic potential. Herpesviruses in other species are often latent chronic infections, exacerbated in the context of concurrent infection or immunosuppression. Therefore, further research on these viruses would help elucidate whether they represent a risk to the giant tortoise population.
2.2.4.6 Mycobacteria
Tuberculosis is an important disease worldwide, causing respiratory disease with characteristic granulomatous pulmonary lesions, and can be transmitted between humans, domestic animals, and wildlife, including between pinnipeds and humans (McDaniel et al., 2014; Bos et al., 2014; Brosch et al., 2002; Macedo et al., 2020). The Mycobacterium tuberculosis complex includes M. tuberculosis, M. bovis, and M. pinnipedii, among many others. Tuberculosis remains a public health concern in mainland Ecuador, and has been described in one case report from a patient in the Galápagos Islands (Garzon-Chavez et al., 2020). Although tuberculosis has not been identified in Galápagos pinnipeds, infections with the M. tuberculosis complex have been documented in multiple species of seals and sea lions (Forshaw and Phelps, 1991; Katz et al., 2022; Barnett et al., 2013), suggesting that Galápagos pinnipeds may be at risk. Given the potential for cross-species transmission, severity of clinical disease, and potential economic ramifications to the livestock industry, tuberculosis should be considered a disease of importance in humans, domestic animals, and wildlife.
2.2.4.7 Mycoplasma
Mycoplasma spp. are commensal organisms common to the respiratory tract of many reptiles, birds, and mammals, including pinnipeds (Greig et al., 2005). In the context of concurrent respiratory disease, Mycoplasma spp. can complicate and exacerbate clinical signs. Mycoplasma spp. isolated from Galápagos sea lions with concurrent respiratory signs appear to be distinct species from those commonly found in cats and dogs (Sarzosa et al., 2021), and thus merit further study with regards to their pathogenic potential. California sea lions are also host to the respiratory agent Mycoplasma zalophi (Sarzosa et al., 2021; Haulena et al., 2006), and the hemoplasm Mycoplasma haemozalophi (Volokhov et al., 2011); these species may pose risks to the related Galápagos sea lions.
2.2.5 Future infectious disease risks
This section includes pathogens that have yet to be documented in the Galápagos Islands, but for which competent vectors and/or reservoirs are present and for which the risk of introduction is high.
2.2.5.1 West Nile Virus (WNV) and other mosquito-transmitted arboviruses
WNV is a mosquito-transmitted flavivirus that causes disease in birds and mammals, including humans. WNV has not been detected in the Galápagos Islands, with negative surveys of wild birds on multiple islands (Eastwood et al., 2014), and a recent serological survey of horses finding no positive cases (Zanella et al., 2024). However, WNV is present in mainland Ecuador (Coello-Peralta et al., 2019). Given that two competent mosquito vectors of WNV, C. quinquefasciatus and A. aegypti, are naturalized in the Galápagos Islands (Eastwood et al., 2011, 2013, 2019; Bataille et al., 2009b), and with growing tourism and rising global temperatures, the potential establishment of WNV in the Galápagos Islands is an ongoing risk (Kilpatrick et al., 2006).
While WNV causes only sporadic disease among birds in Europe, its relatively recent introduction to immunologically naïve North American birds resulted in high morbidity and mortality, with severe febrile encephalitis (McLean, 2006; Sejvar, 2003). Galápagos birds are likely also naïve to this Old World virus, thus an outbreak would likely similarly result in major population declines. Thus, preventing the introduction of WNV into the archipelago is of paramount importance.
Other mosquito-transmitted arboviruses such as Eastern equine encephalitis virus (EEEV), Western equine encephalitis virus (WEEV), Venezuelan equine encephalitis virus (VEEV), and Japanese encephalitis virus (JEV) also pose risks as emerging zoonotic threats, in the context of climate change, urbanization, and tourism (Go et al., 2014; Mackenzie et al., 2016). To date, only one study has evaluated the seroprevalence of these viruses in Galápagos wildlife, with Travis et al. (2006a) finding no Galápagos penguins seropositive for these viruses. Nonetheless, the presence of competent mosquito vectors of these viruses raises the question of whether these pathogens could become established in the region and cause disease in both humans and animals.
2.2.5.2 Coxiella burnetii
Coxiella burnetii is an intracellular bacterial pathogen causing Q fever in humans and abortions in ruminants. C. burnetii is transmitted to humans via inhalation of aerosolized dust containing spores or direct contact with contaminated fluids or tissues from goats, sheep, or cattle (Welch, 2016). C. burnetii can also be vectored between wildlife and livestock via ticks. Due to its hardiness in the environment, potential for aerosolization, rapid spread and high infectivity, C. burnetii has also been identified as a potential bioterrorism agent (Kagawa et al., 2003) and poses a significant public health, veterinary health, and economic risk.
In infected humans, C. burnetii may cause acute, severe fever, headache, respiratory signs, and muscle and joint pain. Chronically infected individuals may develop hepatitis and endocarditis, with higher mortality than acute cases. In some cases, patients may be asymptomatic during acute disease and only diagnosed after development of chronic sequelae, at which time some changes may be irreversible.
C. burnetii has been identified in ruminant herds worldwide (Bauer et al., 2022; Bwatota et al., 2022; El-Mahallawy et al., 2015; Epelboin et al., 2023; Georgiev et al., 2013; Kim et al., 2005). However, overall, this pathogen remains understudied in Latin America (Epelboin et al., 2023). C. burnetii is endemic in Ecuador, with a seroprevalence of 43-53% in dairy cattle and 34% in farm workers (Carbonero et al., 2015; Changoluisa et al., 2019; Echeverría et al., 2019). Two studies to date have investigated the prevalence of C. burnetii in the Galápagos Islands. In one study, 500 bovine serum samples were analyzed by enzyme-linked immunosorbent assay (ELISA), with no positive cases (Chalan and Omar, 2021). In the second study, 5 milk samples from dairy cattle were negative on molecular testing (Rojas et al., 2013). However, these studies are likely insufficient to fully declare the Galápagos Islands free of C. burnetii, and ongoing surveillance should be performed. C. burnetii has also been identified in Australian fur seals (Arctocephalus pusillus doriferus), although its significance as a causative agent of abortion in this species remains unclear (Gardner et al., 2022); this pathogen should thus also be considered a potential risk to the Galápagos fur seal.
The emergence of C. burnetii in the Galápagos Islands would pose a threat to human health, wildlife health, economic stability, and food security. Occupational exposure among workers in Ecuador is likely underreported, posing a barrier to timely diagnosis and treatment. Given the potential for chronic disease sequelae, such a situation in the Galápagos Islands could compromise long-term health and increase the burden on healthcare systems. In addition, reduced production from livestock would reduce income while increasing pressures on other sectors to provide local sources of food. Given that Galápagos fur seals bear only one pup per breeding season, a pathogen that causes abortion could devastate their already declining populations.
2.2.5.3 Fungal pathogens
With climate change and rising global temperatures, fungal agents are poised to emerge as important pathogens (Nnadi and Carter, 2021), yet fungi remain understudied globally. Fungal spores are also notoriously resilient to degradation. Several fungal organisms have emerged as wildlife pathogens in the past decades, including chytrid fungus (Batrachochytrium dendrobatidis) of amphibians and white-nose syndrome (Pseudogymnoascus destructans) of bats. Cryptococcus gattii, a systemic fungal pathogen of humans and animals, has caused outbreaks of pulmonary and neurologic disease in humans, marine mammals, and penguins (Rosenberg et al., 2016; Fenton et al., 2017; Huckabone et al., 2015; Venn-Watson et al., 2012; Brito Devoto et al., 2022).
Several studies have surveyed Galápagos fungi in association with soil, trees, vegetation, and insects (Ajello and Padhye, 1974; Schoenborn et al., 2023; Nelder et al., 2004; James et al., 2015; Freitas et al., 2013; Guamán-Burneo et al., 2015), but few studies focus on fungi as pathogens (Carvajal Barriga et al., 2014). There are only two clinical reports of fungal lesions in the region; Sutton et al. (2013) and Christman et al. (2020) identified two novel fungal species in Galápagos tortoises, from carapace and pulmonary lesions, respectively. Environmental surveillance efforts should be implemented to assess for the presence of fungi with pathogenic potential.
2.2.5.4 Other pathogens for future surveillance
Surveillance of infectious diseases in cattle, goats, pigs, and horses in the Galápagos Islands has been limited. Livestock may transmit diseases to humans (McDaniel et al., 2014) or to wildlife, either through direct contact or through fomite transmission. ABG conducted extensive surveillance of cattle between 2014 and 2015, with no evidence of Brucella abortus (Gioia et al., 2018), Foot and Mouth Disease, or bovine leukosis virus. Nonetheless, ongoing surveillance is necessary. Some pathogens affecting livestock are also related to those that cause disease in wildlife – for instance, the virus that causes Vesicular Exanthema of Swine is genetically indistinguishable from San Miguel Sea Lion Virus.
Many pathogens affecting the California sea lion likely have the potential to affect the Galápagos sea lion, even if they have yet to be studied in the latter, and thus should be targets for future surveillance in the Galápagos Islands. For example, otarine adenovirus 1, related to canine adenovirus 1 and 2, causes sea lion viral hepatitis (Goldstein et al., 2011). California sea lions are also host to otariine herpesvirus-1 (Gulland et al., 2020) and their own caliciviruses (Smith and Boyt, 1990), the respiratory agent Mycoplasma zalophi (Sarzosa et al., 2021; Haulena et al., 2006), and the hemoplasm Mycoplasma haemozalophi (Volokhov et al., 2011).
2.3 Antimicrobial resistance
In 2013, the United States Centers for Disease Control and Prevention (CDC) highlighted the global public health implications of antimicrobial resistance (AMR) via the first Antibiotic Resistance Threats report (CDC, 2013). In early 2022, the CDC reported that the SARS-CoV-2 pandemic prompted a significant increase in antibiotic use and AMR-associated infections worldwide, in part due to enormous pressure placed on global healthcare networks (CDC, 2022). AMR affects human, domestic animal and wildlife health. Factors contributing to AMR include overuse, prophylactic use, and subtherapeutic use (e.g. growth-promotion in livestock) of broad-spectrum antibiotics (CDC, 2013; FDA, 2017). Antibiotics exert selective pressure for antimicrobial resistance genes (ARGs), which can then be horizontally transferred between bacteria. Despite efforts to safeguard public health through judicious use of antibiotics, AMR remains a significant threat to healthcare worldwide. AMR infections result in prolonged hospitalization, economic hardship, failure of treatment and increased morbidity and mortality (Dadgostar, 2019).
Several studies have evaluated AMR in the Galápagos Islands, all supporting a link between human-wildlife contact and the presence of bacteria with ARGs. Thaller et al. (2010) reported that AMR was exceedingly rare (~1%) among land iguanas (Conolophus pallidus) in remote areas of Santa Fé Island. Conversely, Wheeler et al. (2012) documented a higher rate of AMR in E. coli isolated from reptiles in close proximity to human areas compared to remote areas (Wheeler et al., 2012). Nieto-Claudin et al. (2021) also found that fecal ARGs were more prevalent in giant tortoises from urban and agricultural zones compared to remote areas. Taken together, these studies suggest that proximity to humans increases the risk of exposure to bacteria with ARGs. Sewage discharge at beaches on San Cristóbal Island was associated with higher concentrations of Enterococcus and more AMR in E. coli (Overbey et al., 2015), providing one potential explanation for transfer of AMR strains in association with human activities. In addition, antibiotics carried in tourist luggage with inappropriate use or disposal could contribute to environmental contamination, with downstream effects for human and wildlife health.
While the presence of AMR bacteria does not necessarily confirm a pathogenic role, their presence is concerning for two main reasons: 1) many commensal or environmental microbes may become opportunistic pathogens in the context of concurrent stress, disease, or immune compromise; and 2) if an infectious disease outbreak were to occur in endemic wildlife, the presence of AMR would complicate treatment, particularly in a region where access to antibiotics is limited even in the public health sector.
3 Regulations and surveillance
3.1 Institutions with a role in building the “One Health” strategy for the Galápagos Islands
At the national level, several agencies overlap in responsibilities to protect public health. INSPI is the national surveillance and reference laboratory that provides specialized public health services for Ecuador (Gobierno del Ecuador, 2022a). The Phytosanitary and Zoosanitary Regulation and Control Agency (AGROCALIDAD), associated with the Ministry of Agriculture and Livestock is responsible for the regulation, protection, and improvement of animal and plant health, and food safety (Gobierno del Ecuador, 2022b). The National Agency for Regulation, Control and Sanitary Surveillance (ARCSA) regulates the quality, safety, and efficacy of medicines, processed foods, and cosmetics, and conducts sanitary surveillance (Gobierno del Ecuador, 2022c). Notably, these institutions act primarily at the continental level, but have agreements with agencies in charge of health of the Galápagos Islands.
In 2003, the Ecuadorian government enacted the Regulation for the Total Control of Introduced Species in Galápagos Province (RCTEI), which further defined regulations on transport of food products into and within the archipelago, and established a Provincial Agricultural Health and Quarantine Committee, responsible for reviews and recommendations every 5 years. RCTEI also outlines biosecurity procedures, such as disinfection of vessels and a system of vessel certification, with reported compliance of 95% (Brewington et al., 2012). Additional recommendations have been made to reduce the risk of contaminated maritime equipment and ballast water (Brewington et al., 2012).
The institutions that govern sustainability of the Galápagos Islands locally are the Government Council (CREG), focused on public policy; the GNPD, centered on management of protected areas and wildlife; and ABG, safeguarding animal, vegetal, and human health. ABG is a technical-public entity attached to the Ministry of the Environment, responsible for monitoring diseases of public health and veterinary importance. ABG’s lines of action were framed to comply with the Invasive Species Management Plan for Galápagos, summarized in Table 5 (Espinosa and Cedeño, 2022). ABG carries out surveillance, diagnostics, and research for timely identification of threats in urban and wild fauna. ABG is also responsible for emergency response plans, disease control programs, and epidemiological research. To monitor the food production sector, ABG institutes biosecurity and containment measures for livestock, including quarantine procedures and slaughterhouse inspections, and certifies the movement of animals and animal products between islands (Gobierno del Ecuador, 2022d).
Given the unique ecological status of the Galápagos Islands, international and intergovernmental organizations play key roles in setting conservation goals and evaluating progress. Approximately every 8 years, the UNESCO World Heritage Committee evaluates the status of World Heritage sites based on voluntary legislative and administrative updates provided by the State Party (UNESCO, 2023a). New Action Plans are then formulated with discrete goals to address urgent challenges. The most recent report for the Galápagos Islands was presented on November 28, 2022, highlighting threats of tourism, land use, unregulated or illegal fishing, and invasive species (UNESCO, 2023b). The corresponding conclusions from the World Heritage Centre and Advisory Bodies will be further discussed in Part II of this review.
The IUCN Red List monitors wildlife populations and is an invaluable monitoring and assessment tool. However, several caveats should be considered when using the IUCN Red List to assess the conservation status of Galápagos species. The interval between IUCN assessments is variable, thus the most recent IUCN classification may not accurately reflect a species’ current conservation status. Accurate assessments rely on available scientific data, which may be scarce. For instance, the San Cristóbal giant tortoise (C. n. chathamensis) was first assessed in 1996 as Vulnerable and not re-assessed until 2017, when it was classified as Endangered (Caccone et al., 2017). In addition, many species have yet to be evaluated by the IUCN. Thus, the IUCN Red List should not be used to exclude certain species from conservation simply because they have not yet been classified. Most importantly, the global status of a species may not fully reflect the conservation status of local or regional populations. The IUCN states that the Red List was “developed for assessing extinction risk at the global level … If the criteria are used on their own to assess non-endemic species at regional or national levels, this could result in incorrect or even misleading assessments” (IUCN, 2022). Particularly on oceanic islands, isolated populations have diversified into subspecies and are subject to distinct pressures compared to the parent species. For instance, the blue-footed booby (Sula nebouxii) is native to the Galápagos Islands but is also found throughout the Gulf of California and western Central and South America. The Galápagos endemic subspecies, S. n. excisa, has declined by over 50% in the past two decades (Anchundia et al., 2014) and is considered Endangered by the Charles Darwin Foundation, yet the parent species remains listed as Least Concern by the IUCN as of 2020 (CDF, 2023b; BirdLife International, 2021). Similarly, endemic subspecies of the short-eared owl (Asio flammeus ssp. galapagoensis), great blue heron (Ardea herodias ssp. cognata), and yellow-crowned night heron (Nyctanassa violacea ssp. pauper) are all regionally threatened, despite global populations remaining of Least Concern according to the IUCN. These considerations may in part explain differences between the IUCN and CDF assessments of risk status of Galápagos endemic species.
Multidisciplinary collaboration between governmental institutions, non-profit and non-governmental organizations, and private entities or private research centers are key to maintain biosafety and identify strategies to mitigate existing threats. Organizations such as the CDF, Galápagos Science Center (GSC), and the Galápagos Conservancy promote sustainability through conservation and research, providing personnel, funding, and expertise to drive key projects forward. These organizations also conduct global campaigns to increase public awareness of threats facing the Galápagos Islands, leading to a valuable source of external funding.
3.2 Discussion and recommendations
The terrestrial and marine environments of the Galápagos Islands are subject to distinct, but sometimes overlapping, pressures. As defined by Snell et al. (2002), the primary threat to marine biodiversity is the exploitation of key species through fishing, poaching, or accidental killing, compounded by the effects of climate change and invasive species. Terrestrial ecosystems are most threatened by invasive species and associated predation, competition, and habitat alteration (Snell et al., 2002). As an interdisciplinary field, Planetary Health holds that understanding these threats is key in developing appropriate management and prevention strategies.
In this review, we outlined key invasive species threats in the region and review key control and eradication efforts, as well as emerging technologies. It is necessary to continue researching and developing eradication plans for invasive species. Currently, biological control agents appear to be a promising avenue for further research, given their potential to be highly selective for the target species. However, due to the often-irreversible nature of biological control agents, stringent risk assessments must be conducted before implementation. Otherwise, species introduced with the goal of serving as biological control agents could themselves become invasive and have downstream consequences for native species.
Ongoing monitoring for recurrence is also important even in areas where eradication efforts are thought to have been successful. Care should be taken when interpreting a lack of sightings as conclusive evidence of eradication. Additionally, until a given invasive species is entirely eradicated from the archipelago, there is an ever-present risk of reintroduction through anthropogenic interisland movement, and thus a need for ongoing surveillance and prevention.
In developing control and eradication strategies, it is critical to engage various sectors (Gardener et al., 2013). However, reaching a consensus on management plans can be challenging, given differences in values between stakeholders. For example, many invasive species in the archipelago were introduced for agricultural purposes and were once considered economically important. Community education is therefore critical to enhance buy-in and encourage property owners to allow land access. In addition, Trueman et al. (2010) also suggested the implementation of inter-island quarantine efforts to reduce invasive plant introductions.
We also recommend the implementation of a reporting system that can be utilized by the public to alert authorities to invasive species sightings, thus facilitating a rapid, targeted response. For instance, while ABG conducts routine surveillance throughout the archipelago, a cluster of reports of invasive arthropods on one island would allow ABG personnel to increase the frequency or scope of their surveillance at that site, as well as adopt techniques particularly suited to the sighted species, such as aerial traps for mosquitoes, or ground baits for ants. A particular challenge for a publicly accessible reporting system is the large volume of reports and the capacity of personnel to manually review all the submitted images. The use of emerging artificial intelligence technologies offers a potential avenue to process images, remove false positive results, and prioritize only likely invasive species for further review by biosecurity officials. Given the volume of tourism in the archipelago, engagement of citizen science (for instance, through an application downloaded by tourists) may be an unexplored avenue to augment regional biosecurity efforts in the Galápagos Islands. For example, the mobile application iNaturalist has been used to track the spread of invasive invertebrates and plants in other areas (Fisher et al., 2022; Pawson et al., 2020; Dimson et al., 2023). Several research groups have also reported bulk molecular surveillance protocols to identify invasive arthropods from mixed-species samples on insect traps (Butterwort et al., 2022; Mee et al., 2021).
In this review, we also document many pathogens with the potential to cause disease in humans and endemic wildlife in the Galápagos Islands, and prioritized these pathogens with a “Risk Level” in Table 4. Notably, while we acknowledge the importance of prioritizing surveillance for diseases that are involved in active outbreaks, we also include in this list some agents that have been documented in domestic animals but not in wildlife in the Galápagos Islands, and some that have never been documented in the region but have a high potential for introduction and/or severe consequences for human or animal health. Inclusion of these latter two categories is crucial when considering biosecurity from a One Health perspective, because it acknowledges 1) that disease transmission across human-domestic animal-wildlife interfaces is a viable route for pathogen introduction, and 2) existing factors may make spread of certain pathogens very likely in the future, such as the potential for anthropogenic transport or the presence of competent arthropod vectors. Outbreaks of pathogens that affect humans, domestic animals, and wildlife, such as HPAI or C. burnetii, have the potential to simultaneously cause crises in public health and animal health, while also impacting food security, economic sustainability, and biodiversity. Furthermore, once emerging pathogens establish wildlife reservoirs, eradication efforts would become vastly more technically difficult, costly, and time-intensive, if not impossible. Detection of biologic threats before they become established is therefore critical. Thus, our goal was to highlight agents which should be considered important threats, regardless of their current presence in the region, as maintaining vigilance for these pathogens will inform appropriate biosecurity measures to exclude or contain them.
ABG is a bold institution due to its administrative autonomy and sanctioning/regulatory power, and its acceptance as a regulatory unit by the local, national, and international community. On some Islands, ABG sites have the most advanced infrastructure, laboratory equipment, and technical and managerial capacity. ABG also has the scope to collaborate with inter-institutional and international organizations. As a relatively young entity, however, ABG can still strive to implement improvements at an institutional and operational level.
Due to the large volume of tourist activity and reliance on imported products, air and sea are potential routes for introduction of invasive species or pathogens via passenger or cargo vessels, and require rigorous, active surveillance. For inspection and quarantine, ABG maintains a list of approved and prohibited organic products (ABG, 2013), devised by balancing supplying internal markets with the risk of biologic threats (Rogg et al., 2005). Currently, the list of prohibited products is only published in Spanish, limiting full accessibility to tourists. Providing lists in English to tour companies and cruise ships would increase awareness and improve compliance. Additionally, having these lists is not entirely beneficial if baggage or import inspections are not rigorous. X-ray scanning of crates and baggage is performed on the mainland and upon arrival at official ports of entry. However, there is often a time delay between scanning, cargo loading, and vessel departure, during which time crates may be stored for days in open air. Improving the efficiency of cargo surveillance and loading would decrease exposure to pests and contamination. Cargo could be tagged with designated ship dates and X-ray scanning and visual inspection conducted just prior to loading. Additionally, where pre-shipment storage is necessary, cargo should be stored indoors on raised pallets in clean, dry, and vermin-proof areas. Cargo crates are currently randomly selected for visual inspection, but it is not possible to open every crate. To mitigate this, we recommend the incorporation of sniffer dogs trained to detect organic materials to allow targeted inspections of cargo, similar to current policies for passenger baggage arriving to Baltra and Cristóbal airports.
Since ABG is considered a public investment project, activities may also be constrained by budget availability and delays in accessing external funding. There are also limitations in equipment, reagents, or infrastructure to support advanced diagnostic techniques. ABG requires personnel with specialized training in microbiology, epidemiology, biosecurity, and related fields. Current legislation, meant to promote local economic growth, restricts the contracts available to non-Galapagueños. In practice, however, there is a lack of investment in education opportunities for Galápagos residents, thus these positions may remain unfilled. Expansion of human talent acquisition is essential to ensure that ABG has the expertise and bandwidth to carry out all necessary surveillance and monitoring.
We recognize the challenges inherent in conducting surveillance for multiple pathogens, particularly when ABG must prioritize resources toward current public health efforts and/or actively emerging threats. Ultimately, surveillance goals must be developed at the regional level, considering many overlapping factors. Regardless of the pathogens prioritized, however, surveillance strategies should aim to span human, domestic animal, and wildlife interfaces. For instance, in 1985, humans on Floreana Island were documented to have a high rate of seropositivity for antibodies against D. immitis, a pathogen of humans, dogs, and pinnipeds. However, no studies in the past 40 years have evaluated the prevalence of this disease in humans in the Galápagos Islands. Serologic testing is more readily conducted in human patients and pet dogs than in wildlife, and results would inform both public health and the regional risk to sea lions. Surveillance efforts should also utilize a combination of diagnostic tools to build a comprehensive picture, recognizing that seropositivity does not indicate active infection, and even infected individuals may be subclinical for disease. For instance, while the archipelago may currently be considered free of C. burnetii based on the two studies in the literature, more comprehensive surveys should be conducted of livestock serum samples and bulk milk tank samples. EEEV, WNV, yellow fever virus, and dengue virus can be identified directly from mosquitoes via polymerase chain reaction (PCR) (Hadfield et al., 2001; Ali et al., 2022), which may prove useful in identifying and managing newly introduced and/or emerging pathogens before clinical cases occur.
In addition, occupational surveillance and education on disease recognition should be provided for farmers. Research to identify new vectors of disease and potentially novel pathogens should also be prioritized. For example, the arthropod vector of Leishmania in the region has not yet been identified; without a full picture of this pathogen’s life cycle, it is extremely difficult to develop appropriate risk mitigation strategies.
Control of canine overpopulation, vaccination campaigns, limiting contact with wildlife, and appropriate education of pet owners is necessary to prevent the spread of diseases for which domestic dogs and cats serve as a reservoir (Vega-Mariño et al., 2023). Preventing domestic cats and dogs from roaming freely would also decrease environmental contamination with fecal parasites. Pet owners should be diligent about collecting waste to reduce the risk of groundwater contamination. For instance, T. gondii takes 48 hours to sporulate in the environment, thus discarding cat feces daily reduces household exposure to infectious stages of the parasite. At the regional level, development of appropriate wastewater treatment and solid waste handling strategies are necessary to prevent groundwater contamination from pet feces discarded in municipal trash. In addition, further research should evaluate AMR in the Galápagos Islands in the context of domestic and wild species to develop guidelines for antibiotic stewardship in the public health, agriculture, and regulatory sectors.
Most of the Galápagos Islands is a protected area (PA). Research in Southeast Asia has shown that effectively managed PAs are an asset to conservation, with increased wildlife diversity inside reserves and in adjacent unprotected areas (Brodie et al., 2023). However, many large PAs suffer from lack of resources and infrastructure for efficient management, thus creating “paper parks.” It is imperative that the Galápagos Islands do not fall prey to this pitfall, particularly where human presence and wildlife conservation are so closely intertwined and managed visitation of protected areas are a core economic component. Continued assessment of the biodiversity landscape is necessary to ensure that GNP protections are functioning as intended.
Given that many challenges cross international boundaries or stem from global anthropogenic activities, collaboration between regional and national authorities and international stakeholders has become a necessary component of building effective management strategies for the region. However, research efforts by international scientists must actively strive to produce tangible and direct regional benefits, and integrate solutions for preservation of biodiversity while supporting local development. Scientists must prioritize ethical research practices (MacClancy and Fuentes, 2013). This includes consideration of the ecological impacts of specimen collection and field studies (ranging from stress caused by human presence to transmission of pathogens), and appropriately compensating and acknowledging local participants. Furthermore, research methodology should be able to be replicated using local resources, enabling study results to be translated into actionable management plans and compared to future data. Under the current infrastructure, barriers to communication also impede the efficiency of surveillance programs. Information-sharing must be enhanced by expanding scientific journal access to avoid information gatekeeping and establishing digital repositories and translations of print literature to ensure preservation and accessibility. A central electronic database for sharing diagnostic, epidemiological, and geographic reports would enhance information transfer and transparency, ensuring that different sectors are not operating in silos. This database would be of most utility in the context of a One Health monitoring network, to consolidate information generated by research institutions for access by policymakers, regulatory agencies, and scientists, enabling efficient and informed decision-making. Ultimately, a reciprocal exchange of knowledge should be prioritized.
Author contributions
IAJ: Conceptualization, Data curation, Investigation, Methodology, Project administration, Supervision, Writing – original draft, Writing – review & editing. PV-M: Conceptualization, Investigation, Writing – original draft, Writing – review & editing. TV: Investigation, Writing – original draft, Writing – review & editing. ELH: Conceptualization, Funding acquisition, Investigation, Resources, Supervision, Writing – original draft, Writing – review & editing.
Funding
The author(s) declare that no financial support was received for the research, authorship, and/or publication of this article.
Conflict of interest
PV-M is a paid employee of ABG.
The remaining authors declare that the research was conducted in the absence of any commercial or financial relationships that could be construed as a potential conflict of interest.
Publisher’s note
All claims expressed in this article are solely those of the authors and do not necessarily represent those of their affiliated organizations, or those of the publisher, the editors and the reviewers. Any product that may be evaluated in this article, or claim that may be made by its manufacturer, is not guaranteed or endorsed by the publisher.
Supplementary material
The Supplementary Material for this article can be found online at: https://www.frontiersin.org/articles/10.3389/fcosc.2024.1351707/full#supplementary-material
References
Aaziz R., Vinueza R. L., Vorimore F., Schnee C., Jiménez-Uzcátegui G., Zanella G., et al. (2023). Avian Chlamydia abortus Strains Detected in Galápagos Waved Albatross (Phoebastria irrorata). J. Wildl Dis. 59, 143–148. doi: 10.7589/JWD-D-21-00163
Abedrabbo S. (1994). “Control of the little fire ant Wasmannia auropunctata on Santa Fe Island in the Galápagos Islands,” in Exotic Ants: Biology, Impact, and Control of Introduced Species. Ed. Williams D. F. (CRC Press, Boca Raton), 219–227. doi: 10.1201/9780429040795
Adame F. (2021). Meaningful collaborations can end “helicopter research. Nat. Career Columns. doi: 10.1038/d41586-021-01795-1
Agencia de Regulación y Control de la Bioseguridad y Cuarentena para Galápagos. (2013). Subproductos y derivados de origen vegetal y animal reglamentados para su ingreso a la provincial de Galápagos. Available online at: https://bioseguridadGalápagos.gob.ec/lista-de-productos/ (Accessed 18 September, 2023).
Ajello L., Padhye A. (1974). Keratinophilic fungi of the Galápagos Islands. Mykosen 17, 239–243. doi: 10.1111/j.1439-0507.1974.tb04484.x
Alho A. M., Marcelino I., Colella V., Flanagan C., Silva N., Correia J. J., et al. (2017). Dirofilaria immitis in pinnipeds and a new host record. Parasit Vectors 10, 142. doi: 10.1186/s13071-017-2073-0
Ali E. O. M., Babalghith A. O., Bahathig A. O. S., Dafalla O. M., Al-Maghamsi I. W., Mustafa N. E. A. G., et al. (2022). Detection of dengue virus from Aedes aegypti (Diptera, culicidae) in field-caught samples from Makkah Al-Mokarramah, Kingdom of Saudi Arabia, using RT-PCR. Front. Public Health 10, 850851. doi: 10.3389/fpubh.2022.850851
Alvarado-Esquivel C., Sánchez-Okrucky R., Dubey J. P. (2012). Serological evidence of Toxoplasma gondii infection in captive marine mammals in Mexico. Vet. Parasitol. 184, 321–324. doi: 10.1016/j.vetpar.2011.08.036
Anchundia D., Huyvaert K. P., Anderson D. J. (2014). Chronic lack of breeding by Galápagos Blue-footed Boobies and associated population decline. Avian Conserv. Ecol. 9, 6. doi: 10.5751/ACE-00650-090106
Arbogast B. S., Drovetski S. V., Curry R. L., Boag P. T., Seutin G., Grant P. R., et al. (2006). The origin and diversification of Galápagos mockingbirds. Evolution 60, 370–382.
Armbrecht I., Ulloa-Chacón P. (2003). The little fire ant Wasmannia auropunctata (Roger) (Hymenoptera: Formicidae) as a diversity indicator of ants in tropical dry forest fragments of Colombia. Environ. Entomol. 32, 542–547. doi: 10.1603/0046-225X-32.3.542
Audino T., Berrone E., Grattarola C., Giorda F., Mattioda V., Martelli W., et al. (2022). Potential SARS-coV-2 susceptibility of cetaceans stranded along the Italian coastline. Pathogens 11, 1096. doi: 10.3390/pathogens11101096
Ayala A. J., Yabsley M. J., Hernandez S. M. (2020). A review of pathogen transmission at the backyard chicken-wild bird interface. Front. Vet. Sci. 7. doi: 10.3389/fvets.2020.539925
Baert L. (1994). Notes on the Status of Terrestrial Arthropods in Galápagos. Noticias de Galápagos, 15–21.
Ballesteros-Mejia L., Angulo E., Diagne C., Cooke B., Nuñez M. A., Courchamp F. (2021). Economic costs of biological invasions in Ecuador: the importance of the Galápagos Islands. NeoBiota 67, 375–400. doi: 10.3897/neobiota.67.59116
Barnett B. D. (1985a). El gusano del corazon del perro (Dirofilaria immitis) en las Galápagos (Galápagos Ecuador: Biblioteca Charles Darwin).
Barnett B. D. (1985b). “Impact of domestic dog populations in the Galápagos: prevalence and transmission of canine heartworm,” in Dogs of the Galápagos Islands: Evolution, Ecology, Impact and Control (Davis, California, USA: University of California). Davis Doctoral dissertation. (Retrieved from University Microfilms International Dissertation Abstracts database. Accession No. 852195).
Barnett B. D. (1986). “Eradication and control of feral and free-ranging dogs in the Galápagos Islands,” in Proc. 12th Vertebr. Pest C. Ed. Salmon T. (University of California, Davis), 358–368.
Barnett J. E., Booth P., Brewer J. I., Chanter J., Cooper T., Crawshaw T., et al. (2013). Mycobacterium bovis infection in a grey seal pup (Halichoerus grypus). Vet. Rec. 173, 168. doi: 10.1136/vr.101480
Barnett K. M., Civitello D. J. (2020). Ecological and evolutionary challenges for wildlife vaccination. Trends Parasitol. 36, 970–978. doi: 10.1016/j.pt.2020.08.006
Barnett B. D., Rudd R. L. (1983). Feral dogs of the Galápagos Islands: impact and control. Int. J. Stud. Anim. Prob. 4, 44–58.
Barr D. A., Reece D. L., O’Rourke D., Button C., Faragher J. T. (1988). Isolation of infectious bronchitis virus from a flock of racing pigeons. Aust. Veterinary J. 65 (7), 228. doi: 10.1111/j.1751-0813.1988.tb14468.x
Barragan V., Chiriboga J., Miller E., Olivias S., Birdsell D., Hepp C., et al. (2016). High leptospira diversity in animals and humans complicates the search for common reservoirs of human disease in rural ecuador. PloS Negl. Trop. Dis. 10 (9), e0004990. doi: 10.1371/journal.pntd.0004990
Bataille A., Cunningham A. A., Cedeno V., Cruz M., Eastwood G., Fonseca D. M., et al. (2009a). Evidence for regular ongoing introductions of mosquito disease vectors into the Galápagos Islands. Proc. R. Soc Lond. Biol. 276, 3769–3775. doi: 10.1098/rspb.2009.0998
Bataille A., Cunningham A. A., Cedeno V., Patino L., Constantinou A., Kramer L. D., et al. (2009b). Natural colonization and adaptation of a mosquito species in Galápagos and its implications for disease threats to endemic wildlife. Proc. Natl. Acad. Sci. 106, 10230–10235. doi: 10.1073/pnas.0901308106
Bauer B. U., Schoneberg C., Herms T. L., Runge M., Ganter M. (2022). Surveillance of Coxiella burnetii Shedding in Three Naturally Infected Dairy Goat Herds after Vaccination, Focusing on Bulk Tank Milk and Dust Swabs. Vet. Sci. 9, 102. doi: 10.3390/vetsci9030102
Beineke A., Baumgärtner W., Wohlsein P. (2015). Cross-species transmission of canine distemper virus-an update. One Health 1, 49–59. doi: 10.1016/j.onehlt.2015.09.002
Bengtson J. L., Boveng P., Franzen U., Have P., Heidejorgensen M.-P., Harkönen T. J. (1991). Antibodies to canine distemper virus in Antarctic seals. Mar. Mammal Sci. 7, 85–87. doi: 10.1111/j.1748-7692.1991.tb00553.x
Bensted-Smith R. (2002). A Biodiversity Vision for the Galápagos Islands: Based on an International Workshop of Conservation Biologists in Galápagos in May 1999 (Puerto Ayora, Galápagos: Charles Darwin Foundation).
Bensted-Smith R., Powell G., Dinerstein E. (2002). “Planning for the ecoregion,” in A biodiversity vision for the Galápagos Islands (Puerto Ayora, Galápagos: Charles Darwin Foundation and World Wildlife Fund), 1–5.
BirdLife International. (2021). Sula nebouxii (The IUCN Red List of Threatened Species). doi: 10.2305/IUCN.UK.2021-3.RLTS.T22696683A168988087.en
BirdLife International. (2017). Pyrocephalus dubius (amended version of 2016 assessment) (The IUCN Red List of Threatened Species). doi: 10.2305/IUCN.UK.2017-3.RLTS.T103682916A119211257.en
Blackburn T. M., Bellard C., Ricciardi A. (2019). Alien versus native species as drivers of recent extinctions. Front. Ecol. Environment. 17, 203–207. doi: 10.1002/fee.2019.17.issue-4
Blazius R. D., Romão P R. T., Blazius E. M. C. G., da Silva O. S. (2005). Occurrence of Leptospira spp. seropositive stray dogs in Itapema, Santa Catarina, Brazil. Cad Saude Publica. 21, 1952–1956. doi: 10.1590/s0102-311x2005000600046
Bos K. I., Harkins K. M., Herbig A., Coscolla M., Weber N., Comas I., et al. (2014). Pre-Columbian mycobacterial genomes reveal seals as a source of New World human tuberculosis. Nature 514, 494–497. doi: 10.1038/nature13591
Bosco-Lauth A. M., Nemeth N. M., Kohler D. J., Bowen R. A. (2016). Viremia in North American mammals and birds after experimental infection with Chikungunya viruses. Am. J. Trop. Med. Hyg. 94, 504–506. doi: 10.4269/ajtmh.15-0696
Boulton R. A., Bulgarella M., Ramirez I. E., Causton C. E., Heimpel G. E. (2019). “Management of an invasive avian parasitic fly in the Galapagos Islands: is biological control a viable option?,” in Island invasives: scaling up to meet the challenge. Occasional Paper SSC no. 62. Eds. Veitch C. R., Clout M. N., Martin A. R., Russell J. C., West C. J. (IUCN, Gland, Switzerland), 360–363.
Brewington L., Rosero O., Bigue M., Cervantes K. (2012). The quarantine chain: establishing an effective biosecurity system to prevent the introduction of invasive species into the Galápagos Islands (San Francisco: Wildaid). Available online at: https://wildaid.org/wp-content/uploads/2017/09/QuarantineChain.pdf (Accessed 10 September, 2023).
Brito Devoto T., Toscanini M. A., Hermida Alava K., Etchecopaz A. N., Pola S. J., Martorell M. M., et al. (2022). Exploring fungal diversity in Antarctic wildlife: isolation and molecular identification of culturable fungi from penguins and pinnipeds. N Z Vet. J. 70, 263–272. doi: 10.1080/00480169.2022.2087784
Brodie J. F., Mohd-Azlan J., Chen C., Wearn O. R., Deith M. C. M., Ball J. G. C., et al. (2023). Landscape-scale benefits of protected areas for tropical biodiversity. Nature (620), 807–812. doi: 10.1038/s41586-023-06410-z
Brosch R., Gordon S. V., Marmiesse M., Brodin P., Buchrieser C., Eiglmeier K., et al. (2002). A new evolutionary scenario for the Mycobacterium tuberculosis complex. Proc. Natl. Acad. Sci. U.S.A. 99, 3684–3689. doi: 10.1073/pnas.052548299
Bruno A., Alfaro-Núñez A., de Mora D., Armas R., Olmedo M., Garcés J., et al. (2023). Phylogenetic analysis reveals that the H5N1 avian influenza A outbreak in poultry in Ecuador in November 2022 is associated with the highly pathogenic clade 2.3.4.4b. Int. J. Infect. Dis. 133, 27–30. doi: 10.1016/j.ijid.2023.04.403
Bueno M. G., Martinez N., Abdalla L., Duarte Dos Santos C. N., Chame M. (2016). Animals in the zika virus life cycle: what to expect from megadiverse Latin American countries. PloS Negl. Trop. Dis. 10, e0005073. doi: 10.1371/journal.pntd.0005073
Bueno I., Singer R. S., Yoe C., Parrish R., Travis D. A., Ponder J. B. (2021). Optimizing risk management strategies for the control of Philornis downsi—A threat to birds in the Galápagos islands. Front. Conserv. Sci. 2. doi: 10.3389/fcosc.2021.721892
Bush M. B., Conrad S., Restrepo A., Thompson D. M., Lofverstrom M., Conroy J. L. (2022). Human-induced ecological cascades: extinction, restoration, and rewilding in the Galápagos highlands. Proc Natl Acad Sci USA. 119 (24), e2203752119. doi: 10.1073/pnas.2203752119
Butterwort V., Dansby H., Zink F. A., Tembrock L. R., Gilligan T. M., Godoy A., et al. (2022). A DNA extraction method for insects from sticky traps: targeting a low abundance pest, Phthorimaea absoluta (Lepidoptera: Gelechiidae) in mixed species communities. J. Economic Entomol. 115, 844–851. doi: 10.1093/jee/toac046
Bwatota S. F., Shirima G. M., Hernandez-Castro L. E., de Clare Bronsvoort B. M., Wheelhouse N., Mengele I. J., et al. (2022). Seroprevalence and Risk Factors for Q fever (Coxiella burnetii) Exposure in Smallholder Dairy Cattle in Tanzania. Veterinary Sci. 9, 662. doi: 10.3390/vetsci9120662
Caccone A., Cayot L. J., Gibbs J. P., Tapia W. (2017). Chelonoidis chathamensis (The IUCN Red List of Threatened Species) (Accessed 10 June, 2023).
Calderón Alvarez C., Causton C. E., Hoddle M. S., Hoddle C., Van Driesche R., Stanek E. III (2012). Monitoring the effects of Rodolia cardinalis on Icerya purchasi populations on the Galápagos Islands. BioControl 57, 167–179. doi: 10.1007/s10526-011-9429-8
Calvopiña M., Romero-Alvarez D., Vasconez E., Valverde-Muñoz G., Trueba G., Garcia-Bereguiain M. A., et al. (2023). Leptospirosis in Ecuador: current status and future prospects. Trop. Med. Infect. Dis. 8, 202. doi: 10.3390/tropicalmed8040202
Calvopiña M., Vásconez E., Coral-Almeida M., Romero-Alvarez D., Garcia-Bereguiain M. A., Orlando A. (2022). Leptospirosis: Morbidity, mortality, and spatial distribution of hospitalized cases in Ecuador. A nationwide study 2000-2020. PloS Negl. Trop. Dis. 16, e0010430. doi: 10.1371/journal.pntd.0010430
Carbonero A., Guzmán L. T., Montaño K., Torralbo A., Arenas-Montes A., Saa L. R. (2015). Coxiella burnetii seroprevalence and associated risk factors in dairy and mixed cattle farms from Ecuador. Prev. Vet. Med. 118, 427–435. doi: 10.1016/j.prevetmed.2015.01.007
Carlson-Bremer D., Colegrove K. M., Gulland F. M., Conrad P. A., Mazet J. A., Johnson C. K. (2015). Epidemiology and pathology of Toxoplasma gondii in free-ranging California sea lions (Zalophus californianus). J. Wildl Dis. 51, 362–373. doi: 10.7589/2014-08-205
Carlton J. T., Keith I., Ruiz G. M. (2019). Assessing marine bioinvasions in the Galápagos Islands: Implications for conservation biology and marine protected areas. Aquat. Invasions 14, 1–20. doi: 10.3391/ai.2019.14.1.01
Carmi O., Witt C. C., Jaramillo A., Dumbacher J. P. (2016). Phylogeography of the vermilion flycatcher species complex: Multiple speciation events, shifts in migratory behavior, and an apparent extinction of a Galápagos-endemic bird species. Mol. Phylogenet Evol. 102, 152–173. doi: 10.1016/j.ympev.2016.05.029
Carrion V., Donlan C. J., Campbell K., Lavoie C., Cruz F. (2007). Feral donkey (Equus asinus) eradications in the Galápagos. Biodiversity Conserv. 16, 437–445. doi: 10.1007/s10531-005-5825-7
Carrion V., Donlan C. J., Campbell K. J., Lavoie C., Cruz F. (2011). Archipelago-wide island restoration in the Galápagos Islands: Reducing Costs of Invasive Mammal Eradication Programs and reinvasion risk. PloS One 6, 18835. doi: 10.1371/journal.pone.0018835
Carvajal Barriga E. J., Barahona P. P., Tufiño C., Bastidas B., Guamán-Burneo C., Freitas L., et al. (2014). An Overview of the Yeast Biodiversity in the Galápagos Islands and Other Ecuadorian Regions (InTech). doi: 10.5772/58303
Caserta L. C., Frye E. A., Butt S. L., Laverack M., Nooruzzaman M., Covaleda L. M., et al. (2024). Spillover of highly pathogenic avian influenza H5N1 virus to dairy cattle. Nature. doi: 10.1038/s41586-024-07849-4
Causton C., Cunninghame F., Tapia W. (2013). “Management of the avian parasite Philornis downsi in the Galápagos Islands: A collaborative and strategic action plan,” in Galápagos Report 2011-2012 (GNPS, GCREG, CDF and GC, Puerto Ayora, Galápagos, Ecuador), 167–173.
Causton C. E., Peck S. B., Sinclair B. J., Roque-Albelo L., Hodgson C. J., Landry B. (2006). Alien insects: threats and implications for conservation of Galápagos Islands. Ann. Entomol. Soc Am. 99, 121–143. doi: 10.1603/0013-8746(2006)099[0121:AITAIF]2.0.CO;2
Causton C. E., Sevilla C. R., Porter S. D. (2005). Eradication of the little fire ant, wasmannia auropunctata (Hymenoptera: Formicidae) from marchena island, Galápagos: on the edge of success? Florida Entomologist. 88 (2), 159–168.
Cayot L. J., Gibbs J. P., Tapia W., Caccone A. (2022). Chelonoidis niger abingdonii (amended version of 2016 assessment) (The IUCN Red List of Threatened Species). doi: 10.2305/IUCN.UK.2022-1.RLTS.T9017A217759270.en
Centers for Disease Control and Prevention (2013). Antibiotic resistance threats in the United State. Available online at: https://www.cdc.gov/drugresistance/pdf/ar-threats-2013-508.pdf (Accessed 9 May, 2023).
Centers for Disease Control and Prevention (2022). COVID-19: U.S. Impact on antimicrobial resistance, special report 2022 (Atlanta, GA: U.S. Department of Health and Human Services). Available online at: https://www.cdc.gov/drugresistance/covid19.html (Accessed 9 May, 2023).
Chalan M., Omar D. (2021). Seroprevalencia y factores de riesgo asociados a Fiebre Q Coxiella burnetii en bovinos en las Islas Galápagos Ecuador. Available online at: http://dspace.utpl.edu.ec/jspui/handle/20.500.11962/27785 (Accessed 10 September, 2023).
Changoluisa D., Rivera-Olivero I. A., Echeverria G., Garcia-Bereguiain M. A., de Waard J. H., the working group “Applied Microbiology” of the School of Biological Sciences and Engineering at Yachay Tech University. (2019). Serology for Neosporosis, Q fever and Brucellosis to assess the cause of abortion in two dairy cattle herds in Ecuador. BMC Vet. Res. 15, 194. doi: 10.1186/s12917-019-1924-7
Charles Darwin Foundation. (2023a). 2022 impact report (52nd General Assembly of the Charles Darwin Foundation). Available online at: https://www.darwinfoundation.org/en/publications/annual-report/impact-report-2022 (Accessed 1 July 2023).
Charles Darwin Foundation. (2023b). Galápagos species checklist. Available online at: https://www.darwinfoundation.org/en/datazone/checklist (Accessed 28 Jan, 2023).
Charles Darwin Foundation. (2023c). Restoring endangered scalesia forests. Available online at: https://www.darwinfoundation.org/en/our-work/land/scalesia-forest-restoration/ (Accessed 3 August 2024).
Charles Darwin Foundation. (2024). Galápagos Species Database, Solenopsis geminata. Available online at: https://datazone.darwinfoundation.org/en/checklist/?species=6941 (Accessed 10 August 2024).
Chin A., Baje L., Donaldson T., Gerhardt K., Jabado R. W., Kyne P. M., et al. (2019). The scientist abroad: Maximizing research impact and effectiveness when working as a visiting scientist. Biol. Conserv. 238, 108231. doi: 10.1016/j.biocon.2019.108231
Christman J. E., Alexander A. B., Donnelly K. A., Ossiboff R. J., Stacy N. I., Richardson R. L., et al. (2020). Clinical manifestation and molecular characterization of a novel member of the Nannizziopsiaceae in a pulmonary granuloma from a Galápagos Tortoise (Chelonoidis nigra). Front. Vet. Sci. 7. doi: 10.3389/fvets.2020.00024
Clark D. B., Guayasamin C., Pazmino O., Donoso C., Paez de Villacis Y. (1982). The tramp ant Wasmannia auropunctata: Autoecology and effects on ant diversity and distribution on Santa Cruz Island, Galapagos. Biotropica 14, 196–207. doi: 10.2307/2388026
Coello-Peralta R., González-González M., Martínez-Cepeda G. (2019). West nile virus in Ecuador. J. MVZ Cordoba 24, 7151–7156. doi: 10.21897/rmvz.1603
Connett L., Guézou A., Herrera H. W., Carrión V., Parker P. G., Deem S. L. (2016). Gizzard contents of the smooth-billed ani Crotophaga ani in Santa Cruz, Galápagos Islands, Ecuador. Galápagos Res. 68, 43–48.
Conrad C., Gibbs J. P. (2021). “The era of exploitation: 1535–1959,” in Galápagos giant tortoises. biodiversity of world: Conservation from genes to landscapes. Eds. Gibbs J. P., Cayot L. J., Tapia W. A. (Cambridge: Academic Press), 63–81. doi: 10.1016/B978-0-12-817554-5.00002-2
Cooke S. C., Anchundia D., Caton E., Haskell L. E., Jäger H., Kalki Y., et al. (2020). Endemic species predation by the introduced smooth-billed ani in Galápagos. Biol. Invasions 22, 2113–2120. doi: 10.1007/s10530-020-02251-3
Cooke S. C., Haskell L. E., van Rees C. B., Fessl B. (2019). A review of the introduced smooth-billed ani Crotophaga ani in Galápagos. Biol. Conserv. 229, 38–49. doi: 10.1016/j.biocon.2018.11.005
Crits-Christoph A., Levy J. I., Pekar J. E., Goldstein S. A., Singh R., Hensel Z., et al. (2023). Genetic tracing of market wildlife and viruses at the epicenter of the COVID-19 pandemic. bioRxiv, 557637. doi: 10.1101/2023.09.13.557637
Cruz S. (2024). A brief account of the 2023 HPAI-H5N1 outbreak in the Galápagos Islands and impacts on local seabirds (Pacific Seabirds Publication). Available online at: https://pacificseabirdgroup.org/news/a-brief-account-of-the-2023-hpai-h5n1-outbreak-in-the-galapagos-islands-and-impacts-on-local-seabirds/ (Accessed 3 August 2024).
Cruz F., Carrion V., Campbell K. J., Lavoie C., Donlan C. J. (2009). Bio-economics of large-scale eradication of feral goats from Santiago Island, Galápagos. J. Wildlife Manage. 73, 191–200. doi: 10.2193/2007-551
Cruz F., Donlan C. J., Campbell K., Carrion V. (2005). Conservation action in the Galápagos: feral pig (Sus scrofa) eradication from Santiago Island. Biol. Conserv. 121, 473–478. doi: 10.1016/j.biocon.2004.05.018
Culda C. A., Dionnet R., Barbu A. C., Cârstolovean A. S., Dan T., Grijalva J., et al. (2022). The presence of dirofilaria immitis in domestic dogs on san cristobal island, galapagos. Pathogens. 11 (11), 1287. doi: 10.3390/pathogens11111287
Dadgostar P. (2019). Antimicrobial resistance: implications and costs. Infect. Drug Resist. 12, 3903–3910. doi: 10.2147/IDR.S234610
Damas J., Hughes G. M., Keough K. C., Painter C. A., Persky N. S., Corbo M., et al. (2020). Broad host range of SARS-CoV-2 predicted by comparative and structural analysis of ACE2 in vertebrates. PNAS 117, 22311–22322. doi: 10.1073/pnas.2010146117
Deem S. L., Cruz M. B., Higashiguchi J. M., Parker G. P. (2012a). Diseases of poultry and endemic birds in Galápagos: implications for the reintroduction of native species. Anim. Conserv. 15, 73–82. doi: 10.1111/j.1469-1795.2011.00489.x
Deem S. L., Merkel J., Ballweber L., Vargas F. H., Cruz M. B., Parker P. G. (2010). Exposure to Toxoplasma gondii in Galápagos Penguins (Spheniscus mendiculus) and flightless cormorants (Phalacrocorax harrisi) in the Galápagos Islands, Ecuador. J. Wildl Dis. 46, 1005–1011. doi: 10.7589/0090-3558-46.3.1005
Deem S. L., Parker P. G., Cruz M. B., Merkel J., Hoeck P. E. A. (2011). Comparison of blood values and health status of Floreana Mockingbirds (Mimus trifasciatus) on the islands of Champion and Gardner-by-Floreana, Galápagos Islands. J. Wildl Dis. 47, 94–106. doi: 10.7589/0090-3558-47.1.94
Deem S. L., Rivera-Parra J. L., Parker P. G. (2012b). Health evaluation of Galápagos Hawks (Buteo galapagoensis) on Santiago Island, Galápagos. J. Wildl Dis. 48, 39–46. doi: 10.7589/0090-3558-48.1.39
Delaney N. F., Balenger S., Bonneaud C., Marx C. J., Hill G. E., Ferguson-Noel N., et al. (2012). Ultrafast evolution and loss of CRISPRs following a host shift in a novel wildlife pathogen, Mycoplasma gallisepticum. PloS Genet. 8, e1002511. doi: 10.1371/journal.pgen.1002511
Delprete P. G. (1995). Systematic study of the genus delilia (Asteraceae, heliantheae). Pl. Syst. Evol. 194 (1/2), 111–122. doi: 10.1007/BF00983220
Denkinger J., Gordillo L., Montero-Serra I., Murillo J. C., Guevara N., Hirschfeld M., et al. (2015). Urban life of Galápagos sea lions (Zalophus wollebaeki) on San Cristobal Island, Ecuador: colony trends and threats. J. Sea Res. 105, 10–14. doi: 10.1016/j.seares.2015.07.004
Denkinger J., Guevara N., Ayala S., Murillo J. C., Hirschfeld M., Montero-Serra I., et al. (2017). Pup mortality and evidence for pathogen exposure in Galápagos sea lions (Zalophus wollebaeki) on San Cristobal Island, Galápagos, Ecuador. J. Wildl Dis. 53, 491–498. doi: 10.7589/2016-05-092
Denkinger J., Quiroga D., Murillo J. C. (2014). “Assessing human–wildlife conflicts and benefits of Galápagos sea lions on San Cristobal Island, Galápagos,” in The Galápagos Marine Reserve, vol. 2014. (Springer, Cham), 285–305.
deVries T., Black J. (1983). Of men, goats, & guava – problems caused by introduced species in the Galápagos. Noticias Galápagos 38, 18–21.
Diaz N. M., Mendez G. S., Grijalva C. J., Walden H. S., Cruz M., Aragon E., et al. (2016). Dog overpopulation and burden of exposure to canine distemper virus and other pathogens on Santa Cruz Island, Galápagos. Prev. Vet. Med. 123, 128–137. doi: 10.1016/j.prevetmed.2015.11.016
Dimson M., Berio-Fortini L., Tingley M. W., Gillespie T. W. (2023). Citizen science can complement professional invasive plant surveys and improve estimates of suitable habitat. Diversity Distributions 29, 1141–1156. doi: 10.1111/ddi.1374
Dirección del Parque Nacional Galápagos (2014). Plan de Manejo de las Áreas Protegidas de Galápagos pagos para el Buen Vivir (Galápagos Ecuador, Puerto Ayora: Ministerio del Ambiente Parque Nacional Galápagos). Available from: https://www.galapagos.gob.ec/wp-content/uploads/downloads/2016/07/DPNG_Plan_de_Manejo_2014.pdf.
Dowdle W. R., Hopkins D. R. (Eds.) (1998). The eradication of infectious diseases. (Report of the Dahlem workshop on the eradication of infection diseases, Berlin, March 16–22, 1997) (New York: John Wiley & Sons).
Dowler R. C., Carroll D. S., Edwards C. W. (2009). Rediscovery of rodents (Genus nesoryzomys) considered extinct in the Galápagos islands. Oryx 34 (2), 109–117. doi: 10.1046/j.1365-3008.2000.00104.x
Dubey J. P., Zarnke R., Thomas N. J., Wong S. K., Van Bonn W., Davis J., et al. (2003). Toxoplasma gondii, Neospora caninum, Sarcocystis neurona, and Sarcocystis canis-like infections in marine mammals. Vet. Parasitol. 116, 275–296. doi: 10.1016/S0304-4017(03)00263-2
Duignan P. J., Van Bressem M. F., Baker J. D., Barbieri M., Colegrove K. M., De Guise S., et al. (2014). Phocine distemper virus: current knowledge and future directions. Viruses 6, 5093–5134. doi: 10.3390/v6125093
Dumbacher J. P., West B. (2010). Collecting the Galápagos and the Pacific: How Rollo Howard Beck shaped our understanding of evolution. Proc. California Acad. Sci. 4, 211–243.
Dvorak M., Fessl B., Nemeth E., Kleindorfer S., Tebbich S. (2012). Distribution and abundance of Darwin’s finches and other land birds on Santa Cruz Island, Galápagos: evidence for declining populations. Oryx 46 (1), 78–86. doi: 10.1017/S0030605311000597
Dvorak M., Nemeth E., Wendelin B., Herrera P., Mosquera D., Anchundia D., et al. (2017). Conservation status of landbirds on Floreana: the smallest inhabited Galápagos island. J. Field Ornithol. 88, 132–145. doi: 10.1111/jofo.12197
Earle J. A. P., Melia M. M., Doherty N. V., Nielsen O., Cosby S. L. (2006). Phocine distemper virus in seals, east coast, United States. Emerg. Infect. Dis. 17, 215–220. doi: 10.3201/eid1702.100190
Eastwood G., Cunningham A. A., Kramer L. D., Goodman S. J. (2019). The vector ecology of introduced Culex quinquefasciatus populations, and implications for future risk of West Nile virus emergence in the Galápagos archipelago. Med. Vet. Entomol. 33, 44–55. doi: 10.1111/mve.12329
Eastwood G., Goodman S. J., Cunningham A. A., Kramer L. D. (2013). Aedes taeniorhynchus vectorial capacity informs a pre-emptive Assessment of West Nile Virus establishment in Galápagos. Sci. Rep. 3, 1519. doi: 10.1038/srep01519
Eastwood G., Goodman S. J., Hilgert N., Cruz M., Kramer L. D., Cunningham A. A. (2014). Using avian surveillance in Ecuador to assess the imminence of West Nile virus incursion to Galápagos. Ecohealth 11, 53–62. doi: 10.1007/s10393-014-0911-5
Eastwood G., Kramer L. D., Goodman S. J., Cunningham A. A. (2011). West Nile virus vector competency of Culex quinquefasciatus mosquitoes in the Galápagos Islands. Am. J. Trop. Med. Hyg. 85, 426–433. doi: 10.4269/ajtmh.2011.10-0739
Echeverría G., Reyna-Bello A., Minda-Aluisa E., Celi-Erazo M., Olmedo L., García H. A., et al. (2019). Serological evidence of Coxiella burnetii infection in cattle and farm workers: is Q fever an underreported zoonotic disease in Ecuador? Infect. Drug Resist. 12, 701–706. doi: 10.2147/IDR.S195940
El-Mahallawy H., Lu G., Kelly P., Xu D., Li Y., Fan W., et al. (2015). Q fever in China: A systematic review 1989–2013. Epidemiol. Infection 143, 673–681. doi: 10.1017/S0950268814002593
Epelboin L., De Souza Ribeiro Mioni M., Couesnon A., Saout M., Guilloton E., Omar S., et al. (2023). Coxiella burnetii infection in livestock, pets, wildlife, and ticks in Latin America and the Caribbean: a comprehensive review of the literature. Curr. Trop. Med. Rep. 10, 94–137. doi: 10.1007/s40475-023-00288-7
Espinosa M., Cedeño M. (2022). Plan estratégico isntitucional de la Agencia de regulación y control del Bioseguridad y Cuarente de Galápagos 2019-2022 L. Available online at: https://www.fao.org/faolex/results/details/es/c/LEX-FAOC197011/ (Accessed 9 May, 2023).
Espinosa R., Tago D., Treich N. (2020). Infectious diseases and meat production. Environ. Resour Econ (Dordr) 76, 1019–1044. doi: 10.1007/s10640-020-00484-3
Evans T. S., Aung O., Cords O., Coffey L. L., Wong T., Weiss C. M., et al. (2022). Sylvatic transmission of Chikungunya Virus among Nonhuman Primates in Myanmar. Emerg. Infect. Dis. 28, 2548–2551. doi: 10.3201/eid2812.220893
Fagre A. C., Kading R. C. (2019). Can bats serve as reservoirs for arboviruses? Viruses 11, 215. doi: 10.3390/v11030215
Fauci A. S. (2006). Emerging and re-emerging infectious diseases: influenza as a prototype of the host-pathogen balancing act. Cell 124, 665–670. doi: 10.1016/j.cell.2006.02.010
Fenton H., Daoust P. Y., Forzán M. J., Vanderstichel R. V., Ford J. K. B., Spaven L., et al. (2017). Causes of mortality of harbor porpoises Phocoena phocoena along the Atlantic and Pacific coasts of Canada. Dis. Aquat Organ 122, 171–183. doi: 10.3354/dao03080
Fessl B., Heimpel G. E., Causton C. E. (2018). “Chapter 9: Invasion of an Avian Nest Parasite, Philornis downsi, to the Galápagos Islands: Colonization History, Adaptations to Novel Ecosystems, and Conservation Challenges,” in Disease Ecology, Social and Ecological Interactions in the Galápagos Islands. Ed. Parker P. G. (Springer). doi: 10.1007/978-3-319-65909-1_9
Finet Y. (1994). Marine Molluscs of the Galapagos. Gastropods - A monograph and revision of the families Haliotidae, Scissurellidae, Fissurellidae and Lottiidae Vol. 1 (Ancona: L’Informatore Piceno), 110.
Fisher S., Fisher R. N., Pauly G. B. (2022). Hidden in plain sight: detecting invasive species when they are morphologically similar to native species. Front. Conserv. Sci. 3. doi: 10.3389/fcosc.2022.846431
Food and Drug Administration. (2017). Veterinary feed directive. Available online at: https://www.fda.gov/animal-veterinary/development-approval-process/veterinary-feed-directive-vfd (Accessed 9 May, 2023).
Forshaw D., Phelps G. R. (1991). Tuberculosis in a captive colony of pinnipeds. J. Wildl Dis. 27, 288–295. doi: 10.7589/0090-3558-27.2.288
Foucaud J., Orivel J., Fournier D., Delabie J. H. C., Loiseau A., Le Breton J., et al. (2009). Reproductive system, social organization, human disturbance and ecological dominance in native populations of the little fire ant, Wasmannia auropunctata. Mol. Ecol. 18, 5059–5073. doi: 10.1111/j.1365-294X.2009.04440.x
Frazier J. (2021). “The Galapagos: Island Home of Giant Tortoises,” in Galapagos Giant Tortoises. Eds. Gibbs J., Cayot L., Washington T. A. (Cambridge: Academic Press), 3–21. doi: 10.1016/B978-0-12-817554-5.00004-6
Freitas L. F. D., Carvajal-Barriga E. J., Barahona P. P., Lachance M. A., Rosa C. A. (2013). Kodamaea transpacifica f.a., sp. nov., a yeast species isolated from ephemeral flowers and insects in the Galápagos Islands and Malaysia: further evidence for ancient human transpacific contacts. Int. J. Syst. Evol. Microbiol. 63, 4324–4329. doi: 10.1099/ijs.0.052282-0
Galápagos Conservancy. (2014). Annual report. Available online at: https://www.galapagos.org/wp-content/uploads/2022/08/2014.pdf (Accessed 9 August 2024).
Galapagos Conservancy. (2022). Developing a biocontrol agent to eradicate the invasive Asian blackberry. Available online at: https://www.galapagos.org/projects/rewilding-galapagos/developing-a-biocontrol-agent-to-eradicate-the-invasive-asian-blackberry/ (Accessed 2 August 2024).
Galapagos Conservancy. (2023). Alliance against invasion: tackling the ant threat in Galapagos. Available online at: https://www.galapagos.org/newsroom/tackling-the-ant-threat-in-galapagos/ (Accessed 10 August 2024).
Galapagos Conservation Trust. (2023a). Restoring Floreana. Available online at: https://galapagosconservation.org.uk/our-work/projects/restoring-floreana/ (Accessed 3 August 2024).
Galapagos Conservation Trust. (2023b). 12 missing species set to return to Floreana. Available online at: https://galapagosconservation.org.uk/12-missing-species-set-to-return-to-floreana/ (Accessed 3 August 2024).
Gardener M. R., Atkinson R., Renteria J. L. (2010). Eradications and people: lessons from the plant eradication program in Galapagos. Restor. Ecol. 18, 20–29. doi: 10.1111/j.1526-100X.2009.00614.x
Gardener M. R., Trueman M., Buddenhagen C., Heleno R., Jäger H., Atkinson R., et al. (2013). “A Pragmatic Approach to the Management of Plant Invasions in Galapagos,” in Plant Invasions in Protected Areas. Invading Nature - Springer Series in Invasion Ecology, vol. 7 . Eds. Foxcroft L., Pyšek P., Richardson D., Genovesi P. (Springer, Dordrecht). doi: 10.1007/978-94-007-7750-7_16
Gardner B. R., Stenos J., Hufschmid J., Arnould J. P. Y., McIntosh R. R., Tadepalli M., et al. (2022). An Old Pathogen in a New Environment–Implications of Coxiella burnetii in Australian Fur Seals (Arctocephalus pusillus doriferus). Front. Mar. Sci. 9, 809075. doi: 10.3389/fmars.2022.809075
Garzon-Chavez D., Garcia-Bereguiain M. A., Mora-Pinargote C., Granda-Pardo J. C., Leon-Benitez M., Franco-Sotomayor G., et al. (2020). Population structure and genetic diversity of Mycobacterium tuberculosis in Ecuador. Sci. Rep. 10, 6237. doi: 10.1038/s41598-020-62824-z
Georgiev M., Afonso A., Neubauer H., Needham H., Thiery R., Rodolakis A., et al. (2013). Q fever in humans and farm animals in four European countries 1982 to 2010. Euro Surveill 18, 20407. doi: 10.2807/ese.18.08.20407-en
Gifford E. W. (1908). The rehabilitation of the California Academy of Sciences. Condor. 10, 95–96. doi: 10.2307/1361021
Gilbert M., Sulikhan N., Uphyrkina O., Goncharuk M., Kerley L., Castro E. H., et al. (2020). Distemper, extinction, and vaccination of the Amur tiger. Proc. Natl. Acad. Sci. U.S.A. 117, 31954–31962. doi: 10.1073/pnas.2000153117
Gingrich E. N., Scorza A. V., Clifford E. L., Olea-Popelka F. J., Lappin M. R. (2010). Intestinal parasites of dogs on the Galápagos Islands. Vet. Parasitol. 169, 404–407. doi: 10.1016/j.vetpar.2010.01.018
Gioia G., Vinueza R. L., Cruz M., Jay M., Corde Y., Marsot M., et al. (2018). Estimating the probability of freedom from bovine brucellosis in the Galápagos Islands. Epidemiol. Infect. 147, e9. doi: 10.1017/S0950268818002534
Go Y. Y., Balasuriya U. B., Lee C. K. (2014). Zoonotic encephalitides caused by arboviruses: transmission and epidemiology of alphaviruses and flaviviruses. Clin. Exp. Vaccine Res. 3, 58–77. doi: 10.7774/cevr.2014.3.1.58
Gobierno del Ecuador. (2022a). Instituto Nacional de Investigación en Salud Pública-INSPI-Dr. Leopoldo Izquieta Pérez: Misión-Visión-Objetivos. Available online at: http://www.investigacionsalud.gob.ec/mision-vision-objetivos/ (Accessed 9 May, 2023).
Gobierno del Ecuador. (2022b). AGROCALIDAD: Misión y visión. Available online at: https://www.agrocalidad.gob.ec/mision-vision/ (Accessed 9 May, 2023).
Gobierno del Ecuador. (2022c). ARCSA: Misivón,visión y valores. Available online at: https://www.controlsanitario.gob.ec/valores/ (Accessed 9 May, 2023).
Gobierno del Ecuador. (2022d). ABG: vigilancia sanitaria. Available online at: https://bioseguridadGalapagos.gob.ec/vigilancia-zoosanitaria/ (Accessed 9 May, 2023).
Gobierno del Ecuador. (2023). Comunicado Oficial: Activación de protocolos ante possible afectación de aves en Galápagos (Parque Nacional Galápagos: Ministerio del Ambiente, Agua y Transición Ecológica) (Accessed 18 September, 2023).
Goldstein T., Colegrove K. M., Hanson M., Gulland F. M. (2011). Isolation of a novel adenovirus from California sea lions Zalophus californianus. Dis. Aquat Organ 94, 243–248. doi: 10.3354/dao02321
Goldstein T., Mazet J. A., Gill V. A., Doroff A. M., Burek K. A., Hammond J. A. (2009). Phocine distemper virus in Northern sea otters in the Pacific Ocean, 690 Alaska, USA. Emerg. Infect. Dis. 15, 925–927. doi: 10.3201/eid1506.090056
Gottdenker N. L., Walsh T., Vargas H., Merkel J., Jiménez G. U., Miller R. E., et al. (2005). Assessing the risks of introduced chickens and their pathogens to native birds in the Galápagos Archipelago. Biol. Conserv. 126, 429–439. doi: 10.1016/j.biocon.2005.06.025
Grant P., Grant B., Petren K., Keller L. (2005). Extinction behind our backs: the possible fate of one of the Darwin’s finch species on Isla Floreana, Galápagos. Biol. Conserv. 122, 499–503. doi: 10.1016/j.biocon.2004.09.001
Gregory T. M., Livingston I., Hawkins E. C., Loyola A., Cave A., Vaden S. L., et al. (2023). Dirofilaria immitis identified in Galápagos sea lions (Zalophus wollebaeki): a wildlife health and conservation concern. J. Wildl Dis. 59, 487–494. doi: 10.7589/JWD-D-22-00119
Greig D. J., Gulland F. M., Kreuder C. (2005). A decade of live California sea lion (Zalophus californianus) strandings along the central California coast: Causes and trends 1999–2000. Aquat Mamm 31, 11–22. doi: 10.1578/AM.31.1.2005.11
Guamán-Burneo M. C., Dussán K. J., Cadete R. M., Cheab M. A. M., Portero P., Carvajal-Barriga E. J., et al. (2015). Xylitol production by yeasts isolated from rotting wood in the Galápagos Islands, Ecuador, and description of Cyberlindnera galapagoensis f.a., sp. nov. Antonie Van Leeuwenhoek 108, 919–931. doi: 10.1007/s10482-015-0546-8
Guerrero E., Mejia M., Azuero R., Duque V., Loaiza J., et al. (2019). Measures to reduce the risk of ant invasions on Galapagos. Available online at: https://reports.galapagos.org/english/2019/6/29/measures-to-reduce-the-risk-of-invasive-ants-entering-galapagos/ (Accessed 10 August 2024).
Guerrero-Latorre L., Ballesteros I., Villacrés-Granda I., Granda M. G., Freire-Paspuel B., Ríos-Touma B. (2020). SARS-CoV-2 in river water: Implications in low sanitation countries. Sci. Total Environ. 743, 140832. doi: 10.1016/j.scitotenv.2020.140832
Guertin S. (2019). Examining the impacts of disease on wildlife conservation and management. US fish and wildlife service. Available online at: https://www.fws.gov/testimony/examining-impacts-disease-wildlife-conservation-and-management (Accessed 3 August 2024).
Guézou A., Trueman M., Buddenhagen C. E., Chamorro S., Guerrero A. M., Pozo P., et al. (2010). An extensive alien plant inventory from the inhabited areas of Galápagos. PloS One 5, 1–8. doi: 10.1371/journal.pone.0010276
Gulland F. M. D., Hall A. J., Ylitalo G. M., Colegrove K. M., Norris T., Duignan P. J., et al. (2020). Persistent contaminants and Herpesvirus OtHV1 are positively associated with Cancer in wild California Sea Lions (Zalophus californianus). Front. Mar. Sci. 7. doi: 10.3389/fmars.2020.602565
Guo K., Pyšek P., van Kleunen M., Kinlock N. L., Lučanová M., Leitch I. J., et al. (2024). Plant invasion and naturalization are influenced by genome size, ecology and economic use globally. Nat. Commun. 15, 1330. doi: 10.1038/s41467-024-45667-4
Gutierrez-Bugallo G., Piedra L. A., Rodriguez M., Bisset J. A., Lourenco-de-Oliveira R., Weaver S. C., et al. (2019). Vector-borne transmission and evolution of Zika virus. Nat. Ecol. Evolution 3, 561–569. doi: 10.1038/s41559-019-0836-z
Gwee S. X. W., St. John A. L., Gray G. C., Pang J. (2021). Animals as potential reservoirs for dengue transmission: A systematic review. One Health 12, 100216. doi: 10.1016/j.onehlt.2021.100216
Hadfield T. L., Turell M., Dempsey M. P., David J., Park E. J. (2001). Detection of West Nile virus in mosquitoes by RT-PCR. Mol. Cell Probes 15, 147–150. doi: 10.1006/mcpr.2001.0353
Härkönen T., Dietz R., Reijnders P., Teilmann J., Harding K., Hall A., et al. (2006). The 1988 and 2002 phocine distemper virus epidemics in European harbour seals. Dis. Aquat Organ 68, 115–130. doi: 10.3354/dao068115
Harvey-Samuel T., Ant T., Sutton J., Niebuhr C. N., Asigau S., Parker P., et al. (2021). Culex quinquefasciatus: status as a threat to island avifauna and options for genetic control. CABI Agric. Biosci. 2, 9. doi: 10.1186/s43170-021-00030-1
Haulena M., Gulland F. M., Lawrence J. A., Fauquier D. A., Jang S., Aldridge B., et al. (2006). Lesions associated with a novel Mycoplasma sp. in California sea lions (Zalophus californianus) undergoing rehabilitation. J. Wildl Dis. 42, 40–45. doi: 10.7589/0090-3558-42.1.40
Haydon D. T., Cleaveland S., Taylor L. H., Laurenson M. K. (2002). Identifying reservoirs of infection: a conceptual and practical challenge. Emerging Infect. Diseases 8, 1468–1473. doi: 10.3201/eid0812.010317
Hendrix C., Brunner C., Bellamy L. (1986). Natural transmission of Dirofilaria immitis by Aedes aegypti. J. Am. Mosq. Control. Assoc. 2, 48–51.
Heraty J. M. (1994). “Biology and Importance of Two Eucharitid Parasites of Wasmannia and Solenopsis,” in Exotic Ants: Biology, Impact, and Control of Introduced Species. Ed. Williams D. F. (CRC Press, Boca Raton), 104–120. doi: 10.1201/9780429040795-9
Heraty J. M., Rogers D. V., Johnson M. T., Perreira W. D., Baker A. J., Bitume E., et al. (2021). “New record in the Hawaiian Islands of Orasema minutissima (Hymenoptera: Eucharitidae), an ant-parasitic wasp and a potential biocontrol agent against the Little Fire Ant, Wasmannia auropunctata (Hymenoptera: Formicidae),” in Evenhuis N. L. Ed. Records of the Hawaii Biological Survey for 2020. Bishop Museum Occasional Papers. (State Museum of Cultural and Natural History, Honolulu, Hawaii), vol. 137, 7–18.
Herbert A. (2014). Caracterización Molecular, Morfológica y Ultra Estructural Del Nematodo Hematófago Uncinaria Sp. en el Lobo Marino de Galápagos. Univ. Queretaro, Queretaro, Mexico, 71.
Hernandez J. A., Yoak A. J., Walden H. S., Thompson N., Zuniga D., Criollo R., et al. (2020). Dog overpopulation on Santa Cruz Island, Galápagos 2018. Conserv. Sci. Pract. 2 (6), e201. doi: 10.1111/csp2.v2.6
Herrera H. W., Causton C. E. (2008). Distribution of fire ants solenopsis geminata and wasmannia auropunctata (Hymenoptera: Formicidae) in the Galápagos islands. Galapagos Res. 65, 11–14.
Hickman C. P. (2009). Evolutionary responses of marine invertebrates to insular isolation in Galapagos. Galapagos Res. 66, 32–42.
Holway D. A., Lach L., Suarez A. V., Tsutsui N. D., Case T. J. (2002). The causes and consequences of ant invasions. Annu. Rev. Ecol. Systematics 33, 33181–33233. doi: 10.1146/annurev.ecolsys.33.010802.150444
Huckabone S. E., Gulland F. M., Johnson S. M., Colegrove K. M., Dodd E. M., Pappagianis D., et al. (2015). Coccidioidomycosis and other systemic mycoses of marine mammals stranding along the central California, USA coast: 1998-2012. J. Wildl. Dis. 51, 295–308. doi: 10.7589/2014-06-143
Iannone B. V., Carnevale S., Main M. B., Hill J. E., McConnell J. B. (2020). Invasive species terminology: standardizing for stakeholder education. J. Extension 58. doi: 10.34068/joe.58.03.27
International Union for Conservation of Nature (2022). The IUCN Red List of Threatened Species. Version 2022-2. Available online at: https://www.iucnredlist.org (Accessed 8 May, 2023).
Jäger H., San-José M., Peabody C., Chango R., Sevilla C. (2024). Restoring the threatened Scalesia forest: insights from a decade of invasive plant management in Galapagos. Front. Forests Global Change 7. doi: 10.3389/ffgc.2024.1350498
James S. A., Carvajal-Barriga E. J., Barahona P. P., Nueno-Palop C., Cross K., Bond C. J., et al. (2015). Kazachstania yasuniensis sp. nov., an ascomycetous yeast species found in mainland Ecuador and on the Galápagos. Int. J. Syst. Evol. Microbiol. 65, 1304–1309. doi: 10.1099/ijs.0.000102
Jensen E. L., Gaughran S. J., Fusco N. A., Poulakakis N., Tapia W., Sevilla C., et al. (2022). The Galapagos giant tortoise Chelonoidis phantasticus is not extinct. Commun. Biol. 5, 546. doi: 10.1038/s42003-022-03483-w
Jimenez I. A., Vega Mariño P. A., Stapleton G. S., Prieto J. B., Bowman D. D. (2020). Canine vector-borne disease in domestic dogs on Isla Santa Cruz, Galápagos. Vet. Parasitol. Reg. Stud. Rep. 19, 100373. doi: 10.1016/j.vprsr.2020.100373
Jiménez-Uzcátegui G., Milstead B., Márquez C., Zabala J., Buitrón P., Llerena A., et al. (2007). Galapagos vertebrates: endangered status and conservation actions. Galapagos Report 2006–2007 (Puerto Ayora: Charles Darwin Foundation), 104–110.
Johnstone C., Baez J. C. (2021). Placing the COVID-19 pandemic in a marine ecological context: potential risks for conservation of marine air-breathing animals and future zoonotic outbreaks. Front. Mar. Sci. 8. doi: 10.3389/fmars.2021.691682
Jourdan H. (1997). Threats on Pacific Island: the spread of the Tramp Ant Wasmannia auropunctata. Pacific Conserv. Biol. 3, 61–64. doi: 10.1071/PC970061
Jourdan H., Bourguet E., Mille C., Gula R., Theuerkauf J. (2022). Impact of invasive little fire ants Wasmannia auropunctata on rainforest soil fauna: implications for conservation of the endangered flightless kagu of New Caledonia. Biol. Invasions 24, 3675–3680. doi: 10.1007/s10530-022-02882-8
Jourdan H., Sadlier R. A., Bauer A. M. (2001). Little fire ant invasion (Wasmannia auropunctata) as a threat to New Caledonian lizards: evidences from a sclerophyll forest (Hymenoptera: formicidae). Sociobiology 38, 283–301.
Kagawa F. T., Wehner J. H., Mohindra V. (2003). Q fever as a biological weapon. Semin. Respir. Infect. 18, 183–195.
Kaplan B. S., Webby R. J. (2013). The avian and mammalian host range of highly pathogenic avian H5N1 influenza. Virus Res. 178, 3–11. doi: 10.1016/j.virusres.2013.09.004
Kappes A., Tozooneyi T., Shakil G., Railey A. F., McIntyre K. M., Mayberry D. E., et al. (2023). Livestock health and disease economics: a scoping review of selected literature. Front. Vet. Sci. 10. doi: 10.3389/fvets.2023.1168649
Kastdalen A. (1982). Changes in the biology of santa cruz island between 1935 and 1965. Notic. Galápagos. 35, 7–12.
Katz H., Schelotto F., Bakker D., Castro-Ramos M., Gutiérrez-Expósito D., Panzera Y., et al. (2022). Survey of selected pathogens in free-ranging pinnipeds in Uruguay. Dis. Aquat Organ 150, 69–83. doi: 10.3354/dao03676
Keith I., Bensted-Smith W., Banks S., Suarez J., Riegl B. (2022). Caulerpa chemnitzia in Darwin threatening Galápagos coral reefs. PloS One 17, e0272581. doi: 10.1371/journal.pone.0272581
Keith I., Dawson T. P., Collins K. J., Campbell M. L. (2016). Marine invasive species: establishing pathways, their presence and potential threats in the Galápagos Marine Reserve. Pacific Conserv. Biol. 22, 377–385. doi: 10.1071/PC15020
Kennedy J. M., Earle J. A. P., Omar S., Abdullah H., Nielsen O., Roelke-Parker M. E., et al. (2019). Canine and phocine distemper viruses: global spread and genetic basis of jumping species barriers. Viruses 11, 944. doi: 10.3390/v11100944
Key G., Sangoquiza M. (2008). Activity patterns and distribution of Galápagos bats. Galápagos Res. 65, 20–24. Available at: http://hdl.handle.net/1834/35978.
Kilpatrick A. M., Daszak P., Goodman S. J., Rogg H., Kramer L. D., Cedeño V., et al. (2006). Predicting pathogen introduction: West Nile virus spread to Galápagos. Conserv. Biol. 20, 1224–1231. doi: 10.1111/j.1523-1739.2006.00423.x
Kim S. G., Kim E. H., Lafferty C. J., Dubovi E. (2005). Coxiella burnetii in bulk tank milk samples, United States. Emerg. Infect. Dis. 11, 619–621. doi: 10.3201/eid1104.041036
Kleindorfer S., Dudaniec R. Y. (2008). Increasing prevalence of avian poxvirus in Darwin’s finches and its effect on male pairing success. J. Avian Biol. 37 (1), 69–76. doi: j.0908-8857.2006.03503.x
Knutie S. A., McNew S. M., Bartlow A. W., Vargas D. A., Clayton D. H. (2014). Darwin’s finches combat introduced nest parasites with fumigated cotton. Curr. Biol. 24, R355–R356. doi: 10.1016/j.cub.2014.03.058
Kruuk H., Snell H. (1981). Prey selection by feral dogs from a population of marine iguanas (Amblyrhynchus cristatus). J. Appl. Ecol. 18, 197–204. doi: 10.2307/2402489
Lawesson J. E., Adsersen H., Bentley P. (1987). “An updated and annotated checklist of the vascular plants of the Galápagos islands,” in Reports from the botanical institute, university of aarhus, vol. 16. (Aarhus, Denmark: Aarhus University Press), 1–74.
Lean F. Z. X., Cox R., Madslien K., Spiro S., Nymo I. H., Bröjer C., et al. (2023). Tissue distribution of angiotensin-converting enzyme 2 (ACE2) receptor in wild animals with a focus on artiodactyls, mustelids and phocids. One Health 16, 100492. doi: 10.1016/j.onehlt.2023.100492
Leguia M., Garcia-Glaessner A., Muñoz-Saavedra B., Juarez D., Barrera P., Calvo-Mac C., et al. (2023). Highly pathogenic avian influenza A (H5N1) in marine mammals and seabirds in Peru. Nat. Commun. 14, 5489. doi: 10.1038/s41467-023-41182-0
Levin I. I., Outlaw D. C., Vargas F. H., Parker P. G. (2009). Plasmodium blood parasite found in endangered Galapagos penguins (Spheniscus mendiculus). Biol. Conserv. 142, 3191–3195. doi: 10.1016/j.biocon.2009.06.017
Levin I. I., Zwiers P., Deem S. L., Geest E. A., Higashiguchi J. M., Iezhova T. A., et al. (2013). Multiple lineages of Avian malaria parasites (Plasmodium) in the Galápagos Islands and evidence for arrival via migratory birds. Conserv. Biol. 27, 1366–1377. doi: 10.1111/cobi.12127
Levy J. K., Crawford P. C., Lappin M. R., Dubovi E. J., Levy M. G., Alleman R., et al. (2008). Infectious diseases of dogs and cats on Isabela Island, Galápagos. J. Vet. Intern. Med. 22, 60–65. doi: 10.1111/j.1939-1676.2007.0034.x
Ley D. H., Hawley D. M., Geary S. J., Dhondt A. A. (2016). House finch (Haemorhous mexicanus) conjunctivitis, Mycoplasma spp. Isolated from North American wild birds 1994-2015. J. Wildlife Dis. 52, 669–673. doi: 10.7589/2015-09-244
Lloyd-Smith J. O., Greig D. J., Hietala S., Ghneim G. S., Palmer L., St. Leger J., et al. (2007). Cyclical changes in seroprevalence of leptospirosis in California sea lions: endemic and epidemic disease in one host species? BMC Infect. Dis. 7, 125. doi: 10.1186/1471-2334-7-125
Luan J., Jin X., Lu Y., Zhang L. (2020). SARS-CoV-2 spike protein favors ACE2 from Bovidae and Cricetidae. J. Med. Virol. 92, 1649–1656. doi: 10.1002/jmv.25817
Lubin Y. D. (1984). Changes in the native fauna of the Galápagos Islands following invasion by the little red fire ant, Wasmannia auropunctata. Biol. J. Linn. Society 21, 229–242. doi: 10.1111/j.1095-8312.1984.tb02064.x
Lynton-Jenkins J. G., Russell A. F., Chaves J., Bonneaud C. (2021). Avian disease surveillance on the island of San Cristóbal, Galápagos. Ecol. Evolution 11, 18422–18433. doi: 10.1002/ece3.8431
MacClancy J., Fuentes A. (Eds.) (2013). Ethics in the field: contemporary challenges. 1st ed (Berghahn Books). Available at: http://www.jstor.org/stable/j.ctt9qcqc8.
Macedo R., Isidro J., Gomes M. C., Botelho A., Albuquerque T., Sogorb A., et al. (2020). Animal-to-human transmission of Mycobacterium pinnipedii. Eur. Respir. J. 56, 2000371. doi: 10.1183/13993003.00371-2020
MacFarland C. G., Villa J., Toro B. (1974). The Galápagos giant tortoises (Geochelone elephantopus) part I: status of the surviving populations. Biol. Conserv. 6, 118–133. doi: 10.1016/0006-3207(74)90024-X
Machalaba C. C., Loh E. H., Daszak P., Karesh W. B. (2015). Emerging diseases from animals. State World 2015, 105–116. doi: 10.5822/978-1-61091-611-0_8
Mackenzie J. S., Childs J. E., Field H. E., Wang L. F., Breed A. C. (2016). The role of bats as reservoir hosts of emerging neuroviruses. Neurotropic Viral Infections 8, 403–454. doi: 10.1007/978-3-319-33189-8_12
Marquez C., Wiedenfeld D., Snell H., Fritts T., Belen M. F., MacFarland C., et al. (2004). Estado actual de las poblaciones de tortugas terrestres gigantes (Geochelone spp., Chelonia: Testudinae) en las islas Galápagos. ‘Current status of the populations of giant land tortoises (Geochelone spp., Chelonya: Testudinae) in the Galápagos islands’. Ecologia Aplicada 3 (1,2), 98–111. Available at: http://www.scielo.org.pe/scielo.php?script=sci_arttext&pid=S1726-22162004000100014.
Martinez-Gutierrez M., Ruiz-Saenz J. (2016). Diversity of susceptible hosts in canine distemper virus infection: a systematic review and data synthesis. BMC Vet. Res. 12, 78. doi: 10.1186/s12917-016-0702-z
Mathavarajah S., Stoddart A. K., Gagnon G. A., Dellaire G. (2021). Pandemic danger to the deep: The risk of marine mammals contracting SARS-CoV-2 from wastewater. Sci. Total Environment. 760, 143346. doi: 10.1016/j.scitotenv.2020.143346
McCracken G. F., Hayes J. P., Cevallos J., Guffey S. Z., Carlos Romero F. (1997). Observations on the distribution, ecology, and behaviour of bats on the Galápagos Islands. J. Zool. 243, 757–770. doi: 10.1111/j.1469-7998.1997.tb01974.x
McDaniel C. J., Cardwell D. M., Moeller R. B. Jr, Gray G. C. (2014). Humans and cattle: a review of bovine zoonoses. Vector Borne Zoonotic Dis. 14, 1–19. doi: 10.1089/vbz.2012.1164
McLean R. G. (2006). West nile virus in North American birds. Ornithological Monogr. 60, 44–64. doi: 10.2307/40166827
McNew S. M., Loyola D. C., Yepez J., Andreadis C., Gotanda K., Saulsberry A., et al. (2022). Transcriptomic responses of Galápagos finches to avian poxvirus infection. Mol. Ecol. 31, 5552–5567. doi: 10.1111/mec.16690
Mee P. T., Wong S., Brown K., Lynch S. E. (2021). Quantitative PCR assay for the detection of Aedes vigilax on mosquito trap collections containing large numbers of morphologically similar species and phylogenetic analysis of specimens collected in Victoria, Australia. Parasites Vectors 14. doi: 10.1186/s13071-021-04923-y
Michael S. A., Howe L., Chilvers B. L., Morel P., Roe W. D. (2016). Seroprevalence of Toxoplasma gondii in mainland and sub-Antarctic New Zealand sea lion (Phocarctos hookeri) populations. N Z Vet. J. 64, 293–297. doi: 10.1080/00480169.2016.1191974
Migaki G., Allen J. F., Casey H. W. (1977). Toxoplasmosis in a California sea lion (Zalophus californianus). Am. J. Vet. Res. 38, 135–136.
Miguel E., Grosbois V., Caron A., Pople D., Roche B., Donnelly C. A. (2020). A systemic approach to assess the potential and risks of wildlife culling for infectious disease control. Commun. Biol. 3, 353. doi: 10.1038/s42003-020-1032-z
Miller G. D., Hofkin B. V., Snell H., Hahn A., Miller R. D. (2001). Avian malaria and Marek’s disease: potential threats to Galápagos penguins Spheniscus mendiculus. Mar. Ornithol. 29, 43–46.
Minasny B., Fiantis D., Mulyanto B., Sulaeman Y., Widyatmanti W. (2020). Global soil science research collaboration in the 21st century: Time to end helicopter research. Geoderma 373, 114299. doi: 10.1016/j.geoderma.2020.114299
Morick D., Osinga N., Gruys E., Harrus S. (2009). Identification of a Bartonella species in the harbor seal (Phoca vitulina) and in seal lice (Echinophtirius horridus). Vector Borne Zoonotic Dis. 9, 751–753. doi: 10.1089/vbz.2008.0202
Mosquera J. D., Valle C. A., Nieto-Claudin A., Fessl B., Lewbart G. A., Deresienski D., et al. (2023). Prevalence of Toxoplasma gondii in Galapagos birds: Inference of risk factors associated with diet. PloS One 18, e0287403. doi: 10.1371/journal.pone.0287403
National Invasive Species Information Center (1999). Executive order 13112 – invasive species (US Department of Agriculture). Available online at: https://www.invasivespeciesinfo.gov/executive-order-13112#details–section-1-definitions (Accessed 25 Mar 2024).
Nelder M. P., McCreadie J. W., Coscarón C., Brockhouse C. L. (2004). First report of a trichomycete fungus (Zygomycota: Trichomycetes) inhabiting larvae of Simulium ochraceum sensu lato Walker (Diptera: Simuliidae) from the Galápagos Islands. J. Invertebr. Pathol. 87, 39–44. doi: 10.1016/j.jip.2004.06.005
Nicholls H. (2021). “Human perceptions of Galápagos tortoises through history,” in Galápagos giant tortoises. biodiversity of world: Conservation from genes to landscapes. Eds. Gibbs J. P., Cayot L. J., Tapia W. A. (Cambridge: Academic Press), 49–62. doi: 10.1016/b978-0-12-817554-5.00007-1
Nieto-Claudin A., Deem S. L., Rodríguez C., Cano S., Moity N., Cabrera F., et al. (2021). Antimicrobial resistance in Galápagos tortoises as an indicator of the growing human footprint. Environ. pollut. 284, 117453. doi: 10.1016/j.envpol.2021.117453
Nishida G. M., Evenhuis N. L. (2000). “Arthropod pests of conservation significance in the Pacific: a preliminary assessment of selected groups,” in Invasive species in the Pacific: a technical review and draft regional strategy. Ed. Sherley G. (South Pacific Regional Environment Programme, Samoa), 115D142.
Nnadi N. E., Carter D. A. (2021). Climate change and the emergence of fungal pathogens. PloS Pathog. 17, e1009503. doi: 10.1371/journal.ppat.1009503
O’Connor J., Sulloway F., Kleindorfer S. (2010). Avian population survey in the Floreana highlands: is Darwin’s Medium Tree Finch declining in remnant patches of Scalesia forest? Bird Conserv. Int. 20, 1–11. doi: 10.1017/S0959270910000195
One Health High-Level Expert Panel (OHHLEP), Adisasmito W. B., Almuhairi S., Behravesh C. B., Bilivogui P., Bukachi S. A., et al. (2022). One Health: A new definition for a sustainable and healthy future. PloS Pathogens 18, e1010537. doi: 10.1371/journal.ppat.1010537
Oude Munnink B. B., Sikkema R. S., Nieuwenhuijse D. F., Molenaar R. J., Munger E., Molenkamp R., et al. (2021). Transmission of SARS-CoV-2 on mink farms between humans and mink and back to humans. Science 371, 172–177. doi: 10.1126/science.abe5901
Overbey K. N., Hatcher S. M., Stewart J. R. (2015). Water quality and antibiotic resistance at beaches of the Galápagos Islands. Front. Environ. Sci. 3. doi: 10.3389/fenvs.2015.00064
Padilla L. R., Huyvaert K. P., Merkel J., Miller R. E., Parker P. G. (2003). Hematology, plasma chemistry, serology, and Chlamydophila status of the waved albatross (Phoebastria irrorata) on the Galápagos Islands. J. Zoo Wildl Med. 34, 278–283. doi: 10.1638/02-076
Padilla L. R., Santiago-Alarcon D., Merkel J., Miller R. E., Parker P. G. (2004). Survey for Haemoproteus spp., Trichomonas gallinae, Chlamydophila psittaci, and Salmonella spp. in Galápagos Islands columbiformes. J. Zoo Wildl Med. 35, 60–64. doi: 10.1638/03-029
Palmer J. L., McCutchan T. F., Vargas F. H., Deem S. L., Cruz M., Hartman D. A., et al. (2013). Seroprevalence of malarial antibodies in Galápagos penguins (Spheniscus mendiculus). J. Parasitol. 99, 770–776. doi: 10.1645/12-57.1
Parent C. E., Caccone A., Petren K. (2008). Colonization and diversification of Galápagos terrestrial fauna: a phylogenetic and biogeographical synthesis. Philos. Trans. R. Soc. London Ser. B Biol. Sci. 363, 3347–3361. doi: 10.1098/rstb.2008.0118
Parker P. G., Buckles E. L., Farrington H., Petren K., Whiteman N. K., Ricklefs R. E., et al. (2011). 110 years of avipoxvirus in the Galápagos islands. PloS One 6, e15989. doi: 10.1371/journal.pone.0015989
Pawson S. M., Sullivan J. J., Grant A. (2020). Expanding general surveillance of invasive species by integrating citizens as both observers and identifiers. J. Pest Sci. 93, 1155–1166. doi: 10.1007/s10340-020-01259-x
Peck S. B., Heraty J., Landry B., Sinclair B. J. (1998). Introduced insect fauna of an oceanic archipelago: The Galápagos islands, ecuador. Am. Entomol. 44, 218–237. doi: 10.1093/ae/44.4.218
Phillips R. B., Cooke B. D., Campbell K., Carrion V., Marquez C., Snell H. L. (2012b). Eradicating feral cats to protect Galápagos land iguanas: methods and strategies. Pacific Conserv. Biol. 11, 257–267. doi: 10.1016/j.biocon.2012.01.013
Phillips R. B., Cooke B. D., Carrión V., Snell H. L. (2012a). Eradication of rock pigeons, Columba livia, from the Galápagos Islands. Biol. Conserv. 147, 264–269. doi: 10.1016/j.biocon.2012.01.013
Pizzitutti F., Walsh S. J., Rindfuss R. R., Gunter R., Quiroga D., Tippett R., et al. (2016). Scenario planning for tourism management: a participatory and system dynamics model applied to the Galápagos Islands of Ecuador. J. Sustain. Tourism 25, 1117–1137. doi: 10.1080/09669582.2016.1257011
Planetary Health Alliance. (2021). Planetary health. Available online at: https://www.planetaryhealthalliance.org/planetary-health (Accessed 2 August 2024).
Pollard K., Kurose D., Buddie A., Ellison C. (2019). The prospects for biological control of Rubus niveus in the Galapagos Islands (CABI). Available online at: https://www.cabi.org/wp-content/uploads/invasive%20blackberry/Poster.pdf (Accessed 10 August 2024).
Primicias R. (2023). Evalúan la salud de pingüinos y piqueros en Galápagos, tras caso de gripe aviar [Evaluating the health of penguins and boobies in the Galápagos after a case of avian flu]. Available online at: https://www.primicias.ec/noticias/sociedad/evaluan-salud-pinguinos-piqueros-galapagos/ (Accessed 10 August 2024).
Puente-Rodríguez D., Bos A. P. B., Koerkamp P. W. G. G. (2019). Rethinking livestock production systems on the Galápagos Islands: Organizing knowledge-practice interfaces through reflexive interactive design. Environ. Sci. Policy 101, 166–174. doi: 10.1016/j.envsci.2019.08.019
Puryear W., Sawatzki K., Hill N., Foss A., Stone J. J., Doughty L., et al. (2023). Highly pathogenic avian influenza A(H5N1) virus outbreak in New England seals, United States. Emerging Infect. Diseases 29, 786–791. doi: 10.3201/eid2904.221538
Rentería J. L., Atkinson R., Crespo C., Gardener M. R., Grosholz E. D. (2021). Challenges for the management of the invasive blackberry (Rubus niveus) in the restoration of the Scalesia forest in the Galapagos Islands. Invasive Plant Sci. Management 14, 20–28. doi: 10.1017/inp.2021.5
Rentería J. L., Ellison C. (2004). Potential biological control of Lantana camara in the Galápagos using the rust Puccinia lantanae. SIDA Contributions to Botany 21, 1009–1017.
Rentería J. L., Gardener M. R., Panetta F. D., Atkinson R., Crawley M. J. (2012). Possible impacts of the invasive plant Rubus niveus on the native vegetation of the Scalesia forest in the Galápagos Islands. PloS One 10), e48106. doi: 10.1371/journal.pone.0048106
Reponen S. E., Brown S. K., Barnett B. D., Sacks B. N. (2014). Genetic and morphometric evidence on a Galápagos Island exposes founder effects and diversification in the first-known (truly) feral western dog population. Mol. Ecol. 23, 269–283. doi: 10.1111/mec.2014.23.issue-2
Riegl B., Walentowitz A., Sevilla C., Chango R., Jäger H. (2023). Invasive blackberry outcompetes the endemic Galapagos tree daisy Scalesia pedunculata. Ecol. Applications 33, e2846. doi: 10.1002/eap.2846
Riofrío-Lazo M., Arreguín-Sánchez F., Páez-Rosas D. (2017). Population abundance of the endangered Galápagos sea lion Zalophus wollebaeki in the Southeastern Galápagos archipelago. PloS One 12, e0168829. doi: 10.1371/journal.pone.0168829
Rodriguez A. L., Ferro B. E., Varona M. X., Santafé M. (2004). Exposure to Leptospira in stray dogs in the city of Cali. Biomedica 24, 291–295.
Rogg H., Buddenhagen C., Causton C. (2005).Experiences and limitations with pest risk analysis in the Galápagos Islands. In: Identification of risks and management of invasive alien species using the IPPC framework. Proceedings of the workshop on invasive alien species and the International Plant Protection Convention, Braunschweig, Germany, 22-26 September 2003 (Rome, Italy: FAO). Available online at: https://www.fao.org/3/y5968e/y5968e0m.htm (Accessed 10 September, 2023).
Rojas M. I., Barragán V., Trueba G., Hornstra H., Pearson T., Keim P. (2013). Detección de Coxiella burnetii en leche de bovinos domésticos del Ecuador. Avances 5, B5–B9. doi: 10.18272/aci.v5i1.115
Roque-Albelo L., Causton C. (1999). El Niño and introduced insects in the Galápagos Islands: different dispersal strategies, similar effects. Noticias Galápagos 60, 30–36.
Rosenberg J. F., Haulena M., Hoang L. M., Morshed M., Zabek E., Raverty S. A. (2016). Cryptococcus gattii Type VGIIa Infection in Harbor Seals (Phoca vitulina) in British Columbia, Canada. J. Wildl Dis. 52, 677–681. doi: 10.7589/2015-11-299
Rosselli D., Wetterer J. K. (2017). Stings of the ant wasmannia auropunctata (Hymenoptera: formicidae) as cause of punctate corneal lesions in humans and other animals. J. Med. Entomol. 54, 1783–1785. doi: 10.1093/jme/tjx167
Rueda D., Campbell K. J., Fisher P., Cunninghame F., Ponder J. B. (2016). Biologically significant residual persistence of brodifacoum in reptiles following invasive rodent eradication, Galapagos islands, ecuador. Conserv. Evidence 13, 38.
Rueda D., Carrion V., Castaño P. A., Cunninghame F., Fisher P. M., Hagen E., et al. (2019). “Preventing extinctions: planning and undertaking invasive rodent eradication from Pinzón Island, Galápagos,” in Island invasives: scaling up to meet the challenge, Occasional Paper SSC no. 62. Eds. Veitch C. R., Clout M. N., Martin A. R., Russell J. C., West C. J. (IUCN, Gland, Switzerland), 51–56.
Ruiz-Saenz J., Barragan V., Grijalva-Rosero C. J., Diaz E. A., Páez-Rosas D. (2023). Seroconversion in Galápagos sea lions (Zalophus wollebaeki) confirms the presence of canine distemper virus in rookeries of San Cristóbal island. Anim. (Basel) 13, 3657. doi: 10.3390/ani13233657
Ryan S. J., Lippi C. A., Nightingale R., Hamerlinck G., Borbor-Cordova M. J., Cruz B. M., et al. (2019). Socio-ecological factors associated with dengue risk and Aedes aegypti presence in the Galápagos Islands, Ecuador. Int. J. Environ. Res. Public Health 16, 682. doi: 10.3390/ijerph16050682
Sano Y., Aoki M., Takahashi H., Miura M., Komatsu M., Abe Y., et al. (2005). The first record of Dirofilaria immitis infection in a Humboldt penguin, Spheniscus humboldti. J. Parasitol. 91, 1235–1237. doi: 10.1645/GE-3492-RN.1
Sardelis M. R., Turell M. J., Dohm D. J., O’Guinn M. L. (2001). Vector competence of selected North American Culex and Coquillettidia mosquitoes for West Nile virus. Emerg. Infect. Dis. 7, 1018D1022. doi: 10.3201/eid0706.010617
Sarzosa M. S., Duignan P., DeRango E. J., Field C., Ríos C., Sanchez S., et al. (2021). Occurrence of Mycoplasmas in Galápagos Sea Lions (Zalophus wollebaeki) and their Association with Other Respiratory Pathogens. J. Wildl Dis. 57, 623–627. doi: 10.7589/JWD-D-20-00081
Sawicka A., Durkalec M., Tomczyk G., Kursa O. (2020). Occurrence of Mycoplasma gallisepticum in wild birds: A systematic review and meta-analysis. PloS One 15, e0231545. doi: 10.1371/journal.pone.0231545
Sax D. F., Gaines S. D. (2008). Colloquium paper: species invasions and extinction: the future of native biodiversity on islands. Proc. Natl. Acad. Sci. 105 Suppl 1, 11490–11497. doi: 10.1073/pnas.0802290105
Sax D. F., Gaines S. D., Brown J. H. (2002). Species invasions exceed extinctions on islands worldwide: a comparative study of plants and birds. Am. Naturalist 160, 766–783. doi: 10.1086/343877
Schoenborn A. A., Yannarell S. M., MacVicar C. T., Barriga-Medina N. N., Bonham K. S., Leon-Reyes A., et al. (2023). Microclimate is a strong predictor of the native and invasive plant-associated soil microbiome on San Cristóbal Island, Galápagos archipelago. Environ. Microbiol. 25 (8), 1377–1392. doi: 10.1111/1462-2920.16361
Sebastian P., Schaefer H., Renner S. S. (2010). Darwin’s Galapagos gourd: providing new insights 175 years after his visit. J. Biogeogr. 37, 975–980. doi: 10.1111/j.1365-2699.2010.02270.x
Seguel M., Gottdenker N. (2017). The diversity and impact of hookworm infections in wildlife. Int. J. Parasitol. Parasites Wildl. 6, 177–194. doi: 10.1016/j.ijppaw.2017.03.007
Sepúlveda M. A., Seguel M., Alvarado-Rybak M., Verdugo C., Muñoz-Zanzi C., Tamayo R. (2015). Postmortem findings in four south American sea lions (Otaria byronia) from an urban colony in Valdivia, Chile. J. Wildl Dis. 51, 279–282. doi: 10.7589/2013-07-161
Shapiro K., Bahia-Oliveira L., Dixon B., Dumètre A., de Wit L. A., VanWormer E., et al. (2019). Environmental transmission of Toxoplasma gondii: Oocysts in water, soil and food. Food Waterborne Parasitol. 15, e00049. doi: 10.1016/j.fawpar.2019.e00049
Sharma S., Hinds L. A. (2012). Formulation and delivery of vaccines: Ongoing challenges for animal management. J. Pharm. Bioallied Sci. 4, 258–266. doi: 10.4103/0975-7406.103231
Silberglied R. (1972). The little fire ant, wasmannia auropunctata, a serious pest in the Galápagos islands. Notic. Galápagos. 19, 13–15.
Silva L. A., Dermody T. S. (2017). Chikungunya virus: epidemiology, replication, disease mechanisms, and prospective intervention strategies. J. Clin. Invest. 127, 737–749. doi: 10.1172/JCI84417
Sinclair B. J. (2017). “CDF checklist of Galápagos flies,” in Charles Darwin Foundation Galápagos Species Checklist, vol. 45 . Eds. Bungartz F., Herrera H., Jaramillo P., Tirado N., Jiménez-Uzcátegui G., Ruiz D., Guézou A., Ziemmeck F. (Charles Darwin Foundation, Puerto Ayora, Galápagos).
Smith G. T. C. (1979). Looking Back on Twenty Years of the Charles Darwin Foundation Vol. 30 (Charles Darwin Foundation. Noticias De Galápagos), 5–13.
Smith A. W., Boyt P. M. (1990). Caliciviruses of ocean origin: a review. J. Zoo Wildl. Med. 21, 3–23. doi: 10.3201/eid0401.980103
Snell H. L., Powell G., Tye A., Bensted-Smith R., Bustamante R. H., Branch G. M. (2002). “Approach to projecting the future of Galápagos biodiversity,” in A biodiversity vision for the Galápagos Islands (Charles Darwin Foundation and World Wildlife Fund, Puerto Ayora, Galápagos).
Snow D. W. (1964). The giant tortoises of the Galapagos islands. their present status and future chances. Oryx. 7, 277–290. doi: 10.1017/S0030605300003458
Soos C., Padilla L., Iglesias A., Gottdenker N., Bédon M. C., Rios A., et al. (2008). Comparison of pathogens in broiler and backyard chickens on the Galápagos Islands: implications for transmission to wildlife. Auk. 125, 445–455. doi: 10.1525/auk.2008.125.issue-2
Stanton J. B., Brown C. C., Poet S., Lipscomb T. P., Saliki J., Frasca S. Jr. (2004). Retrospective differentiation of canine distemper virus and phocine distemper virus in phocids. J. Wildl Dis. 40, 53–59. doi: 10.7589/0090-3558-40.1.53
Steadman D. W., Stafford T. W., Donahue D. J., Jull A. J. T. (1991). Chronology of holocene vertebrate extinction in the Galapagos islands. Quarternary Res. 36, 126–133. doi: 10.1016/0033-5894(91)90021-V
Stephen C., Wilcox A., Sine S., Provencher J. (2023). A reimagined One Health framework for wildlife conservation. Res. Directions: One Health 1, E12. doi: 10.1017/one.2023.2
Stokstad E. (2023). Deadly avian flu reaches Galápagos islands. Science. Available at: https://www.science.org/content/article/deadly-avian-flu-reaches-Galápagos-islands.
Sutton D. A., Marín Y., Thompson E. H., Wickes B. L., Fu J., García D., et al. (2013). Isolation and characterization of a new fungal genus and species, Aphanoascella Galápagosensis, from carapace keratitis of a Galápagos tortoise (Chelonoidis nigra microphyes). Med. Mycol. 51, 113–120. doi: 10.3109/13693786.2012.701767
Szymańska-Czerwińska M., Mitura A., Niemczuk K., Zaręba K., Jodełko A., Pluta A., et al. (2017). Dissemination and genetic diversity of chlamydial agents in Polish wildfowl: isolation and molecular characterisation of avian Chlamydia abortus strains. PloS One 12, e0174599. doi: 10.1371/journal.pone.0174599
Tapia W. (1997). Estado actual y distribución estacional de las tortugas gigantes (Geochelone elephantopus spp.) de Cinco Cerros, volcan, Cerro Azul, Isla Isabela, Galapagos, Ecuador. [‘Current status and seasonal distribution of giant tortoises (Geochelone elephantopus spp.) from Cinco Cerros, volcano, Cerro Azul, Isabela Island, Galápagos, Ecuador’]. Ibarra, Ecuador: Thesis, Universidad Tecnica del Norte.
Thaller M. C., Migliore L., Marquez C., Tapia W., Cedeño V., Rossolini G. M., et al. (2010). Tracking acquired antibiotic resistance in commensal bacteria of Galápagos land iguanas: no man, no resistance. PloS One 5, e8989. doi: 10.1371/journal.pone.0008989
Thomas S. E., Evans H. C., Cortat G., Koutsidou C., Day M. D., Ellison C. A. (2021). Assessment of the microcyclic rust Puccinia lantanae as a classical biological control agent of the pantropical weed Lantana camara. Biol. Control. 160, 104688. doi: 10.1016/j.biocontrol.2021.104688
Tirira D. G., Weksler M. (2019a). Nesoryzomys darwini (The IUCN Red List of Threatened Species). doi: 10.2305/IUCN.UK.2019-3.RLTS.T14706A22390382.en
Tirira D. G., Weksler M. (2019b). Nesoryzomys indefessus (The IUCN Red List of Threatened Species). doi: 10.2305/IUCN.UK.2019-1.RLTS.T14708A22390443.en
Tompkins E. M., Anderson D. J., Pabilonia K. L., Huyvaert K. P. (2017). Avian pox discovered in the critically endangered waved albatross (Phoebastria irrorata) from the Galápagos islands, Ecuador. J. Wildl Dis. 53, 891–895. doi: 10.7589/2016-12-264
Tonnis B., Grant P. R., Grant B. R., Petren K. (2005). Habitat selection and ecological speciation in Galápagos warbler finches (Certhidea olivacea and Certhidea fusca). Proc. Biol. Sci. 272, 819–826. doi: 10.1098/rspb.2004.3030
Toral-Granda M. V., Causton C. E., Jäger H., Trueman M., Izurieta J. C., Araujo E., et al. (2017). Alien species pathways to the Galápagos Islands, Ecuador. PloS One 12, e0184379. doi: 10.1371/journal.pone.0184379
Tounta D. D., Panagiotis T. T., Tesseromatis C. (2022). Human activities and zoonotic epidemics: A two-way relationship. The case of the COVID-19 pandemic. Glob Sustain 5, e19. doi: 10.1017/sus.2022.18
Townsend C. H. (1925). The Galápagos tortoises in their relation to the whaling industry: A study of old logbooks. Zoologica 4, 55–135. doi: 10.5962/p.203768
Travis E. K., Vargas F. H., Merkel J., Gottdenker N., Miller R. E., Parker P. G. (2006a). Hematology, serum chemistry, and serology of Galápagos penguins (Spheniscus mendiculus) in the Galápagos Islands, Ecuador. J. Wildl Dis. 42, 625–632. doi: 10.7589/0090-3558-42.3.625
Travis E. K., Vargas F. H., Merkel J., Gottdenker N., Miller R. E., Parker P. G. (2006b). Hematology, plasma chemistry, and serology of the flightless cormorant (Phalacrocorax harrisi) in the Galápagos Islands, Ecuador. J. Wildl Dis. 42, 133–141. doi: 10.7589/0090-3558-42.1.133
Trueman M., Atkinson R., Guézou A., Wurm P. (2010). Residence time and human-mediated propagule pressure at work in the alien flora of Galapagos. Biol. Invasions 12, 3949–3960. doi: 10.1007/s10530-010-9822-8
Tye A. (2006). Can we infer island introduction and naturalization rates from inventory data? Evidence from introduced plants in Galápagos. Biol. Invasions 8, 201–215. doi: 10.1007/s10530-004-3574-2
Tye A., Francisco-Ortega J. (2011). “Origins and evolution of Galapagos endemic vascular plants,” in The Biology of Island Floras. Eds. Bramwell D., Caujapé-Castells J. (Cambridge University Press), 89–153.
Tye A., Snell H. L., Peck S. B., Adsersen H. (2002). “Outstanding terrestrial features of the Galápagos archipelago,” in A biodiversity vision for the Galápagos Islands (Charles Darwin Foundation and World Wildlife Fund, Puerto Ayora, Galápagos), 12–23.
Ulloa-Chacon P., Cherix D. (1994). “Perspectives on Control of the Little Fire Ant (Wasmannia auropunctata) on the Galápagos Islands,” in Exotic Ants: Biology, Impact, and Control of Introduced Species. Ed. Williams D. F. (CRC Press, Boca Raton), 63–72. doi: 10.1201/9780429040795-5
UNESCO. (2006). “Joint IUCN/UNESCO Mission Report: Galápagos Islands (Ecuador)”. World Heritage Committee: Thirtieth session. Available online at: https://whc.unesco.org/en/sessions/30COM/documents/ (Accessed 24 March, 2023).
UNESCO. (2007). World Heritage Committee: Thirty-first session. Available online at: https://whc.unesco.org/en/sessions/31COM/documents/ (Accessed 24 March, 2023).
UNESCO. (2023a). Periodic reporting. Available online at: https://whc.unesco.org/en/periodicreporting (Accessed 20 October, 2023).
UNESCO. (2023b). State of conservation reports. Available online at: https://whc.unesco.org/en/soc/4511 (Accessed 20 October, 2023).
UNESCO. (2024). UNESCO provides Avian influenza response in the Galapagos. Available online at: https://whc.unesco.org/en/news/2668 (Accessed 10 August 2024).
Uribe C. (2021). Press release: invasive rodents no longer threaten wildlife on seymour Norte Island and mosquera islet. Available online at: https://www.islandconservation.org/invasive-rodents-no-longer-threaten-wildlife-seymour-norte-island-mosquera-islet/ (Accessed 3 August 2024).
van de Bildt M. W., Kuiken T., Visee A. M., Lema S., Fitzjohn T. R., Osterhaus A. D. (2002). Distemper outbreak and its effect on African wild dog conservation. Emerg. Infect. Dis. 8, 211–213. doi: 10.3201/eid0802.010314
van Dijk P. P., Rhodin A. G. J., Cayot L. J., Caccone A. (2017). Chelonoidis niger (The IUCN Red List of Threatened Species). doi: 10.2305/IUCN.UK.2017-3.RLTS.T9023A3149101.en
van Riper C., van Riper S. C., Goff M. L., Laird M. C. (1986). The epizootiology and ecological significance of malaria on the birds of Hawaii. Ecol. Monogr. 56, 327–344. doi: 10.2307/1942550
VanWormer E., Conrad P. A., Miller M. A., Melli A. C., Carpenter T. E., Mazet J. A. (2013). Toxoplasma gondii, source to sea: higher contribution of domestic felids to terrestrial parasite loading despite lower infection prevalence. Ecohealth 10, 277–289. doi: 10.1007/s10393-013-0859-x
VanWormer E., Mazet J. A. K., Hall A., Gill V. A., Boveng P. L., London J. M., et al. (2019). Viral emergence in marine mammals in the North Pacific may be linked to Arctic sea ice reduction. Sci. Rep. 9, 15569. doi: 10.1038/s41598-019-51699-4
VanWormer E., Miller M. A., Conrad P. A., Grigg M. E., Rejmanek D., Carpenter T. E., et al. (2014). Using molecular epidemiology to track Toxoplasma gondii from terrestrial carnivores to marine hosts: implications for public health and conservation. PloS Negl. Trop. Dis. 8, e2852. doi: 10.1371/journal.pntd.0002852
Vargas H. (1996). What is happening with the avifauna of san cristóbal? Notic. Galápagos. 57, 23–24.
Vasilakis N., Cardosa J., Hanley K. A., Holmes E. C., Weaver S. C. (2011). Fever from the forest: prospects for the continued emergence of sylvatic dengue virus and its impact on public health. Nat. Rev. Microbiol. 9, 532–541. doi: 10.1038/nrmicro2595
Vega-Mariño P., Olson J., Howitt B., Criollo R., Figueroa L., Orlando S. A., et al. (2023). A recent distemper virus outbreak in the growing canine populations of Galápagos Islands: a persistent threat for the endangered Galápagos Sea Lion. Front. Vet. Sci. 10. doi: 10.3389/fvets.2023.1154625
Venn-Watson S., Daniels R., Smith C. (2012). Thirty year retrospective evaluation of pneumonia in a bottlenose dolphin Tursiops truncatus population. Dis. Aquat Organ 99, 237–242. doi: 10.3354/dao02471
Volokhov D. V., Norris T., Rios C., Davidson M. K., Messick J. B., Gulland F. M., et al. (2011). Novel hemotrophic Mycoplasma identified in naturally infected California sea lions (Zalophus californianus). Vet. Microbiol. 149, 262–268. doi: 10.1016/j.vetmic.2010.10.026
Watkins G., Cruz F. (2007). Galápagos at risk: A Socioeconomic Analysis of the Situation in the Archipelago (Puerto Ayora: Province of Galápagos, Ecuador, Charles Darwin Foundation).
Wauters N., Dekoninck W., Hendrickx F., Herrera H. W. (2015). Habitat association and coexistence of endemic and introduced ant species in the Galápagos Islands. Ecol. Entomol. 41, 40–50. doi: 10.1111/een.12256
Wauters N., Dekoninck W., Herrera H. W., Fournier D. (2014). Distribution, behavioral dominance and potential impacts on endemic fauna of tropical fire ant Solenopsis geminata (Fabricius 1804) (Hymenoptera: Formicidae: Myrmicinae) in the Galápagos archipelago. Pan-Pacific Entomologist 90, 205–220. doi: 10.3956/2014-90.4.205
Weaver S. C., Winegar R., Manger I. D., Forrester N. L. (2012). Alphaviruses: population genetics and determinants of emergence. Antiviral Res. 94 (3), 242–257. doi: 10.1016/j.antiviral.2012.04.002
Weksler M., Tirira D. G. (2019). Megaoryzomys curioi (The IUCN Red List of Threatened Species). doi: 10.2305/IUCN.UK.2019-1.RLTS.T136657A22330270.en
Welch D. (2016).Coxiella burnetii. In: Sentinel level clinical laboratory guidelines for suspected agents of bioterrorism and emerging infectious diseases (American Society for Microbiology). Available online at: https://www.asm.org/Articles/Policy/Laboratory-Response-Network-LRN-Sentinel-Level-C (Accessed 10 September 2023).
Wetterer J. K., Porter S. D. (2003). The Little Fire Ant, Wasmannia auropunctata: Distribution, Impact, and Control. Sociobiology 41, 1–41. Available at: https://www.ars.usda.gov/arsuserfiles/60360510/publications/Wetterer_and_Porter-2003(M-3778).pdf.
Wheeler W. M. (1919). The Formicidae of the Harrison Williams Galapagos expedition. Zoologica 5, 101–122.
Wheeler E., Hong P. Y., Bedon L. C., Mackie R. I. (2012). Carriage of antibiotic-resistant enteric bacteria varies among sites in Galápagos reptiles. J. Wildl. Dis. 48, 56–67. doi: 10.7589/0090-3558-48.1.56
Whitehead A. B. R., Butcher G. D., Walden H. S., Duque V., Cruz M., Hernandez J. A. (2018). Burden of exposure to infectious bursal disease virus, infectious bronchitis virus, Newcastle disease virus, Mycoplasma gallisepticum, and intestinal parasites in introduced broiler chickens on the Galápagos. PloS One 13, e0203658. doi: 10.1371/journal.pone.0203658
Whiteman N. K., Goodman S. J., Sinclair B. J., Walsh T., Cunningham A. A., Kramer L. D., et al. (2005). Establishment of the avian disease vector Culex quinquefasciatus SAY 1823 (Diptera: Culicidae) on the Galápagos Islands, Ecuador. Ibis 147, 844–847. doi: 10.1111/j.1474-919X.2005.00468.x
Whitmee S., Haines A., Beyrer C., Boltz F., Capon A. G., de Souza Dias B. F., et al. (2015). Safeguarding human health in the Anthropocene epoch: report of The Rockefeller Foundation–Lancet Commission on planetary health. Lancet Commissions 386, 1973–2028. Available at: https://www.thelancet.com/article/S0140-6736(15)60901-1/.
Wikelski M., Foufopoulos J., Vargas H., Snell H. (2004). Galápagos birds and diseases: invasive pathogens as threats for island species. Ecol. Soc. 9, 5. Available at: http://www.ecologyandsociety.org/vol9/iss1/art5/.
Williams E. S., Thome E. T., Appel M. J. G., Belitsky D. W. (1988). Canine Distemper in black-footed ferrets (Mustela nigripes) from Wyoming. J. Wildlife Diseases 24, 385–398. doi: 10.7589/0090-3558-24.3.385
Williams D. F., Whelan P. M. (1991). Bait attraction of the introduced pest ant, wasmannia auropunctata (Hymenoptera: Formicidae) in the Galápagos islands. J. Entomol. Sci. 27 (1), 29–34.
Wyss J. H. (2000). Screwworm eradication in the Americas. Ann. N Y Acad. Sci. 916, 186–193. doi: 10.1111/j.1749-6632.2000.tb05289.x
Zanella G., Beck C., Valle-Casuso J. C., Anthony M., Cruz M., Vélez A., et al. (2024). Undetection of vector-borne viruses in equids of Galapagos Islands. Front. Veterinary Sci. 11. doi: 10.3389/fvets.2024.1411624
Zapata C. E. (2008). “Evaluation of the Quarantine and Inspection System for Galapagos (SICGAL) after seven years,” in Galapagos Report 2006–2007(Puerto Ayora, Galápagos: Galapagos National Park Service, Charles Darwin Foundation, and the National Institute of Galapagos), 60–66.
Keywords: Galapagos, One Health, planetary health, wildlife, conservation, endemic species, invasive species
Citation: Jimenez IA, Vega-Mariño PA, Villacres T and Houck EL (2024) Review of One Health in the Galápagos Islands (Part 1): historical perspective, invasive species, and emerging infectious diseases. Front. Conserv. Sci. 5:1351707. doi: 10.3389/fcosc.2024.1351707
Received: 06 December 2023; Accepted: 30 August 2024;
Published: 17 October 2024.
Edited by:
Silvio Marchini, University of Oxford, United KingdomReviewed by:
Henrik Lerner, Marie Cederschiöld University, SwedenCristián Bonacic, Pontifical Catholic University of Chile, Chile
Washington Tapia, Galapagos Conservancy, Ecuador
Copyright © 2024 Jimenez, Vega-Mariño, Villacres and Houck. This is an open-access article distributed under the terms of the Creative Commons Attribution License (CC BY). The use, distribution or reproduction in other forums is permitted, provided the original author(s) and the copyright owner(s) are credited and that the original publication in this journal is cited, in accordance with accepted academic practice. No use, distribution or reproduction is permitted which does not comply with these terms.
*Correspondence: Isabel A. Jimenez, aXNhYmVsamltZW5lemR2bUBnbWFpbC5jb20=; Patricio A. Vega-Mariño, cGF0cmljaW9hbGVqYW5kcm8udmVnYW1hcmlub0BnbWFpbC5jb20=
†These authors have contributed equally to this work and share first authorship